Research progress on biochar-based material adsorption and removal of ibuprofen
- 1Health Management (Physical Examination) Department of Hubei Third People’s Hospital Affiliated to Jianghan University, Wuhan, China
- 2Wuchang District Shouyilu Street Community Health Service Center, Wuhan, China
Ibuprofen, commonly used for pain relief, inflammation, and to reduce high fever, etc., is a widely available over-the-counter drug. In recent years, due to the excessive use of ibuprofen, its presence in the aquatic environments has shown a significant increasing trend, raising concerns about potential risks to environmental safety, which attracted people’s close attention. Notably, biochar, known as an environmentally friendly functional material, had been widely studied and applied for the removal of ibuprofen in water environments. According to current reports, the adsorption capacity value of biochar for IBP is between 9.69–309 mg/g, and the adsorption mechanism mainly includes π-π stacking, hydrogen bonding, pore filling, etc. In response to this research hotspot, this study reviewed the most recent research progress on the adsorption of ibuprofen using biochar-based materials, including the modified preparation process of biochar and the adsorption mechanism of IBP on various modified biochar surfaces. Additionally, potential challenges and future development directions for the practical applications of biochar were discussed and proposed.
1 Introduction
Ibuprofen (IBP), a widely used non-steroidal anti-inflammatory drug, analgesic and antipyretic medication, is commonly used to treat fever, pain, rheumatoid arthritis and osteoarthritis (Calisici et al., 2023). In recent years, the use of IBP has been steadily increasing due to the growing demand for pain, fever, and inflammation relief. According to statistics, by 2022, the global annual consumption of IBP had surpassed 2 million tons (Farhadi et al., 2020; Gaffar et al., 2020). It’s worth noting that IBP is typically not completely metabolized within the human body, and a significant portion enters the environment through urine and feces (Nourmoradi et al., 2018). Furthermore, a large amount of IBP can also be found in medical waste, expired medications and animal husbandry excrement, which subsequently enter the aquatic environment, exerting a significant impact on the aquatic ecosystem. Although the concentration of IBP in the environment is low, it possessed high solubility and high mobility in water due to the presence of benzene and carboxylic acid functional groups in the IBP molecule (Solubility at 21°C is 0.25 mg/L, pKa = 4.90) (Brillas, 2022). Current wastewater treatment technologies cannot completely remove IBP, which became a novel pollutant in the environment after entering the water environment (Rizvi and Ahammad, 2021). The unutilized IBP in environments accumulated in animals for a long time through the food chain, and subsequently caused further damage to humans and animals, such as destroying the endocrine system of animals and causing damage to the neurons of organisms (Show et al., 2021).
Presently, there is a search for effective methods to remove IBP from aquatic environments. Compared with other technologies, adsorption possessed the advantages of high efficiency (Dison et al., 2022; Georgin et al., 2022), high universality (Jordana et al., 2023a; Vieira et al., 2023) and reproducibility (Jordana et al., 2023b), and exhibited great potential in drug removal from water environments. Notably, biochar, as an environmentally friendly and cost-effective material, had been widely studied. In more detail, biochar is a carbonaceous material prepared by high-temperature pyrolysis of organic materials, such as plant residues and wood, see Figure 1 (Das et al., 2021). Besides, it possessed high porosity, large specific surface area and good adsorption performance, and was widely used in the removal and degradation of environmental pollutants (Zhang et al., 2021). Therefore, using biochar to remove IBP was considered as a potential solution. The use of biochar in water treatment needs to first meet specific requirements, that is, biochar needs to maintain stability in water and not decompose or release harmful substances; be renewable to reduce costs and waste generation; and have good adsorption properties. Therefore, these requirements need to be considered comprehensively when selecting and designing biochar to remove IBP. However, biochar still faced some challenges in removing IBP from water environments, including the impact of the complexity of real wastewater samples on IBP removal, the suitability of adsorbents for treating IBP in real wastewater, the cost effectiveness of adsorbents, and the disposal of adsorbents after use (Ahmed et al., 2023). With the widespread use of over-the-counter ibuprofen, the hazards of residual ibuprofen in the environment attracted widespread attention. Specifically, long-term exposure to ibuprofen resulted in serious harm to aquatic life and ecosystems, so effective removal was required. Biochar, as an efficient adsorbent, exhibited potential application prospects. Here, this article reviewed the modification process of biochar and the adsorption behavior of IBP on the surface of modified biochar, which can provide a reference for the removal of emerging pollutants like IBP by biochar.
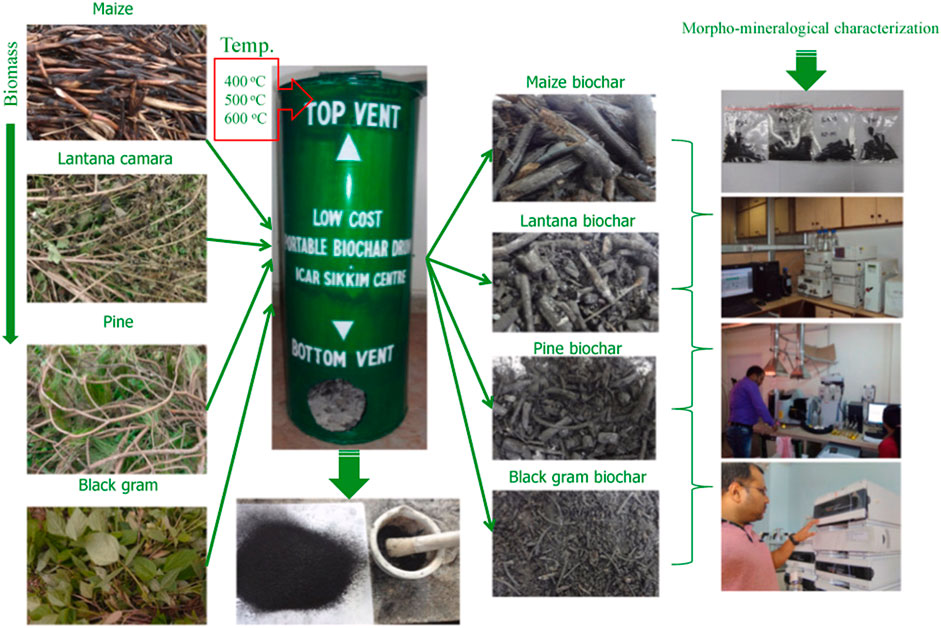
FIGURE 1. Morphological structure of biochar derived from crops, weeds, and trees (Dison et al., 2022).
2 Overview of biochar
Biochar is a cheap, carbon-rich, porous material produced by pyrolysis of biomass. The selection of a suitable carbon source was very critical, which was duo to the fact that its type and properties directly affected the physical/chemical properties of biochar, further affecting its performance. Besides, during the preparation process of biochar, the control of carbonization conditions also had an important impact on the production performance of biochar. In particular, surface modification and functionalization was a common method to improve the performance of biochar, which can obtain specific functions or optimize its own structure by changing the surface properties and chemical composition of biochar.
2.1 Carbon source selection
In the selection process of carbon sources, factors, such as the availability of raw materials, carbon content, carbon source structure and pretreatment, should be given priority. Second, the cheapness, renewable, and easy availability of carbon sources served as economic and sustainable considerations. At present, commonly used raw materials mainly originated from agricultural waste, forest waste, food processing waste, etc. In addition, the carbon content of the carbon source directly determined the carbon content and quality of biochar. Wherein, wood and bamboo were rich in cellulose and lignin, which were ideal carbon sources for biochar production (Li et al., 2023a). Different carbon sources also had significant differences in their chemical structures and components, which would affect the physical and chemical properties, adsorption performance and application scenarios of biochar (see Figure 2). Carbon sources with higher cellulose content usually possessed higher porosity and specific surface area, which were conductive to preparing biochar with efficient adsorption properties (Li et al., 2023b; Zhang et al., 2023). In addition, pretreatment, such as crushing, soaking, drying and other steps, can effectively remove impurities and improve carbonization efficiency, further improving the adsorption capacity of biochar (Habaki et al., 2019). Overall, the selection of carbon sources during biochar preparation should take the above factors into consideration, which would help to obtain high-quality, high-performance biochar.
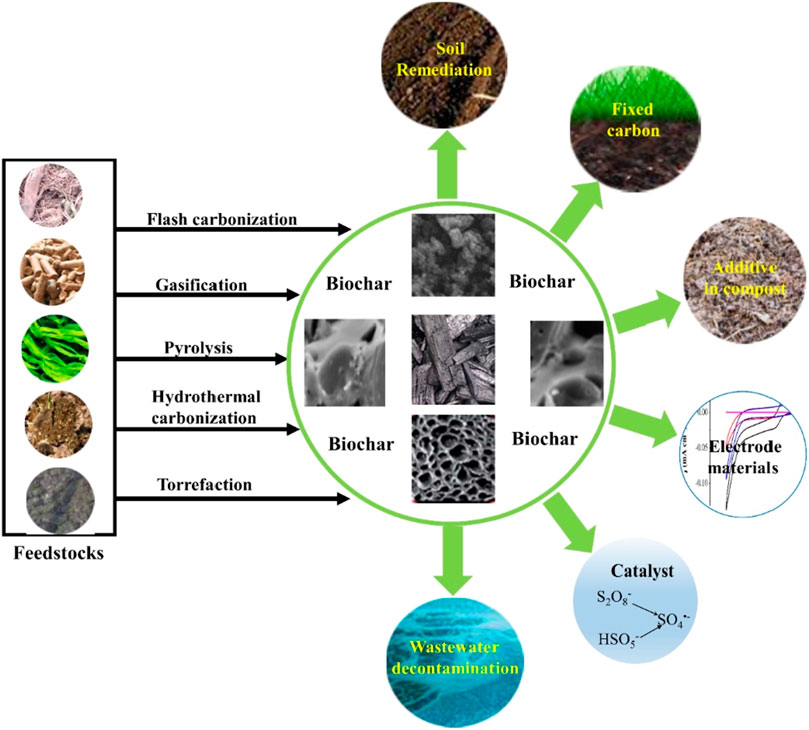
FIGURE 2. Preparation and application of biochar from different carbon sources (Wang and Wang, 2019).
