Antarctic extreme events
- 1Tremough House, University of Exeter, Penryn, Cornwall, United Kingdom
- 2Department of Geography, Durham University, Durham, United Kingdom
- 3Plymouth Marine Laboratory, Plymouth, Devon, United Kingdom
- 4British Antarctic Survey, Natural Environment Research Council (NERC), Cambridge, United Kingdom
- 5Department of Zoology, University of Johannesburg, Johannesburg, South Africa
- 6Biodiversity of Antarctic and Sub-Antarctic Ecosystems, Santiago, Chile
- 7School of Geography, Politics and Sociology, Newcastle University, Newcastle uponTyne, United Kingdom
- 8WWF UK, The Living Planet Centre, Leeds, United Kingdom
- 9School of Earth and Environment, University of Leeds, Leeds, United Kingdom
- 10Polar Regions Department, UK Foreign and Commonwealth Office, London, United Kingdom
There is increasing evidence that fossil-fuel burning, and consequential global heating of 1.1°C to date, has led to the increased occurrence and severity of extreme environmental events. It is well documented how such events have impacted society outside Antarctica through enhanced levels of rainfall and flooding, heatwaves and wildfires, drought and water/food shortages and episodes of intense cooling. Here, we briefly examine evidence for extreme events in Antarctica and the Southern Ocean across a variety of environments and timescales. We show how vulnerable natural Antarctic systems are to extreme events and highlight how governance and environmental protection of the continent must take them into account. Given future additional heating of at least 0.4°C is now unavoidable (to contain heating to the “Paris Agreement 1.5°C” scenario), and may indeed be higher unless drastic action is successfully taken on reducing greenhouse gas emissions to net zero by mid-Century, we explain it is virtually certain that future Antarctic extreme events will be more pronounced than those observed to date.
Introduction
The past decade has seen a great awareness of increases in the size and frequency of extreme environmental events across a variety of global settings, and the associated consequential damage to lives and livelihoods (Fischer et al., 2021). Many of these events have been attributed primarily to the burning of fossil fuels and the loss of nature. For some time now the science of such attribution has been robust at the level that is needed beyond reasonable doubt (e.g., Otto et al., 2018), which has led to serious efforts to consider ‘loss and damage’ payments from rich developed fossil-fuel-based economies to parts of the world experiencing the effects of extreme events. While much attention has been given to weather-driven events such as heatwaves and rainfall elsewhere in the world, there is yet to be as great an appreciation of the occurrence and impact of extreme events in Antarctica. Here, we open a discussion of Antarctic Extreme Events, focusing on their records across a variety of realms (ocean, atmosphere, cryosphere, biosphere, etc.), indicating their likely causes and suggesting how they may change in future. We do not restrict ourselves solely to those derived from enhanced greenhouse gases, rather we aim to understand a range of ways in which Antarctica has and can experience extreme events and their consequences. In the Antarctic, extreme events are manifested in many ways, including the effects of: climatic extremes such as extreme weather events; catastrophic events such as ice shelf collapse; possible step changes in the environment such as recent sea ice loss; very rapid periods of environmental change and corresponding rapid changes in key biota; and sudden, human-induced direct perturbations, such as the effects of whaling and sealing. By taking an inclusive approach it allows us to understand how and why (relatively) rapid change can occur in Antarctica through high-magnitude low-frequency events of a variety of types.
Atmosphere/weather
The fact that Antarctica is able to maintain a gigantic ice sheet is a consequence of its very cold climate, driven by atmospheric processes. The Antarctic atmospheric system is influenced heavily by the topographic effect of the continent. Weather systems progress clockwise around the continent, with less longitudinal penetration toward the pole than occurs in the Arctic (see Siegert M. et al., 2020). However, that is not to say that weather systems do not move poleward, penetrating inland across Antarctica. These poleward moving cyclones are particularly important for disturbing the otherwise cold and dry weather with warmer and more moist conditions (Sinclair and Dacre, 2019).
Notably, the most extreme ‘heatwave’ ever recorded globally occurred over East Antarctica in March 2022 when surface temperature anomalies of up to 38.5°C were observed (Berkeley Earth, 2022). This event was associated with an ‘atmospheric river’; a long filament-shaped atmospheric structure that carries abundant moisture across large distances (many hundreds of kilometres), leading to extreme localised heat and precipitation. These atmospheric rivers transport heat and moisture from the subtropics into the heart of the Antarctic continent.
Although it was so extreme, a formal attribution of the March 2022 event to human factors has not yet been conducted. However, an attribution analysis of an earlier record-breaking heatwave, that affected the Antarctic Peninsula in February 2020 and led to the highest recorded temperature in the Antarctic mainland (18.3°C at Esperanza Station), concluded a likely significant contribution from fossil-fuel burning (González-Herrero et al., 2023). More research is required to quantify the anthropogenic contribution to the severity of the March 2022 event, and the extent to which we may expect similar events both in the near term and further into the future. Moreover, we must determine whether such large temperature extremes could occur over regions of potentially high impact, such as vulnerable ice shelves.
Other types of extreme weather events have had significant impacts in recent years. Extreme cyclones were implicated in a major iceberg calving event of the Brunt Ice Shelf in 2020 (Francis et al., 2022) and the rapid sea ice decline in the Weddell Sea in 2016/17 (Turner et al., 2020). Although atmospheric rivers and cyclones are key drivers of Antarctic extremes, there is a significant proportion of events that are not associated with either and require other explanations (Hepworth et al., 2022), such as persistent high pressure systems and mid-latitude weather fronts.
Possibly the most recognisable extreme event that occurred in the atmosphere was the loss of stratospheric ozone, discovered above Antarctica in the 1980s. This loss was caused largely by a particular class of chemicals: Chlorofluorocarbons (CFCs). Whilst this event catalysed rapid and effective policy action by the global community in the development of the Montreal Protocol (adopted in 1987), the effects of the “ozone hole” are being felt decades later, with massive increases in ultra-violet radiation still detectable in the Antarctic spring. Recovery of the Antarctic ozone layer later this century is predicted but there are still ongoing effects. These relate not only to biology (discussed below) but also to the climate of Antarctica, where ozone depletion has led to stratospheric cooling and resulting intensification and poleward shift of the belt of westerly winds that encircle Antarctica (Thompson and Solomon, 2002). This change in circulation patterns has led to warming over the Antarctic Peninsula and cooling in parts of East Antarctica (Thompson et al., 2011).
Sea ice
Sea ice is the frozen ocean surface and is an integral part of the Earth system. When present it limits the transfer of heat and gases between the ocean and atmosphere, and reduces ocean flow and mixing. Sea ice growth and melt processes influence ocean salinity, and therefore stratification and global ocean circulation, and the ice itself provides an important ecological niche for many species. In addition, changes in sea ice impact biological production, the transport of biogeochemical properties into the ocean interior and the marine uptake of anthropogenic carbon (Henley et al., 2020). Extreme low sea ice years can provide significant stresses to the marine environment (as discussed later). Sea ice is recognised by the World Meteorological Organisation as one of seven headline Global Climate Indicators which are key to describing the changing climate (WMO, 2023).
Antarctic sea ice extent varies annually between a low of 2–4 million km2, usually in late February, and a September high of 18–20 million km2 (NSIDC, 2023). Accurate measurements of sea ice extent are available from satellite observations dating back to October 1978. During much of the satellite period, total Antarctic sea-ice extent underwent ‘gradual but uneven’ increases (Parkinson, 2019), despite the warming global climate and, by contrast, persistent Arctic sea ice loss (Stroeve and Notz, 2018). Indeed, Antarctic sea ice extent hit a record high in winter 2014. However, it then retreated to a record low in summer 2017 (Turner et al., 2017; Parkinson and DiGirolamo, 2021), and the four lowest annual minimum sea ice extents of the satellite era have occurred since, with both 2022 and 2023 setting new records (Figure 1A; NSIDC, 2023). In 2022, the summer minimum extent dropped to below 2 million km2 for the first time, and winter extent remained at near-record lows for the time of year (Figure 1A). Both the high sea ice extents of 2013–2015 and the low sea ice extents since 2017 lie far outside the observed variability of the baseline period 1981–2010 (Figure 1A), indicating them as ‘extreme’ based on our existing understanding of the system and highlighting crucial yet poorly understood recent increases in year-to-year variability.
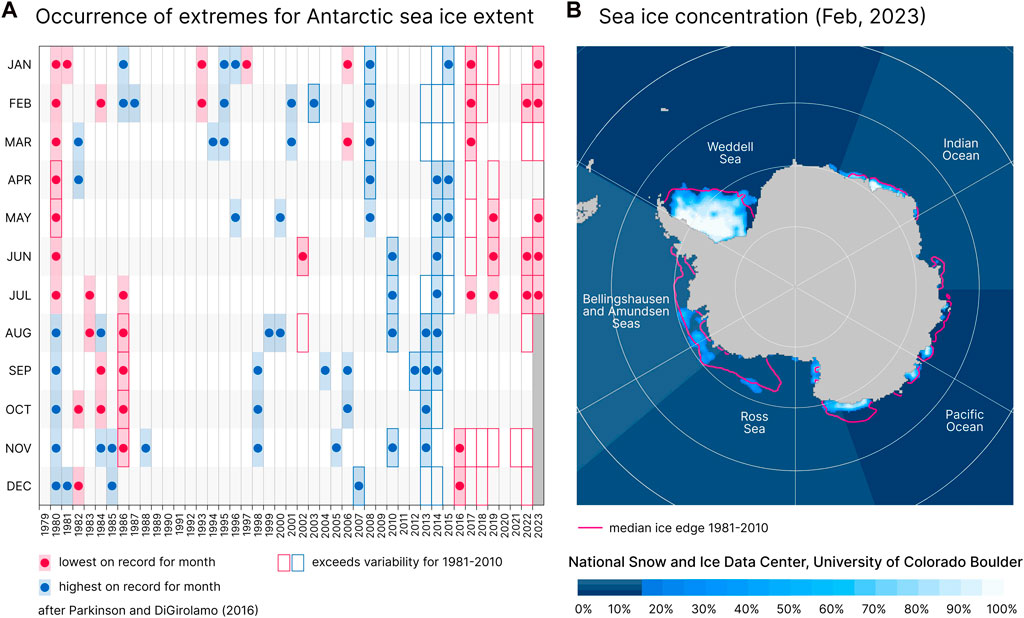
FIGURE 1. Sea ice extremes. (A) Occurrence of record-breaking and extreme months. Months where Antarctic sea ice extent broke the previously observed record for that month are indicated in blue (high) or red (low). Squares indicate extremes, defined as months where anomalies lie outside the 1981–2010 variability for that month (defined as 2-standard-deviations from the mean). Based on Parkinson and DiGirolamo (2016), updated to July 2023. (B) February 2023 monthly-mean sea ice concentration, with the 1981–2010 median overlaid (adapted from NSIDC figure). In this month there was widespread low sea ice cover and a remarkable lack of sea ice in the Ross and Amundsen-Bellinghausen Seas.
