- Faculty of Chemical and Food Engineering, Bahir Dar Institute of Technology, Bahir Dar University, Bahir Dar, Ethiopia
Petroleum hydrocarbons (PHCs) are key energy sources for several industries and daily life. Soil contamination from oily PHC spills is commonly detected in cities and industrial facilities where crude oil is used. The release of PHC pollutants into the environment, whether accidentally from petroleum industries or human activities, has become a leading source of soil pollution. Consequently, the mineralization of PHC-polluted sites has become a central issue worldwide. Although bioremediation is imperative for environmental safety and management, several approaches have been developed for PHC bioremediation. However, much remains to be explored in this regard. This review explores bioremediation of PHC-contaminated soil and provides a comprehensive examination of the principles, degradation mechanisms, and recent advancements in the field. Several microbial species have been used to study the bioremediation of PHCs, emphasizing the pivotal roles of diverse microbial communities. Aspergillus spp., Proteobacteria, and Firmicutes groups of microorganisms were the most efficient in remediating PHC-contaminated soil. The fundamental concepts behind the bioremediation of PHC and the complex mechanisms that govern degradation were elucidated. Limiting factors in the bioremediation process and recent innovations propelling the field were also discussed. Therefore, understanding the degradation pathway, ensuring complete degradation of contaminants, and flexible legislation for the proper use of genetically engineered microbes can make bioremediation more sustainable and cost-effective.
Highlights
• PHCs from the petroleum industry and human activities are important sources of soil contamination.
• Microbial consortia are more effective PHC degraders in soil than are single isolates.
• Lignolytic enzymes excreted from microbes are more effective in the degradation of PHC.
• Different pathways can appear for the aerobic degradation of PHCs in the soil.
1 Introduction
The rapid growth in industrial, agricultural, and municipal activities, together with the unprecedented global population growth rate, has led to a high demand for petroleum production (Singha and Pandey, 2021). Global demand for petroleum products is expected to reach 100.90 million barrels per day (Gaur et al., 2021). Petroleum industrial activities, such as drilling, exploration, storage, transportation, processing, and refining, are major sources of petroleum hydrocarbon spillage, which leads to terrestrial oil pollution that generally contains hydrophobic compounds (Chettri et al., 2021). The spillage of petroleum hydrocarbons (PHCs) into the environment is a critical hazard to ecosystems, and can pose serious human health and environmental problems (Guarino et al., 2017). The annual crude oil leakage into the environment is estimated to be 0.6 Mt (Kvenvolden and Cooper, 2003). PHCs are fundamentally composed of carbon and hydrogen, and their pollution in the soil results in a disproportion of the C/N fraction at the spill site. This causes nitrogen deficit and hinders soil fertility and microbial activity. Moreover, immense quantities of biodegradable organic compounds in the top layer of the soil deplete oxygen reserves and decelerate the degree of oxygen diffusion into deeper layers (Dindar et al., 2013). Therefore, the remediation of PHC-contaminated soils is presently a significant task, and it is vital to develop eco-friendly and effective approaches to uphold soil ecosystems (Cui et al., 2020).
Various remediation technologies have been proven and deployed to treat petroleum-polluted soils. An actual polluted site may require optimal remediation through a combination of processes for prevalent conditions (Dindar et al., 2013). Recent PHC remediation methods include physical, chemical, and biological approaches (Shi et al., 2022). Physical and chemical pollutant detoxification technologies are often expensive and pose the risk of extra air or water pollution. Biological approaches, owing to the miscellaneous metabolic capabilities of microorganisms and plants, are progressing techniques for the degradation of various environmental pollutants containing petroleum products (Singha and Pandey, 2021).
Bioremediation is an effective, economical, and eco-friendly approach that has gradually become popular for mineralizing organic contaminants in wastewater (Aragaw, 2021) and soil environments (Sayara and Sánchez, 2020). In particular, bioremediation of PHC-contaminated soil has attracted the attention of researchers owing to its eco-friendliness (Roy et al., 2018). Microbes play a crucial role in the biotransformation of intricate pollutant mixtures during the restoration of contaminated soils (Macaulay and Rees, 2014). Evidence in the literature has shown that a large number of microbes (bacteria, fungi, and some algae) and various plant species can mineralize PHCs completely into CO2, H2O, inorganic compounds, cell proteins, and simple organic compounds found in soil and water (Das and Chandran, 2011). However, the biological activity of microorganisms is influenced by environmental factors, including energy sources, bioavailability, bioactivity, biochemistry, oxygen and nutrient availability, pH, temperature, and metabolite inhibition (Kebede et al., 2021). Therefore, several studies have reported the impact of various PHCs on soil and diverse remediation methods. Publication analysis is a popular and successful tool for determining research trends and relevant concerns. The publication trends in Figure 1 confirm that the number of publications on biological remediation techniques for PHC-polluted soils has recently increased. The publication trends with the search key (((“Bioremediation) AND (“Petroleum Hydrocarbon”) AND (“contaminated soil”))) were obtained from PubMed (https://pubmed.ncbi.nlm.nih.gov/) and Scopus (https://www.scopus.com/). A literature search was conducted on 25 December 2022, and 119 and 385 publications from PubMed and Scopus, respectively, with publication dates from 2000 to 2022. A total of 115 articles found in the PubMed database overlapped with those in the Scopus database. Therefore, the total number of articles retrieved from both databases was 389. Journal article publications were filtered and employed based on the contents of the articles that fit the scope of this review. Articles that were as per the scope and had adequate results were used for a detailed discussion (in Tables 1, 2). Articles with limited information were used for the other sections, including the Introduction section. Furthermore, specific search keywords/phrases on microbial remediation of PHC-contaminated soil were retrieved via Google Scholar (https: scholar google com). The publication trend shows that it is highly increasing, suggesting that the microbial-based remediation of PHC-contaminated soil has recently attracted attention.
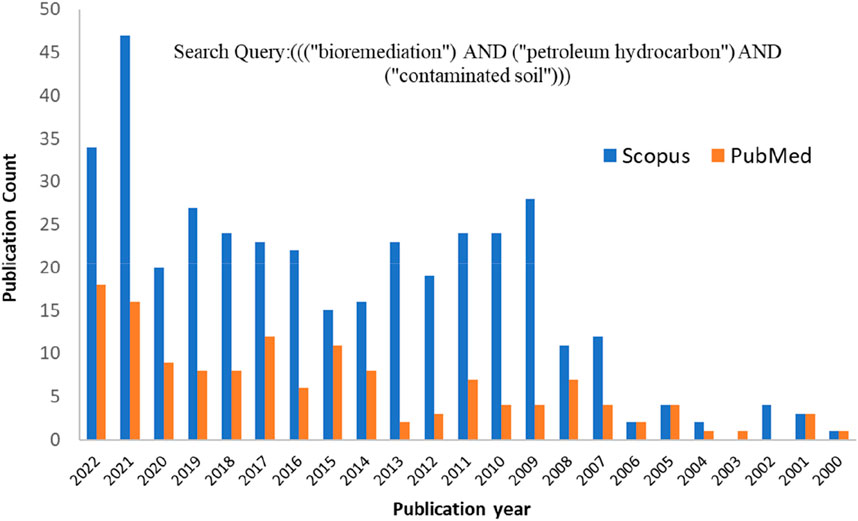
FIGURE 1. Publication trends from 2000 to 2022 in the bioremediation of petroleum hydrocarbon (PHC)-contaminated soil.
Several studies have shown that bioremediation approaches using bacterial, fungal, and rhizosphere microbiome species alone or in combination have been effectively used for the mineralization of PHC-polluted soils for decades. However, these biological treatments are still advancing and need to be understood. This comprehensive review explores the intricate realm of bioremediation as a sustainable and efficient approach for addressing PHC-contaminated soil. Delving into the principles and mechanisms that govern the process, the paper highlights the pivotal role played by microorganisms in remediation efforts. By examining recent advancements in bioremediation technologies, the present work enhances our understanding of the complex interplay between microorganisms and contaminated soil, ultimately contributing to the development of more effective and sustainable bioremediation strategies. Thus, the survey examines the very essence of bioremediation, unraveling the intricate mechanisms at play and spotlighting the silent stars-microbes-whose tiny prowess holds the key to ecological restoration. Beyond mere examination, this review projects us into the cutting-edge field of advancements, where scientific innovation and environmental stewardship converge as proof of the evolving story of our quest to heal the earth.
2 Classification of PHCs and their impact on soil, human health, and environment
Petroleum hydrocarbons occur naturally in great abundance and are complex heterogeneous mixtures containing mostly hydrocarbons and significant amounts of nitrogen, oxygen, sulfur, and trace amounts of metals. It is available in various forms, such as solid, liquid, and gaseous forms. Petroleum hydrocarbons were classified based on different criteria, as shown in Figure 2. Petroleum hydrocarbons can be divided into four main structural categories based on their geographical origin: saturates, aromatics, asphaltenes, and resins (Kebede et al., 2021). Depending on the source, crude oil consists of 82%–85% carbon, 10%–14% hydrogen, 0.01%–7% sulfur, 0.02%–2% nitrogen, and 0.1%–1% oxygen (Stepanova et al., 2022). Petroleum composition can be classified as light (density 0.65–0.87 g/cm3), intermediate (density 0.87–0.91 g/cm3), or heavy (density 0.91–1.05 g/cm3). The effect of light oils on plants is stronger than that of heavy oils (Stepanova et al., 2022). The light fractions of PHCs are quickly degraded by microbial activity; however, the heavy fractions of PHCs that spill into the soil cannot easily degrade and negatively affect the ecosystem and all living organisms (Ite and Ibok, 2019).
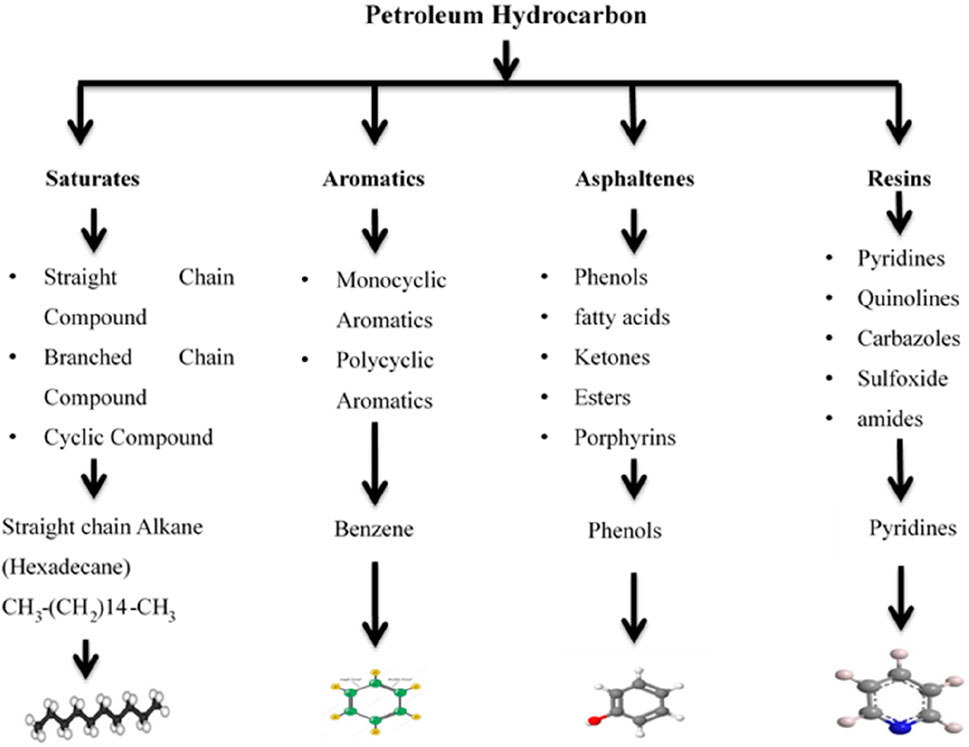
FIGURE 2. Classification of petroleum hydrocarbons. Reprinted with modification from (Koshlaf and Ball, 2017)
Petroleum hydrocarbon spillage is a global environmental problem. Recently, soil pollution by PHCs has increased tremendously, and many arable lands have been contaminated with organic compounds. This contamination reduces the availability of arable land and affects agricultural practices (Popoola et al., 2022). Owing to the persistent nature of petroleum hydrocarbons, spillage has negative consequences on soil properties and ecosystems, such as reduced soil fertility and food chain imbalances. This, in turn, adversely affects seed germination and alters the physicochemical, biochemical, and indigenous microbial diversity properties of the soil (Nassar and Said, 2021). Soil particles coated with hydrophobic films of high-molecular-weight PHC components lose their ability to absorb and retain moisture, which leads to a considerable loss of water conductivity and capacity (Gaur et al., 2021). As a result, the upper contaminated layers dry out, and the cleaner lower layers suffer from excessive moisture, leading to correct air and water conditions and anaerobic processes. Abnormal water conditions reduce the solubility and availability of micronutrients in plants, thereby inhibiting nitrification and ammonification. Higher carbon elevates the C: N ratio and decreases the concentrations of important elements, such as phosphorus and potassium. Thus, petroleum and its products mainly cause negative changes in all soil properties, from the soil layer morphology to humic acid chemistry (Stepanova et al., 2022).