2.2 Carbonization condition control
Reasonable control of carbonization conditions during biochar preparation was the key to obtaining biochar with the required high performance. Adjusting parameters such as carbonization temperature, carbonization rate, and carbonization atmosphere, combined with appropriate additional treatments, can effectively improve the performance of biochar (such as density, structure, conductivity, etc.,). Among them, carbonization temperature was one of the key factors that determined the performance of biochar (Leng and Huang, 2018). The general carbonization temperature range is usually between 400 and 800°C (Cui et al., 2023). Higher carbonization temperatures can promote the carbonization reaction and further increase the carbon content and thermal stability of biochar. Biochar prepared at lower temperatures had abundant functional groups (such as hydroxyl and carboxyl groups), but had lower carbonization degree and thermal stability (Chen et al., 2017). Nevertheless, the carbonization temperature depended on the nature of the raw materials and the physical/chemical properties of the target biochar. Generally speaking, woody raw materials were used to prepare biochar with high carbon content, while cellulosic raw materials (such as straw) were used to prepare biochar with lower carbon content (Min et al., 2023). In addition, the carbonization rate was also an important factor affecting the performance of biochar. Slower carbonization rates was conductive to improve biochar performance, which was ascribed to sufficient carbonization time ensured complete conversion of the feedstock into biochar (Chen et al., 2016). In general, higher carbonization temperature and slower carbonization rate during the carbonization process help to form more microporous and mesoporous structures, thereby increasing the specific surface area and adsorption capacity of biochar. For example, at 650°C using olive tree pruning residues in a continuous screw reactor with a residence time of about 15 min, the biochar yield was 23.38% and the surface area was 341 m2/g (Samer et al., 2022). During the carbonization process, the presence of oxygen may result in a decrease in biochar yield. Therefore, the introduction of an inert atmosphere (such as nitrogen, argon) can effectively inhibit the occurrence of oxidation reactions, which was conductive to obtain biochar with high carbon content and thermal stability (Liao et al., 2022; Zungu et al., 2022). In addition, production time would also affected the physical and chemical properties of biochar (Alivia et al., 2022). Short-term pyrolysis resulted in a decrease in carbon content in biochar. In contrast, prolonged pyrolysis helped remove impurities and volatile substances from the biomass, thereby increasing the carbon content and stability of the biochar. Besides, some additional treatments can be performed during the carbonization process to change the properties of biochar. As reported, adding nanomaterials can change the chemical properties and adsorption characteristics of biochar surfaces, further enhancing the adsorption capability of material (Ahuja et al., 2022). In addition to pyrolysis, there are also biochar preparation methods such as microwave and hydrothermal methods, as shown in Figure 3. Different methods have greatly different structures of prepared biochar. Overall, precise control and optimization of carbonization conditions can obtain biochar with carbon content, thermal stability, pore structure, and physical/chemical properties.
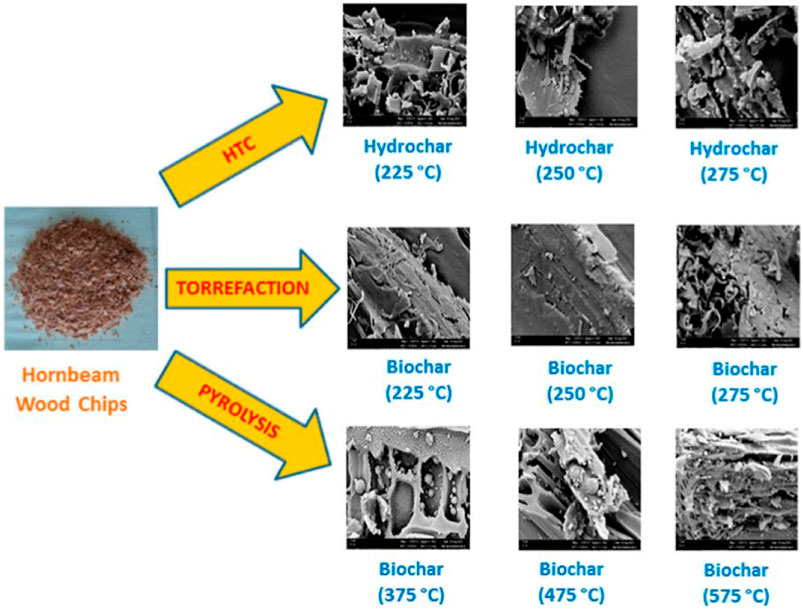
FIGURE 3. Comparative study of biochar preparation by three methods (Betül et al., 2023).
2.3 Surface modification
Surface modification can change the surface properties, pore structure and chemical composition of biochar, thereby giving biochar specific adsorption properties, catalytic properties, ion exchange capabilities and other functions. Modified biochar played an important role in wastewater treatment, gas adsorption, soil improvement and catalytic reactions. In detail, surface modification of biochar included physical modification and chemical modification. Physical modification mainly changed the surface morphology and structural characteristics of biochar through physical methods. As reported, mechanical grinding and ball milling were effective methods to control the particle size and morphology of biochar (Xu et al., 2021). In contrast, chemical modification was a method of introducing new chemical functional groups or changing existing functional groups on the surface of biochar through chemical reactions. Commonly used chemical modification methods included acid-base treatment, oxidant treatment, nitrification treatment, etc, (Wongrod et al., 2018; Zhang et al., 2019). These treatments can effectively improve the chemical reactivity and adsorption capacity of biochar by introducing functional groups, such as hydroxyl, carboxyl, and amino groups. These surface modification methods also changed the surface charge and hydrophilicity of biochar, thereby modulating its interaction with environmental media. It was also beneficial to improve the stability and persistence of biochar in soil, as well as its dispersion and adsorption performance in water. In addition, loading catalysts on the surface of biochar was a common functionalization method. After loading catalysts on the surface of biochar, reactions such as catalytic degradation, catalytic oxidation, and catalytic reduction, can be achieved to accelerate the removal of IBP (Rangarajan et al., 2022; Fan et al., 2023). Currently, used catalysts included metal oxides, metal nanoparticles, etc., Besides, other surface treatment technologies, such as plasma treatment (Mohammed et al., 2021), electron beam irradiation (Croitoru et al., 2014), etc., can also be used to modify the surface of biochar.
2.4 Regeneration of biochar
Currently, the regenerative properties of biochar have been studied and discussed to optimize its sustainable application and reduce costs. Pyrolysis, a common biochar regeneration method, can remove pollutants adsorbed on its surface (Cui et al., 2022). Specifically, the biochar was heated to between 300°C and 700°C to desorb pollutants adsorbed in the pores and effectively restore the biochar’s adsorption performance. However, amounts of energy was consumed during pyrolysis process, so regeneration and usage costs need to be considered together. Also, biochar can be regenerated through acid-base washing, which was a relatively low-cost method (Tariq et al., 2022). Applying an acid-base environment to the surface of biochar can promote the desorption of pollutants and restore the adsorption capacity of biochar. Microorganisms fixed and propagated on the surface of biochar can decompose adsorbed organic pollutants and restore the adsorption performance of biochar. The desorption of contaminants on the surface of biochar can also be promoted by adding appropriate potential (Escudero-Curiel et al., 2021). Nonetheless, the regeneration performance of biochar was still affected by multiple factors, including the type of contaminants adsorbed, the original nature of the biochar, the efficiency of the regeneration method, and the cost of regeneration. Therefore, there was an urgent need to improve regeneration technology to improve the regeneration performance of biochar, reduce economic costs, and promote its wider application.
2.5 Mechanism and practical application of interaction between biochar and pollutants
The interaction mechanisms between biochar and pollutants in water were diverse, including physical adsorption, chemical adsorption, redox and microbial activity. Biochar had a large number of micropores and mesopores, which could absorb dissolved pollutants (Aschermann et al., 2018). In addition, the high specific surface area of biochar also provided more adsorption sites to adsorb various organic and inorganic compounds, such as organic pollutants, heavy metals, nitrogen oxides, etc., (Huang et al., 2022). In addition to physical adsorption, biochar also possessed certain chemical adsorption capabilities. Functional groups on the surface of biochar can react chemically with certain contaminants, forming covalent or hydrogen bonds (Zhou et al., 2019). Biochar can also interact with pollutants through redox reactions. The active functional groups on the surface of biochar promoted chemical reduction or oxidation reactions, converting pollutants into harmless substances (Yuan et al., 2017). In addition, the porous structure of biochar provided a place for microorganisms to grow and provided a stable carbon source, achieving the degradation of organic pollutants.
Previous studies shown that coconut biochar, bamboo biochar and southern yellow pine biochar can remove more than 90% of various pharmaceutical ingredients, such as acetylsalicylic acid, paracetamol, ibuprofen, naproxen, citalopram, carbamazepine and diclofenac (Solanki and Boyer, 2017; Li et al., 2022). In addition, biochar encapsulation method to immobilize microorganisms had been widely used in pollutant removal (Li et al., 2022). Jin et al. (Kim et al., 2019a; Kim et al., 2019b). Used an ultrafiltration activated biochar hybrid system (UF-ABC) to efficiently remove selected target drugs (PhACs), including IBP, 17 α-ethinyl estradiol, and carbamazepine. The results showed that the retention rate of PhACs by UF-ABC was much higher than that of UF system, which was ascribed to the hydrophobic adsorption of biochar. Furthermore, the normalized flux of UF-ABC was significantly better than that of UF-PAC, which was proved to be a suitable alternative to UF-PAC in terms of retention and reduction of fouling. The above applications served as a good reference for biochar in removing residual drugs in water bodies.
2.6 Other technologies for removing pharmaceutical residues from water
Common technologies for removing pharmaceutical residues from water included advanced oxidation processes, biological treatment, reverse osmosis, activated sludge treatment and electrochemical oxidation. Advanced oxidation (such as UV/photocatalysis and ozone treatment) can be used to remove organic pollutants. However, advanced oxidation technology possessed the disadvantages of higher costs and the production of intermediate products (Zhi et al., 2021). Biological treatment was a green and environmentally friendly treatment technology that used microorganisms to degrade drugs into harmless metabolites. Biological treatment was affected by environmental factors (such as temperature and pH), specific microbial communities, and required a lot of time to maintain and manage (Verduzo Garibay et al., 2021). Reverse osmosis technology can efficiently remove drugs dissolved in water, but it also possess the problems of high energy consumption and high operating costs (Ji et al., 2021). Activated sludge treatment removed pharmaceuticals by mixing and activating microorganisms in the sludge and was suitable for wastewater treatment facilities (Wang et al., 2021). Electrochemical oxidation can remove various drugs and micro-pollutants, but it requires power supply, electrode materials and long-term maintenance, which is not conducive to practical applications (Sean et al., 2021). The choice of technology depended on the type of drug, water characteristics, economic factors and environmental goals. Overall, a combination of techniques may be the most effective approach to improve removal efficiency and reduce adverse effects.