Antarctic sea ice loss is not uniform; it varies regionally. In February 2023, the Pacific sector, and Ross and Amundsen-Bellingshausen Seas had near-record low extents with little ice remaining, while the Weddell Sea and Indian sector had less dramatic change, although still lower than the long-term mean (Figure 1B). To truly understand changes in sea ice and its possible vulnerability to further change requires knowledge of sea ice volume and therefore thickness. However, estimating sea ice thickness from satellite data remains challenging, so changes in the volume of sea ice remain poorly resolved. The situation has been stressed in the World Climate Research Program (WCRP) Scientific Committee on Antarctic Research (SCAR) summary report on the International Workshop on Antarctic Sea Ice Thickness: “Sea ice thickness remains arguably the largest single gap in our knowledge of the climate system.”
The low sea ice conditions of the past 7 years certainly begs the question as to whether we are entering a regime where extreme low sea ice extent events become more frequent. Indeed, as in the Arctic where sea-ice loss leads to further absorption of solar heat due to a reduction in surface reflectivity, so too may persistent Antarctic sea ice reductions lead to enhanced warming. Conclusions about the role of human activity in such extremes, and expected future change, rely on reliable climate model simulations. However, coupled climate models have to date almost universally simulated a decrease of Antarctic sea ice cover for the period since 1979, leading to a mismatch with the pre-2017 satellite observations (Roach et al., 2020). This, combined with model biases in the average sea ice extent, has contributed to low confidence in the ability of climate models to simulate sea ice change under various forcing scenarios. Interestingly, the recent loss of sea ice brings long-term trends more in line with climate model simulations, but more analysis is needed before we can better understand the drivers behind this dramatic recent change.
Ocean
In the ocean, extreme temperature events are typically characterised as Marine Heatwaves (MHWs)—extended periods of anomalously high temperatures that can exert significant impacts on marine biodiversity and ecosystems, and the economics of fisheries and other marine industries (Hobday et al., 2016). Although sparser than in some other ocean basins, nineteen such events were documented between 2002 and 2018 in the Southern Ocean, with significant increases in chlorophyll-a concentrations noted in response (Montie et al., 2020). Separately, impacts of MHWs on Southern Ocean diatoms have been recorded, including increased mortality (Samuels et al., 2021). MHWs have increased in frequency globally, though there is strong regional structure and they are thought to have decreased in parts of the Southern Ocean (Oliver et al., 2018). Globally they are predicted to further increase in frequency and intensity in the coming decades, with their effects compounded in some areas by extreme acidity events (Burger et al., 2022), though regional variability and data sparsity challenge projections for the Southern Ocean. Below the sea surface there has been a steady accumulation of heat in the Southern Ocean. This body of heat has been identified as one of the processes responsible for influencing the timing and magnitude of the rapid decrease in sea ice extent subsequent to 2017 (see above; Zhang et al., 2022).
Large-scale horizontal circulation in the Southern Ocean is dominated by the Antarctic Circumpolar Current (ACC). Much energy from the ACC is dispersed in large eddies and can lead to strong local currents. Eddy energy around the Southern Ocean was enhanced at a circumpolar scale in 2000–2002 following a short period of strong winds around 2–3 years previously (Meredith and Hogg, 2006); however, attributing a rising trend in eddy current speeds to the strengthening of Southern Ocean winds is challenged by intrinsic variability in the eddies (Hogg et al., 2022). It was shown recently that these eddies exert a key influence on the intensity and frequency of MHWs via ocean heat flux convergence (Bian et al., 2023), thus potential future increases in eddy energy are likely to be a significant factor in determining extreme ocean temperatures in coming decades.
Changes in the vertical (overturning) circulation in the Southern Ocean are likely less limited by eddies than is the horizontal flow, with important implications for heat and carbon sequestration, both of which are significant for global climate. Consequently, strengthening winds and/or stronger fluctuations in their magnitude are likely to drive proportionately larger changes in overturning flows. Quantifying changes in this component of the global circulation, and characterising its episodic nature and response to extremes in forcing, have become high priorities (Meredith et al., 2019).
Of particular concern are intrusions of water from the mid-depths of the ACC onto the Antarctic continental shelves. Such waters can penetrate across the shelves, especially where glacially-scoured canyons offer a preferred route. This Circumpolar Deep Water (CDW) is markedly warmer than water beneath ice shelves and proximal to marine-terminating glaciers. Hence, an influx of CDW has been identified as critical for melting ice shelves and glaciers in West Antarctica, including the rapidly-retreating Pine Island Glacier, Thwaites Glacier and numerous smaller glaciers along the western coast of the Antarctic Peninsula (Rignot et al., 2014; Cook et al., 2016). Changing wind forcing of ocean flow across the shelf break, including a strengthening anthropogenically-driven component, has been noted as critical (Holland et al., 2019). Thus far, there is comparatively less evidence for changing ocean circulation having influenced the “cold” sector glaciers and ice shelves around Antarctica, such as in the Weddell and Ross Seas. Numerical simulations indicate that these can be susceptible to extreme changes in ocean flows, however, with pronounced sudden consequences for ice shelf and ice sheet stability (Hellmer et al., 2012; Ross et al., 2012), though recent analyses highlight their comparative insensitivity for the near-term future (Naughten et al., 2021).
Ice shelves
Ice shelves fringe three-quarters of Antarctica’s coastline, providing buttressing support that stabilises the rate of ice flow from the grounded ice sheet and its contribution to global sea level (Siegert M. J. et al., 2020) (see Figure 2A for an overview of the ice shelf system). Over the past 5 decades, satellites have observed the retreat, thinning and disintegration of Antarctic ice shelves (Paolo et al., 2015), with change concentrated in two key sectors of the continent.
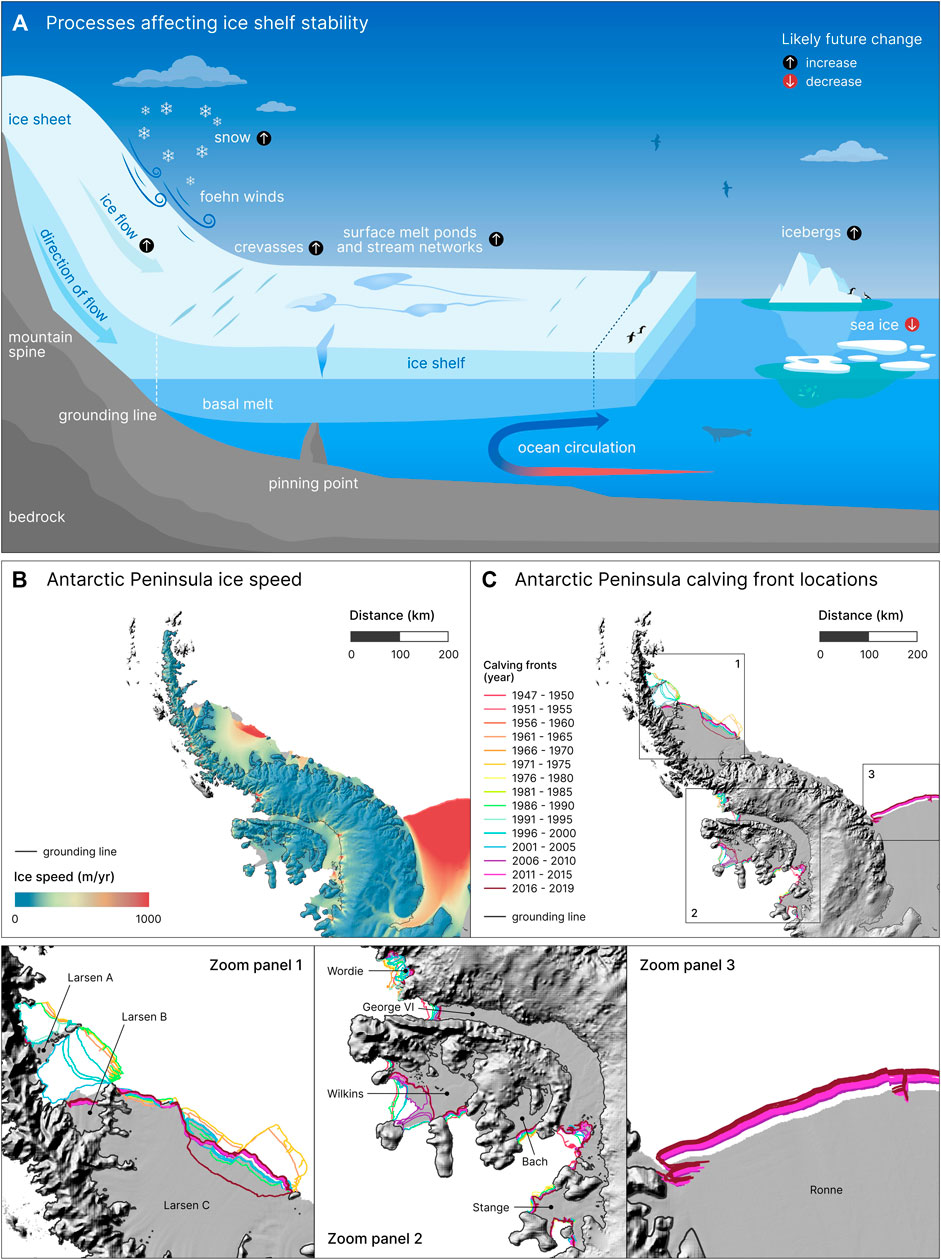
FIGURE 2. Ice Shelf processes and evolution. (A) Schematic showing the processes affecting ice-shelf stability including ice flow, damage, iceberg calving, contact with pinning points, and sea ice. Environmental forcing mechanisms that impact ice shelves are indicated including: ocean circulation and warming, which drive basal melt rates and snowfall; air temperature and winds, which drive surface melt and melt pond formation; change in ice shelf and sea ice extent; and freshwater release into the ocean from iceberg melt, impacting polar ecology through nutrient input to the ocean and algae blooms that act as a source of food for penguins, seals and other marine and bird life. We offer suggestions as to the likely change in cryospheric features under continued climate heating. (B) Mean ice velocity on the Antarctic Peninsula measured from intensity tracking of Sentinel-1a/b SAR images between 2014 and 2022 (Davison et al., 2023), with the ice sheet grounding line location also shown (black line) (Rignot et al., 2016). (C) Annual change in ice shelf calving front position on the Antarctic Peninsula from the 1950’s to 2019 (Cook and Vaughan, 2010; Andreasen et al., 2023). Three panels, covering the Larsen A, B and C ice shelves, the Wordie, George VI and Wilkins ice shelves, and the Ronne Ice Shelf are expanded.