The accumulation of spilled petroleum hydrocarbons in the soil at enormous levels is hazardous to human health and the environment. It is well known that soils are major dumps of organic contaminants of different origins and characteristics. PHC contain toxic components that pollute groundwater and soil, alter the soil microbial community, and affect human health and other living organisms (Chaudhary et al., 2021). PHCs are characterized by their high resistance to physical, chemical, and/or biological degradation and high persistence in the environment for long periods. PHCs can also be concentrated in living organisms (bioaccumulation), biomagnified in the food chain, undergo high movement through different environmental compartments, and exhibit high adsorption on soil organic matter. The toxic properties of PHCs can lead to environmental and human health problems (Morillo et al., 2020). Hydrocarbon contaminants cause immediate or latent effects, such as genetic mutations, immunotoxicity, teratogenicity, neurotoxicity, potent immune toxicity, chromosomal damage, carcinogenesis, high bioaccumulation potential, and deterioration of ecosystem functioning and treatment of animal and plant life (Chaudhary and Kim, 2019). Polycyclic aromatic hydrocarbons (PAHs) are highly hazardous pollutants owing to their high stability, toxicity, and carcinogenicity (Cui et al., 2020).
3 Bioremediation principles of PHC-contaminated soil
Bioremediation involves biological conversion, disintegration, or transformation of organic contaminants by microorganisms (bacteria and fungi), plants, or their metabolites, such as enzymes, to detoxify environmental pollutants (Huang et al., 2019). It involves either partial or complete mineralization of petroleum-hydrocarbon-polluted soil. Bioremediation techniques are classified into ex situ and in situ categories, based on site implementation. Among the ex-situ bioremediation methods, piles, bioreactors, composting, and land farming are the most common. Natural attenuation, bioventing, phytoremediation, biostimulation (addition of microbial growth-promoting formulations), and bioaugmentation (addition of microbial inoculation) are in-situ bioremediation techniques (Chaudhary and Kim, 2019). Bioremediation techniques are cost-effective and environmentally friendly, particularly when applied in-situ. Microbes can persist in hostile environments and remediate the natural conditions of soil, water, and sediments (Bala et al., 2022).
The classification of the bioremediation strategy is presented in Figure 3, and the principles of each remediation technology are highlighted in the subsequent sections.
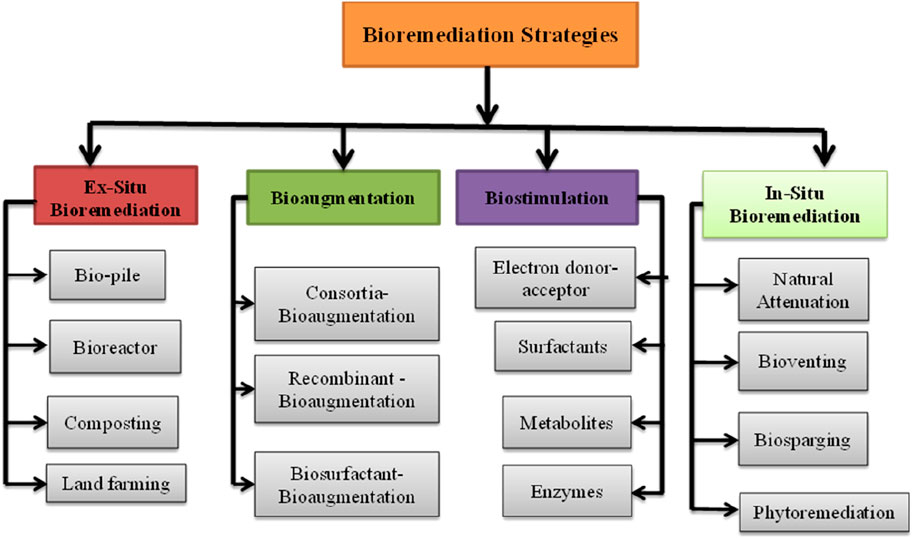
FIGURE 3. Bioremediation strategies for petroleum hydrocarbon-contaminated soils. Adapted with modification from (Chaudhary and Kim, 2019)
3.1 Bacterial remediation
Bioremediation utilizes the natural role of microorganisms and/or their enzymes in contaminated media to transform organic pollutants to levels at which they no longer pose risks to humans, animals, or plants.
Studies have shown that more than 200 species of bacteria can biodegrade PHCs, and various bacteria including Pseudomonas, Nocardia, Vibrio, Corynebacterium, Candida, Arthrobacter, Rhodotorula, Brevibacterium, Flavobacterium, Sporobolomyces, Achromobacter, Bacillus, Aeromonas, Thiobacillus, Acinetobacter, Lactobacter, Staphylococcus, Penicillium, Articulosporium, Halomonas, Klebsiella, Proteus, Aspergillus, Micrococcus, Neurospora, Rhizopus, Mucor, and Trichoderma are known to play vital roles in PHC degradation (Xu et al., 2018; Victor et al., 2020). The most commonly used bacterial remediation techniques are bioaugmentation and biostimulation or a combination of these two processes. Table 1 shows the bioremediation of PHC-polluted soil by the different bacterial strains.
3.1.1 Bioaugmentation
Bioaugmentation increases the extent of decomposition of complex contaminants through the addition of pollutant-degrading microbes and augments their diversity (Omokhagbor Adams et al., 2020). This process involves the addition of indigenous microbes or genetically modified microorganisms to contaminated sites under optimal conditions to accelerate the removal of unwanted compounds (Mrozik and Piotrowska-Seget, 2010).
Many investigations have been conducted on soil remediation using bioaugmentation. For instance, Oualha et al. (2019) investigated the biodegradation of weathered oil hydrocarbons using a biopile technology system along with bioaugmentation with optimum nutrients using an indigenous Bacillus sonorensis strain (Oualha et al., 2019). Bacillus sonorensis was introduced under optimized conditions: carbon/nitrogen/phosphorus (100/10/1), temperature (37°C), surfactant Tween 80 (0.12% (v/w)), and moisture (10%). The diesel range organics and polycyclic aromatic hydrocarbon (PAH) removal efficiencies of weathered oil-contaminated soil after 160 days were 39.2% and 32.4%, respectively, when ammonium nitrate was used as the nitrogen source.
The immobilized bacterial consortium of the more dominant Flavobacterium johnsoniae and Shewanella baltica strains achieved a maximum degradation efficiency of 93.32% for polyaromatic hydrocarbon-contaminated soil. The nanocomposite catalyst increased microbiological reaction rates by stimulating microbial activity during the biodegradation process. This excellent biodegradation efficiency suggests that the immobilized consortium has high potential for industrial applications in the biodegradation of PHC-contaminated soil (El-Sheshtawy et al., 2022). Similarly, Chaudhary et al. (2021) investigated the potential of a bacterial consortium comprising 64.69% Proteobacteria to treat diesel-contaminated aged soil using bioaugmentation along with nutrients and zero-valent iron nanoparticles. The results revealed that 99% of the total petroleum hydrocarbons found in the soil degraded within 60 days (Chaudhary et al., 2021).
In another study, colonies of heavy metal-resistant bacterial strains were isolated from sediments and cultured in Luria Bertani (LB) broth adjusted with 1% metal solution for crude oil and phenanthrene substrate remediation tests. Finally, the bacilli stain showed 94% and 85% degradation of crude oil and phenanthrene, respectively, after 28 days (Oyetibo et al., 2017). Varjani and Upasani (2016) investigated the native species of Pseudomonas aeruginosa NCIM 5514 obtained from petroleum-contaminated soil for off-site field bioremediation. During the ex situ bioremediation, the species exhibited a hydrocarbon degradation capacity of approximately 60.63%. According to these findings, P. aeruginosa can potentially be used for the ex situ and in situ mineralization of PHC contaminants (Varjani and Upasani, 2016). Qi et al. (2017) identified a new class of PAH-degrading genus, Gordonia strain Q8, in a nearby oil field area. These strains degraded naphthalene, phenanthrene, anthracene, and pyrene mixtures at rates of 100%, 95.4%, 73.8%, and 53.4%, respectively, after 7 days. The PAH mineralization efficacy of Gordonia strain Q8 was higher than those of Gordonia alkaliphila and Gordonia paraffinivorans strains (Qi et al., 2017).
3.1.2 Biostimulation
Biostimulation is the most commonly used method to accelerate the detoxification rate of PHC-polluted soil by adding nutrients, such as carbon, nitrogen, phosphorus, and oxygen (Martínez Álvarez et al., 2017). However, the concentration of added nutrients must be controlled, as it may cause a nutrient imbalance in the microflora. Biostimulation of PHCs stimulates bacteria by adding nutrients to severely polluted sites for rapid degradation of harmful and toxic contaminants (Guarino et al., 2017). Biostimulation strategies for PHC mineralization in the soil can be accelerated by the in-situ addition of the most prevalent nutrients that promote microbial growth (phosphate, salts, and nitrate) and highly efficient HC-degrading microbes (Torlapati and Boufadel, 2014).
Koshlaf et al. (2019) studied the biostimulation potential of pea straw, an agricultural by-product, to remove PAHs from the soil. The experimental conditions were as follows: PAH content (7,350 mg/kg−1), soil pH (6.5); C: N ratio, >22:1; and incubation temperature, 28°C. They found that pea straw enhanced the degradation efficiency of PAH-contaminated soil by 66.6% in 102 days, and that the microbial activity was also higher in the pea straw-amended soil than in the untreated soil. Various bacterial phyla were observed in the soil samples; however, the dominant phyla were Proteobacteria, Bacteroidetes, and Firmicutes (Koshlaf et al., 2019). In other studies, biochar from sawdust (Sd) and wheat straw (Ws) was prepared at 300°C and 500°C for PAH-polluted soil removal. The total concentrations of 16 PAHs were 3596.36 ± 80.38 mg/kg, and the soil was categorized as light clay and had a total organic carbon (TOC) content of 0.27%, total phosphorus of 0.04%, and pH of 7.61. The soil samples were incubated at 30°C for 84 days. These results revealed that the addition of biochar increased the degradation rate of PAHs with different rings. The wheat straw biochar prepared at 500°C showed higher degradation rates of PAHs with three aromatic rings, which increased to 69.95%, and the degradation rates of 4-, 5-, and 6-ringed PAHs were 45.96%, 37.92%, and 30.66%, respectively. Proteobacteria, Bacteroidetes, and Actinobacteria are the major bacterial species that degrade PAH (Kong et al., 2018).
Al-Kindi and Abed (2016) investigated the role of two organic wastes, spent mushroom compost (SMC), poultry manure (PM), and urea, in the bioremediation of oil-contaminated desert soil. The desert soil contained 42 mg TPH per Gram soil, silt loam soil with 50% silt, pH of 7.5, nitrate concentration of 0.4 mg L-1, and phosphate concentration of 1.6 mg L-1, and the experiment was conducted for 96 days at 30°C. The assessment showed that hydrocarbon degradation of C18-C30 alkanes was 19.3% of the initial concentrations in the untreated soil to 31.6% and 34% when SMC and PM were added to the soils, respectively. Firmicutes, Actinobacteria, and Proteobacteria have been identified as the dominant bacterial communities that degrade TPH-polluted soils during biostimulation decontamination (Al-Kindi and Abed, 2016).
Qin et al. (2013) studied the bioremediation capacity of petroleum-contaminated soil by a biostimulation process amended with rice straw biochar. The experimental conditions were as follows: soil pH (6.5), humidity (15.2 wt%), total organic carbon (5.42 wt%); total nitrogen (85 mg kg-1); total phosphorus (16 mg kg-1) (Qin et al., 2013). The results of the soil microcosm experiments showed that contaminant degradation efficiency was significantly higher in soils amended with biochar than in soils without biochar. The total petroleum hydrocarbon (TPH) removal efficiencies were 61.2%, 77.8%, and 84.8% in soils without biochar and amended with biochar on the beginning and 80th day, respectively. When biochar was added on the 80th day, the TPH concentration decreased to below the threshold level required for soil quality after 140 days.
Martínez Álvarez et al. (2017) assessed the potential of a pre-optimized biostimulation biopile field assay in the Antarctic region for bioremediation of hydrocarbon-contaminated soils (Martínez Álvarez et al., 2017). The soil had a sandy texture containing organic carbon, total nitrogen, and extractable phosphorous levels of 10.21 g kg−1 (1.02%), 0.32 g kg−1, and 5.0 mg kg−1, respectively, while the pH was 6.8, the water content was 10%, and the total hydrocarbon concentration was 2,180 ± 165 mg kg−1. In their work, N and P levels were optimized at a C: N:P ratio of 100:17.6:1.73 by response surface methodology at the laboratory scale before the biostimulation process that was applied for field assays. Two 0.5-ton piles were used as the experimental units: a control biopile (CC) and a stimulated system (BS) amended with N and P. Hydrocarbon removal was significantly higher in the BS system than in the CC system (75.79% and 49.54%, respectively), indicating that nutrient addition improved the development of bacterial flora.
3.2 Mycoremediation
Mycoremediation refers to the use of different species of fungi for the remediation of PHC-contaminated soils (Akhtar and Mannanul, 2020). Mycoremediation is a fungal species-assisted process for the complete mineralization of pollutants into CO2, H2O, N2, and microbial biomass after the completion of their metabolic activities (Rhodes, 2014). The unique morphology and diverse metabolic competence of fungi make them imperative biological agents for bioremediation of contaminants (Deshmukh et al., 2016). On the other hand, fungi have already been recognized for effective contaminant elimination and the progress of environmental biotechnology in the 1980s. Fungi are diverse, contain millions of species that are ubiquitous in various environments, and have been applied in diverse areas (Hawksworth and Lücking, 2017). Fungi can survive and grow well in terrific environmental situations through the distribution of spores and the secretion of various enzymes. Hence, considerable research has been conducted on the applied and fundamental aspects of mycoremediation in both soil and water (Anderson and Juday, 2016). Consequently, mycoremediation has become a firm technology for soil remediation without the threat of the spread of pollutants or their bioaccumulation in the food chain. The mycoremediation potential of PHC-polluted soils by the most studied and effective fungal strains is shown in Table 2.