2.7 Drug detection and ecotoxicology of drugs in water environment
Drug detection in the water environment was an important part of ensuring water quality safety and ecological environment. With the widespread use of pharmaceuticals and personal care products, pharmaceutical contamination of water bodies had become a global problem. Common drug residues included antibiotics, pain relievers, birth control pills, antidepressants and anti-seizure drugs (Rani et al., 2016). A variety of efficient methods had been developed to detect drug residues in water, such as mass spectrometry, chromatography, spectroscopy and biosensor technology (Aydin et al., 2019).
The ecotoxicology of drugs was an important subject area. Paying attention to the effects of drugs in the natural environment can effectively maintain the stability and biodiversity of the ecosystem. Ecotoxicological studies of drugs included many factors, such as drug concentration, persistence, bioaccessibility, toxicity, bioaccumulation and interactions with environmental factors (Muhammad et al., 2023). These factors influenced the bioavailability and risk of drugs in the environment, resulting in population imbalance or biodiversity loss in ecosystems. To further understand the effects of drugs in ecosystems, a variety of ecotoxicological methods were used for monitoring and assessment, including field sampling, experimental studies and mathematical model analysis (Souza-Silva et al., 2023). The results of monitoring and evaluation was conductive to identify potential risks and take appropriate measures to mitigate adverse effects of drugs on ecosystems. In addition, ecotoxicological studies of drugs was beneficial to develop sustainable drug use and management strategies, which included improving drug design, reducing toxicity, optimizing drug dosage, and developing more environmentally friendly waste disposal methods.
3 Adsorption behavior of IBP on biochar surface
The use of biochar in water treatment requires meeting specific requirements, including biochar stability, excellent adsorption properties and reproducibility (Osman et al., 2022). Therefore, these requirements need to be considered comprehensively in biochar selection and design. The adsorption performance of biochar depended on the reaction conditions, including the raw materials used, kinetic parameters, pH value, specific surface area, etc., Table 1 summarizes the adsorption of IBP by biochar. The adsorption capacity of different biochars for IBP and the conditions for evaluating the adsorption capacity of IBP were shown in Table 1. Currently, sludge (Du et al., 2021), pepper stems (Naima et al., 2022), sugarcane bagasse (Chakraborty et al., 2018), leaves (Yang et al., 2022), and mung bean shells (Mondal et al., 2016) were widely used to prepare biochar to remove IBP from aqueous solutions. Among them, porous biochar obtained from industrial sludge possessed a large specific surface area and an IBP adsorption capacity of 105 mg/g. In another study, the specific surface area of biochar prepared from agave as a precursor was 1,419 m2/g, and the maximum adsorption capacity was 309 mg/g (Du et al., 2021). Moreover, the adsorption process conformed to the Freundlich and Langmuir isothermal adsorption. In addition, the biochar prepared from pine wood had a smaller specific surface area of 1.35 m2/g, but it still showed good IBP adsorption capacity, reaching 22.70 mg/g (Essandoh et al., 2015).
The adsorption and removal of IBP by the biochar adsorption mechanism varied from material to material. The main removal mechanism is shown in Figure 4. Bouzidi et al. (Bouzidi et al., 2023) synthesized biochar by using Erythrina tree pods possessed good porous properties, with a specific surface area of 795.1 m2/g and an average pore volume of 0.422 cm3/g−1. Moreover, the maximum adsorption capacity of IBP reached 96.28 mg/g−1, and IBP was adsorbed through multi-molecule method. Specifically, each functional group on the surface of the adsorbent accommodated more than one molecule at the same time, and it was mainly physical adsorption. Naima et al. (Naima et al., 2022) synthesized ps-biochar material using pepper stems. Experimental results showed that ps-biochar was a mesoporous adsorbent with developed porosity and rich functional groups on the surface, with a Langmuir single-layer adsorption capacity as high as 569.6 mg/g. Its adsorption mechanism was ascribed to π-π interaction, pore filling and hydrogen bonding. Ocampo-Perez et al. (Ocampo-Perez et al., 2019) synthesized biochar by using pepper seeds and showed good IBP adsorption capacity. The results showed that the increase in pyrolysis temperature can promote the increase in disordered graphitic carbon content (51.6%–85.02%) and the decrease in specific surface area (0.52–0.18 m2/g). The adsorption capacity of biochar synthesized at 600°C increased more than 50 times compared to biomass. In addition, the adsorption mechanism under neutral environment was the interaction between π-acceptors and electrostatics, while the adsorption mechanism under alkaline conditions was π-acceptors. Yang et al. (Yang et al., 2022) studied sycamore leaf-derived biochar (p-BC) as an adsorbent to remove IBP from water. The results showed that p-BC possessed a porous structure and abundant hydroxyl groups, and the -OH functional group played a key role in the adsorption process. In a strongly acidic environment (pH = 2), p-BC removed 96.34% of IBP, with a maximum adsorption capacity of 10.4 mg/g. The hydroxyl groups on the surface of p-BC served as donors and acceptors to form hydrogen bonds with IBP and played an important role in its removal. These results indicated that p-BC was an effective and recyclable adsorbent.
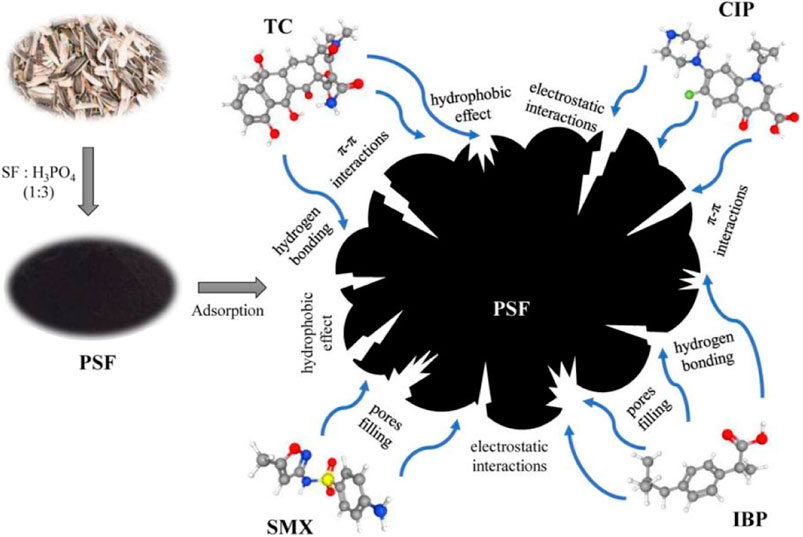
FIGURE 4. The mechanism of biochar to remove IBP and other drug residues (Nguyen et al., 2023).
Biochar prepared from biomass waste can also be used to remove IBP from aqueous environments. Chakraborty et al. (Chakraborty et al., 2018) used sugarcane bagasse as the precursor to prepare steam-activated biochar (SPAB) and chemically activated biochar (SCAB), and the adsorption capacity of IBP reached 13.51 mg/g and 11.90 mg/g. This adsorption process was consistent with the pseudo-second-order kinetic model and the Langmuir/Frendlich isotherm. Ndoun et al. (Ndoun et al., 2023) prepared biochar from cotton gin waste (CG700) and removed IBP from water through fixed bed experiments. The results showed that the removal rate of IBP by CG700 reached 50%. Its adsorption mechanism included pore filling-diffusion, hydrophobic interaction, hydrogen bonding and π-π electron donor-acceptor interaction, see Figure 5. Engelning et al. (Ngernyen et al., 2023) prepared biochar and magnetic biochar (combined with ferromagnetic nanoparticles) from chrysanthemum waste and used it to remove IBP from solution. The results showed that the specific surface areas of non-magnetic biochar and magnetic biochar were 220 m2/g−1 and 194 m2/g−1, and the maximum adsorption capacities for IBP reached 167 mg/g−1 and 140 mg/g−1, respectively. The adsorption process was consistent with the pseudo-second-order kinetic model and the Langmuir-Freundlich isotherm model. In addition, the prepared magnetic biochar overcame the problem of poor separation effect from the liquid phase after adsorption of powdered biochar. Show et al. (Show et al., 2022b) synthesized biochar using tamarind seeds and simulated the removal of IBP in water. The results showed that the maximum adsorption capacity of IBP was 77.51 mg/g, and exhibited good stability during multiple cycle adsorption processes. The above studies demonstrated that biochar prepared from biomass waste can effectively remove IBP present in water as a cost-effective and environmentally friendly alternative.
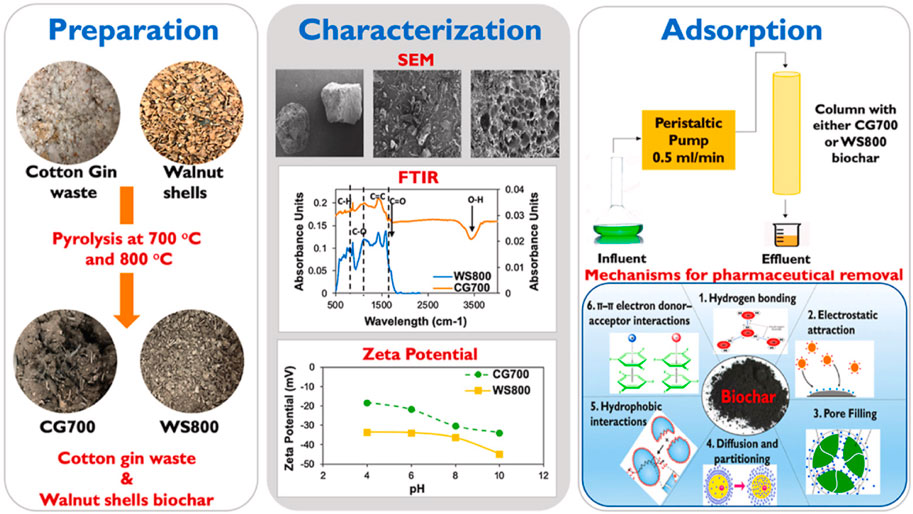
FIGURE 5. Use of cotton ginning waste and walnut shell biochar for removal of pharmaceuticals from aqueous solutions (Ndoun et al., 2023).