On the Antarctic Peninsula, ice shelves have retreated on average over the last 50 years, with large sections of the Larsen-A, Larsen-B, and Wilkins ice shelves collapsing catastrophically in 1995, 2002, and 2008, respectively (Figure 2B, C). Following a period of relative stability in the 1990s, the collapse of the Larsen-B Ice Shelf was triggered by widespread meltwater ponding on the ice shelf surface where crevasse hydrofractures caused pressure-driven disintegration of the shelf in a matter of days (Scambos and Hulbe, 2000). In the austral summer of 2019/20 high levels of surface melting were observed across the Antarctic Peninsula. If such events become more frequent in a warming climate further incidences of ice shelf disintegration may occur. In West Antarctica, dynamic imbalance is driven by incursions of warm modified CDW melting the floating ice shelves (see above), with the interannual and long-term variability of ocean temperatures linked to atmospheric forcing associated with the El Niño-Southern Oscillation (ENSO) (Jenkins et al., 2018).
Since 2009, major iceberg calving events have occurred across the continent on ten Antarctic ice shelves, including the Larsen-C, Wordie and Wilkins ice shelves on the Peninsula, Thwaites and Pine Island Glaciers in West Antarctica, and Nansen, Mertz, Brunt, Amery and Conger ice shelves in East Antarctica. Recent studies have suggested that a complex link between atmospheric and ocean processes may have a role to play in ice shelf stability and calving. Extreme atmospheric conditions drive strong winds that can affect ocean swell, which may have had a role in triggering recent iceberg calving (Francis et al., 2021) and historical ice shelf collapse (Massom et al., 2018). Whilst iceberg calving is part of the normal process of mass loss, if calving frequency changes over time it can require decades of regrowth to replace the lost ice, and therefore may be an indicator of longer-term change. It is now clear that ice shelves can respond to change over short timescales and that long data records are required to disentangle natural variability from longer term more permanent change.
Land ice
New satellite remote sensing datasets have revealed meltwater to be present on the surface of the Antarctic Ice Sheet, far inland of the grounding line (Stokes et al., 2019). In addition to the supraglacial lakes that form because of surface melting, meltwater has also been observed ponding within firn aquifers, also upstream of the Antarctic grounding line (Bell et al., 2018). Supraglacial and firn stores of meltwater have been related to weather and climate-driven events, such as atmospheric rivers, foehn and katabatic winds, rainfall and cloud cover (Bell et al., 2018).
Surficial meltwater has potential to impact ice flow through hydrological connections with the ice-bed interface. In Greenland, these connections are well established; here, surface meltwater drainage events have been directly related to accelerations in surface ice flow speeds, due to a decrease in basal friction and an increase in sliding (Joughin et al., 2008; Moon et al., 2015). On the Antarctic Peninsula, short term accelerations in ice flow have been observed and related to surface melt events (Tuckett et al., 2019; Wallis et al., 2023), though ocean forcing has also been implicated (Boxall et al., 2022). Firn aquifers may act to dampen the signal and buffer the response (Wallis et al., 2023) but may also play an important role in transmitting surface melt to the ice-bed interface (Bell et al., 2018). Surface meltwater and firn aquifers can also drive cryohydrologic heating through the release of latent heat, changing ice rheology and impacting glacier mass balance (Bell et al., 2018).
The abrupt removal of ice shelves (see above) also impacts ice flow (Figure 2C). Ice shelves buttress, or restrain, their tributary grounded glaciers, through drag forces at their lateral margins and basal stresses where ice shelves rest on pinning points (Goldberg et al., 2009) (Figure 2A). Incremental reductions in ice-shelf thickness can reduce these stresses, resulting in a near-instantaneous rise in ice flow (Paulo et al., 2015; Gudmundsson et al., 2019). This effect is dramatically exacerbated if ice shelves disintegrate entirely. On the Antarctic Peninsula, the larger tributary glaciers of the Larsen A and B ice shelves have substantially accelerated, retreated and thinned following the respective ice-shelf disintegration events in 1995 and 2002, with impacts continuing for years following ice-shelf removal (Berthier et al., 2012).
Internally-forced extreme events impacting the speed and direction of Antarctic grounded ice flow are also known. The Siple Coast ice streams in West Antarctica are susceptible to internal instabilities (e.g., water piracy and flow switching, changes in basal thermal regimes) with high-magnitude, ice dynamical implications (Retzlaff and Bentley, 1993; Joughin and Alley, 2012). The Kamb Ice Stream was fast flowing until ∼1865 AD, when it became stagnant, causing its upper catchment to subsequently thicken by up to 0.65 ± 0.07 m yr-1 (Pritchard et al., 2009). The shut-down of this ice stream has been debated in glaciology, with one idea being that water supplied to the bed was ‘pirated’ or rerouted to neighbouring Whillans ice stream (Anandakrishnan and Alley, 1997). It is therefore considered to be a feature of ‘natural variability’. However, the significance of this idea, coupled with the known sensitivity of basal water routes to small surface changes (Wright et al., 2008) and the upstream impact of grounding line loss of ice (Payne et al., 2004), is that mass loss at the ice sheet margin could well be the trigger for considerable glaciological change to the ice sheet interior, suggesting it too may be affected by future fossil-fuel burning.
While only around 0.2%–0.4% of the Antarctic continent is exposed above the ice (Burton-Johnston et al., 2016), this proportion is likely to increase with further warming (particularly in the Antarctic Peninsula; Lee et al., 2017) and, in some places such as the Dry Valleys in East Antarctica, the area of exposure could be substantial. Such ice-free areas may act as sources of dust falling on snow and ice, reducing surface reflectivity. Thus, as extreme winds form enhanced supraglacial dust deposits, so increased melting can occur. In the Dry Valleys this can lead to sudden alteration in lake ecosystems (Doran et al., 2008). Dust-driven melting has also been observed in James Ross Island (Kavan et al., 2020) and Seymour Island (Meinander et al., 2022).
Marine biodiversity
To humans visiting Antarctica, perhaps the most obvious examples of the effect of extreme events are on land breeding predators. Periodic “low krill years” at South Georgia (e.g., 1983, 1994 and 2009) are manifested by widespread breeding failures of krill-reliant predators, for example, with many dead fur seal pups observed on beaches (AA pers. obs.) and coincident indices of high temperature anomalies, predator reproductive output and low fisheries catches (Trathan et al., 2021). Likewise extreme events (a combination of extensive fast ice, absence of polynya and highly unusual occurrence of rain saturating the chicks followed by rapid sub-zero temperatures) led to the complete breeding failure of a well-studied colony of 20,000 pairs of Adélie penguins on Ile des Pétrels, near Dumont D'Urville research station, in 2013/14 and again in 2016/17 (Ropert-Coudert et al., 2014; 2018). In these inshore waters large icebergs can also have massive and abrupt restructuring roles due to grounding and scour. In the Ross Sea they also impact sea ice dynamics, fostering abrupt shifts in open water and phytoplankton blooms (Dayton et al., 2019; Kim et al., 2019).
By contrast, in open-ocean systems and lower down the food web, the effects of extreme events such as MHWs are less clear-cut. MHW study in the Southern Ocean is in its infancy (see above), although multiple stressor extreme events of MHWs and increased ocean acidity have been suggested to pose a threat in some high latitude surface water regions (Gruber et al., 2021). However, phytoplankton intraspecific trait diversity is high (Samuels et al., 2021; Bishop et al., 2022), conferring ability to adapt and providing a buffer to help withstand both MHWs and 21st century warming. In addition to acute multi-stress experimentation, studies also need to consider inbuilt resilience of the biota. This can include their acclimation and adaptive potential, refuges such as in the more stable deeper waters and their typically longer, often multi-year generation times that can buffer the effect of an annual breeding failure. Between 2018 and 2022, 42% of emperor penguin colonies (28 of 66 known colonies) likely experienced total or partial breeding failure due to fast ice break up in at least 1 year.
The effects of sudden step-changes in environmental forcing and in the response of the biota are better documented in Southern Ocean ecosystems than those of MHWs or other reversible extremes. In continental shelf systems, sudden ice shelf collapse has major consequences both for open water, enhancing areas with export potential for primary production and allowing colonization of benthic habitats recently supplied with food from above. These dramatic events foster rapid transitions, with colonization by pioneer species (Gutt et al., 2013). For pelagic systems, an important finding has been that periods of extremely rapid change in the environment are not always followed by commensurate shifts in the biota. A good example here is the poleward range shifts of Antarctic biota in response to the rapid warming and ice loss from the Atlantic sector over the last 80 years. While species distribution models might project that their ranges move poleward to track the isotherms to maintain within the same thermal regime, mesozooplankton species distributions maintained the same position and withstood warming (Tarling and Thorpe, 2017). Likewise, the range of Antarctic krill was stable during this rapid warming period but “jumped” abruptly south during the ∼1995–2015 warming hiatus (Atkinson et al., 2022).
In addition to these climate-induced changes, human over-exploitation of marine resources during the 1800s and 1900s has had long-lasting and catastrophic effects on the relative abundance of higher trophic levels. About one million blue and fin whales were removed between 1904 and 1980—part of a massive, global scale depredation of marine mammals to roughly one-fifth of their original biomass (Bar-on et al., 2018). Whilst whaling is the most widely known direct human perturbation to the Southern Ocean food web, it forms part of a sequence of over-exploitation, first of fur seals, then of successive whale species and followed by major unregulated and illegal fishing around the 1960s, which severely perturbed the balance of benthic predators around islands such as South Georgia and Kerguelen, with fish stocks very slow to recover. We are still debating the effects of these massive, human-induced perturbations, particularly over how far these effects have cascaded down the food chain, even to the possibility on impacting the biogeochemical cycling of key nutrients such as iron (Smetacek and Nicol, 2005).
Land biodiversity
Extreme events affecting terrestrial (including freshwater) ecosystems and biodiversity in Antarctica, as elsewhere, take a range of forms both directly relating to elements of climate and integrating with the consequences of other human-induced and natural events. Although trends and magnitudes of changes yet experienced in Antarctica vary widely, in the Antarctic Peninsula/maritime Antarctic region the degree of warming and precipitation change (including widespread change in summer precipitation from snow to rain) experienced since the mid-20th Century is arguably already a combination of multiple extreme events (Siegert et al., 2019a). However, the potentially damaging biological consequences resulting from the simultaneous development of the ozone hole (see above) do not appear to have come to pass (reviewed by Convey and Peck, 2019).