Dhailappan et al. (2022) studied the degradation rate of PHC-contaminated soil using an edible oyster mushroom (Pleurotus ostreatus). Researchers have used paddy husks to stimulate remediation. The maximum TPH degradation rate was 87% after 21 days (Dhailappan et al., 2022). In another study, the degradation efficiency of a fungal consortium isolated from polluted soil was investigated to detoxify petroleum hydrocarbon-polluted soils. The results showed that the fungal community consisting of two isolates of Aspergillus sp. was the best consortium and had the highest potential to degrade hydrocarbons, mainly n-alkane-contaminated soil, at 50.61% in 16 days (Novianty et al., 2021). Naturally occurring microorganisms in soil matrices play a vital role in the mineralization of crude oil-polluted soil. This study investigated the degradation of conventional diesel, heating diesel fuel, synthetic diesel (syntroleum), fish biodiesel, and a 20% biodiesel–diesel blend by fungal communities under favorable environmental conditions. Fungal communities (Trichoderma sp., Penicillium sp. Giberella sp., Mortierella sp., and Fusarium species) remediation was observed with petroleum, which showed the highest total hydrocarbon decontamination rate of 48% in 28 days (Horel and Schiewer, 2020). Daâssi and Almaghribi (2021) explored the remediation of petroleum-hydrocarbon-contaminated soils using fungal species. After 60 days of incubation, the highest rate of TPH degradation was recorded at approximately 92% ± 2.35% by the Paecilomyces formosus strain (Daâssi and Almaghribi, 2021).
Marín et al. (2018) investigated the potential of endophytic fungi isolated from the stem and leaf of a plant in the Ecuadorian Amazon region for the degradation of oil-contaminated soil. A total of 156 endophytic fungi were isolated, and endophyte Xylaria sp. 1 showed the best TPH removal rate of 98.78% by infrared spectroscopy (IR) and gas chromatography 99.8% (GC) in an in vitro test for 30 days (Marín et al., 2018). Essabri et al. (2019) studied the degradation of TPH in petroleum-contaminated soil with fungi isolated from olive oil effluent using bioaugmentation and biostimulation methods. The results showed that the A. niger fungal isolate greatly degraded total petroleum hydrocarbons, with a degradation of approximately 71.19% at the end of the 60 days treatment (Essabri et al., 2019).
The petroleum hydrocarbon mineralization ability of fungi and plants was investigated by Ani et al. (2021), and the results confirmed that different rates of hydrocarbon degradation by isolated fungi were observed. An in vitro biodegradation study showed that Aspergillus niger, Fusarium solani, Curvularia lunata, and Trichoderma harzianum were the most effective in degrading kerosene (78%), diesel (70%), spent engine oil (83%), and crude oil (77%), respectively (Ani et al., 2021).
Kristanti et al. (2011) investigated microbial decomposition of crude oil-contaminated soil using the white rot fungal species Polyporus sp. S133. The strain was extracted from the PH-contaminated soil site and maintained using the inoculum enrichment technique at 4°C before use. The isolated fungal strain was then applied to a sample containing 200 g of soil and 3,000 mg of crude oil. The maximum degradation rate (93%) was obtained after 60 days of inoculation (Kristanti et al., 2011). Similarly, remediation of n-hexadecane hydrocarbons was investigated using the fungal strain Aspergillus sp. RFC-1 was obtained from oil-contaminated soil samples from the Rumaila oilfield in Basra, Iraq. The experimental results revealed that Aspergillus sp. RFC-1 degraded 86.3% of the initial n-hexadecane concentration after 10 days of incubation when used as the carbon source (Al-Hawash et al., 2018b). Another investigation was carried out by (Mohammadi-Sichani et al. (2019) to assess whether PHC-polluted soil was cleaned using spent mushroom compost as an inoculum for the bioremediation of oil-processing plant spills. The observed results showed that all composts of white-rot fungi, A. bisporus, P. ostratus, and G. lucidum, were able to degrade 71.5%, 69.5%, and 57.5% of the PHCs over 3 months. Among these strains, only A. bisporus showed a high ability to degrade TPHs (71.5%) (Mohammadi-Sichani et al., 2019). Its ability to degrade high- and low-molecular-weight PHCs makes spent mushroom compost potentially useful for mycoremediation. In another study, the biodegradation of phenanthrene was explored using the endophytic fungus Phomopsis liquidambari obtained from the interior of the stem bark of Bischofia polycarpa. The strain reduced more than 77% of the initial concentration of 50 mg/L phenanthrene substrate within 10 days of incubation (Fu et al., 2018). In contrast, a synergistic hydrocarbon (HC) remediation experiment using novel rhizospheric fungal strains in combination with potential plant roots was performed at different concentrations of crude oil-contaminated soil (Asemoloye et al., 2017). The strain was isolated from an aged oil-contaminated site and deployed along with a guinea grass root (Megathyrsus Maximus) for 90 days. The strain achieved 95.36% of total PAH mineralization at 30% and 40% contaminated soil sample concentrations. In addition, the synergistic action of fungal consortia and Megathyrsus maximus roots accelerates the hydrocarbon degradation rate and soil remediation and enriches soil nutrients. Evidence has also shown that fungal strains of marine origin, such as Tinctoporellus sp. CBMAI 1061 and Marasmiellus sp. CBMAI 1062, and Peniophora sp. CBMAI 106) effectively detoxifies large-molecular-weight pyrene and benzo [a]pyrene (BaP) (Vieira et al., 2018). Marasmiellus sp. were subjected to pyrene degradation and were able to completely degrade pyrene after 48 h of incubation. Correspondingly, the ligninolytic fungus Ganoderma lucidum CCG1, extracted from the hardwood stump, degraded phenanthrene and pyrene. G. lucidum could degrade 99.6% of phenanthrene and pyrene in a salt medium after 30 days at 27°C. G. lucidum is a strong PAH degrader in contaminated soil environments (Agrawal et al., 2018). Similarly, the white-rot fungus Coriolopsis byrsina strain APC5 decontaminates pyrene under various soil conditions. The highest pyrene degradation (96.1%) was obtained using ligninolytic enzymes at pH 6°C and 25°C. Based on this examination, C. byrsina APC5 strain can also be used as a potential degrader for PAH contaminants from the contaminated soil environment (Agrawal and Shahi, 2017)
4 PHC-contaminated soil remediation mechanism
Various microorganisms have the potential to mineralize PHC-polluted soil by metabolizing organic contaminants into nontoxic carbon dioxide, water, and biomass using them as the sole energy source (Ji et al., 2013). Microorganisms can activate and oxidize hydrocarbon compounds with the involvement of microbial electron acceptors, such as oxygen, nitrate, manganese, iron, and sulfate microbes in the biotransformation process of PHC-contaminated soil. The activation of aerobically degraded PHCs is achieved by different enzymes. The addition of molecular oxygen to the substrate is catabolized by oxygenase enzymes, whereas the addition of two hydroxyl groups to PHC-contaminated soil is detoxified by dioxygenases or monooxygenases (Wentzel et al., 2007). There are two major mechanisms for PHC detoxification. aerobic and anaerobic pathways). Oxygen is used as an electron acceptor in aerobic mechanisms, whereas sulfate and nitrite accept electrons to complete the process under anaerobic conditions (Mbachu et al., 2020). Anaerobic degradation occurs at a slower rate than that of aerobic microbial degradation. This review presents the mechanisms of remediation of PHC-polluted soil by bacteria and fungi.
4.1 Bacterial remediation mechanism
This review briefly elucidates the aerobic and anaerobic degradation pathways and mechanisms of n-alkane degradation in soil using bacterial strains. The PHC-saturated fraction is composed of n-alkanes ranging from C1 to > C40 (Abbasian et al., 2015).
4.1.1 Aerobic biodegradation pathways of N-alkanes
The aerobic bacterial metabolic pathways involved in PHC transformation are primarily intracellular. Degradation catabolism of alkanes is initiated by oxygenases, which introduce oxygen atoms into n-alkanes via four different pathways. The terminal oxidation pathway involves oxidation of the n-alkane terminal methyl group (Perera et al., 2022). The products of oxidation are primary alcohols, which are further oxidized to fatty acids by alcohol and aldehyde dehydrogenases, and then undergo β-oxidation (Fordwour et al., 2018). This oxidation pathway is found in various bacterial microbes such as Geobacillus thermodenitrificans NG80-2 (Ji et al., 2013). The terminal oxidation pathway is the second oxidative pathway, in which the termini of the n-alkane undergo oxidation to the corresponding fatty acid without rupturing the carbon chain. The yield of this oxidation pathway is a ω-hydroxy fatty acid that is further converted to a dicarboxylic acid, which then undergoes β-oxidation (Winkler and Gray, 2015). The third oxidative pathway is subterminal oxidation, in which alkanes are oxidized at the subterminal position to form primary and secondary alcohols or methyl acetone with the same chain length as the substrate (Yin et al., 2022). Subterminal oxidation of n-alkanes, which degrades propane into secondary alcohols, has been recognized in Gordonia sp. strain 5. The secondary alcohol is further converted to a ketone and then oxidized by Baeyer–Villiger monooxygenase to form an ester. This ester is hydroxylated by esterases to produce alcohols and fatty acids (Ji et al., 2013). The fourth oxidative pathway is a long-chain n-alkane oxidation pathway that is unique to Acinetobacter sp. strain HO1-N (Singer and Finnerty, 1985). In this oxidative pathway, n-alkanes are oxidized to form n-alkyl hydroperoxides, peroxy acids, alkyl aldehydes, and fatty acids. The first step of the Finnerty pathway is the formation of dioxygenase, which is responsible for the first step in the oxidation of n-alkanes by Acinetobacter sp. (Maeng et al., 1996). The aerobic degradation mechanism of n-alkanes and aromatic hydrocarbons is shown in Figures 4, 5.
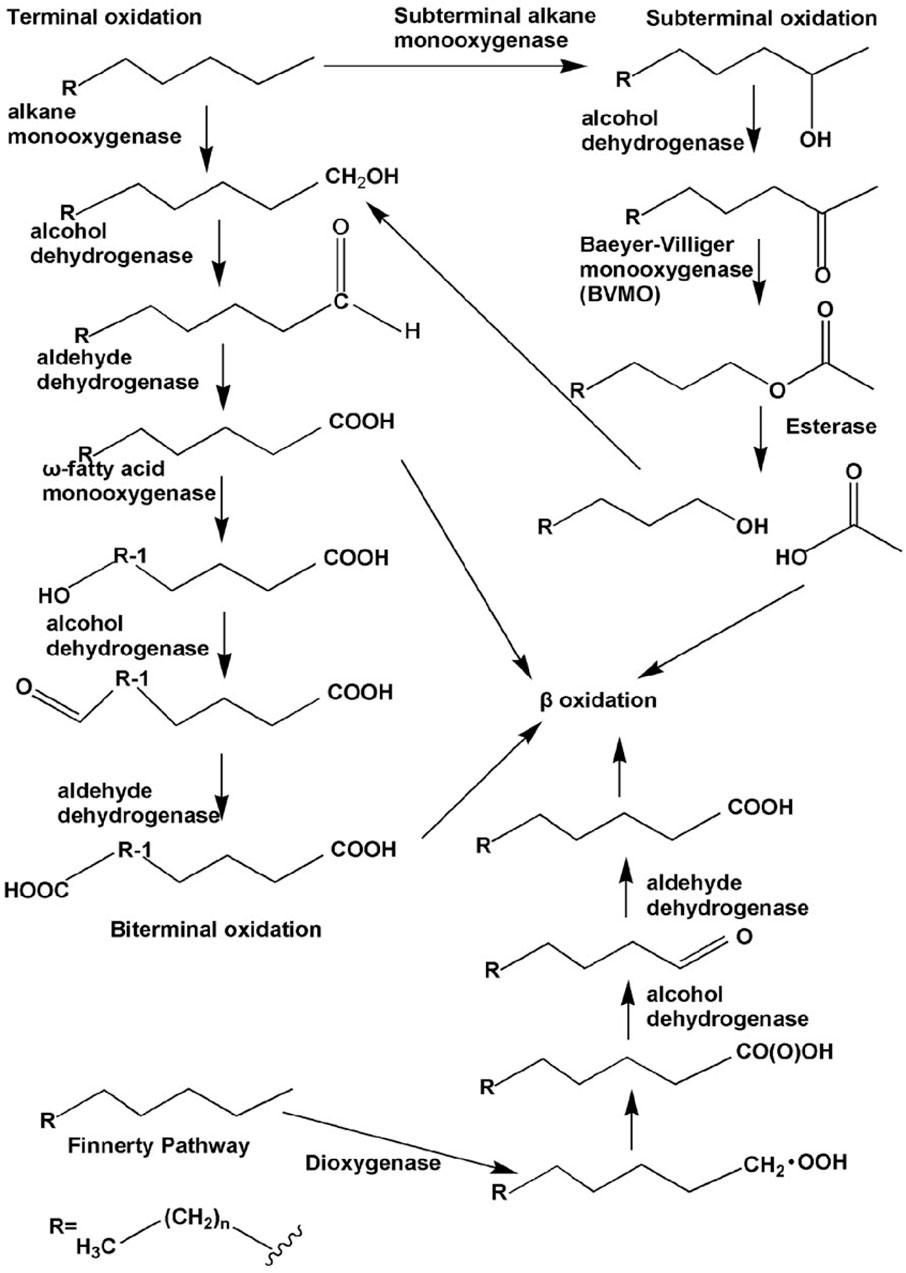
FIGURE 4. Different types of aerobic degradation pathways of n-alkanes by bacteria. Reprinted from (Ji et al., 2013).