Steam activation was an effective strategy to increase the number of active adsorption sites and carbon and oxygen content in adsorbents. Chakraborty et al. (Chakraborty et al., 2020) studied that compared with chemical activation, steam activation can improve the adsorption behavior of date stone biochar for IBP. After 5 cycles, the removal rate of IBP was still as high as 69.54%, and the adsorption process conformed to the Langmuir isotherm and pseudo-second-order kinetic model. Furthermore, at 15°C, the maximum removal rates of unmodified and steam-activated biochar reached 90% and 95%, respectively. The adsorption isotherm of IBP by steam-activated biochar changed from Langmuir to Freundlich, and both processes followed a pseudo-second-order kinetic model. Mondal et al. (Mondal et al., 2016) used steam-activated mung bean shells to prepare biochar, which was a potentially cost-effective adsorbent with a large specific surface area (308 m3/g) and better IBP molecular adsorption capacity (59.76 mg/g).
Chemical activation can also improve the adsorption capacity of biochar for IBP. Jaegwan et al. (Shin et al., 2020) research showed that chemical activation of sodium hydroxide can improve the physical/chemical properties of coffee grounds (SCW) biochar, which was beneficial to the adsorption of the drug IBP. The SCW-enhanced aromatic structure promoted π-π interactions and proceeded spontaneously across the entire range of pH (5.0–11.0) and ionic strength (NaCl: 0–0.125 M). Nguyen et al. (Ngernyen et al., 2023) used phosphoric acid to activate sunflower seed shell biochar to improve its physicochemical properties. The results showed that phosphoric acid increased the specific surface area of biochar (378.8 m2/g) and formed a mesoporous structure, with the maximum adsorption capacity for IBP reaching 251.1 mg/g. The adsorption mechanism of IBP on modified biochar is relatively complex, including chemical adsorption, external diffusion and intra-particle diffusion.
The specific surface area and adsorption properties of biochar can be controlled by changing the pyrolysis temperature (Delgado-Moreno et al., 2021). In detail, higher pyrolysis temperature significantly increases the specific surface area and pH value of biochar, thereby affecting the adsorption capacity (Ocampo-Perez et al., 2019). As reported, the pH value of biochar showed obvious dependence on the adsorption of nonsteroidal antiinflammatory drugs (NSAIDs), especially IBP. Lin et al. (Lin et al., 2017) prepared biochar using pepper (Capsicum annuum) seeds as a precursor. The biochar obtained at lower temperatures possessed more disordered graphitic carbon (51%–85%) and a lower specific surface area (0.52–0.18 m2/g) and fewer functional groups, showing excellent adsorption performance. Alvear-Daza et al. (Alvear-Daza et al., 2022) used H3PO4 (AC-H) and NaOH (AC-N) as activators to activate the synthesized sunflower seed shell biochar. By the optimization of central composite design (CD), the final result was AC-H (H3PO4 in Under the experimental conditions of 80% wt. and 544°C) and AC-N (NaOH at 50% wt. and 300°C), biochar showed the highest specific surface area (S-BET), mesovoid ratio (S meso) The maximum adsorption capacities for IBP and IBP are 23.6 and 4.5 mg/g−1 respectively. During the adsorption process, the presence of the transport pore network was crucial to ensure the accessibility of the internal pores and that the micropores can accommodate IBP molecules.
In addition to common preparation methods, hydrothermal carbonization was a simple and environmentally friendly technology that used relatively low energy and temperature to synthesize biochar in a short time. Hydrochar et al. prepared green tea waste into biochar through hydrothermal carbonization method and showed effective behavior in removing IBP, with a maximum adsorption capacity of 63.69 mg/g (Yudha et al., 2019). Some functional groups on the surface of the adsorbent, such as -OH and -COOH, played a key role in the adsorption process due to their ability to electrostatically interact with the adsorbates. The adsorption process corresponded well to the Langmuir isotherm and pseudo-second-order kinetic model. Delgado-Moreno et al. (Delgado-Moreno et al., 2021) synthesized biochar rich in oxidative functional groups through hydrothermal reaction at low temperature (240°C). Although its specific surface area was only 7.5 m2/g, its adsorption rate of IBP reached 64%.
4 Adsorption behavior of IBP on modified biochar surface
Ball milling was an environmentally friendly and cost-effective physical modification method that had been widely used to improve the adsorption capacity of biochar (Kumar et al., 2020). Compared with non-ball milled biochar, ball-milled biochar prepared under optimal conditions showed a higher removal rate for IBP, which was due to the fact that the electrostatic interaction and porosity of biochar after ball milling were greatly enhanced. Luo et al. (Luo et al., 2020) used ball milling to improve the potential of sawdust-derived biochar to remove IBP from wastewater, reaching a Langmuir maximum adsorption capacity of 132 mg/g. Under optimal ball milling conditions (biochar to ball weight ratio 1:5, 5 h), the negative charge on the biochar surface was significantly reduced, resulting in an increase in the electrostatic attraction between biochar and IBP. Ai et al. (Ai et al., 2020) used orange peel as raw material and prepared methanol-modified ultrafine magnetic biochar (CH3OH-OP-char/Fe3O4) by ball milling method, and used it to evaluate its adsorption behavior for IBP. The results showed that the biochar’s maximum adsorption capacity for IBP was 58.12 mg/g, which was 103% higher than that of untreated biochar. The adsorption mechanism in this adsorption process mainly included single-layer physical adsorption and film diffusion, and was highly dependent on the solution pH.
The adsorption capacity of IBP can also be increased by adding nanoparticles to biochar (Table 2). Li et al. (Liyanage et al., 2020) loaded Fe3O4 nanoparticles on the surface of biochar, which provided unique surface adsorption sites, further improved the IBP adsorption capacity, and facilitated magnetic separation. In addition, Fe-OH groups also promoted adsorption through electrostatic, dipole-dipole, and hydrogen bonding interactions. The Langmuir maximum adsorption capacity of modified biochar treated with NaOH increased to 39.9 mg/g for IBP (Karunanayake et al., 2017). During the adsorption process, Fe-OH groups acted as hydrogen bond donors or acceptors, and strong hydrogen bonds could be formed between IBP and Fe3O4 phases. Liyanage et al. (Liyanage et al., 2020) obtained Fe3O4 nanoparticles from Fe2+/Fe3+ solution through NaOH treatment to modify Douglas fir biochar. Fe3O4 particles in the composite adsorbent were scattered on the surface of biochar, and the adsorption capacity of IBP increased from 17.5 ± 0.4 to 39.9 ± 1.2 mg/g. Moreno-Perez et al. (Moreno-Perez et al., 2021) predicted the adsorption of IBP by ZnAl/biochar composites through a three-dimensional pore volume and surface diffusion model (3D-PVSDM). The results showed that the maximum adsorption capacity of ZnAl/biochar composite for IBP was 1,032.81 mg/g, and the equilibrium adsorption was consistent with the Henry isotherm. Furthermore, 3D-PVSDM simulations indicated that surface diffusion was the preferential transport mechanism during the adsorption of IBP on ZnAl/biochar composites. Nguyen et al. (Van Thom et al., 2021) used peanut shell biomass and potassium ferrate (K2FeO4 0.1 M) to synthesize partially graphitized biochar by a simple strategy for the removal of nonsteroidal anti-inflammatory drug IBP from water. The results showed that the synthesized nanomaterial had the characteristics of both graphitic carbon and porous biochar, a large specific surface area (374.0 m2/g), and a micro/mesoporous structure. Pseudo-second-order, Langmuir and Freundlich models well described the adsorption behavior of IBP on modified biochar. Furthermore, hydrogen bonding interactions may be the main driving force for the attachment of IBP molecules to the adsorbent surface, followed by electrostatic attraction and π–π EDA interactions. Cross-linked magnetic chitosan/activated biochar (CMCAB) was also used for the adsorption and removal of pharmaceutical micropollutants. Mojiri et al. (Mojiri et al., 2019) used artificial neural networks to optimize the adsorption of cross-linked magnetic chitosan/activated biochar, and the optimized cross-linked magnetic chitosan/activated biochar removed 98.8% of IBP at an initial concentration of 2.47 mg/L and pH = 6. The adsorption process was explained by Langmuir and Freundlich isotherms, and desorption tests showed that the cross-linked magnetic chitosan/activated biochar could be used for at least 8 adsorption-desorption cycles.