Lakes in Antarctica may be particularly vulnerable to the impacts of climate warming, with magnified rates of response in terms of factors such as the extent and duration of winter ice cover, water temperature, mixing and eutrophication (Butler, 1999; Quayle et al., 2002). Lake and other freshwater ecosystems are particularly understudied and undocumented in Antarctica, with work in this field representing a high conservation priority (Hawes et al., 2023). Many are small, shallow and vulnerable to changes in inflow and outflow, and catastrophic drainage can occur in instances where glacial or permafrost ‘dams’ are lost with melting (e.g., see Rosa et al., 2022).
The inherent flexibility and well-developed stress tolerance of many Antarctic terrestrial biota generally allows them to cope with the magnitude of climatic changes seen to date. In the absence of other anthropogenic influences, such as invasive species introduction, these features even allow them to respond positively as the changed environments and increased availability of ice-free habitat reduce the major environmental limitations they face at present. A hypothesised negative consequence of this warming has been an apparent increase in the occurrence of phytopathogenic fungal diseases in mosses in the maritime Antarctic (Rosa et al., 2020), although this has yet to be rigorously assessed. Some changes, while yet to be formally documented in Antarctic terrestrial habitats, already have clear negative consequences in analogous and often better-studied, High Arctic terrestrial habitats, which give important warnings. These include winter thaws and increasing rain in winter leading to ‘rain on snow’ events, which can encase the soil surface and its vegetation cover in a layer of impenetrable ice, and lead to anoxia in the underlying soil ecosystem and mortality of its contained invertebrate communities (Coulson et al., 2000; Peeters et al., 2019). Changing snow cover and distribution patterns, resulting from changes in precipitation levels and patterns of wind distribution, also lead to altered patterns of vegetation and invertebrate distribution and survival, driven by the ability (or not) to tolerate increased or reduced periods of snow cover and associated changes to the thermal regimes experienced in microhabitats (reviewed by Pedersen et al., 2022; Coulson et al., 2023). Furthermore, it has recently been discovered that extreme snowfall events can lead to large-scale Antarctic seabird breeding failures (Descamps et al., 2023).
Marine organisms that breed or moult onshore, such as penguins and seals, provide an important transfer of nutrients onto land, which can be a major driver of terrestrial biodiversity (Bokhorst et al., 2019). Anthropogenically-driven changes to sea-ice or other controlling factors will not only change where these penguins and seals are distributed, but have direct impacts on terrestrial biota which rely on the nutrient supply. Major anthropogenic extreme events, as described earlier, predate the onset of climate warming, and continue to accelerate in their impacts on Antarctic terrestrial ecosystems today. The uncontrolled overexploitation of marine mammals (fur seals, then whales) completely destabilised the Southern Ocean marine ecosystem. This caused both short-term local peaks and long-term large-scale changes in nutrient inputs and degrees of ‘natural’ disturbance to terrestrial ecosystems. In synergy with subsequent anthropogenic climate-change-driven changes in land-ice extent, this has been followed by increasingly widespread damaging impacts to terrestrial ecosystems and biodiversity (habitat destruction through trampling and over fertilisation). For example, recovering seal populations are now able to spread ever further south into areas of the maritime Antarctic with no known history of their occurrence (Convey and Hughes, 2022).
The increasing footprint of human activity in Antarctica (by national operations and tourism) brings with it analogous threats of extensive terrestrial ecosystem and biodiversity damage (Lee et al., 2022). These are exacerbated by the very small proportion of Antarctica’s area that is ice-free and the clear and very strong preference of national operators to construct and expand their stations in those areas. Certain parts of Antarctica, such as the Fildes Peninsula area of King George Island where a concentration of multiple national operator stations and facilities are present, and the McMurdo area on Ross Island, are now de facto accepted to be ‘brownfield’ sites even if not generally described as such in official literature. This calls into serious question the effectiveness of current Antarctic environmental management protocols (Convey, 2020). Human activities in Antarctica also carry the very well documented risk of introduction of non-native species, and also the movement of native Antarctic species between different distinct biogeographic regions within Antarctica to which they are not native, some of which may then become invasive (Hughes et al., 2019). The central contribution of human activity to this threat, and particularly that of national operators in the region, should not be underestimated. In simple summary, in the entire history of human contact with the Antarctic regions, there are no known instances of a natural colonisation and establishment event in terrestrial Antarctica, and only two proposed cases in the core sub-Antarctic, while human-assisted introductions account for c. 15 and c. 250 instances, respectively (Frenot et al., 2005; Hughes et al., 2015). Studies have confirmed that all classes of visitors to Antarctica, both within national operators and the tourism industry, carry potentially viable biological propagules with them (Chown et al., 2012). Nevertheless, of all introductions since the mid-20th Century (the start of the “scientific era”), the vast majority if not all are most plausibly linked with national operations, which are the major source of cargo arriving on the continent. While the terrestrial groups and species involved are generally small and cryptic, and might therefore be viewed by some as insignificant, some such species can be true ‘ecosystem engineers’, leading to step changes in key ecosystem functions that are currently rate limiting, or even functionally not important, in native Antarctic ecosystems such as decomposition and nutrient cycling (Bartlett et al., 2023) or predation (Lebouvier et al., 2020).
Deeper time extreme events
Ancient (greater than 10,000 years ago) extreme events
The fact that the Antarctic ice sheet grew 34 Million years ago (Ma) and has existed in a persistent state since ∼14 Ma is a consequence of extreme events in deep time. The opening of the Drake Passage, coupled with steadily declining atmospheric CO2 led to a threshold transition from “greenhouse” to “icehouse” with Antarctica being plunged into the deep freeze (DeConto and Pollard, 2003). At ∼14 Ma the Middle Miocene Climate Transition (MMCT) led to expansion of the Antarctic ice sheet, with variability in its size thereafter but not complete ice decay (Levy et al., 2021). The cause of the MMCT has been debated a lot and at present it is thought to be a consequence of atmospheric CO2 drawdown in the ocean combined with alterations in ocean circulation. Although these were stepwise natural phenomena, they show how changes to the environmental backdrop in Antarctica, especially up to and over thresholds, can have profound long-term consequences.
Events of extreme (warmer than present) warmth have been detected in the Antarctic geological record. These past warm periods are policy-relevant as they provide examples of how the ice sheet responded to warmer-than-present global temperatures, comparable to those projected for the coming decades to centuries (IPCC, 2019; 2023). However, using these past warm periods to inform our understanding of ice sheet sensitivity under different atmospheric CO2 levels remains a challenge. This is in part because Earth system boundary conditions were subtly different than today, and the duration and intensity of these past warm periods was highly variable, from 2 million years for the Mid-Miocene Climate Optimum through to 300,000 years for the Mid-Pliocene Warm Period, and a few thousand years (ka) for Quaternary interglacials (Colleoni et al., 2022). Crucially, the Antarctic ice sheet showed significant volume change, and thus global sea-level rise of several metres, even when CO2 levels were 300–400 ppm during Pliocene “super-interglacials,” still less than present-day ∼420 ppm, and much less than projected changes in the 21st century and beyond. Even larger ice sheet changes were apparent at CO2 concentrations greater than 400 ppm such as during the mid-Pliocene and Mid-Miocene (Colleoni et al., 2022).
Holocene (last 10,000 years) extreme events
During our current interglacial (the Holocene) climate has been relatively stable. However, even small fluctuations have had significant impacts in Antarctica: for example, the geological record shows that ice shelves such as the George VI ice shelf collapsed in response to quite modest ocean or atmosphere warming (Bentley et al., 2005).
Our understanding of what land ice did in the past and what it is capable of has developed rapidly in the last few years. We now know that land ice has shown pronounced changes during and since the Last Glacial Maximum. For example, during deglaciation and in the Holocene the grounded ice around West Antarctica and the Antarctic Peninsula retreated at rates as fast as 10 s of metres per day (>10 km/yr) (e.g., Dowdeswell et al., 2020). In addition to lateral retreat there is evidence that, about 8,000 years ago, at least one of the tributaries to the Pine Island Glacier was thinning at >1 m/yr, and sustained this rate for over a century (Johnson et al., 2014). Such rapid retreat and thinning is what is widely predicted for the future of many Antarctic ice sheet outlets by ice sheet models and would likely have significant effects on sea level if, as seems likely, it is repeated in the 21st century and beyond.
Ice sheet layering, measured by radio-echo sounding, reveals information about the past flow conditions in Antarctica. Much like a sedimentary rock, the ice will fold and buckle in response to stresses applied to it, such as occurs within fast-flowing ice streams compared with the slow-flowing ice sheet interior. Examination of such layering shows us that ice flow in both East and West Antarctica is subject to sudden directional and dynamic change. Much like with the 19th Century shut down of the Kamb Ice Stream (see above), such events are considered a consequence of natural ice sheet processes. Examples include flow changes in the Weddell Sea sector of West Antarctica in the mid-Holocene (Siegert et al., 2019b) in central West Antarctica at 1.9 ka (Siegert et al., 2004) and at South Pole after the last glacial maximum (Bingham et al., 2007).
A clear lesson from the geological record is one of dynamism, which reveals that the Antarctic ice sheet is not a static giant frozen in time but rather that it responds sporadically and unpredictably to large-scale environmental change; a lesson we should learn as Antarctica’s environment is changed further because of fossil-fuel burning.
Cascades of extreme events
Several of the extreme events discussed above do not occur in isolation and their impacts can be linked in “cascades”. For example, the atmospheric river in March 2022 that led to record temperatures in central East Antarctica also led to surface warming of land ice and ice shelves on the coast, break-up of sea-ice, and collapse of the Conger Ice Shelf (Lhermitte et al., 2023). Similarly, for the ocean, anthropogenically-driven changes in winds can drive changes in intrusion of relatively warm waters to the margins of the ice sheet and, thus, affect extreme events of land ice and ice shelf loss. Physical and biological impacts can also interact, such as warming and the loss of land ice combining to lead to greater probability of alien species establishment. We cannot rule out future cascades where extreme events may have wide-ranging linked impacts in multiple realms, some of them potentially hard to predict.
Summary and conclusions
Future extreme events
The examples of Antarctic extreme events vary by geography, realm, spatio-temporal range and, importantly, attribution. Whereas it is an open scientific question as to the level some of these events can be attributed to fossil-fuel burning, in the vast majority of cases it is virtually certain that continued greenhouse gas emissions will lead to increases in the size and frequency of events, even if the causes to date cannot be attributed to it (Table 1). This is particularly concerning because enhanced global heating from fossil-fuel burning is inevitable, to at the very least another 0.4°C (to the 1.5°C Paris limit) on top of historical warming, and possibly much higher if we do not take serious immediate action to curtail emissions. Hence, for the reasons we note, Antarctica’s fragile and vulnerable environments may well be subject to considerable stress and damage in future years and decades.