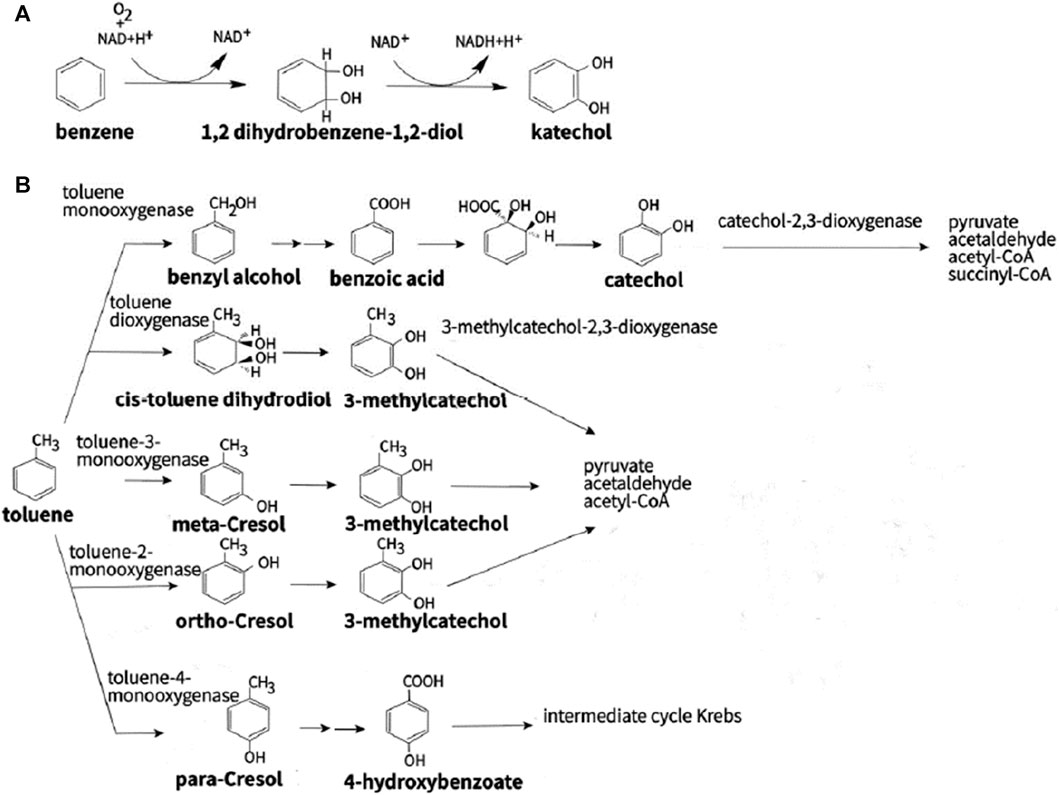
FIGURE 5. Different types of aerobic degradation pathways aromatic compounds by bacteria using (A) benzene and (B) toluene. Reprinted from (Stepanova et al., 2022)
4.1.2 Anaerobic biodegradation pathways of N-alkanes
Recent investigations have shown that petroleum hydrocarbons, including alkanes, are degraded in the absence of oxygen using nitrate, ferrous iron, or sulfate as electron acceptors, or under methanogenic conditions (Ji et al., 2013). Anaerobic biodegradation of PHCs is important in cold regions where oxygen levels are limited, and it has gained more attention because it is considered the predominant mechanism occurring in several polluted environments and oil reservoirs (Yap et al., 2021). Several anaerobic bacterial strains, such as sulfate-reducing bacterial strains (Musat, 2015) and denitrifying bacterial strains (Heyen et al., 2021), can degrade n-alkanes with six or more carbons into CO2 and other less toxic compounds. Currently, there are two known mechanisms for anaerobic degradation of n-alkanes: the fumarate addition pathway and the carboxylation pathway, as shown in Figure 6.
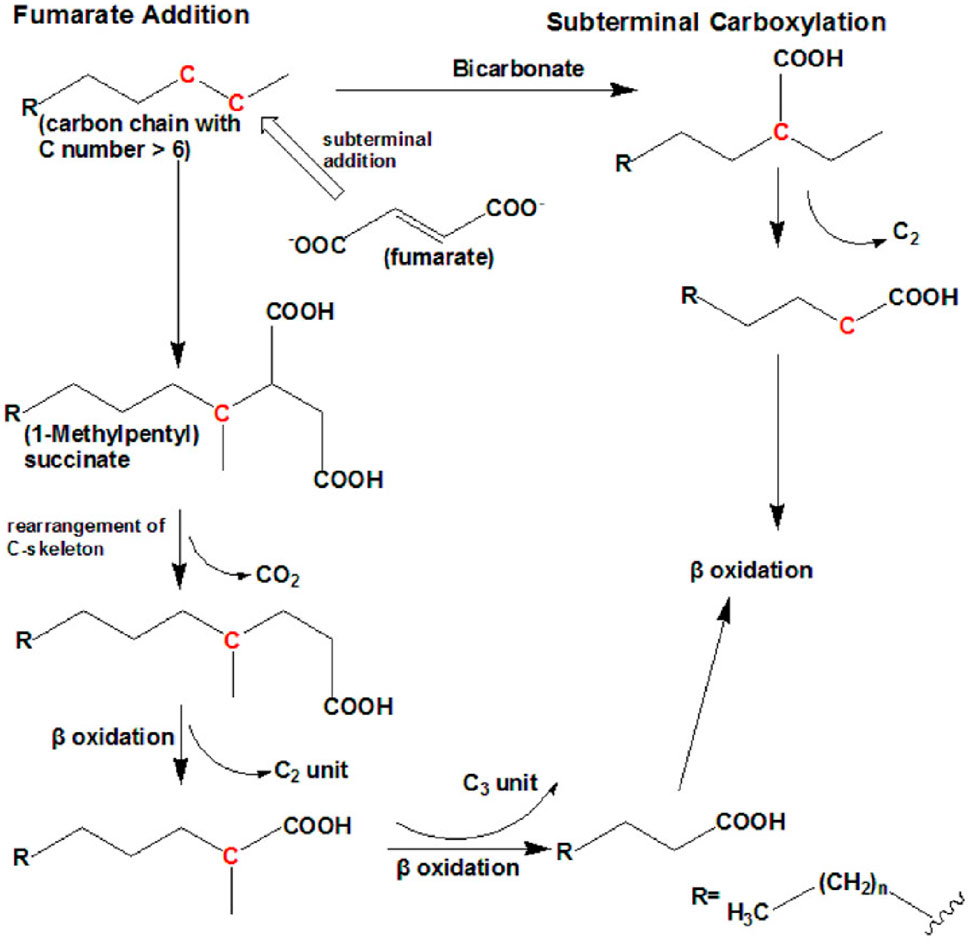
FIGURE 6. Anaerobic bacterial degradation mechanisms in n-alkane-polluted soil. Reprinted from (Ji et al., 2013).
The fumarate addition pathway has been identified in sulfate-reducing bacteria, denitrifying bacteria (HxN1), and a nitrate-reducing consortium (Callaghan et al., 2006). The sulfate-reducing bacteria Desulfatibacillum aliphaticivorans CV2803T (Cravo-Laureau et al., 2005) and Desulfatibacillum alkenivorans AK-01 (So and Young, 1999) anaerobically oxidize n-alkanes to fatty acids via the subterminal addition of fumarate to an alkane at the C-2 position by alkyl succinate synthase to form (1-methyl alkyl) succinate. AK-01 could grow on C13–C18 chain-length n-alkanes, 1-hexadecene, and 1-pentadecene. Alkyl succinate was further degraded via carbon skeleton rearrangement and β-oxidation. After carbon rearrangement and decarboxylation, a methylated fatty acid with two carbons larger than the original n-alkane is formed (Callaghan et al., 2006). The resulting fatty acids were then funneled into the β-oxidation pathway.
The carboxylation pathway for anaerobic alkane degradation is mainly developed by the sulfate-reducing bacterium strain (Hxd3), which is also known as Desulfococcus oleovorans (Aeckersberg et al., 1991). To date, Hxd3 is the only known strain that is anaerobically degraded via the subterminal carboxylation pathway. Strain Hxd3 anaerobically transforms an alkane into a fatty acid through subterminal carboxylation at the C-3 position of the alkane, followed by the elimination of two adjacent terminal carbon atoms (Fuentes et al., 2014). In this mechanism, the initial attack of alkanes involves both carboxylation with inorganic bicarbonate and the removal of two carbon atoms from the alkane chain terminus, resulting in a fatty acid that is shorter by one carbon than the original alkane. In other words, the Hxd3 strain can transform C-odd alkane substrates to C-even fatty acids and vice versa.
4.2 Fungal remediation mechanism
The fungal remediation mechanism in this review elucidates the microbial pathways involved in decontaminating PAH-polluted soil. The mechanisms of detoxification of PAH-polluted soil by fungi are quite different from those of bacterial remediation catabolism pathways. Fungi secrete a series of intracellular and extracellular enzymes such as lignin peroxidase and manganese peroxidase to remove PAHs from the soil (Reyes-César et al., 2014). Based on the group of fungi and secreted enzymes, the degradation of PAH-polluted soil by fungi can be classified as ligninolytic fungi (a group that secretes enzymes to break down lignin in wood) and non-ligninolytic fungi (a group that does not secrete these enzymes) (Marco-Urrea et al., 2015).
White-rot fungi (WRF) and other macrofungi are a major group of fungi that secrete ligninolytic enzymes to degrade lignin in wood and other organic material considered to be ligninolytic fungi (Rathankumar et al., 2022). Ligninolytic enzymes, such as laccases and peroxidases, are produced extracellularly and oxidize PAHs via nonspecific radical-based reactions. The two major types of peroxidase ligninolytic enzymes are manganese peroxidase and lignin peroxidase, which have the potential to oxidize PAHs into non-toxic forms using direct or indirect oxidation (Al-Hawash, 2018). Under ligninolytic conditions, WRF can oxidize PAHs by generating free radicals (hydroxyl free radicals) via donation of one electron, which oxidizes the ring of PAH and converts it into quinones. Overall, ligninolytic fungi mineralize PAHs into quinones and further into inorganic compounds, such as carbon dioxide and water, as shown in Figure 7. On the other hand, the most common PAH-degrading non-ligninolytic fungi are ascomycetes, zygomycetes, hyphomycetes, Aspergillus sp., Cunninghamella, and Penicillium (Rathankumar et al., 2022). The initial step of PAH oxidation by non-ligninolytic fungi is an intracellular cytochrome P450 monooxygenase enzyme (CYP450)-stimulated reaction that forms arene oxide and water (Rathankumar et al., 2020). PAHs adsorbed by the fungal mycelium system are oxidized by the incorporation of a single oxygen molecule and transformed into unstable arene oxide, which is either non-enzymatically transformed into a phenol derivative or hydrated by epoxide hydrolase into trans-dihydrodiols (Dickson et al., 2019). The final phenol derivatives are subjected to enzymatic transformation and can act as substrates for subsequent methylation, sulfation, or conjugation with glucuronic acid, xylose, or glucose (Reyes-César et al., 2014).
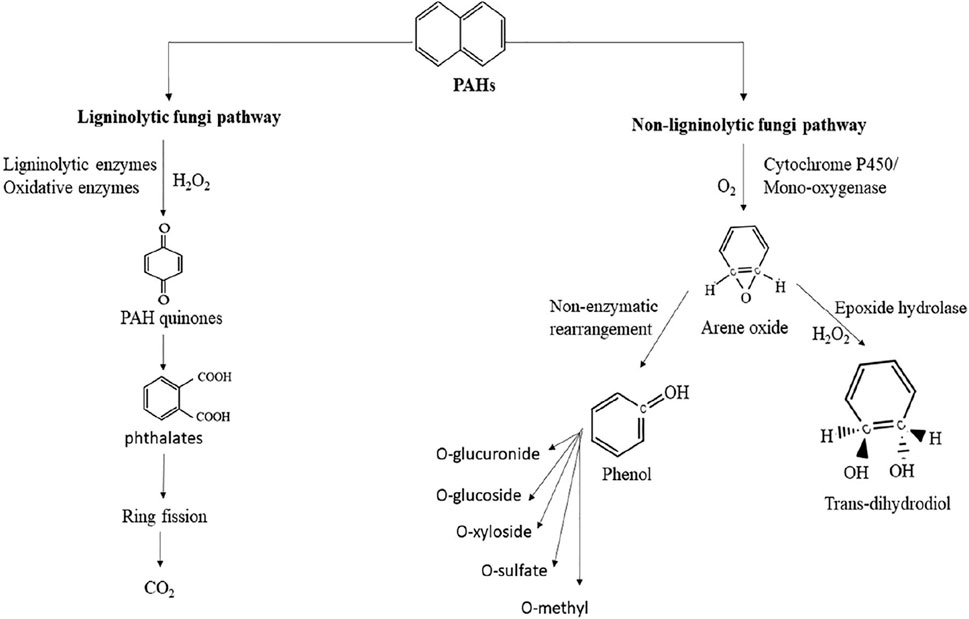
FIGURE 7. Mechanism of PAHs degradation by ligninolytic and non-ligninolytic fungi. Reprinted from (Rathankumar et al., 2022).