Chemical modification can also increase the adsorption capacity of biochar for IBP. Currently, acid/alkali (Chakraborty et al., 2018; Shin et al., 2020) modification had been used to improve the adsorption behavior of biochar, including the adsorption behavior of IBP, see Figure 6 (Hassan and Carr, 2021). In detail, acid/base activation was known to consistently produce high-quality biochar with excellent surface properties, which facilitated the interaction between contaminants and biochar. The chemically modified biochar extracted from coconut shells by Li et al. (Li et al., 2018) showed an IBP adsorption capacity of 12.16 mg/g, while the adsorption capacity on physically modified biochar was 9.69 mg/g. Wang et al. (Chakraborty et al., 2020) found that after chemical modification (pH = 6.4) of biochar prepared from jujube pits, the maximum adsorption capacity of IBP reached 13.87 mg/g. The adsorption mechanism was mainly intra-particle diffusion in the solid-liquid phase. Furthermore, similar results were obtained for chemically modified sugarcane biochar with a maximum adsorption capacity of 13.5 mg/g (Chakraborty et al., 2018). Show et al. (Show et al., 2022a) used acid treatment to prepare biochar from tamarind seeds. The adsorption capacity of IBP increased from 8.6 mg/g to 10.5 mg/g, which was consistent with pseudo-second-order adsorption kinetics and Langmuir isotherm model. The competitive adsorption of pollutants (i.e., IBP, naproxen (NAP), and DCF) from wastewater and lake water by NaOH-activated biochar obtained from coffee waste was studied by Shin et al. (Shin et al., 2021). It is worth noting that NaOH activation improved the physicochemical surface properties and aromaticity of biochar, and significantly increased the adsorption capacity of biochar (from 14.81 to 20.65 μmol/g to 61.25–192.07 μmol/g). Furthermore, the aromatic structure of alkali-activated biochar was enhanced, promoting π-π interactions. Furthermore, by homogenizing the irregular biochar surface using NaOH activation, the adsorption mechanism changed from multilayer adsorption to monolayer adsorption. Karami et al. (Karami et al., 2022) converted corn into biochar at 500°C and subsequently activated it at 900°C using CO2 as an activator (0.5–3 h). After activation, the specific surface area of biochar increased significantly from 63 m2/g to 419 m2/g, and the pore volume increased significantly from 0.04 cm3/g to 0.23 cm3/g. When the activation time was 3 h, biochar exhibited the strongest adsorption capacity for IBP. Mondal et al. (Mondal et al., 2016) treated N-doped biochar with NaOH for surface modification and used it for the removal of IBP in sewage. The results showed that the removal rate of IBP by biochar reached up to 99%, and the adsorption process conformed to the Langmuir isotherm model and pseudo-second-order kinetics. Show et al. found that biochar synthesized from tropical almond shells was used to remove IBP from water through acid/alkali activation. The adsorption process conformed to the Langmuir isotherm model, and linear and nonlinear analysis showed that the maximum adsorption capacity under acid activation is 2.794 mg/g and 8.77 mg/g, respectively.
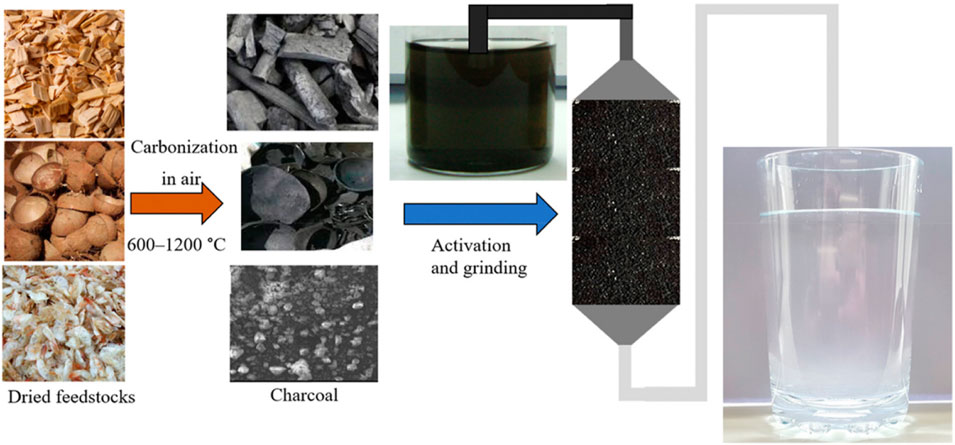
FIGURE 6. Biomass-derived porous carbonaceous materials and their composites as cationic and anionic dye adsorbents (Hassan and Carr, 2021).
5 Conclusion and outlook
Removal of emerging pollutants to protect human health and water recycling was one of the hot environmental issues worldwide. Research on the removal of ibuprofen from water was a multidisciplinary problem, so required collaboration among environmental scientists, materials scientists, and engineers. Current research showed that biochar can effectively adsorb IBP in the water environment and possessed potential application prospects. However, there were still some challenges in the removal of IBP by biochar, such as the stability and longevity of biochar, the feasibility of large-scale application, competitive adsorption with other pollutants, and regeneration of biochar. Therefore, researchers needed to find biochar preparation methods with higher stability, lower cost, and excellent performance to improve its practical application. For example, the published biochar preparation technology and modification conditions were modeled by machine learning to obtain the optimal synthesis conditions, optimal adsorption performance and optimal economic cost, further guiding the preparation and application of biochar. Moreover, researchers should also conduct field trials and large-scale application studies on different water bodies to evaluate the feasibility of biochar adsorption IBP technology. These field trials will allow the evaluation of the actual effectiveness of biochar for IBP adsorption, treatment efficiency, and interaction with other environmental parameters. In addition, the preparation cost, usage amount and actual application effect of biochar need to be considered in practical applications. Biochar recovery and recycling was also an important issue for large-scale applications. Therefore, there was a need to develop efficient biochar recycling technology and explore ways to recycle biochar to improve its sustainability and economy. The practical application of biochar adsorption to remove IBP also faced complex environmental conditions, such as organic matter and anions coexisting in the water environment. Therefore, it was necessary to further study the interaction between biochar and other pollutants and explore methods to improve the adsorption efficiency of target pollutants.
Simultaneous degradation with adsorption was also one of the areas of future research. Biochar degradation of IBP produced a series of degradation products, which may have different toxicity and environmental fate and require detailed analysis and evaluation. In addition, further treatment and removal of degradation products need to be considered to ensure the environmental friendliness and feasibility of the entire degradation process. At the same time, evaluating the feasibility of its practical application can provide support for the actual promotion of biochar degradation IBP technology. Through comprehensive risk assessment and monitoring, the safety and feasibility of biochar IBP removal technology in practical applications can be ensured. Besides, biochar removal of IBP technology can also be combined with other environmental treatment strategies to form a comprehensive pollution control plan, such as combining biochar and aquatic plant remediation technology. The combined application of biochar with other advanced oxidation technologies, such as ozone oxidation, photocatalysis, etc., can also be considered to enhance the removal effect.
Biochar, as an adsorbent material, was used to remove various pollutants in water. However, there are certain limitations in its application. First, biochar had different adsorption efficiencies for different types of drugs. Secondly, the adsorption capacity of biochar was limited, which may require frequent replacement or regeneration of biochar, increasing maintenance and operation costs. Of note, some drugs may be irreversibly combined with biochar in water bodies, making it difficult to regenerate or recycle biochar, resulting in a large waste of biochar and increasing environmental burden. Finally, the relatively high cost of biochar preparation, especially when applied on a large scale, limited its wide application in water treatment. Although biochar had the above limitations, it still had value as an adsorption material for reducing drug residues in water environment. It can effectively reduce the concentration of certain drugs despite adsorption selectivity and saturation issues. Moreover, biochar could be combined with other water treatment technologies (such as biological treatment or advanced oxidation) to improve drug removal efficiency, which overcame the limitations of biochar. In addition, biochar, as an environmentally friendly material, reduced waste through regeneration and recycling.
In summary, although the research on biochar in IBP removal has made some progress, there are still some challenges. Future research should focus on the stability and lifespan of biochar, the feasibility of large-scale application, the competitive adsorption mechanism of biochar and other pollutants, and biochar recovery and regeneration technology. By solving these problems, biochar, as an environmentally friendly material, is expected to play an important role in the treatment of IBP pollution in water bodies and the treatment of other pollutants.
Author contributions
DZ: Methodology, Writing–original draft. AY: Writing–original draft. TH: Conceptualization, Supervision, Writing–review and editing.
Funding
The author(s) declare that no financial support was received for the research, authorship, and/or publication of this article.
Conflict of interest
The authors declare that the research was conducted in the absence of any commercial or financial relationships that could be construed as a potential conflict of interest.
Publisher’s note
All claims expressed in this article are solely those of the authors and do not necessarily represent those of their affiliated organizations, or those of the publisher, the editors and the reviewers. Any product that may be evaluated in this article, or claim that may be made by its manufacturer, is not guaranteed or endorsed by the publisher.