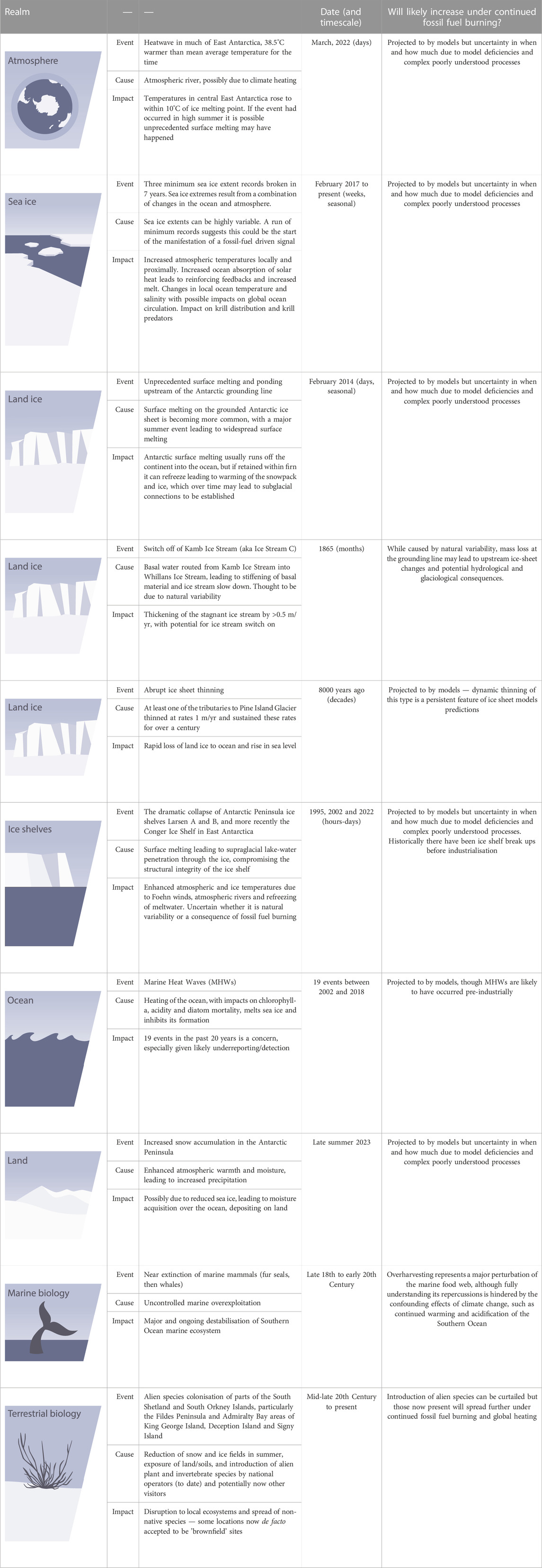
TABLE 1. Examples of Antarctic extreme events. We note some extreme events may be linked in “cascades” such that events in one realm may trigger further events in another.
Since industrialisation (1850) the Antarctic ice sheet has been slow to contribute significantly to global sea level rise but, because of fossil-fuel burning, Antarctica today contributes six times more mass to the ocean than it did just 30 years ago (IMBIE, 2018). It is therefore highly likely that with continued high levels of greenhouse gas emissions global sea level may increase by more than 1 m this century and much more thereafter (Siegert M. J. et al., 2020).
Environmental protection
Human activities in Antarctica contribute little to global carbon emissions, yet many operators are developing net zero targets, in part to signal that the future of Antarctica is linked directly to the global realisation of net zero operations (Lucci et al., 2022). Whilst the Antarctic Treaty System cannot alone prevent future extreme events in Antarctica, it can take measures to seek to reduce further impacts upon Antarctic marine and terrestrial species and ecosystems to withstand and adapt to future change (Njåstad, 2020). Terrestrial and marine protected area tools can be used to minimise additional human stressors on key environments. These include areas newly exposed by ice-loss, or those utilised by marine mammals or native birds whose breeding success can be affected by increased temperatures or increased snow accumulation (Hughes et al., 2021). As sea ice retreats, more areas of Antarctica may become accessible to visitation by ship and careful management will be required to protect vulnerable sites. Biosecurity measures can also help to minimise the risk of non-native species introduction via visiting ships and aircraft and their passengers and cargo (McCarthy et al., 2019; Hughes and Convey, 2022). All Parties to the Protocol on Environmental Protection to the Antarctic Treaty have committed themselves to the comprehensive protection of the Antarctic environment and dependent and associated ecosystems, which has been repeatedly confirmed and reinforced by the Parties, including in the ATCM’s Paris Declaration of 2021 (ATCM XLIII) and its Helsinki Declaration on Climate Change and the Antarctic of 2023 (ATCM XLV). Members of the Commission for the Conservation of Antarctic Marine Living Resources have committed to ensure the conservation of Antarctic marine living organisms, and to the development of a representative system of Antarctic Marine Protected Areas. However, in the face of rapid environmental change and uncertainty, Parties must consider whether existing tools are sufficient to provide the maximum chance that Antarctic ecosystems and species will be able to persist into the future (Wauchope et al., 2019; Sylvester and Brooks, 2020; Teschke et al., 2021; Lee et al., 2022).
Understanding and predicting extreme events in Antarctica requires international scientific collaboration (Kennicutt et al., 2019). As extreme events become more likely, the need to assess their consequences is increasingly urgent (Chown et al., 2022; Hughes et al., 2022). Antarctic change has global implications and, as we have discussed, the effects of human influences on Antarctica are increasingly apparent within the contexts of both global heating and direct interventions. Reducing greenhouse gas emissions to net zero is our best hope of preserving Antarctica, and this must matter to every country—and individual—on the planet.
Author contributions
MS led the review. MB led the past changes section. AA led the marine life section. TB led the atmosphere/weather section. PC led the terrestrial ecosystems section. BD and NR led the land ice section. AH led the ice shelves section and Figure 2. CH and JW led the sea ice section and Figure 1. KH and JR led the governance section. MM led the oceans section. All authors commented and edited the manuscript, and added to Table 1. All authors contributed to the article and approved the submitted version.
Funding
Funding for this work was provided by the UK Foreign, Commonwealth and Development Office (FCDO). CH, JW and MM were funded under NERC project DEFIANT (NE/W004747/1). MB has received funding from the European Research Council (ERC) under the European Union’s Horizon 2020 research and innovation programme (Project INCISED- 885205). PC and KAH are supported by NERC core funding to the BAS “Biodiversity, Evolution and Adaptation” Programme and Environment Office, respectively. AEH was supported by funding from the ESA Polar+ Ice Shelves project (ESA-IPL-POE-EF-cb-LE-2019-834) and the ESA SO-ICE project (ESA AO/1-10461/20/I-NB), which both are part of the ESA Polar Science Cluster. AA was funded jointly by the WWF and by the ESA project Biodiversity in the Open Ocean: Mapping, Monitoring and Modelling (BOOMS) No. 4000137125/22/I-DT.
Acknowledgments
We are very grateful to two referees who offered constructive and helpful comments, which greatly improved the paper.
Conflict of interest
The authors declare that the research was conducted in the absence of any commercial or financial relationships that could be construed as a potential conflict of interest.
Publisher’s note
All claims expressed in this article are solely those of the authors and do not necessarily represent those of their affiliated organizations, or those of the publisher, the editors and the reviewers. Any product that may be evaluated in this article, or claim that may be made by its manufacturer, is not guaranteed or endorsed by the publisher.
References
Anandakrishnan, S., and Alley, R. B. (1997). Stagnation of ice stream C, West Antarctica by water piracy. Geophys. Res. Lett. 24, 265–268. doi:10.1029/96gl04016
Andreasen, J. R., Hogg, A. E., and Selley, H. L. (2023). Change in Antarctic ice shelf area from 2009 to 2019. Cryosphere 17 (5), 2059–2072. doi:10.5194/tc-17-2059-2023
Atkinson, A., Hill, S. L., Reiss, C. S., Pakhomov, E. A., Beaugrand, G., Tarling, G. A., et al. (2022). Stepping stones towards Antarctica: Switch to southern spawning grounds explains an abrupt range shift in krill. Glob. Change Biol. 28, 1359–1375. doi:10.1111/gcb.16009
Bar-on, Y. M., Phillips, R., and Milo, R. (2018). The biomass distribution on Earth. Proc. Natl. Acad. Sci. 115, 6506–6511. doi:10.1073/pnas.1711842115
Bartlett, J. C., Convey, P., Newsham, K. K., Hughes, K. A., and Hayward, S. A. L. (2023). Ecological consequences of a single introduced species to the Antarctic: Terrestrial impacts of the invasive midge Eretmoptera murphyi on signy island. Soil Biol. Biochem. 180, 108965. doi:10.1016/j.soilbio.2023.108965
Bell, R. E., Banwell, A. F., Trusel, L. D., and Kingslake, J. (2018). Antarctic surface hydrology and impacts on ice-sheet mass balance. Nat. Clim. Change 8, 1044–1052. doi:10.1038/s41558-018-0326-3
Bentley, M. J., Hodgson, D. A., Sugden, D. E., Roberts, S. J., Smith, J. A., Leng, M. J., et al. (2005). Early Holocene retreat of the George VI Ice Shelf, Antarctic Peninsula. Geology 33 (3), 173–176. doi:10.1130/G21203.1
Berkeley Earth (2022). Antarctic heatwave: A rapid analysis of the March 2022 dome C record heatwave. Available at: https://berkeleyearth.org/antarctic-heatwave-rapid-attribution-review-dome-c-record/.