Fungi are well-known organisms that can degrade lignocellulose, which is the most abundant renewable material available in nature, into desired monomeric products (Kumar and Chandra, 2020). Fungi produce lignolytic enzymes that break down polysaccharides, cellulose, hemicellulose, and lignin, which are natural aromatic polymers. Ligninolytic enzymes constitute a group of oxidoreductase enzymes that are highly specialized in the degradation of lignolytic biomass (Chukwuma et al., 2020). The structure of lignin macromolecules determines the properties of the degrading enzyme system, which is extracellular, nonspecific, and nonhydrolytic. White-rot fungi (WRF) are the most common fungal microbes and are potential lignolytic enzyme-producing microorganisms (Weng et al., 2021). They produce four main lignin-modifying enzymes, lignin peroxidase, manganese peroxidase, laccase, and versatile peroxidase, which are capable of completely mineralizing PHCs from contaminated soil.
White-rot fungi are potential microbes that are active against a wide range of organic compounds because they release extracellular lignin-modifying enzymes (LMEs) with low substrate specificity, allowing them to act on various molecules that are broadly similar to lignin (Dao et al., 2021). LMEs, such as lignin peroxidase (LiP), manganese peroxidase (MnP), various H2O2-producing enzymes, and laccase are potential enzymes for the degradation of lignin materials. However, these enzymatic activities may not be observed in lignolytic fungi. LiP, MnP, and versatile peroxidase (VP) have potential synergistic actions during lignin degradation (Falade et al., 2017). In addition to fungal oxidative enzymes, chemical reactions during lignin biodegradation produce fungal degraded lignin metabolites such as phenolics and other aromatic compounds, peptides, organic acids, and metal ions (Wang et al., 2018). It can be concluded that fungi, especially WRF, are potential microorganisms that can degrade a wide range of toxic and emerging contaminants, including PHC.
5 Factors affecting bioremediations of petroleum hydrocarbon pollutants in soil
Effective biological applications to restore contaminated soil involve the identification and knowledge of the site and limiting factors for the microbial degradation of pollutants. Many factors controlling the biodegradation of PHCs have been documented (Kebede et al., 2021). The major factors affecting bioremediation of PHC-polluted soils are discussed below and are shown in Figure 8.
5.1 Substrate properties and concentration
The mineralization of organic PCH to nontoxic inorganic compounds is heavily affected by the amount, composition, and inherent properties of the substrate. Evidence has shown that higher TPH concentrations in soil are toxic to microbes involved in degradation, and affect growth and remediation activity (Towell et al., 2011). However, extremely low TPHs provide marginal support for microbial growth and carbon supply as energy sources, leading to microbial decomposition of TPH inhibition in the soil. Moreover, the degree of PHC degradation is influenced by the chemical structure, composition, and bioavailability of PHC (Ramadass et al., 2018). Microbial decomposition of HCs can be ranked in the following order: linear HCs > branched HCs > low-molecular-weight alkyl aromatics > single aromatic rings > cyclic HCs > PAHs > asphaltenes (Koshlaf and Ball, 2017). Furthermore, biodegradability naturally depends on the composition of the contaminant, and less complex compounds are preferentially utilized by HC degraders (Shanmugaraju et al., 2013). For example, kerosene and saturated and/or aromatic crude oils, which consist of medium-chain alkenes, can be completely biodegraded. However, crude oil from heavy asphaltic-naphthenic acid may be degraded with low efficiency by several microbial species, but some novel strains, such as Pseudoalteromonas sp., can effectively degrade it under anaerobic conditions (Zan et al., 2022).
5.2 Temperature
Temperature plays a paramount role in microbial remediation of organic contaminants (Varjani and Upasani, 2019). It affects both the chemistry and physiology of pollutants and the microbial flora (Chandra et al., 2013). Generally, temperature influences the microbial metabolism, microbial growth, solubility, and solid phases of the soil (Megharaj et al., 2011). For instance, higher temperatures enhance the water solubility of hydrophobic contaminants. In addition, higher temperatures decrease the viscosity of the contaminant, which enhances the diffusion of long-chain n-alkanes into the aqueous phase. Conversely, low temperatures cause increases in oil viscosity, reduced vaporization of noxious short-chain alkanes, and a decrease in water solubility, leading to a lag at the outset of microbial degradation (Ajona and Vasanthi, 2020). Although HC microbial degradation can occur over a range of temperatures, the speed of biological degradation decreases because of the limited enzymatic activity at decreasing temperatures (Fuentes et al., 2014). In addition, temperature affects the rate of degradation, and the physicochemical composition of oil leads to enriched hydrocarbon bioavailability in the microbial communities (Si-Zhong et al., 2009). Generally, the maximum bioremediation level occurs at 30°C–40°C in the soil environment, 20°C–30°C in freshwater systems, and 15°C–20°C in marine environments (Pithawala et al., 2012). Recently, researchers have investigated thermophilic microbes as alternatives to optimize the effect of temperature on the bioremediation of contaminants that can be operated at elevated temperatures (Aragaw et al., 2022). These microorganisms can adapt to stress and regulate their mechanisms under harsh conditions.
5.3 Nutrients availability
Nutrient accessibility also plays a significant role in microbial activity during the remediation of PH-contaminated soil. Typically, nutrients such as carbon, phosphorus, and nitrogen have a substantial impact on microbial growth for remediation of hydrocarbons (Dias et al., 2012). According to a review report by Varjani, PHCs are rich in carbon and energy sources but do not contain a significant amount of nitrogen and phosphorous needed to promote microbial growth (Varjani, 2017). However, the deficit in C-N-P-K ratios can be preserved by expanding CH₄ N₂ O, PO₄³⁻, N-P-K fertilizers, and NH₄⁺ with the most adequate 100:10:1 C: N:P ratio. Nitrate has been reported to be a nitrogen source for the growth and production of amphiphilic compounds (Toda and Itoh, 2012). As a result, petroleum-contaminated site treatment in the presence of nitrogen increases microbial cell growth and HC degradation rates. In addition, the presence of nitrogen in the culture decreases the PAH degrader growth lag phase of PAHs and maintains microbes at high pollutant degradation activity levels (Peng et al., 2008). However, it was revealed that an excess concentration of nitrogen in the soil causes inhibition, and maintaining its concentration (<1800 mg N2/kg H2O) provides optimal biological degradation of HC pollutants (Walworth et al., 2007). Likewise, the unbalanced high nutrient content of NPK inhibits biodegradation of HC contaminants (Boopathy, 2000). Furthermore, high quantities of NPK in the contaminated sites disrupted the C:N:P ratio, causing an oxygen deficit in the remediation sites. The soil type and organic matter in the soil significantly affected bioremediation. For example, the occurrence of high concentrations of soil organic matter in fine soils enhances microbial tumors and stimulates the mineralization of HCs (Scherr et al., 2007).
5.4 Soil characteristics
The soil type also plays a critical role in the success of bioremediation. A higher rate of hydrocarbon degradation occurred in the order of silky soil > sandy soil > clay soil because of the poor microbial content in the sand fraction and clay, which corresponded to a high C:N ratio and lower internal surface structure. For instance, more than 70% degradation of TPH was achieved in sandy soil and was relatively low in clay soil (Haghollahi et al., 2016). Clay soil develops a high surface area adhesive surface in water, resulting in a lower bioremediation rate due to the blockage of effective oxygen transmission through the soil. In addition, the robust adsorption of impurities on the surface of clay soil particles causes low degradation of HCS. In contrast, sandy soils have higher porosity than clays and permit superior oxygen transmission, allowing for the effective biodegradation of hydrocarbons. Moreover, the high porosity of sandy soils offers adequate space for microbial growth (Haghollahi et al., 2016). In conclusion, soil with a high porosity and oxygen transfer capacity has an improved HC bioremediation rate.
5.5 Oxygen availability
Oxygen is a pivotal element in the bioremediation of petroleum hydrocarbon-polluted soil, and the first biodegradation step begins with the presence of oxygen in PHC-polluted soil. Molecular oxygen is the most common electron acceptor element because most hydrocarbon substances are highly reduced substrates that require an electron acceptor. Providing a sufficient oxygen supply for the complete mineralization of PHC contaminants in soil is often challenging and expensive (Abbasian et al., 2015). In many aerobic bioremediation sites, oxygen is a crucial limiting factor that affects the complete decomposition of PHCs in the soil. Therefore, the addition of oxygen can significantly improve the remediation rate of microbial mineralization of PHCs and oxygenase-catalyzed remediation reactions (Waigi et al., 2015). It has been reported that aerobic microbial degradation is favorable for the participation of oxygenase and soil. The first steps in the catabolism of aliphatic (Peng et al., 2008), cyclic (Perry and Gibson, 1977), and aromatic (Elufisan et al., 2020) hydrocarbons by bacteria and fungi require molecular oxygen to be oxidized by oxygenase enzymes. However, the availability of oxygen in soil is dependent on a number of factors, such as the microbial oxygen consumption rate, type of soil, whether the soil is waterlogged, and the presence of utilizable substrates that can lead to oxygen depletion (Wang et al., 2021). The concentration of oxygen has been identified as a rate-limiting factor (Kumar et al., 2019). For example, soil usually requires 2 × 106 m3 of water saturated with 10 mg/L O2 for the effective oxidation of 10 m3 of hydrocarbons to carbon dioxide and water (Koshlaf and Ball, 2017). Therefore, dissolved oxygen in soil is crucial for the subsequent degradation of hydrocarbons and the active involvement of oxygenase enzymes.
5.6 Microorganisms and inoculums
The presence of active hydrocarbon-utilizing microorganisms is vital for bioremediation. Microbial groups such as bacteria, fungi, algae, protozoa, and viruses are the most widely used degraders of hydrocarbon contaminants (Ubani et al., 2016). These microbial strains can persist and use pollutants as sources of growth and metabolism in contaminated soils. Therefore, contaminated soils must contain a sufficient number of active hydrocarbon-utilizing microorganisms (Perelo, 2010). In contrast, the microbial inoculum size affects the breakdown of PHC pollutants. For instance, it has been reported that 106 CFU/g soil microbes are optimal for PHCs degradation (Towell et al., 2011).
5.7 Soil pH
Soil acidity and alkalinity are significant factors in petroleum hydrocarbon biodegradation. Unlike most aquatic ecosystems, soil pH can be extremely variable, ranging from 2.5 in mine spoils to 11.0 in alkaline deserts (Wang et al., 2021). Soil pH inhibits microorganisms and enzyme activity, whereas most microbes prefer neutral pH for growth and HC remediation (Kumar et al., 2019). Most microorganisms prefer neutral or low-alkaline conditions to decompose hydrocarbon compounds (Wang et al., 2016; Muangchinda et al., 2018). For instance, most heterotrophic bacteria and fungi prefer a pH near neutral, with fungi being more tolerant to acidic conditions (Al-Hawash et al., 2018a). Therefore, the ability of the microbial population to degrade hydrocarbons is expected to be negatively affected by increased pH, as has been observed in some soils. Hence, the optimum microbial growth rate and HC biodegradation can be maintained at pH values between 6 and 9 with inadequate nutrient and oxygen supplies (Das and Chandran, 2011). Additionally, PHCs have ecotoxic effects on bacterial activity, plant species, and earthworms, resulting in reduced biodegradation rates (Tang et al., 2011).
5.8 Soil salinity
Soil salinity also affects plants and microorganisms. Higher salinity may decrease the growth of microbes in water and soil. Additionally, some microbial communities are more prone to salinity. Increased salt concentration reduces microbial growth by inhibiting enzyme secretion due to the high osmotic pressure of the surrounding environment (Qin et al., 2012; He et al., 2017), reducing available oxygen to microorganisms (Liang et al., 2017) and hydrocarbon compound solubility (Truskewycz et al., 2019), and also causes cell membrane lysis (Muangchinda et al., 2018). Typically, extreme salt concentrations inhibit bacterial growth and cause bacterial cell dehydration, leading to cell death. Furthermore, high salinity levels decrease the HC metabolism rate as a source of carbon and energy. Consequently, high salt concentrations deactivate HC degraders and soil decontamination becomes ineffective (Rath et al., 2016). Several investigations have shown that lower salinities increase mineralization of HCs, but extremely low salinities significantly reduce biodegradation (Qin et al., 2012). Table 3 shows the factors affecting bioremediation of PHC-polluted soils.
6 Prospects and conclusion
6.1 Prospects
Petroleum hydrocarbon biodegradation metabolic pathways and routes are poorly understood, and inefficient transformation results in the accumulation of toxic intermediate substances that can resist further degradation by existing pathways. Hence, appropriate inoculum selection, optimized inoculation time, reaction conditions (aerobic, anoxic, and anaerobic), and physicochemical parameters are critical for the complete and effective bioremediation of petroleum hydrocarbon contaminants. Furthermore, recombinant DNA approaches have been introduced in the microbial bioremediation of contaminants to evolve the desired gene manipulation of native microorganisms and their degradation pathways to enhance their bioremediation potential by obtaining novel contaminant-degrading genes and enzymes. Although several approaches have been developed in the field of bioremediation of petroleum hydrocarbons, much remains to be explored. In this regard, the strengths, weaknesses, opportunities, and threats in the field of PHC remediation are crucial for developing a comprehensive understanding of bioremediation prospects.