References
Ahmed, I. O., Ali, A., Mohamed, F., Pavel, K., Bahareh, T., Hassan, K.-M., et al. (2023). Advanced adsorbents for ibuprofen removal from aquatic environments: a review. Environ. Chem. Lett. doi:10.1007/s10311-023-01647-6
Ahuja, R., Kalia, A., Sikka, R., and Chaitra, P. (2022). Nano modifications of biochar to enhance heavy metal adsorption from wastewaters: a review. Acs Omega 7, 45825–45836. doi:10.1021/acsomega.2c05117
Ai, T., Jiang, X., Zhong, Z., Li, D., and Dai, S. (2020). Methanol-modified ultra-fine magnetic orange peel powder biochar as an effective adsorbent for removal of ibuprofen and sulfamethoxazole from water. Adsorpt. Sci. Technol. 38, 304–321. doi:10.1177/0263617420944659
Ali, S. N. F., El-Shafey, E. I., Al-Busafi, S., and Al-Lawati, H. A. J. (2019). Adsorption of chlorpheniramine and ibuprofen on surface functionalized activated carbons from deionized water and spiked hospital wastewater. J. Environ. Chem. Eng. 7, 102860. doi:10.1016/j.jece.2018.102860
Alivia, M., Biswa, R. P., Jiban, P., and Ajay, K. D. (2022). Synthesis of biochar from lignocellulosic biomass for diverse industrial applications and energy harvesting: effects of pyrolysis conditions on the physicochemical properties of biochar. Front. Mater. 9. doi:10.3389/fmats.2022.870184
Alvear-Daza, J. J., Canneva, A., Donadelli, J. A., Manrique-Holguin, M., Rengifo-Herrera, J. A., and Pizzio, L. R. (2022). Removal of diclofenac and ibuprofen on mesoporous activated carbon from agro-industrial wastes prepared by optimized synthesis employing a central composite design. Biomass Convers. Biorefinery 13, 13197–13219. doi:10.1007/s13399-021-02227-w
An, H. J., Bhadra, B. N., Khan, N. A., and Jhung, S. H. (2018). Adsorptive removal of wide range of pharmaceutical and personal care products from water by using metal azolate framework-6-derived porous carbon. Chem. Eng. J. 343, 447–454. doi:10.1016/j.cej.2018.03.025
Aschermann, G., Zietzschmann, F., and Jekel, M. (2018). Influence of dissolved organic matter and activated carbon pore characteristics on organic micropollutant desorption. Water Res. 133, 123–131. doi:10.1016/j.watres.2018.01.015
Aydin, E. B., Aydin, M., and Sezginturk, M. K. (2019). Biosensors in drug discovery and drug analysis. Curr. Anal. Chem. 15, 467–484. doi:10.2174/1573411014666180912131811
Bello, O. S., Alao, O. C., Alagbada, T. C., Agboola, O. S., Omotoba, O. T., and Abikoye, O. R. (2021). A renewable, sustainable and low-cost adsorbent for ibuprofen removal. Water Sci. Technol. 83, 111–122. doi:10.2166/wst.2020.551
Bello, O. S., Alao, O. C., Alagbada, T. C., and Olatunde, A. M. (2019). Biosorption of ibuprofen using functionalized bean husks. Sustain. Chem. Pharm. 13, 100151. doi:10.1016/j.scp.2019.100151
Betül, E., Koray, A., Suat, U., and Selhan, K. (2023). Comparative studies of hydrochars and biochars produced from lignocellulosic biomass via hydrothermal carbonization, torrefaction and pyrolysis. J. Energy Inst. 109, 101298. doi:10.1016/j.joei.2023.101298
Bhadra, B. N., Ahmed, I., Kim, S., and Jhung, S. H. (2017). Adsorptive removal of ibuprofen and diclofenac from water using metal-organic framework-derived porous carbon. Chem. Eng. J. 314, 50–58. doi:10.1016/j.cej.2016.12.127
Bouzidi, M., Sellaoui, L., Mohamed, M., Franco, D. S. P., Erto, A., and Badawi, M. (2023). A comprehensive study on paracetamol and ibuprofen adsorption onto biomass-derived activated carbon through experimental and theoretical assessments. J. Mol. Liq. 376, 121457. doi:10.1016/j.molliq.2023.121457
Brillas, E. (2022). A critical review on ibuprofen removal from synthetic waters, natural waters, and real wastewaters by advanced oxidation processes. Chemosphere 286, 131849. doi:10.1016/j.chemosphere.2021.131849
Calisici, D., Yilmaz, S., and Goktas, B. (2023). Toxic, genotoxic and teratogenic effects of ibuprofen and its derivatives. Curr. Drug Targets 24, 361–370. doi:10.2174/1389450124666230104160435
Chakraborty, P., Show, S., Banerjee, S., and Halder, G. (2018). Mechanistic insight into sorptive elimination of ibuprofen employing bi-directional activated biochar from sugarcane bagasse: performance evaluation and cost estimation. J. Environ. Chem. Eng. 6, 5287–5300. doi:10.1016/j.jece.2018.08.017
Chakraborty, P., Show, S., Rahman, W. U., and Halder, G. (2019). Linearity and non-linearity analysis of isotherms and kinetics for ibuprofen remotion using superheated steam and acid modified biochar. Process Saf. Environ. Prot. 126, 193–204. doi:10.1016/j.psep.2019.04.011
Chakraborty, P., Singh, S. D., Gorai, I., Singh, D., Rahman, W.-U., and Halder, G. (2020). Explication of physically and chemically treated date stone biochar for sorptive remotion of ibuprofen from aqueous solution. J. Water Process Eng. 33, 101022. doi:10.1016/j.jwpe.2019.101022
Chen, D., Li, Y., Cen, K., Luo, M., Li, H., and Lu, B. (2016). Pyrolysis polygeneration of poplar wood: effect of heating rate and pyrolysis temperature. Bioresour. Technol. 218, 780–788. doi:10.1016/j.biortech.2016.07.049
Chen, Y., Zhang, X., Chen, W., Yang, H., and Chen, H. (2017). The structure evolution of biochar from biomass pyrolysis and its correlation with gas pollutant adsorption performance. Bioresour. Technol. 246, 101–109. doi:10.1016/j.biortech.2017.08.138
Croitoru, C., Patachia, S., Doroftei, F., Parparita, E., and Vasile, C. (2014). Ionic liquids influence on the surface properties of electron beam irradiated wood. Appl. Surf. Sci. 314, 956–966. doi:10.1016/j.apsusc.2014.06.142
Cui, X., Wang, J., Wang, X., Du, G., Khan, K. Y., Yan, B., et al. (2022). Pyrolysis of exhausted hydrochar sorbent for cadmium separation and biochar regeneration. Chemosphere 306, 135546. doi:10.1016/j.chemosphere.2022.135546
Cui, Z., Xu, G., Ormeci, B., and Hao, J. (2023). Kill two birds with one stone: the management of hazardous waste and the preparation of efficient adsorbents for Pb(II) were realized by the pyrolysis of penicillin mycelial dreg. Environ. Pollut. 316, 120508. doi:10.1016/j.envpol.2022.120508
Das, S. K., Ghosh, G. K., Avasthe, R., and Sinha, K. (2021). Morpho-mineralogical exploration of crop, weed and tree derived biochar. J. Hazard. Mater. 407, 124370. doi:10.1016/j.jhazmat.2020.124370
Delgado-Moreno, L., Bazhari, S., Gasco, G., Mendez, A., El Azzouzi, M., and Romero, E. (2021). New insights into the efficient removal of emerging contaminants by biochars and hydrochars derived from olive oil wastes. Sci. Total Environ. 752, 141838. doi:10.1016/j.scitotenv.2020.141838
Dison, S. P. F., Jordana, G., Eder, C. L., and Luis, F. O. S. (2022). Advances made in removing paraquat herbicide by adsorption technology: a review. J. Water Process Eng. 49, 102988. doi:10.1016/j.jwpe.2022.102988
Du, Y.-d., Zhang, X.-q., Shu, L., Feng, Y., Lv, C., Liu, H.-q., et al. (2021). Safety evaluation and ibuprofen removal via anAlternanthera philoxeroides-based biochar. Environ. Sci. Pollut. Res. 28, 40568–40586. doi:10.1007/s11356-020-09714-z
Escudero-Curiel, S., Acevedo-García, V., Sanromán, M. Á., and Pazos, M. (2021). Eco-approach for pharmaceutical removal: thermochemical waste valorisation, biochar adsorption and electro-assisted regeneration. Electrochimica Acta 389, 138694. doi:10.1016/j.electacta.2021.138694
Essandoh, M., Kunwar, B., Pittman, C. U., Mohan, D., and Mlsna, T. (2015). Sorptive removal of salicylic acid and ibuprofen from aqueous solutions using pine wood fast pyrolysis biochar. Chem. Eng. J. 265, 219–227. doi:10.1016/j.cej.2014.12.006
Fan, X., Hao, L., Gu, X., and Li, S. (2023). Low-temperature selective catalytic reduction of NO with NH3 over a biochar-supported perovskite oxide catalyst. Energy & Fuels 37, 7339–7352. doi:10.1021/acs.energyfuels.2c04291
Farhadi, N., Tabatabaie, T., Ramavandi, B., and Amiri, F. (2020). Optimization and characterization of zeolite-titanate for ibuprofen elimination by sonication/hydrogen peroxide/ultraviolet activity. Ultrason. Sonochemistry 67, 105122. doi:10.1016/j.ultsonch.2020.105122
Frohlich, A. C., dos Reis, G. S., Pavan, F. A., Lima, E. C., Foletto, E. L., and Dotto, G. L. (2018a). Improvement of activated carbon characteristics by sonication and its application for pharmaceutical contaminant adsorption. Environ. Sci. Pollut. Res. 25, 24713–24725. doi:10.1007/s11356-018-2525-x
Frohlich, A. C., Ocampo-Perez, R., Diaz-Blancas, V., Salau, N. P. G., and Dotto, G. L. (2018b). Three-dimensional mass transfer modeling of ibuprofen adsorption on activated carbon prepared by sonication. Chem. Eng. J. 341, 65–74. doi:10.1016/j.cej.2018.02.020
Gaffar, J., Gabrielli, S., Lavine, E., Pitt, T., Abrams, E., Atkinson, A., et al. (2020). Diagnosis of Ibuprofen allergy through oral challenge. Clin. Exp. Allergy 50, 636–639. doi:10.1111/cea.13596
Georgin, J., Franco, D. S. P., da Boit Martinello, K., Lima, E. C., and Silva, L. F. O. (2022). A review of the toxicology presence and removal of ketoprofen through adsorption technology. J. Environ. Chem. Engineering 10, 107798. doi:10.1016/j.jece.2022.107798
Habaki, H., Hayashi, T., Sinthupinyo, P., and Egashira, R. (2019). Purification of glycerol from transesterification using activated carbon prepared from Jatropha Shell for biodiesel production. J. Environ. Chem. Eng. 7, 103303. doi:10.1016/j.jece.2019.103303
Hassan, M. M., and Carr, C. M. (2021). Biomass-derived porous carbonaceous materials and their composites as adsorbents for cationic and anionic dyes: a review. Chemosphere 265, 129087. doi:10.1016/j.chemosphere.2020.129087
Huang, A., Bai, W., Yang, S., Wang, Z., Wu, N., Zhang, Y., et al. (2022). Adsorption characteristics of chitosan-modified bamboo biochar in Cd(II) contaminated water. J. Chem. 2022, 1–10. doi:10.1155/2022/6303252
Ji, Y.-L., Lu, H.-H., Gu, B.-X., Ye, R.-F., Zhou, Y., An, Q.-F., et al. (2021). Tailoring the asymmetric structure of polyamide reverse osmosis membrane with self-assembled aromatic nanoparticles for high-efficient removal of organic micropollutants. Chem. Eng. J. 416, 129080. doi:10.1016/j.cej.2021.129080
Jordana, G., Dison Stracke Pfingsten, F., Claudete Gindri, R., Daniel, G. A. P., Eder, C. L., and Farooq, S. (2023a). A review of the antibiotic ofloxacin: current status of ecotoxicology and scientific advances in its removal from aqueous systems by adsorption technology. Chem. Eng. Res. Des. 193, 99–120. doi:10.1016/j.cherd.2023.03.025
Jordana, G., Dison Stracke Pfingsten, F., Lucas, M., Younes, D., Glaydson, S. d.R., and Eder, C. L. (2023b). Main advances and future prospects in the remediation of the antibiotic amoxicillin with a focus on adsorption technology: a critical review. J. Water Process Eng. 56, 104407. doi:10.1016/j.jwpe.2023.104407
Karami, S., Papari, S., and Berruti, F. (2022). Conversion of waste corn biomass to activated bio-char for applications in wastewater treatment. Front. Mater. 9. doi:10.3389/fmats.2022.839421