Berthier, E., Scambos, T., and Schuman, C. A. (2012). Mass loss of Larsen B tributary glaciers (Antarctic Peninsula) unabated since 2002. Geophys. Res. Lett. 39, L13501. doi:10.1029/2012GL051755
Bian, C., Jing, Z., Wang, H., Wu, L., Chen, Z., Gan, B., et al. (2023). Oceanic mesoscale eddies as crucial drivers of global marine heatwaves. Nat. Commun. 14, 2970. doi:10.1038/s41467-023-38811-z
Bingham, R. G., Siegert, M. J., Blankenship, D. D., and Young, D. A. (2007). Organized flow from the South Pole to the Filchner-Ronne Ice Shelf: An assessment of balance velocities in interior East Antarctica using radio-echo sounding data. J. Geophys. Res. 112, F03S26. doi:10.1029/2006JF000556
Bishop, I. W., Anderson, S. I., Collins, S., and Rynearson, T. A. (2022). Thermal trait variation may buffer Southern Ocean phytoplankton from anthropogenic warming. Glob. Change Biol. 28 (19), 5755–5767. doi:10.1111/gcb.16329
Bokhorst, S., Convey, P., and Aerts, R. (2019). Nitrogen inputs by marine vertebrates drive abundance and richness in Antarctic terrestrial ecosystems. Curr. Biol. 29, 1721–1727.e3. doi:10.1016/j.cub.2019.04.038
Boxall, K., Christie, F. D. W., Willis, I. C., Wuite, J., and Nagler, T. (2022). Seasonal land-ice-flow variability in the Antarctic Peninsula. Cryosphere 16, 3907–3932. doi:10.5194/tc-16-3907-2022
Burger, F. A., Terhaar, J., and Frölicher, T. L. (2022). Compound marine heatwaves and ocean acidity extremes. Nat. Commun. 13 (1), 4722. doi:10.1038/s41467-022-32120-7
Burton-Johnson, A., Black, M., Fretwell, P. T., and Kaluza-Gilbert, J. (2016). An automated methodology for differentiating rock from snow, clouds and sea in Antarctica from Landsat 8 imagery: A new rock outcrop map and area estimation for the entire Antarctic continent. Cryosphere 10, 1665–1677. doi:10.5194/tc-10-1665-2016
Butler, H. G. (1999). Seasonal dynamics of the planktonic microbial community in a maritime Antarctic lake undergoing eutrophication. J. Plankton Res. 21, 2393–2419. doi:10.1093/plankt/21.12.2393
Chown, S. L., Huiskes, A. H. L., Gremmen, N. J. M., Lee, J. E., Terauds, A., Crosbie, K., et al. (2012). Continent-wide risk assessment for the establishment of nonindigenous species in Antarctica. Proc. Natl. Acad. Sci. U.S.A. 109, 4938–4943. doi:10.1073/pnas.1119787109
S. L. Chown, R. I. Leihy, T. R. Naish, C. M. Brooks, P. Convey, B. J. Henleyet al. (Editors) (2022). Antarctic climate change and the environment: A decadal synopsis and recommendations for action (Cambridge, United Kingdom: Scientific Committee on Antarctic Research).
Colleoni, F., De Santis, L., Naish, T. R., DeConto, R. M., Escutia, C., Stocchi, P., et al. (2022). “Past Antarctic ice sheet dynamics (PAIS) and implications for future sea-level change,” in Antarctic climate evolution. Editors F. Florindo, M. Siegert, L. De Santis, and T. Naish 2nd Edition (Elsevier), 806. doi:10.1016/B978-0-12-819109-5.00010-4
Convey, P., and Hughes, K. A. (2022). Untangling unexpected terrestrial conservation challenges arising from the historical human exploitation of marine mammals in the Atlantic sector of the Southern Ocean. Ambio 52, 357–375. doi:10.1007/s13280-022-01782-4
Convey, P., and Peck, L. S. (2019). Antarctic environmental change and biological responses. Sci. Adv. 11, eaaz0888. doi:10.1126/sciadv.aaz0888
Convey, P. (2020). The price of cumulative human activities in the Antarctic. Antarct. Sci. 32, 425. doi:10.1017/S0954102020000577
Cook, A. J., Holland, P. R., Meredith, M. P., Murray, T., Luckman, A., and Vaughan, D. G. (2016). Ocean forcing of glacier retreat in the Western Antarctic Peninsula. Science 353 (6296), 283–286. doi:10.1126/science.aae0017
Cook, A. J., and Vaughan, D. G. (2010). Overview of areal changes of the ice shelves on the Antarctic Peninsula over the past 50 years. Cryosphere 4, 77–98. doi:10.5194/tc-4-77-2010
Coulson, S. J., Convey, P., Schuuring, S., and Lang, S. (2023). Interactions between winter temperatures and duration of exposure may structure Arctic microarthropod communities. J. Therm. Biol. 114, 103499. doi:10.1016/j.jtherbio.2023.103499
Coulson, S. J., Leinaas, H. P., Ims, R. A., and Søvik, G. (2000). Experimental manipulation of the winter surface ice layer: The effects on a High Arctic soil microarthropod community. Ecography 23, 299–306. doi:10.1034/j.1600-0587.2000.d01-1638.x
Davison, B. J., Hogg, A. E., Rigby, R., Veldhuijsen, S., van Wessem, J. M., van den Broeke, M. R., et al. (2023). Sea level rise from West Antarctic mass loss significantly modified by large snowfall anomalies. Nat. Commun. 14, 1479. doi:10.1038/s41467-023-36990-3
Dayton, P. K., Jarrell, S. C., Kim, S., Parnell, P., Thrush, S. F., Hammerstrom, K., et al. (2019). Benthic responses to an Antarctic regime shift: Food particle size and recruitment biology. Ecol. Appl. 29 (1), e01823. doi:10.1002/eap.1823
DeConto, R. M., and Pollard, D. (2003). Rapid Cenozoic glaciation of Antarctica induced by declining atmospheric CO2. Nature 421, 245–249. doi:10.1038/nature01290
Descamps, S., Hudson, S., Sulich, J., Wakefield, E., Grémillet, D., Carravieri, A., et al. (2023). Extreme snowstorms lead to large-scale seabird breeding failures in Antarctica. Curr. Biol. 33 (5), R176–R177. doi:10.1016/j.cub.2022.12.055
Doran, P., McKay, C., Fountain, A., Nylen, T., McKnight, D., Jaros, C., et al. (2008). Hydrologic response to extreme warm and cold summers in the McMurdo Dry Valleys, East Antarctica. Antarct. Sci. 20 (5), 499–509. doi:10.1017/S0954102008001272
Dowdeswell, J. A., Batchelor, C. L., Montelli, A., Ottesen, D., Christie, F. D. W., Dowdeswell, E. K., et al. (2020). Delicate seafloor landforms reveal past Antarctic grounding-line retreat of kilometers per year. Science 368 (6494), 1020–1024. doi:10.1126/science.aaz3059
Fischer, E. M., Sippel, S., and Knutti, R. (2021). Increasing probability of record-shattering climate extremes. Nat. Clim. Chang. 11, 689–695. doi:10.1038/s41558-021-01092-9
Francis, D., Fonseca, R., Mattingly, K. S., Marsh, O. J., Lhermitte, S., and Cherif, C. (2022). Atmospheric triggers of the Brunt Ice Shelf calving in February 2021. J. Geophys. Res. Atmos. 127, e2021JD036424. doi:10.1029/2021JD036424
Francis, D., Mattingly, K. S., Lhermitte, S., Temimi, M., and Heil, P. (2021). Atmospheric extremes caused high oceanward sea surface slope triggering the biggest calving event in more than 50 years at the Amery Ice Shelf. Cryosphere 15, 2147–2165. doi:10.5194/tc-15-2147-2021
Frenot, Y., Chown, S. L., Whinam, J., Selkirk, P., Convey, P., Skotnicki, M., et al. (2005). Biological invasions in the Antarctic: Extent, impacts and implications. Biol. Rev. 80, 45–72. doi:10.1017/s1464793104006542
Goldberg, D., Holland, D. M., and Schoof, C. (2009). Grounding line movement and ice shelf buttressing in marine ice sheets. J. Geophys. Res. Earth Surf. 114, F04026. doi:10.1029/2008jf001227
González-Herrero, S., Vasallo, F., Bech, J., Gorodetskaya, I., Elvira, B., and Justel, A. (2023). Extreme precipitation records in Antarctica. Int. J. Climatol. 43, 3125–3138. doi:10.1002/joc.8020
Gruber, N., Boyd, P. W., Frölicher, T. L., and Vogt, M. (2021). Biogeochemical extremes and compound events in the ocean. Nature 600 (7889), 395–407. doi:10.1038/s41586-021-03981-7
Gudmundsson, G. H., Paolo, F. S., Adusumilli, S., and Fricker, H. A. (2019). Instantaneous Antarctic ice sheet mass loss driven by thinning ice shelves. Geophys. Res. Lett. 46, 13903–13909. doi:10.1029/2019gl085027
Gutt, J., Cape, M., Dimmler, W., Fillinger, L., Isla, E., Lieb, V., et al. (2013). Shifts in Antarctic megabenthic structure after ice-shelf disintegration in the Larsen area east of the Antarctic Peninsula. Polar Biol. 36, 895–906. doi:10.1007/s00300-013-1315-7
Hawes, I., Howard-Williams, C., Gilbert, N., Hughes, K. A., Convey, P., and Quesada, A. (2023). The need for increased protection of Antarctica’s inland waters. Antarct. Sci. 35, 64–88. doi:10.1017/S0954102022000463
Hellmer, H. H., Kauker, F., Timmermann, R., Determann, J., and Rae, J. (2012). Twenty-first century warming of a large Antarctic ice-shelf cavity by a redirected coastal current. Nature 485 (7397), 225–228. doi:10.1038/nature11064
Henley, S. F., Cavan, E. L., Fawcett, S. E., Kerr, R., Monteiro, T., Sherrell, R. M., et al. (2020). Changing biogeochemistry of the Southern Ocean and its ecosystem implications. Front. Mar. Sci. 7, 581. doi:10.3389/fmars.2020.00581
Hepworth, E., Messori, G., and Vichi, M. (2022). Association between extreme atmospheric anomalies over Antarctic sea ice, Southern Ocean polar cyclones and atmospheric rivers. J. Geophys. Res. Atmos. 127, e2021JD036121. doi:10.1029/2021jd036121
Hobday, A. J., Alexander, L. V., Perkins, S. E., Smale, D. A., Straub, S. C., Oliver, E. C. J., et al. (2016). A hierarchical approach to defining marine heatwaves. Prog. Oceanogr. 141, 227–238. doi:10.1016/j.pocean.2015.12.014
Hogg, A. McC., Penduff, T., Close, S. E., Dewar, W. K., Constantinou, N. C., and Martínez-Moreno, J. (2022). Circumpolar variations in the chaotic nature of Southern Ocean eddy dynamics. J. Geophys. Res. Oceans 127 (5). doi:10.1029/2022JC018440
Holland, P. R., Bracegirdle, T. J., Dutrieux, P., Jenkins, A., and Steig, E. J. (2019). West Antarctic ice loss influenced by internal climate variability and anthropogenic forcing. Nat. Geosci. 12 (9), 718–724. doi:10.1038/s41561-019-0420-9
Hughes, K. A., Cavanagh, R. D., and Convey, P. (2022). Advancing Antarctic climate change policy: Upcoming opportunities for scientists and policymakers to work together. Antarct. Sci. 34 (6), 403–407. doi:10.1017/s095410202200044x
Hughes, K. A., and Convey, P. (2022). “Non-native species in Antarctic terrestrial environments: How climate change and increasing human activity are compounding the threat of invasion,” in Invasive species and global climate change (GB: CABI), 95–118.