Strengths:
• PHC-contaminated sites can be cleaned in a sustainable and eco-friendly manner by utilizing natural microbial processes.
• In many cases, bioremediation of PHCs is more cost-effective and involves less equipment and labor than conventional soil remediation practices.
• Different PHC contaminants can be remediated by using a diverse range of microorganisms.
• Microbes can be adapted to target specific hydrocarbon pollutants, thereby improving the efficiency of remediation processes.
Weaknesses:
• Microbial degradation of contaminants, including PHCs, is time consuming.
• Bioremediation of PHCs is highly site-specific with respect to soil type, climate, and contaminant concentration.
• The incomplete degradation of PHCs may introduce harmful chemicals into the environment.
Opportunities:
• Continuing progress in biotechnology can lead to the creation of more efficient and robust microorganisms for PHCs bioremediation.
• Rising technologies, such as genetic engineering and synthetic biology, offer opportunities to enhance microbial strains and degradation efficiency.
• Bioremediation technology can be integrated with other sustainable practices, such as agroecology, to offer universal solutions for PHC-contaminated soil remediation.
• Collaborations with industries and other scientific fields, such as chemistry and engineering, involved in bioremediation technology, can lead to the development of innovative solutions and improved methodologies.
Threats:
• Traditional chemical remediation techniques may still be preferred because of their apparent speed and instant results, posing a threat to the widespread adoption of bioremediation.
• The introduction of genetically engineered microorganisms or other bioremediation microbes poses a threat to the ecosystem.
• Changes in environmental conditions such as temperature and pH can affect the success of bioremediation, posing a threat to its reliability.
• Rapid industrialization may introduce new and challenging HC contaminants that develop resistance to microbial degradation, leading to reduced effectiveness over time.
6.2 Conclusion
The use of petroleum and petroleum products as energy sources contributes to oil spills in the environment, presenting a challenge for the remediation of polluted sites. Consequently, petroleum hydrocarbon contamination poses significant environmental pollution, and bioremediation has emerged as a promising and sustainable approach for mitigating its impact on soil ecosystems. This review comprehensively evaluates the current state of knowledge to contribute to a better understanding of the complexities involved in bioremediation processes and highlights potential avenues for future research and applications in the restoration of PHC-contaminated soil. From the evaluation of the aforementioned discussion of bioremediation efforts, the following conclusions and recommendations were drawn.
• Microbial consortia, such as bacteria and fungi, and the degradation mechanisms employed by them showcase the diverse and efficient nature of biological processes in breaking down complex hydrocarbons.
• Many methods/techniques, such as bioaugmentation and biostimulation, offer promising paths for accelerating the degradation of petroleum hydrocarbons and tailoring solutions to specific contamination scenarios.
• The effectiveness of PHC bioremediation is influenced by site-specific factors and other challenges.
• Microbial activity, nutrient availability, and environmental conditions play pivotal roles in the success of bioremediation.
• Progress in bioremediation technologies has highlighted continual improvements in optimizing strategies for enhanced soil restoration.
• Investigation of interactions between bioremediation and other technologies, such as phytoremediation or chemical treatments, to establish integrated and more thorough methods for PHC clean-up.
• Devote research and development to boost our understanding of microbial consortia and their synergies with different PHCs.
• Invest in research to find and develop efficient microbial strains with enhanced capabilities for degrading specific PHCs.
• Novel nutrient distribution systems must be exploited for the targeted delivery of essential nutrients to contaminated sites for optimal microbial growth and hydrocarbon degradation.
Author contributions
BM: Data curation, Formal Analysis, Investigation, Visualization, Writing–original draft, Methodology. TA: Conceptualization, Investigation, Methodology, Supervision, Validation, Visualization, Writing–review and editing. MG: Data curation, Formal Analysis, Investigation, Writing–original draft.
Funding
The author(s) declare that no financial support was received for the research, authorship, and/or publication of this article.
Acknowledgments
We would like to thank the organizations and scholars who have used the previous findings related to this work. The authors also apologize to all intellectuals and organizations whose involvement in the field of bioremediation of PHC-contaminated soil may have been reviewed by mistake or inadequately recognized.
Conflict of interest
The authors declare that the research was conducted in the absence of any commercial or financial relationships that could be construed as a potential conflict of interest.
Publisher’s note
All claims expressed in this article are solely those of the authors and do not necessarily represent those of their affiliated organizations, or those of the publisher, the editors and the reviewers. Any product that may be evaluated in this article, or claim that may be made by its manufacturer, is not guaranteed or endorsed by the publisher.
Abbreviations
HCs, Hydrocarbons; PHCs, Petroleum hydrocarbons; PAHs, polycyclic aromatic hydrocarbons; TPHs, total petroleum hydrocarbons; MYE, malt yeast extract; LB, Luria broth; C-N-P-K, carbon/nitrogen/phosphorus/potassium ratio; CH₄ N₂ O, Urea; PO₄³⁻, Phosphate; N-P-K, Nitrogen-Phosphorous-Potassium fertilizers; NH₄⁺, Ammonium.
References
Abbasian, F., Lockington, R., Mallavarapu, M., and Naidu, R. (2015). A comprehensive review of aliphatic hydrocarbon biodegradation by bacteria. Appl. Biochem. Biotechnol. 176, 670–699. doi:10.1007/s12010-015-1603-5
Aeckersberg, F., Bak, F., and Widdel, F. (1991). Anaerobic oxidation of saturated hydrocarbons to CO2 by a new type of sulfate-reducing bacterium. Arch. Microbiol. 156, 5–14. doi:10.1007/BF00418180
Agrawal, N., and Shahi, S. K. (2017). Degradation of polycyclic aromatic hydrocarbon (pyrene) using novel fungal strain Coriolopsis byrsina strain APC5. Int. Biodeterior. Biodegr. 122, 69–81. doi:10.1016/j.ibiod.2017.04.024
Agrawal, N., Verma, P., and Shahi, S. K. (2018). Degradation of polycyclic aromatic hydrocarbons (phenanthrene and pyrene) by the ligninolytic fungi Ganoderma lucidum isolated from the hardwood stump. Bioresour. Bioprocess. 5, 11. doi:10.1186/s40643-018-0197-5
Ajona, M., and Vasanthi, P. (2020). Bioremediation of petroleum contaminated soils – a review. Mater. Today Proc. 45, 7117–7122. doi:10.1016/j.matpr.2021.01.949
Akhtar, N., and Mannan, M. A. (2020). Mycoremediation: Expunging environmental pollutants. Bio. Rep. 26, 00452. doi:10.1016/j.btre.2020.e00452
Al-Hawash, A. B. (2018). Fungal degradation of polycyclic aromatic hydrocarbons. Int. J. Pure Appl. Biosci. 6, 8–24. doi:10.18782/2320-7051.6302
Al-Hawash, A. B., Dragh, M. A., Li, S., Alhujaily, A., Abbood, H. A., Zhang, X., et al. (2018a). Principles of microbial degradation of petroleum hydrocarbons in the environment. Egypt. J. Aquat. Res. 44, 71–76. doi:10.1016/j.ejar.2018.06.001
Al-Hawash, A. B., Zhang, J., Li, S., Liu, J., Ghalib, H. B., Zhang, X., et al. (2018b). Biodegradation of n-hexadecane by Aspergillus sp. RFC-1 and its mechanism. Ecotoxicol. Environ. Saf. 164, 398–408. doi:10.1016/j.ecoenv.2018.08.049
Al-Kindi, S., and Abed, R. M. M. (2016). Comparing oil degradation efficiency and bacterial communities in contaminated soils subjected to biostimulation using different organic wastes. Water. Air. Soil Pollut. 227 227, 36. doi:10.1007/s11270-015-2722-x
Anderson, C., and Juday, G. (2016). Mycoremediation of petroleum: a literature review. J. Environ. Sci. Eng. A 5, 397–405. doi:10.17265/2162-5298/2016.08.002
Ani, E., Adekunle, A. A., Kadiri, A. B., and Njoku, K. L. (2021). Rhizoremediation of hydrocarbon contaminated soil using Luffa aegyptiaca (Mill) and associated fungi. Int. J. Phytoremediation 23, 1444–1456. doi:10.1080/15226514.2021.1901852
Aragaw, T. A. (2021). Functions of various bacteria for specific pollutants degradation and their application in wastewater treatment: a review. Int. J. Environ. Sci. Technol. 18, 2063–2076. doi:10.1007/s13762-020-03022-2
Aragaw, T. A., Bogale, F. M., and Gessesse, A. (2022). Adaptive response of thermophiles to redox stress and their role in the process of dye degradation from textile industry wastewater. Front. Physiol. 13, 908370. doi:10.3389/fphys.2022.908370
Asemoloye, M. D., Ahmad, R., and Jonathan, S. G. (2017). Synergistic action of rhizospheric fungi with Megathyrsus maximus root speeds up hydrocarbon degradation kinetics in oil polluted soil. Chemosphere 187, 1–10. doi:10.1016/j.chemosphere.2017.07.158
Bala, S., Garg, D., Thirumalesh, B. V., Sharma, M., Sridhar, K., Inbaraj, B. S., et al. (2022). Recent strategies for bioremediation of emerging pollutants: a review for a green and sustainable environment. Toxics 10, 484. doi:10.3390/toxics10080484
Boopathy, R. (2000). Factors limiting bioremediation technologies. Bioresour. Technol. 74, 63–67. doi:10.1016/S0960-8524(99)00144-3
Callaghan, A. V., Gieg, L. M., Kropp, K. G., Suflita, J. M., and Young, L. Y. (2006). Comparison of mechanisms of alkane metabolism under sulfate-reducing conditions among two bacterial isolates and a bacterial consortium. Appl. Environ. Microbiol. 72, 4274–4282. doi:10.1128/AEM.02896-05
Chandra, S., Sharma, R., Singh, K., and Sharma, A. (2013). Application of bioremediation technology in the environment contaminated with petroleum hydrocarbon. Ann. Microbiol. 63, 417–431. doi:10.1007/s13213-012-0543-3
Chaudhary, D. K., Bajagain, R., Woo, S., and Jaisoo, J. (2021). Effect of consortium bioaugmentation and biostimulation on remediation efficiency and bacterial diversity of diesel-contaminated aged soil. World J. Microbiol. Biotechnol. 37, 1–12. doi:10.1007/s11274-021-02999-3
Chaudhary, D. K., and Kim, J. (2019). New insights into bioremediation strategies for oil-contaminated soil in cold environments. Int. Biodeterior. Biodegr. 142, 58–72. doi:10.1016/j.ibiod.2019.05.001
Chettri, B., Singha, N. A., and Singh, A. K. (2021). Efficiency and kinetics of Assam crude oil degradation by Pseudomonas aeruginosa and Bacillus sp. Arch. Microbiol. 203, 5793–5803. doi:10.1007/s00203-021-02567-1
Chukwuma, O. B., Rafatullah, M., Tajarudin, H. A., and Ismail, N. (2020). Lignocellulolytic enzymes in biotechnological and industrial processes: a review. Sustain. 12, 7282. doi:10.3390/su12187282
Cravo-Laureau, C., Grossi, V., Raphel, D., Matheron, R., and Hirschler-Réa, A. (2005). Anaerobic n-alkane metabolism by a sulfate-reducing bacterium, Desulfatibacillum aliphaticivorans strain CV2803T. Appl. Environ. Microbiol. 71, 3458–3467. doi:10.1128/aem.71.7.3458-3467.2005
Cui, J. Q., He, Q. S., Liu, M. H., Chen, H., Sun, M. B., and Wen, J. P. (2020). Comparative study on different remediation strategies applied in petroleum-contaminated soils. Int. J. Environ. Res. Public Health 17, 1606. doi:10.3390/ijerph17051606
Daâssi, D., and Almaghribi, F. Q. (2021). Soil microcosms for bioaugmentation with fungal isolates to remediate petroleum hydrocarbon-contaminated soil. Preprint, 1–38. doi:10.21203/rs.3.rs-1086969/v1
Dao, A. T. N., Smits, M., Dang, H. T. C., Brouwer, A., and de Boer, T. E. (2021). Elucidating fungal Rigidoporus species FMD21 lignin-modifying enzyme genes and 2,3,7,8-tetrachlorodibenzo-p-dioxin degradation by laccase isozymes. Enzyme Microb. Technol. 147, 109800. doi:10.1016/j.enzmictec.2021.109800
Das, N., and Chandran, P. (2011). Microbial degradation of petroleum hydrocarbon contaminants: an overview. Microb. Degrad. Petroleum Hydrocarbon Contam. Overv. Biotechnol. Res. Int. 2011, 1–13. doi:10.4061/2011/941810
Deshmukh, R., Khardenavis, A. A., and Purohit, H. J. (2016). Diverse metabolic capacities of fungi for bioremediation. Indian J. Microbiol. 56, 247–264. doi:10.1007/s12088-016-0584-6
Dhailappan, A., Arumugam, A., Rajendran, G., and Ravichandran, M. (2022). A dualistic approach to investigate the remedial potential and edible property of Pleurotus ostreatus on hydrocarbon-contaminated soil. J. Appl. Biol. Biotechnol. 10, 70–78. doi:10.7324/jabb.2022.100310
Dias, R. L., Ruberto, L., Hernández, E., Vázquez, S. C., Lo Balbo, A., Del Panno, M. T., et al. (2012). Bioremediation of an aged diesel oil-contaminated Antarctic soil: evaluation of the “on site” biostimulation strategy using different nutrient sources. Int. Biodeterior. Biodegr. 75, 96–103. doi:10.1016/j.ibiod.2012.07.020
Dickson, U. J., Coffey, M., Mortimer, R. J. G., Di Bonito, M., and Ray, N. (2019). Mycoremediation of petroleum contaminated soils: progress, prospects and perspectives. Environ. Sci. Process. Impacts 21, 1446–1458. doi:10.1039/c9em00101h
Dindar, E., Olcay, F., Sagban, T., and Baskaya, H. S. (2013). Bioremediation of petroleum-contaminated soil petrol ile kirlenmiş toprakların biyoremediasyonu. J. Biol. Environ. Sci. 7, 39–47. doi:10.3390/ijms19113373
El-Sheshtawy, H. S., Aman, D., and Nassar, H. N. (2022). A novel bioremediation technique for petroleum hydrocarbons by bacterial consortium immobilized on goethite-chitosan nanocomposite. Soil Sediment. Contam. 31, 176–199. doi:10.1080/15320383.2021.1916737
Elufisan, T. O., Rodríguez-Luna, I. C., Oyedara, O. O., Sánchez-Varela, A., Hernández-Mendoza, A., Gonzalez, E. D., et al. (2020). The Polycyclic Aromatic Hydrocarbon (PAH) degradation activities and genome analysis of a novel strain Stenotrophomonas sp. Pemsol isolated from Mexico. PeerJ 8, e8102. doi:10.7717/peerj.8102
Essabri, A. M. A., Aydinlik, N. P., and Williams, N. E. (2019). Bioaugmentation and biostimulation of total petroleum hydrocarbon degradation in a petroleum-contaminated soil with fungi isolated from olive oil effluent. Water. Air. Soil Pollut. 230 230, 76. doi:10.1007/s11270-019-4127-8
Falade, A. O., Nwodo, U. U., Iweriebor, B. C., Green, E., Mabinya, L. V., and Okoh, A. I. (2017). Lignin peroxidase functionalities and prospective applications. Microbiologyopen 6, 003944–e414. doi:10.1002/mbo3.394
Fordwour, O. B., Luka, G., Hoorfar, M., and Wolthers, K. R. (2018). Kinetic characterization of acetone monooxygenase from Gordonia sp. strain TY-5. Amb. Express 8, 181. doi:10.1186/s13568-018-0709-x
Fu, W., Xu, M., Sun, K., Hu, L., Cao, W., Dai, C., et al. (2018). Biodegradation of phenanthrene by endophytic fungus Phomopsis liquidambari in vitro and in vivo. Chemosphere 203, 160–169. doi:10.1016/j.chemosphere.2018.03.164
Fuentes, S., Méndez, V., Aguila, P., and Seeger, M. (2014). Bioremediation of petroleum hydrocarbons: catabolic genes, microbial communities, and applications. Appl. Microbiol. Biotechnol. 98, 4781–4794. doi:10.1007/s00253-014-5684-9
Gaur, V. K., Gupta, S., and Pandey, A. (2021). CURRENT TRENDS AND RESEARCH IN INDUSTRIAL WASTEWATER TREATMENT THROUGH Evolution in mitigation approaches for petroleum oil-polluted environment: recent advances and future directions.