Karunanayake, A. G., Todd, O. A., Crowley, M. L., Ricchetti, L. B., Pittman, C. U., Anderson, R., et al. (2017). Rapid removal of salicylic acid, 4-nitroaniline, benzoic acid and phthalic acid from wastewater using magnetized fast pyrolysis biochar from waste Douglas fir. Chem. Eng. J. 319, 75–88. doi:10.1016/j.cej.2017.02.116
Kim, S., Muñoz-Senmache, J. C., Jun, B.-M., Park, C. M., Jang, A., Yu, M., et al. (2019a). A metal organic framework-ultrafiltration hybrid system for removing selected pharmaceuticals and natural organic matter. Chem. Eng. J. 382, 122920. doi:10.1016/j.cej.2019.122920
Kim, S., Park, C. M., Jang, A., Jang, M., Hernández-Maldonado, A. J., Yu, M., et al. (2019b). Removal of selected pharmaceuticals in an ultrafiltration-activated biochar hybrid system. J. Membr. Sci. 570-571, 77–84. doi:10.1016/j.memsci.2018.10.036
Kumar, M., Xiong, X., Wan, Z., Sun, Y., Tsang, D. C. W., Gupta, J., et al. (2020). Ball milling as a mechanochemical technology for fabrication of novel biochar nanomaterials. Bioresour. Technol. 312, 123613. doi:10.1016/j.biortech.2020.123613
Leng, L., and Huang, H. (2018). An overview of the effect of pyrolysis process parameters on biochar stability. Bioresour. Technol. 270, 627–642. doi:10.1016/j.biortech.2018.09.030
Li, L., Long, A., Fossum, B., and Kaiser, M. (2023a). Effects of pyrolysis temperature and feedstock type on biochar characteristics pertinent to soil carbon and soil health: a meta-analysis. Soil Use Manag. 39, 43–52. doi:10.1111/sum.12848
Li, R., Wang, B., Niu, A., Cheng, N., Chen, M., Zhang, X., et al. (2022). Application of biochar immobilized microorganisms for pollutants removal from wastewater: a review. Sci. Total Environ. 837, 155563. doi:10.1016/j.scitotenv.2022.155563
Li, R., Wang, Z., Guo, J., Li, Y., Zhang, H., Zhu, J., et al. (2018). Enhanced adsorption of ciprofloxacin by KOH modified biochar derived from potato stems and leaves. Water Sci. Technol. 77, 1127–1136. doi:10.2166/wst.2017.636
Li, X., Jiang, Y., Chen, T., Zhao, P., Niu, S., Yuan, M., et al. (2023b). Adsorption of norfloxacin from wastewater by biochar with different substrates. Environ. Geochem. Health 45, 3331–3344. doi:10.1007/s10653-022-01414-6
Liao, J., Chen, H., Zhang, Y., and Zhu, W. (2022). Pyrolysis of animal manure under nitrogen atmosphere: an environment protection way to obtain animal manure biochar for high-efficient adsorption of uranium (VI). J. Anal. Appl. Pyrolysis 163, 105493. doi:10.1016/j.jaap.2022.105493
Lin, L., Jiang, W., and Xu, P. (2017). Comparative study on pharmaceuticals adsorption in reclaimed water desalination concentrate using biochar: impact of salts and organic matter. Sci. Total Environ. 601, 857–864. doi:10.1016/j.scitotenv.2017.05.203
Liyanage, A. S., Canaday, S., Pittman, C. U., and Mlsna, T. (2020). Rapid remediation of pharmaceuticals from wastewater using magnetic Fe3O4/Douglas fir biochar adsorbents. Chemosphere 258, 127336. doi:10.1016/j.chemosphere.2020.127336
Luo, R., Li, X., Xu, H., Sun, Y., and Wu, J. (2020). Effects of temperature, solution pH, and ball-milling modification on the adsorption of non-steroidal anti-inflammatory drugs onto biochar. Bull. Environ. Contam. Toxicol. 105, 422–427. doi:10.1007/s00128-020-02948-0
Mestre, A. S., Hesse, F., Freire, C., Ania, C. O., and Carvalho, A. P. (2019). Chemically activated high grade nanoporous carbons from low density renewable biomass (Agave sisalana) for the removal of pharmaceuticals. J. Colloid Interface Sci. 536, 681–693. doi:10.1016/j.jcis.2018.10.081
Min, H., Yudan, H., Xuewen, Y., Yuchun, Y., Xu, L., Yongxing, F., et al. (2023). Preparation of biomass biochar with components of similar proportions and its methylene blue adsorption. Molecules 28, 6261. doi:10.3390/molecules28176261
Mohammed, Z., Jeelani, S., and Rangari, V. (2021). Low temperature plasma treatment of rice husk derived hybrid silica/carbon biochar using different gas sources. Mater. Lett. 292, 129678. doi:10.1016/j.matlet.2021.129678
Mojiri, A., Kazeroon, R. A., and Gholami, A. (2019). Cross-linked magnetic chitosan/activated biochar for removal of emerging micropollutants from water: optimization by the artificial neural network. Water 11, 551. doi:10.3390/w11030551
Mondal, S., Aikat, K., and Halder, G. (2016). Biosorptive uptake of ibuprofen by chemically modified Parthenium hysterophorus derived biochar: equilibrium, kinetics, thermodynamics and modeling. Ecol. Eng. 92, 158–172. doi:10.1016/j.ecoleng.2016.03.022
Moreno-Perez, J., Pauletto, P. S., Cunha, A. M., Bonilla-Petriciolet, A., Salau, N. P. G., and Dotto, G. L. (2021). Three-dimensional mass transport modeling of pharmaceuticals adsorption inside ZnAl/biochar composite. Colloids Surfaces a-Physicochemical Eng. Aspects 614, 126170. doi:10.1016/j.colsurfa.2021.126170
Muhammad, J., Zohaib, A., Junaid Ali, S., Shulin, L., Shamas, T., Qiang, Y., et al. (2023). Ecotoxicological impacts associated with the interplay between micro(nano)plastics and pesticides in aquatic and terrestrial environments. Trends Anal. Chem. 165, 117133. doi:10.1016/j.trac.2023.117133
Naima, A., Ammar, F., Abdelkader, O., Rachid, C., Lynda, H., Syafiuddin, A., et al. (2022). Development of a novel and efficient biochar produced from pepper stem for effective ibuprofen removal. Bioresour. Technol. 347, 126685. doi:10.1016/j.biortech.2022.126685
Ndoun, M. C., Knopf, A., Preisendanz, H. E., Vozenilek, N., Elliott, H. A., Mashtare, M. L., et al. (2023). Fixed bed column experiments using cotton gin waste and walnut shells-derived biochar as low-cost solutions to removing pharmaceuticals from aqueous solutions. Chemosphere 330, 138591. doi:10.1016/j.chemosphere.2023.138591
Ngernyen, Y., Petsri, D., Sribanthao, K., Kongpennit, K., Pinijnam, P., Pedsakul, R., et al. (2023). Adsorption of the non-steroidal anti-inflammatory drug (ibuprofen) onto biochar and magnetic biochar prepared from chrysanthemum waste of the beverage industry. Rsc Adv. 13, 14712–14728. doi:10.1039/D3RA01949G
Nguyen, T.-K.-T., Nguyen, T.-B., Chen, W.-H., Chen, C.-W., Kumar Patel, A., Bui, X.-T., et al. (2023). Phosphoric acid-activated biochar derived from sunflower seed husk: selective antibiotic adsorption behavior and mechanism. Bioresour. Technol. 371, 128593. doi:10.1016/j.biortech.2023.128593
Nourmoradi, H., Moghadam, K. F., Jafari, A., and Kamarehie, B. (2018). Removal of acetaminophen and ibuprofen from aqueous solutions by activated carbon derived from Quercus Brantii (Oak) acorn as a low-cost biosorbent. J. Environ. Chem. Eng. 6, 6807–6815. doi:10.1016/j.jece.2018.10.047
Ocampo-Perez, R., Padilla-Ortega, E., Medellin-Castillo, N. A., Coronado-Oyarvide, P., Aguilar-Madera, C. G., Segovia-Sandoval, S. J., et al. (2019). Synthesis of biochar from chili seeds and its application to remove ibuprofen from water. Equilibrium and 3D modeling. Sci. Total Environ. 655, 1397–1408. doi:10.1016/j.scitotenv.2018.11.283
Omorogie, M. O., Babalola, J. O., Ismaeel, M. O., McGettrick, J. D., Watson, T. M., Dawson, D. M., et al. (2021). Activated carbon from Nauclea diderrichii agricultural waste-a promising adsorbent for ibuprofen, methylene blue and CO2. Adv. Powder Technol. 32, 866–874. doi:10.1016/j.apt.2021.01.031
Osman, A. I., Fawzy, S., Farghali, M., El-Azazy, M., Elgarahy, A. M., Fahim, R. A., et al. (2022). Biochar for agronomy, animal farming, anaerobic digestion, composting, water treatment, soil remediation, construction, energy storage, and carbon sequestration: a review. Environ. Chem. Lett. 20, 2385–2485. doi:10.1007/s10311-022-01424-x
Pap, S., Taggart, M. A., Shearer, L., Li, Y., Radovic, S., and Sekulic, M. T. (2021). Removal behaviour of NSAIDs from wastewater using a P-functionalised microporous carbon. Chemosphere 264, 128439. doi:10.1016/j.chemosphere.2020.128439
Rangarajan, G., Jayaseelan, A., and Farnood, R. (2022). Photocatalytic reactive oxygen species generation and their mechanisms of action in pollutant removal with biochar supported photocatalysts: a review. J. Clean. Prod. 346, 131155. doi:10.1016/j.jclepro.2022.131155
Rani, S., Malik, A. K., Kaur, R., Kaur, R., and null, n. (2016). A Review for the Analysis of antidepressant, antiepileptic and quinolone type drugs in Pharmaceuticals and Environmental samples. Crit. Rev. Anal. Chem. 46, 424–442. doi:10.1080/10408347.2016.1141670
Rizvi, S. G., and Ahammad, S. Z. (2021). COVID-19 and antimicrobial resistance: a cross-study. Sci. Total Environ. 2, 150873. doi:10.1016/j.scitotenv.2021.150873
Samer, F., Ahmed, I. O., Neha, M., Donal, M., Ala'a, H.A.-M., and David, W. R. (2022). Atmospheric carbon removal via industrial biochar systems: a techno-economic-environmental study. J. Clean. Prod. 371, 133660. doi:10.1016/j.jclepro.2022.133660
Sean, T. M., Adrián Serrano, M., Fatemeh Asadi, Z., Brooke, K. M., Patrick, M., Madjid, M., et al. (2021). Progress and prospect of anodic oxidation for the remediation of perfluoroalkyl and polyfluoroalkyl substances in water and wastewater using diamond electrodes. Curr. Opin. Electrochem. 30, 100865. doi:10.1016/j.coelec.2021.100865
Shin, J., Kwak, J., Lee, Y.-G., Kim, S., Choi, M., Bae, S., et al. (2021). Competitive adsorption of pharmaceuticals in lake water and wastewater effluent by pristine and NaOH-activated biochars from spent coffee wastes: contribution of hydrophobic and π-π interactions. Environ. Pollut. 270, 116244. doi:10.1016/j.envpol.2020.116244
Shin, J., Lee, Y.-G., Lee, S.-H., Kim, S., Ochir, D., Park, Y., et al. (2020). Single and competitive adsorptions of micropollutants using pristine and alkali-modified biochars from spent coffee grounds. J. Hazard. Mater. 400, 123102. doi:10.1016/j.jhazmat.2020.123102
Show, S., Chowdhury, S., Maji, M., Sarkar, P., Ghosh, M., Sillanpaa, M., et al. (2022a). Sorptive elimination of ibuprofen using activated biochar: modelling, non-linear isotherm and kinetic, cost assessment and toxicity analysis. Biomass Convers. Biorefinery. doi:10.1007/s13399-022-03161-1
Show, S., Karmakar, B., and Halder, G. (2022b). Sorptive uptake of anti-inflammatory drug ibuprofen by waste biomass-derived biochar: experimental and statistical analysis. Biomass Convers. Biorefinery 12, 3955–3973. doi:10.1007/s13399-020-00922-8
Show, S., Mukherjee, S., Devi, M. S., Karmakar, B., and Halder, G. (2021). Linear and non-linear analysis of Ibuprofen riddance efficacy by Terminalia catappa active biochar: equilibrium, kinetics, safe disposal, reusability and cost estimation. Process Saf. Environ. Prot. 147, 942–964. doi:10.1016/j.psep.2021.01.024
Sivarajasekar, N., Mohanraj, N., Sivamani, S., Maran, J. P., Moorthy, I. G., and Balasubramani, K. (2017). “Statistical optimization studies on adsorption of ibuprofen onto Albizialebbeck seed pods activated carbon prepared using microwave irradiation,” in Proceedings of the 1st International Conference on Emerging Trends in Materials and Manufacturing Engineering (IMME), Tiruchirappalli, INDIA, January 2017, 7264–7274.