Hughes, K. A., Convey, P., and Turner, J. (2021). Developing resilience to climate change impacts in Antarctica: An evaluation of Antarctic Treaty System protected area policy. Environ. Sci. Policy 124, 12–22. doi:10.1016/j.envsci.2021.05.023
Hughes, K. A., Pertierra, L. R., Molina-Montenegro, M. A., and Convey, P. (2015). Biological invasions in Antarctica: What is the current status and can we respond? Biodivers. Conservation 24, 1031–1055. doi:10.1007/s10531-015-0896-6
Hughes, K., Convey, P., Pertierra, L. R., Vega, G. C., Aragón, P., and Olalla-Tárraga, M. A. (2019). Human-mediated dispersal of terrestrial species between Antarctic biogeographic regions: A preliminary risk assessment. J. Environ. Manag. 232, 73–89. doi:10.1016/j.jenvman.2018.10.095
IMBIE (2018). Mass balance of the Antarctic Ice Sheet from 1992 to 2017. Nature 558, 219–222. doi:10.1038/s41586-018-0179-y
IPCC (2019). “Summary for policymakers,” in IPCC special report on the ocean and cryosphere in a changing climate. Editors H.-O. Pörtner, D. C. Roberts, V. Masson-Delmotte, P. Zhai, M. Tignor, E. Poloczanskaet al. (Cambridge, UK and New York, NY: Cambridge University Press), 3–35. doi:10.1017/9781009157964.001
IPCC (2023). “Summary for policymakers,” in Climate change 2023: Synthesis report. A report of the intergovernmental panel on climate change. Contribution of working groups I, II and III to the sixth assessment report of the intergovernmental panel on climate change [core writing team. Editors H. Lee, and J. Romero (Geneva, Switzerland: IPCC), 36.
Jenkins, A., Shoosmith, D., Dutrieux, P., Jacobs, S., Kim, T. W., Lee, S. H., et al. (2018). West Antarctic ice sheet retreat in the Amundsen Sea driven by decadal oceanic variability. Nat. Geosci. 11, 733–738. doi:10.1038/s41561-018-0207-4
Johnson, J. S., Bentley, M. J., Smith, J. A., Finkel, R. C., Rood, D. H., Gohl, K., et al. (2014). Rapid thinning of Pine Island Glacier in the early Holocene. Science 343 (6174), 999–1001. doi:10.1126/science.1247385
Joughin, I., and Alley, R. B. (2012). Stability of the West Antarctic Ice Sheet in a warming world. Nat. Geosci. 4, 506–513. doi:10.1038/ngeo1194
Joughin, I., Das, S. B., King, M. A., Smith, B. E., Howat, I. M., and Moon, T. (2008). Seasonal speedup along the western flank of the Greenland Ice Sheet. Science 320, 781–783. doi:10.1126/science.1153288
Kavan, J., Nývlt, D., Láska, K., Engel, Z., and Kňažková, M. (2020). High-latitude dust deposition in snow on the glaciers of James Ross Island, Antarctica. Earth Surf. Process. Landf. 45 (7), 1569–1578. doi:10.1002/esp.4831
Kennicutt, M. C., Bromwich, D., Liggett, D., Njåstad, B., Peck, L., Rintoul, S. R., et al. (2019). Sustained Antarctic research: A 21st century imperative. One Earth 1 (1), 95–113. doi:10.1016/j.oneear.2019.08.014
Kim, S., Hammerstrom, K., and Dayton, P. (2019). Epifaunal community response to iceberg-mediated environmental change in McMurdo Sound, Antarctica. Mar. Ecol. Prog. Ser. 613, 1–14. doi:10.3354/meps12899
Lebouvier, M., Lambret, P., Garnier, A., Convey, P., Frenot, Y., Vernon, P., et al. (2020). Spotlight on the invasion of a carabid beetle on an oceanic island over a 105-year period. Sci. Rep. 10, 17103. doi:10.1038/s41598-020-72754-5
Lee, J. R., Raymond, B., Bracegirdle, T. J., Chades, I., Fuller, R. A., Shaw, J. D., et al. (2017). Climate change drives expansion of Antarctic ice-free habitat. Nature 547, 49–54. doi:10.1038/nature22996
Lee, J. R., Terauds, A., Carwardine, J., Shaw, J. D., Fuller, R. A., Possingham, H. P., et al. (2022). Threat management priorities for conserving Antarctic biodiversity. PLoS Biol. 20, e3001921. doi:10.1371/journal.pbio.3001921
Leihy, R. I., Peake, L., Clarke, D. A., Chown, S. L., and McGeoch, M. A. (2023). Introduced and invasive alien species of Antarctica and the Southern Ocean islands. Sci. Data 10, 200. doi:10.1038/s41597-023-02113-2
Levy, R. H., Dolan, A. M., Escutia, C., Gasson, E. G. W., McKay, R. M., Naish, T. R., et al. (2021). “Antarctic environmental change and ice sheet evolution through the Miocene to Pliocene – A perspective from the Ross Sea and George V to Wilkes land coasts,” in Antarctic climate evolution. Editors F. Florindo, M. Siegert, L. De Santis, and T. Naish second edition (Amsterdam: Elsevier), 389–522.
Lhermitte, S., Wouters, B., and Team, H. (2023). The triggers for Conger Ice Shelf demise: Long-term weakening vs. short-term collapse. Vienna, Austria: EGU General Assembly 2023. 24–28 Apr 2023, EGU23-16400. doi:10.5194/egusphere-egu23-16400
Lucci, J. J., Alegre, M., and Vigna, L. (2022). Renewables in Antarctica: An assessment of progress to decarbonize the energy matrix of research facilities. Antarct. Sci. 34 (5), 374–388. doi:10.1017/s095410202200030x
Massom, R. A., Scambos, T. A., Bennetts, L. G., Reid, P., Squire, V. A., and Stammerjohn, S. E. (2018). Antarctic ice shelf disintegration triggered by sea ice loss and ocean swell. Nature 558, 383–389. doi:10.1038/s41586-018-0212-1
McCarthy, A. H., Peck, L. S., Hughes, K. A., and Aldridge, D. C. (2019). Antarctica: The final frontier for marine biological invasions. Glob. Change Biol. 25 (7), 2221–2241. doi:10.1111/gcb.14600
Meinander, O., Dagsson-Waldhauserova, P., Amosov, P., Aseyeva, E., Atkins, C., Baklanov, A., et al. (2022). Newly identified climatically and environmentally significant high-latitude dust sources. Atmos. Chem. Phys. 22, 11889–11930. doi:10.5194/acp-22-11889-2022
Meredith, M. P., and Hogg, A. M. (2006). Circumpolar response of Southern Ocean eddy activity to a change in the southern annular mode. Geophys. Res. Lett. 33 (16), L16608. doi:10.1029/2006GL026499
Meredith, M., Sommerkorn, M., Cassotta, S., Derksen, C., Ekaykin, A., Hollowed, A., et al. (2019). Polar regions. In IPCC special report on the ocean and cryosphere in a changing climate [H.-O. Pörtner, D. C. Roberts, V. Masson-Delmotte, P. Zhai, M. Tignor, E. Poloczanskaet al. (eds.)] (pp. 203–320). Cambridge University Press, Cambridge, UK and New York, NY, USA.
Montie, S., Thomsen, M. S., Rack, W., and Broady, P. A. (2020). Extreme summer marine heatwaves increase chlorophyll a in the Southern Ocean. Antarct. Sci. 32 (6), 508–509. doi:10.1017/S0954102020000401
Moon, T., Joughin, I., and Smith, B. (2015). Seasonal to multiyear variability of glacier surface velocity, terminus position, and sea ice/ice mélange in northwest Greenland. J. Geophys. Res. Earth Surf. 120, 818–833. doi:10.1002/2015jf003494
Naughten, K. A., De Rydt, J., Rosier, S. H. R., Jenkins, A., Holland, P. R., and Ridley, J. K. (2021). Two-timescale response of a large Antarctic ice shelf to climate change. Nat. Commun. 12 (1), 1991. doi:10.1038/s41467-021-22259-0
Njåstad, B. (2020). An effort to make the impossible possible–managing Antarctica for climate change. Antarct. Aff. 7, 29–44.
NSIDC (2023). Sea_Ice_Index_Monthly_Data_with_Statistics_G02135_vX.x.xlsx. Available at: https://nsidc.org/arcticseaicenews/sea-ice-tools/(accessed March 23, 2023).
Oliver, E. C. J., Donat, M. G., Burrows, M. T., Moore, P. J., Smale, D. A., Alexander, L. V., et al. (2018). Longer and more frequent marine heatwaves over the past century. Nat. Commun. 9 (1), 1324. doi:10.1038/s41467-018-03732-9
Otto, F. E. L., Philip, S., Kew, S., King, A., and Cullen, H. (2018). Attributing high-impact extreme events across timescales—A case study of four different types of events. Clim. Change 149, 399–412. doi:10.1007/s10584-018-2258-3
Paolo, F. S., Fricker, H. A., and Padman, L. (2015). Volume loss from Antarctic ice shelves is accelerating. Science 348, 327–331. doi:10.1126/science.aaa0940
Parkinson, C. L., 2019. A 40-y record reveals gradual Antarctic sea ice increases followed by decreases at rates far exceeding the rates seen in the Arctic. Proc. Natl. Acad. Sci. (PNAS), 116 (29), 14414–14423. doi:10.1073/pnas.1906556116
Parkinson, C. L., and DiGirolamo, N. E. (2016). New visualizations highlight new information on the contrasting Arctic and Antarctic sea-ice trends since the late 1970s. Remote Sens. Environ. 183, 198–204. doi:10.1016/j.rse.2016.05.020
Parkinson, C. L., and DiGirolamo, N. E. (2021). Sea ice extents continue to set new records: Arctic, Antarctic, and global results. Remote Sens. Environ. 267, 112753. doi:10.1016/j.rse.2021.112753
Payne, A. J., Vieli, A., Shepherd, A. P., Wingham, D. J., and Rignot, E. (2004). Recent dramatic thinning of largest West Antarctic ice stream triggered by oceans. Geophys.Res. Lett. 31, L23401. doi:10.1029/2004GL021284
Pedersen, Å. Ø., Convey, P., Newsham, K. K., Mosbacher, J. B., Fuglei, E., Ravolainen, V., et al. (2022). Five decades of terrestrial and freshwater research at Ny-Ålesund, Svalbard. Polar Res. 41, 6310. doi:10.33265/polar.v41.6310
Peeters, B., Pedersen, Å. Ø., Loe, L. E., Isaksen, K., Veiberg, V., Stien, A., et al. (2019). Spatiotemporal patterns of rain-on-snow and basal ice in high Arctic Svalbard: Detection of a climate–cryo. Environmental Research Letters 1, 015002. doi:10.1088/1748-9326/aaefb3
Pritchard, H. D., Arthern, R. J., Vaughan, D. G., and Edwards, L. A. (2009). Extensive dynamic thinning on the margins of the Greenland and Antarctic ice sheets. Nature 461, 971–975. doi:10.1038/nature08471
Quayle, W. C., Peck, L. S., Peat, H., Ellis-Evans, J. C., and Harrigan, P. R. (2002). Extreme responses to climate change in Antarctic lakes. Science 295, 645. doi:10.1126/science.1064074
Retzlaff, R., and Bentley, C. R. (1993). Timing of stagnation of Ice Stream C, West Antarctica, from short-pulse radar studies of buried surface crevasses. J. Glaciol. 39, 553–561. doi:10.1017/s0022143000016440
Rignot, E., Mouginot, J., Morlighem, M., Seroussi, H., and Scheuchl, B. (2014). Widespread, rapid grounding line retreat of Pine Island, Thwaites, Smith, and Kohler glaciers, West Antarctica, from 1992 to 2011. Geophys. Res. Lett. 41 (10), 3502–3509. doi:10.1002/2014GL060140
Rignot, E., Mouginot, J., and Mouginot, B. S. (2016). MEaSUREs Antarctic grounding line from differential satellite radar interferometry, version 2. Boulder, Color. USA. NASA Natl. Snow Ice Data Cent. Distrib. Act. Arch. Cent. Available at: https//doi.org/10.5067/IKBWW4RYHF1Q 02/04/2018.