Guarino, C., Spada, V., and Sciarrillo, R. (2017). Assessment of three approaches of bioremediation (Natural Attenuation, Landfarming and Bioagumentation – assistited Landfarming) for a petroleum hydrocarbons contaminated soil. Chemosphere 170, 10–16. doi:10.1016/j.chemosphere.2016.11.165
Haghollahi, A., Hassan, M., and Schaf, M. (2016). The effect of soil type on the bioremediation of petroleum contaminated soils. ournal Environ. Manag. 180, 197–201. doi:10.1016/j.jenvman.2016.05.038
Hawksworth, D. L., and Lücking, R. (2017). Fungal diversity revisited: 2.2 to 3.8 million species. Fungal Kingd. 5, 79–95. doi:10.1128/9781555819583.ch4
He, H., Chen, Y., Li, X., Cheng, Y., Yang, C., and Zeng, G. (2017). Influence of salinity on microorganisms in activated sludge processes: a review. Int. Biodeterior. Biodegr. 119, 520–527. doi:10.1016/j.ibiod.2016.10.007
Heyen, S., Scholz-Böttcher, B. M., Rabus, R., and Wilkes, H. (2021). Release of carboxylic acids into the exometabolome during anaerobic growth of a denitrifying bacterium with single substrates or crude oil. Org. Geochem. 154, 104179. doi:10.1016/j.orggeochem.2020.104179
Horel, A., and Schiewer, S. (2020). Microbial degradation of different hydrocarbon fuels with mycoremediation of volatiles. Microorg. 8 8, 163. doi:10.3390/microorganisms8020163
Huang, Y., Pan, H., Wang, Q., Ge, Y., Liu, W., and Christie, P. (2019). Enrichment of the soil microbial community in the bioremediation of a petroleum-contaminated soil amended with rice straw or sawdust. Chemosphere 224, 265–271. doi:10.1016/j.chemosphere.2019.02.148
Ite, A. E., and Ibok, U. J. (2019). Role of plants and microbes in bioremediation of petroleum hydrocarbons contaminated soils. Int. J. Environ. Bioremediation Biodegr. Vol. 7, 2019, Pages 1-19 7, 1–19. doi:10.12691/ijebb-7-1-1
Ji, Y., Mao, G., Wang, Y., and Bartlam, M. (2013). Structural insights into diversity and n-alkane biodegradation mechanisms of alkane hydroxylases. Front. Microbiol. 4 4, 58. doi:10.3389/fmicb.2013.00058
Kebede, G., Tafese, T., Abda, E. M., Kamaraj, M., and Assefa, F. (2021). Factors influencing the bacterial bioremediation of hydrocarbon contaminants in the soil: mechanisms and impacts. J. Chem. 2021, 1–17. doi:10.1155/2021/9823362
Kong, L., Gao, Y., Zhou, Q., Zhao, X., and Sun, Z. (2018). Biochar accelerates PAHs biodegradation in petroleum-polluted soil by biostimulation strategy. J. Hazard. Mater. 343, 276–284. doi:10.1016/j.jhazmat.2017.09.040
Koshlaf, E., and Ball, A. S. (2017). Soil bioremediation approaches for petroleum hydrocarbon polluted environments. AIMS Microbiol. 3, 25–49. doi:10.3934/microbiol.2017.1.25
Koshlaf, E., Shahsavari, E., Haleyur, N., Mark Osborn, A., and Ball, A. S. (2019). Effect of biostimulation on the distribution and composition of the microbial community of a polycyclic aromatic hydrocarbon-contaminated landfill soil during bioremediation. Geoderma 338, 216–225. doi:10.1016/j.geoderma.2018.12.001
Kristanti, R. A., Hadibarata, T., Toyama, T., Tanaka, Y., and Mori, K. (2011). Bioremediation of crude oil by white rot fungi Polyporus sp. S133. J. Microbiol. Biotechnol. 21, 995–1000. doi:10.4014/jmb.1105.05047
Kumar, A., and Chandra, R. (2020). Ligninolytic enzymes and its mechanisms for degradation of lignocellulosic waste in environment. Heliyon 6 6, e03170. doi:10.1016/j.heliyon.2020.e03170
Kumar, V., Kumar, M., and Prasad, R. (2019). “Microbial action on hydrocarbons,” in. Microb. Action Hydrocarbons, 1–667. doi:10.1007/978-981-13-1840-5
Kvenvolden, K. A., and Cooper, C. K. (2003). Natural seepage of crude oil into the marine environment. Geo-Marine Lett. 23, 140–146. doi:10.1007/s00367-003-0135-0
Li, X., He, W., Du, M., Zheng, J., Du, X., and Li, Y. (2021). Design of a microbial remediation inoculation program for petroleum hydrocarbon contaminated sites based on degradation pathways. Int. J. Environ. Res. Public Heal. 18, 8794. doi:10.3390/ijerph18168794
Liang, Y., Zhu, H., Bañuelos, G., Yan, B., Zhou, Q., Yu, X., et al. (2017). Constructed wetlands for saline wastewater treatment: a review. Ecol. Eng. 98, 275–285. doi:10.1016/j.ecoleng.2016.11.005
Macaulay, B., and Rees, D. (2014). Bioremediation of oil spills: a review of challenges for research advancement. Ann. Environ. Sci. 8, 9–37.
Maeng, J. H. O., Sakai, Y., Tani, Y., and Kato, N. (1996). Isolation and characterization of a novel oxygenase that catalyzes the first step of n-alkane oxidation in Acinetobacter sp. strain M-1. J. Bacteriol. 178, 3695–3700. doi:10.1128/jb.178.13.3695-3700.1996
Marco-Urrea, E., García-Romera, I., and Aranda, E. (2015). Potential of non-ligninolytic fungi in bioremediation of chlorinated and polycyclic aromatic hydrocarbons. N. Biotechnol. 32, 620–628. doi:10.1016/j.nbt.2015.01.005
Marín, F., Navarrete, H., and Narvaez-Trujillo, A. (2018). Total petroleum hydrocarbon degradation by endophytic fungi from the Ecuadorian Amazon. Adv. Microbiol. 08, 1029–1053. doi:10.4236/aim.2018.812070
Martínez Álvarez, L. M., Ruberto, L. A. M., Lo Balbo, A., and Mac Cormack, W. P. (2017). Bioremediation of hydrocarbon-contaminated soils in cold regions: development of a pre-optimized biostimulation biopile-scale field assay in Antarctica. Sci. Total Environ. 590–591, 194–203. doi:10.1016/j.scitotenv.2017.02.204
Mbachu, A. E., Chukwura, E. I., and Mbachu, N. A. (2020). Role of microorganisms in the degradation of organic pollutants: a review. Energy Environ. Eng. 7, 1–11. doi:10.13189/eee.2020.070101
Megharaj, M., Ramakrishnan, B., Venkateswarlu, K., Sethunathan, N., and Naidu, R. (2011). Bioremediation approaches for organic pollutants: a critical perspective. Environ. Int. 37, 1362–1375. doi:10.1016/j.envint.2011.06.003
Mohammadi-Sichani, M., Mazaheri Assadi, M., Farazmand, A., Kianirad, M., Ahadi, A. M., and Hadian-Ghahderijani, H. (2019). Ability of Agaricus bisporus, Pleurotus ostreatus and Ganoderma lucidum compost in biodegradation of petroleum hydrocarbon-contaminated soil. Int. J. Environ. Sci. Technol. 16, 2313–2320. doi:10.1007/s13762-017-1636-0
Morillo, E., Madrid, F., Lara-Moreno, A., and Villaverde, J. (2020). Soil bioremediation by cyclodextrins. A review. Int. J. Pharm. 591, 119943. doi:10.1016/j.ijpharm.2020.119943
Mrozik, A., and Piotrowska-Seget, Z. (2010). Bioaugmentation as a strategy for cleaning up of soils contaminated with aromatic compounds. Microbiol. Res. 165, 363–375. doi:10.1016/j.micres.2009.08.001
Muangchinda, C., Rungsihiranrut, A., Prombutara, P., Soonglerdsongpha, S., and Pinyakong, O. (2018). 16S metagenomic analysis reveals adaptability of a mixed-PAH-degrading consortium isolated from crude oil-contaminated seawater to changing environmental conditions. J. Hazard. Mater. 357, 119–127. doi:10.1016/j.jhazmat.2018.05.062
Musat, F. (2015). The anaerobic degradation of gaseous, nonmethane alkanes - from in situ processes to microorganisms. Comput. Struct. Biotechnol. J. 13, 222–228. doi:10.1016/j.csbj.2015.03.002
Nassar, S. E., and Said, R. M. (2021). Bioremediation assessment, hematological, and biochemical responses of the earthworm (Allolobophora caliginosa) in soil contaminated with crude oil. Environ. Sci. Pollut. Res. 28, 54565–54574. doi:10.1007/s11356-021-13889-4
Novianty, R., Saryono, A., Pratiwi, N. W., Hidayah, A., and Juliantari, E. (2021). The diversity of fungi consortium isolated from polluted soil for degrading petroleum hydrocarbon. Biodiversitas 22, 5077–5084. doi:10.13057/BIODIV/D221145
Omokhagbor Adams, G., Tawari Fufeyin, P., Eruke Okoro, S., and Ehinomen, I. (2020). Bioremediation, biostimulation and bioaugmention: a review. Int. J. Environ. Bioremediation Biodegr. 3, 28–39. doi:10.12691/ijebb-3-1-5
Oualha, M., Al-Kaabi, N., Al-Ghouti, M., and Zouari, N. (2019). Identification and overcome of limitations of weathered oil hydrocarbons bioremediation by an adapted Bacillus sorensis strain. J. Environ. Manage. 250, 109455. doi:10.1016/j.jenvman.2019.109455
Oyetibo, G. O., Chien, M. F., Ikeda-Ohtsubo, W., Suzuki, H., Obayori, O. S., Adebusoye, S. A., et al. (2017). Biodegradation of crude oil and phenanthrene by heavy metal resistant Bacillus subtilis isolated from a multi-polluted industrial wastewater creek. Int. Biodeterior. Biodegr. 120, 143–151. doi:10.1016/j.ibiod.2017.02.021
Peng, R. H., Xiong, A. S., Xue, Y., Fu, X. Y., Gao, F., Zhao, W., et al. (2008). Microbial biodegradation of polyaromatic hydrocarbons. FEMS Microbiol. Rev. 32, 927–955. doi:10.1111/j.1574-6976.2008.00127.x
Perelo, L. W. (2010). Review: in situ and bioremediation of organic pollutants in aquatic sediments. J. Hazard. Mater.177, 81–89. doi:10.1016/j.jhazmat.2009.12.090
Perera, M., Wijesundera, S., Wijayarathna, C. D., Seneviratne, G., and Jayasena, S. (2022). Identification of long-chain alkane-degrading (LadA) monooxygenases in Aspergillus flavus via in silico analysis. Front. Microbiol. 13, 898456. doi:10.3389/fmicb.2022.898456
Perry, J. J., and Gibson, D. T. (1977). Microbial metabolism of cyclic hydrocarbons and related compounds. Crit. Rev. Microbiol. 5, 387–412. doi:10.3109/10408417709102811
Popoola, L. T., Yusuff, A. S., Adeyi, A. A., and Omotara, O. O. (2022). Bioaugmentation and biostimulation of crude oil contaminated soil: process parameters influence. South Afr. J. Chem. Eng. 39, 12–18. doi:10.1016/j.sajce.2021.10.003
Qi, Y. B., Wang, C. Y., Lv, C. Y., Lun, Z. M., and Zheng, C. G. (2017). Removal capacities of polycyclic aromatic hydrocarbons (PAHs) by a newly isolated strain from oilfield produced water. Int. J. Environ. Res. Public Health 14, 215. doi:10.3390/ijerph14020215
Qin, G., Gong, D., and Fan, M. Y. (2013). Bioremediation of petroleum-contaminated soil by biostimulation amended with biochar. Int. Biodeterior. Biodegr. 85, 150–155. doi:10.1016/j.ibiod.2013.07.004