Solanki, A., and Boyer, T. H. (2017). Pharmaceutical removal in synthetic human urine using biochar. Environ. Sci. Water Res. Technol. 3, 553–565. doi:10.1039/C6EW00224B
Souza-Silva, G., de Souza, C. R., Pereira, C. A. d.J., Dos Santos Lima, W., Mol, M. P. G., and Silveira, M. R. (2023). Using freshwater snail Biomphalaria glabrata (Say, 1818) as a biological model for ecotoxicology studies: a systematic review. Environ. Sci. Pollut. Res. 30, 28506–28524. doi:10.1007/s11356-023-25455-1
Streit, A. F. M., Collazzo, G. C., Druzian, S. P., Verdi, R. S., Foletto, E. L., Oliveira, L. F. S., et al. (2021). Adsorption of ibuprofen, ketoprofen, and paracetamol onto activated carbon prepared from effluent treatment plant sludge of the beverage industry. Chemosphere 262, 128322. doi:10.1016/j.chemosphere.2020.128322
Tariq, A., Emanne, R., Mohamed, E.-Q., and Ramy, H. M. (2022). A comprehensive review on the chemical regeneration of biochar adsorbent for sustainable wastewater treatment. npj Clean. Water 29. doi:10.1038/s41545-022-00172-3
Thuan Van, T., Duyen Thi Cam, N., Le, H. T. N., Long Giang, B., Vo, D.-V. N., Dao, T.-U. T., et al. (2019). Effect of thermolysis condition on characteristics and nonsteroidal anti-inflammatory drugs (NSAIDs) absorbability of Fe-MIL-88B-derived mesoporous carbons. J. Environ. Chem. Eng. 7, 103356. doi:10.1016/j.jece.2019.103356
Van Thom, N., Thi Minh Tam, N., Liu, Y.-g., and Cai, Q.-y. (2021). Fabrication of partially graphitic biochar for the removal of diclofenac and ibuprofen from aqueous solution: laboratory conditions and real sample applications. Environ. Eng. Sci. 38, 974–989. doi:10.1089/ees.2020.0202
Verduzo Garibay, M., Fernández del Castillo, A., de Anda, J., Senés-Guerrero, C., and Gradilla-Hernández, M. S. (2021). Structure and activity of microbial communities in response to environmental, operational, and design factors in constructed wetlands. Int. J. Environ. Sci. Technol. 19, 11587–11612. doi:10.1007/s13762-021-03719-y
Vieira, Y., Ribeiro, T. H., Leichtweis, J., Dotto, G. L., Foletto, E. L., Georgin, J., et al. (2023). A critical review of the current environmental risks posed by the antidiabetic Metformin and the status, advances, and trends in adsorption technologies for its remediation. J. Water Process Eng. 54, 103943. doi:10.1016/j.jwpe.2023.103943
Wang, C., Li, J., Fang, W., Chen, W., Zou, M., Li, X., et al. (2021). Lipid degrading microbe consortium driving micro-ecological evolvement of activated sludge for cooking wastewater treatment. Sci. Total Environ. 804, 150071. doi:10.1016/j.scitotenv.2021.150071
Wang, J., and Wang, S. (2019). Preparation, modification and environmental application of biochar: a review. J. Clean. Prod. 227, 1002–1022. doi:10.1016/j.jclepro.2019.04.282
Wongrod, S., Simon, S., Guibaud, G., Lens, P. N. L., Pechaud, Y., Huguenot, D., et al. (2018). Lead sorption by biochar produced from digestates: consequences of chemical modification and washing. J. Environ. Manag. 219, 277–284. doi:10.1016/j.jenvman.2018.04.108
Xu, X., Xu, Z., Huang, J., Gao, B., Zhao, L., Qiu, H., et al. (2021). Sorption of reactive red by biochars ball milled in different atmospheres: Co-effect of surface morphology and functional groups. Chem. Eng. J. 413, 127468. doi:10.1016/j.cej.2020.127468
Yang, X., Zhang, X., Ngo, H. H., Guo, W., Huo, J., Du, Q., et al. (2022). Sorptive removal of ibuprofen from water by natural porous biochar derived from recyclable plane tree leaf waste. J. Water Process Eng. 46, 102627. doi:10.1016/j.jwpe.2022.102627
Yuan, Y., Bolan, N., Prévoteau, A., Vithanage, M., Biswas, J. K., Ok, Y. S., et al. (2017). Applications of biochar in redox-mediated reactions. Bioresour. Technol. 246, 271–281. doi:10.1016/j.biortech.2017.06.154
Yudha, S. P., Tekasakul, S., Phoungthong, K., and Chuenchom, L. (2019). Green synthesis of low-cost and eco-friendly adsorbent for dye and pharmaceutical adsorption: kinetic, isotherm, thermodynamic and regeneration studies. Mater. Res. Express 6, 125526. doi:10.1088/2053-1591/ab58ae
Zhang, J., Zhang, N., Tack, F. M. G., Sato, S., Alessi, D. S., Oleszczuk, P., et al. (2021). Modification of ordered mesoporous carbon for removal of environmental contaminants from aqueous phase: a review. J. Hazard. Mater. 418, 126266. doi:10.1016/j.jhazmat.2021.126266
Zhang, P., Liu, S., Tan, X., Liu, Y., Zeng, G., Yin, Z., et al. (2019). Microwave-assisted chemical modification method for surface regulation of biochar and its application for estrogen removal. Process Saf. Environ. Prot. 128, 329–341. doi:10.1016/j.psep.2019.06.009
Zhang, W., Chen, R., Li, J., Huang, T., Wu, B., Ma, J., et al. (2023). Synthesis optimization and adsorption modeling of biochar for pollutant removal via machine learning. Biochar 5, 25. doi:10.1007/s42773-023-00225-x
Zhi, K., Li, Z., Ma, P., Tan, Y., Zhou, Y., Zhang, W., et al. (2021). A review of activation persulfate by iron-based catalysts for degrading wastewater. Appl. Sci. 11, 11314. doi:10.3390/app112311314
Zhou, N., Wang, Y., Yao, D., Li, S., Tang, J., Shen, D., et al. (2019). Novel wet pyrolysis providing simultaneous conversion and activation to produce surface-functionalized biochars for cadmium remediation. J. Clean. Prod. 221, 63–72. doi:10.1016/j.jclepro.2019.02.176
Keywords: ibuprofen, aquatic environment, biochar, modification, adsorption
Citation: Zhan D, Ye A and Hou T (2023) Research progress on biochar-based material adsorption and removal of ibuprofen. Front. Environ. Sci. 11:1327000. doi: 10.3389/fenvs.2023.1327000
Received: 24 October 2023; Accepted: 20 November 2023;
Published: 20 December 2023.
Edited by:
Yalçin Tepe, Giresun University, TürkiyeReviewed by:
Ahmed Ibrahim Osman, Queen’s University Belfast, United KingdomMukarram Zubair, Imam Abdulrahman Bin Faisal University, Saudi Arabia
Jordana Georgin, Federal University of Santa Maria, Brazil
Copyright © 2023 Zhan, Ye and Hou. This is an open-access article distributed under the terms of the Creative Commons Attribution License (CC BY). The use, distribution or reproduction in other forums is permitted, provided the original author(s) and the copyright owner(s) are credited and that the original publication in this journal is cited, in accordance with accepted academic practice. No use, distribution or reproduction is permitted which does not comply with these terms.
*Correspondence: Tingting Hou, houtingting6666@163.com