Roach, L. A., Dörr, J., Holmes, C. R., Massonnet, F., Blockley, E. W., Notz, D., et al. (2020). Antarctic sea ice area in CMIP6. Geophys. Res. Lett. 47, e2019GL086729. doi:10.1029/2019GL086729
Ropert-Coudert, Y., Kato, A., Shiomi, K., Barbraud, C., Angelier, F., Delord, K., et al. (2018). Two recent massive breeding failures in an Adélie penguin colony call for the creation of a marine protected area in D'urville Sea/Mertz. Front. Mar. Sci. 5, 264. doi:10.3389/fmars.2018.00264
Ropert-Coudert, Y., Kato, A., Meyer, X., Pellé, M., MacIntosh, A. J. J., Angelier, F., et al. (2014). A complete breeding failure in an Adélie penguin colony correlates with unusual and extreme environmental events. Ecography 37, 111–113. doi:10.1111/ecog.01182
Rosa, L. H., de Sousa, J. R. P., de Menezes, G. C. A., Carvalho-Silva, M., Convey, P., Câmara, P. E. A. S., et al. (2020). Opportunistic fungi found in fairy rings are present on different moss species in the Antarctic Peninsula. Polar Biol. 43, 587–596. doi:10.1007/s00300-020-02663-w
Rosa, L. H., Ogaki, M. B., Lirio, J. M., Vieira, R., Coria, S. H., Pinto, O. H. B., et al. (2022). Fungal diversity in a sediment core from climate change impacted Boeckella Lake, Hope Bay, north-eastern Antarctic Peninsula assessed using metabarcoding. Extremophiles 26, 16. doi:10.1007/s00792-022-01264-1
Ross, N., Bingham, R. G., Corr, H., Ferraccioli, F., Jordan, T. A., Le Brocq, A., et al. (2012). Steep reverse bed slope at the grounding line of the Weddell Sea sector in West Antarctica. Nat. Geosci. 5, 393–396. doi:10.1038/ngeo1468
Samuels, T., Rynearson, T. A., and Collins, S. (2021). Surviving heatwaves: Thermal experience predicts life and death in a Southern Ocean diatom. Front. Mar. Sci. 8, 600343. doi:10.3389/fmars.2021.600343
Scambos, T. A., Hulbe, C., Fahnestock, M., and Bohlander, J. (2000). The link between climate warming and break-up of ice shelves in the Antarctic Peninsula. J. Glaciol. 46, 516–530. doi:10.3189/172756500781833043
Schaap, T., Roach, M. J., Peters, L. E., Cook, S., Kulessa, B., and Schoof, C. (2020). Englacial drainage structures in an East Antarctic outlet glacier. J. Glaciol. 66, 166–174. doi:10.1017/jog.2019.92
Siegert, M., Bacon, S., Barnes, D., Brooks, I., Burgess, H., Cottier, F., et al. (2020). The Arctic and the UK: Climate, research and engagement. Grantham Institute discussion paper. London, UK: Imperial College London, 7–8. doi:10.25561/80095
Siegert, M. J., Alley, R. B., Rignot, E., Englander, J., and Corell, R. (2020). Twenty-first century sea-level rise could exceed IPCC projections for strong-warming futures. One Earth 3, 691–703. doi:10.1016/j.oneear.2020.11.002
Siegert, M. J., Atkinson, A., Banwell, A., Brandon, M., Convey, P., Davies, B., et al. (2019a). The Antarctic Peninsula under a 1.5°C global warming scenario. Front. Environ. Sci. 7, 102. doi:10.3389/fenvs.2019.00102
Siegert, M. J., Kingslake, J., Ross, N., Whitehouse, P. L., Woodward, J., Jamieson, S. S. R., et al. (2019b). Major ice sheet change in the Weddell Sea sector of West Antarctica over the last 5,000 years. Rev. Geophys. 57, 1197–1223. doi:10.1029/2019rg000651
Siegert, M. J., Welch, B., Morse, D., Vieli, A., Blankenship, D. D., Joughin, I., et al. (2004). Ice flow direction change in interior West Antarctica. Science 305, 1948–1951. doi:10.1126/science.1101072
Sinclair, V. A., and Dacre, H. F. (2019). Which extratropical cyclones contribute most to the transport of moisture in the Southern Hemisphere? J. Geophys. Res. Atmos. 124, 2525–2545. doi:10.1029/2018JD028766
Smetacek, V., and Nicol, S. (2005). Polar ocean ecosystems in a changing world. Nature 437 (7057), 362–368. doi:10.1038/nature04161
Stokes, C. R., Sanderson, J. E., Miles, B. W., Jamieson, S. S., and Leeson, A. A. (2019). Widespread distribution of supraglacial lakes around the margin of the East Antarctic Ice Sheet. Sci. Rep. 9, 13823. doi:10.1038/s41598-019-50343-5
Stroeve, J., and Notz, D. (2018). Changing state of Arctic sea ice across all seasons. Environ. Res. Lett. 13, 103001. doi:10.1088/1748-9326/aade56
Sylvester, Z. T., and Brooks, C. M. (2020). Protecting Antarctica through co-production of actionable science: Lessons from the CCAMLR marine protected area process. Mar. Policy 111, 103720. doi:10.1016/j.marpol.2019.103720
Tarling, G. A., and Thorpe, S. E. (2017). Oceanic swarms of Antarctic krill perform satiation sinking. Proc. R. Soc. B 284, 2017201520172015. doi:10.1098/rspb.2017.2015
Teschke, K., Brtnik, P., Hain, S., Herata, H., Liebschner, A., Pehlke, H., et al. (2021). Planning marine protected areas under the CCAMLR regime–The case of the Weddell Sea (Antarctica). Mar. Policy 124, 104370. doi:10.1016/j.marpol.2020.104370
Thompson, D. W. J., and Solomon, S. (2002). Interpretation of recent southern hemisphere climate change. Science 296, 895–899. doi:10.1126/science.1069270
Thompson, D. W. J., Solomon, S., Kushner, P. J., England, M. H., Grise, K. M., and Karoly, D. J. (2011). Signatures of the Antarctic ozone hole in Southern Hemisphere surface climate change. Nat. Geosci. 4, 741–749. doi:10.1038/NGEO1296
Trathan, P. N., Fielding, S., Hollyman, P. R., Murphy, E. J., Warwick-Evans, V., and Collins, M. A. (2021). Enhancing the ecosystem approach for the fishery for Antarctic krill within the complex, variable, and changing ecosystem at South Georgia. ICES J. Mar. Sci. 78, 2065–2081. doi:10.1093/icesjms/fsab092
Tuckett, P. A., Ely, J. C., Sole, A. J., Livingstone, S. J., Davison, B. J., Melchior van Wessem, J., et al. (2019). Rapid accelerations of Antarctic Peninsula outlet glaciers driven by surface melt. Nat. Commun. 10, 4311. doi:10.1038/s41467-019-12039-2
Turner, J., Guarino, M. V., Arnatt, J., Jena, B., Marshall, G. J., Phillips, T., et al. (2020). Recent decrease of summer sea ice in the Weddell Sea, Antarctica. Geophys. Res. Lett. 47, e2020GL087127. doi:10.1029/2020GL087127
Turner, J., Phillips, T., Marshall, G. J., Hosking, J. S., Pope, J. O., Bracegirdle, T. J., et al. (2017). Unprecedented springtime retreat of Antarctic sea ice in 2016. Geophys. Res. Lett. 44, 6868–6875. doi:10.1002/2017GL073656
Wallis, B. J., Hogg, A. E., van Wessem, J. M., Davison, B. J., and van den Broeke, M. R. (2023). Widespread seasonal speed-up of west Antarctic Peninsula glaciers from 2014 to 2021. Nat. Geosci. 16, 231–237. doi:10.1038/s41561-023-01131-4
Wauchope, H. S., Shaw, J. D., and Terauds, A. (2019). A snapshot of biodiversity protection in Antarctica. Nat. Commun. 10 (1), 946. doi:10.1038/s41467-019-08915-6
WMO (2023). Global climate indicators. Available at: https://gcos.wmo.int/en/global-climate-indicators.
Wright, A. P., Siegert, M. J., Le Brocq, A., and Gore, D. (2008). High sensitivity of subglacial hydrological pathways in Antarctica to small ice sheet changes. Geophys. Res. Lett. 35, L17504. doi:10.1029/2008gl034937
Keywords: extreme weather, polar, glacier, biodiversity, marine ecology, terrestrial ecology, ice shelf
Citation: Siegert MJ, Bentley MJ, Atkinson A, Bracegirdle TJ, Convey P, Davies B, Downie R, Hogg AE, Holmes C, Hughes KA, Meredith MP, Ross N, Rumble J and Wilkinson J (2023) Antarctic extreme events. Front. Environ. Sci. 11:1229283. doi: 10.3389/fenvs.2023.1229283
Received: 26 May 2023; Accepted: 13 July 2023;
Published: 08 August 2023.
Edited by:
Andrea Storto, National Research Council (CNR), ItalyCopyright © 2023 Siegert, Bentley, Atkinson, Bracegirdle, Convey, Davies, Downie, Hogg, Holmes, Hughes, Meredith, Ross, Rumble and Wilkinson. This is an open-access article distributed under the terms of the Creative Commons Attribution License (CC BY). The use, distribution or reproduction in other forums is permitted, provided the original author(s) and the copyright owner(s) are credited and that the original publication in this journal is cited, in accordance with accepted academic practice. No use, distribution or reproduction is permitted which does not comply with these terms.
*Correspondence: Martin J. Siegert, m.siegert@exeter.ac.uk