Qin, X., Tang, J. C., Li, D. S., and Zhang, Q. M. (2012). Effect of salinity on the bioremediation of petroleum hydrocarbons in a saline-alkaline soil. Lett. Appl. Microbiol. 55, 210–217. doi:10.1111/j.1472-765X.2012.03280.x
Ramadass, K., Megharaj, M., Venkateswarlu, K., and Naidu, R. (2018). Bioavailability of weathered hydrocarbons in engine oil-contaminated soil: impact of bioaugmentation mediated by Pseudomonas spp. on bioremediation. Sci. Total Environ. 636, 968–974. doi:10.1016/j.scitotenv.2018.04.379
Rath, K. M., Maheshwari, A., Bengtson, P., and Rousk, J. (2016). Comparative toxicities of salts on microbial processes in soil. Appl. Environ. Microbiol. 82, 2012–2020. doi:10.1128/AEM.04052-15
Rathankumar, A. K., Saikia, K., Kumar, P. S., Varjani, S., Kalita, S., Bharadwaj, N., et al. (2022). Surfactant-aided mycoremediation of soil contaminated with polycyclic aromatic hydrocarbon (PAHs): progress, limitation, and countermeasures. J. Chem. Technol. Biotechnol. 97, 391–408. doi:10.1002/jctb.6721
Rathankumar, A. K., Saikia, K., Ramachandran, K., Batista, R. A., Cabana, H., and Vaidyanathan, V. K. (2020). Effect of soil organic matter (SOM) on the degradation of polycyclic aromatic hydrocarbons using Pleurotus dryinus IBB 903-A microcosm study. J. Environ. Manage. 260, 110153. doi:10.1016/j.jenvman.2020.110153
Reyes-César, A., Absalón, E., Fernández, F. J., González, J. M., and Cortés-Espinosa, D. V. (2014). Biodegradation of a mixture of PAHs by non-ligninolytic fungal strains isolated from crude oil-contaminated soil. World J. Microbiol. Biotechnol. 30, 999–1009. doi:10.1007/s11274-013-1518-7
Rhodes, C. J. (2014). Mycoremediation (bioremediation with fungi) - growing mushrooms to clean the earth. Chem. Speciat. Bioavailab. 26, 196–198. doi:10.3184/095422914X14047407349335
Roy, A., Dutta, A., Pal, S., Gupta, A., Sarkar, J., Chatterjee, A., et al. (2018). Biostimulation and bioaugmentation of native microbial community accelerated bioremediation of oil refinery sludge. Bioresour. Technol. 253, 22–32. doi:10.1016/j.biortech.2018.01.004
Sayara, T., and Sánchez, A. (2020). Bioremediation of PAH-contaminated soils: process enhancement through composting/compost. Appl. Sci. 10, 3684. doi:10.3390/app10113684
Scherr, K., Aichberger, H., Braun, R., and Loibner, A. P. (2007). Influence of soil fractions on microbial degradation behavior of mineral hydrocarbons. Eur. J. Soil Biol. 43, 341–350. doi:10.1016/j.ejsobi.2007.03.009
Shanmugaraju, V., Arun, P., Rengaramanujam, J., and Senthilprabhu, S. (2013). Isolation of bacteria from seacoasts and screening for its antibacterial substance production. Int. J. Curr. Microbiol. App. Sci. 2, 347–358.
Shi, L., Liu, Z., Yang, L., and Fan, W. (2022). Effect of plant waste addition as exogenous nutrients on microbial remediation of petroleum - contaminated soil. Ann. Microbiol. 72, 22. doi:10.1186/s13213-022-01679-3
Singer, M. E., and Finnerty, W. R. (1985). Alcohol dehydrogenases in Acinetobacter sp. strain HO1-N: role in hexadecane and hexadecanol metabolism. J. Bacteriol. 164, 1017–1024. doi:10.1128/jb.164.3.1017-1024.1985
Singha, L. P., and Pandey, P. (2021). Rhizosphere assisted bioengineering approaches for the mitigation of petroleum hydrocarbons contamination in soil. Crit. Rev. Biotechnol. 41, 749–766. doi:10.1080/07388551.2021.1888066
Si-Zhong, Y., Hui-Jun, J., Zhi, W., Rui-Xia, H. E., Yan-Jun, J. I., Xiu-Mei, L. I., et al. (2009). Bioremediation of oil spills in cold environments: a review. Pedosphere 19, 371–381. doi:10.1016/S1002-0160(09)60128-4
So, C. M., and Young, L. Y. (1999). Initial reactions in anaerobic alkane degradation by a sulfate reducer, strain AK-01. Appl. Environ. Microbiol. 65, 5532–5540. doi:10.1128/aem.65.12.5532-5540.1999
Stepanova, A. Y., Gladkov, E. A., Osipova, E. S., Gladkova, O. V., and Tereshonok, D. V. (2022). Bioremediation of soil from petroleum contamination. Processes 10, 1224. doi:10.3390/pr10061224
Tang, J., Wang, M., Wang, F., Sun, Q., and Zhou, Q. (2011). Eco-toxicity of petroleum hydrocarbon contaminated soil. J. Environ. Sci. 23, 845–851. doi:10.1016/S1001-0742(10)60517-7
Toda, H., and Itoh, N. (2012). Isolation and characterization of styrene metabolism genes from styrene-assimilating soil bacteria Rhodococcus sp. ST-5 and ST-10. J. Biosci. Bioeng. 113, 12–19. doi:10.1016/j.jbiosc.2011.08.028
Torlapati, J., and Boufadel, M. C. (2014). Evaluation of the biodegradation of Alaska North Slope oil in microcosms using the biodegradation model BIOB. Front. Microbiol. 5 5, 212. doi:10.3389/fmicb.2014.00212
Towell, M. G., Paton, G. I., and Semple, K. T. (2011). The biodegradation of cable oil components: impact of oil concentration, nutrient addition and bioaugmentation. Environ. Pollut. 159, 3777–3783. doi:10.1016/j.envpol.2011.06.043
Truskewycz, A., Gundry, T. D., Khudur, L. S., Kolobaric, A., Taha, M., Aburto-Medina, A., et al. (2019). Petroleum hydrocarbon contamination in terrestrial ecosystems—fate and microbial responses. Molecules 24, 3400–3420. doi:10.3390/molecules24183400
Ubani, O., Atagana, H. I., Thantsha, M. S., and Adeleke, R. (2016). Characterisation of oil degrading bacteria from tailored compost containing crude oil sludge. Indian J. Biotechnol. 15, 243–250.
Varjani, S. J. (2017). Microbial degradation of petroleum hydrocarbons. Bioresour. Technol. 223, 277–286. doi:10.1016/j.biortech.2016.10.037
Varjani, S. J., and Upasani, V. N. (2016). Biodegradation of petroleum hydrocarbons by oleophilic strain of Pseudomonas aeruginosa NCIM 5514. Bioresour. Technol. 222, 195–201. doi:10.1016/j.biortech.2016.10.006
Varjani, S. J., and Upasani, V. N. (2019). Influence of abiotic factors, natural attenuation, bioaugmentation and nutrient supplementation on bioremediation of petroleum crude contaminated agricultural soil. J. Environ. Manage. 245, 358–366. doi:10.1016/j.jenvman.2019.05.070
Victor, I. A., For, C., Medical, A., Archibong, I. A., Okon, E., and Andem, A. B. (2020). The biochemical mechanisms of petroleum degradation by bacteria.
Vieira, G. A. L., Magrini, M. J., Bonugli-Santos, R. C., Rodrigues, M. V. N., and Sette, L. D. (2018). Polycyclic aromatic hydrocarbons degradation by marine-derived basidiomycetes: optimization of the degradation process. Braz. J. Microbiol. 49, 749–756. doi:10.1016/j.bjm.2018.04.007
Waigi, M. G., Kang, F., Goikavi, C., Ling, W., and Gao, Y. (2015). Phenanthrene biodegradation by sphingomonads and its application in the contaminated soils and sediments: a review. Int. Biodeterior. Biodegr. 104, 333–349. doi:10.1016/j.ibiod.2015.06.008
Walworth, J., Pond, A., Snape, I., Rayner, J., Ferguson, S., and Harvey, P. (2007). Nitrogen requirements for maximizing petroleum bioremediation in a sub-Antarctic soil. Cold Reg. Sci. Technol. 48, 84–91. doi:10.1016/j.coldregions.2006.07.001
Wang, F., Li, C., Wang, H., Chen, W., and Huang, Q. (2016). Characterization of a phenanthrene-degrading microbial consortium enriched from petrochemical contaminated environment. Int. Biodeterior. Biodegr. 115, 286–292. doi:10.1016/j.ibiod.2016.08.028
Wang, L., Cheng, Y., Naidu, R., and Bowman, M. (2021). The key factors for the fate and transport of petroleum hydrocarbons in soil with related in/ex situ measurement methods: an overview. Front. Environ. Sci. 9, 1–15. doi:10.3389/fenvs.2021.756404
Wang, X., Yao, B., and Su, X. (2018). Linking enzymatic oxidative degradation of lignin to organics detoxification. Int. J. Mol. Sci. 19, 3373. doi:10.3390/ijms19113373
Weng, C., Peng, X., and Han, Y. (2021). Depolymerization and conversion of lignin to value-added bioproducts by microbial and enzymatic catalysis. Biotechnol. Biofuels 14, 84–22. doi:10.1186/s13068-021-01934-w
Wentzel, A., Ellingsen, T. E., Kotlar, H. K., Zotchev, S. B., and Throne-Holst, M. (2007). Bacterial metabolism of long-chain n-alkanes. Appl. Microbiol. Biotechnol. 76, 1209–1221. doi:10.1007/s00253-007-1119-1
Winkler, J. R., and Gray, H. B. (2015). Electron flow through biological molecules: does hole hopping protect proteins from oxidative damage? Q. Rev. Biophys. 48, 411–420. doi:10.1017/S0033583515000062
Xu, X., Liu, W., Tian, S., Wang, W., Qi, Q., Jiang, P., et al. (2018). Petroleum hydrocarbon-degrading bacteria for the remediation of oil pollution under aerobic conditions: a perspective analysis. Front. Microbiol. 9, 2885. doi:10.3389/fmicb.2018.02885
Yap, H. S., Zakaria, N. N., Zulkharnain, A., Sabri, S., Gomez-Fuentes, C., and Ahmad, S. A. (2021). Bibliometric analysis of hydrocarbon bioremediation in cold regions and a review on enhanced soil bioremediation. Biol. (Basel). 10, 354. doi:10.3390/biology10050354
Yin, C. F., Xu, Y., Li, T., and Zhou, N. Y. (2022). Wide distribution of the sad gene cluster for sub-terminal oxidation in alkane utilizers. Environ. Microbiol. 24, 6307–6319. doi:10.1111/1462-2920.16124
Keywords: oil spill, PHCs, contaminated sites, bioremediation, microbes, degradation mechanisms
Citation: Mekonnen BA, Aragaw TA and Genet MB (2024) Bioremediation of petroleum hydrocarbon contaminated soil: a review on principles, degradation mechanisms, and advancements. Front. Environ. Sci. 12:1354422. doi: 10.3389/fenvs.2024.1354422
Received: 12 December 2023; Accepted: 08 February 2024;
Published: 22 February 2024.
Edited by:
Meng Jiang, Zhejiang University, ChinaCopyright © 2024 Mekonnen, Aragaw and Genet. This is an open-access article distributed under the terms of the Creative Commons Attribution License (CC BY). The use, distribution or reproduction in other forums is permitted, provided the original author(s) and the copyright owner(s) are credited and that the original publication in this journal is cited, in accordance with accepted academic practice. No use, distribution or reproduction is permitted which does not comply with these terms.
*Correspondence: Tadele Assefa Aragaw, dGFhYWFkODJAZ21haWwuY29t; Bassazin Ayalew Mekonnen, YmFzc2EuY2hlZEBnbWFpbC5jb20=