- 1Revive & Restore, Sausalito, CA, United States
- 2Department of Ecology and Evolutionary Biology, University of California, Santa Cruz, Santa Cruz, CA, United States
- 3VCA San Francisco Veterinary Specialists, San Francisco, CA, United States
For tens of thousands of years, passenger pigeons (Ectopistes migratorius) were a dominant member of eastern North American forest communities, with megaflocks comprising up to several billion individuals. The extinction of passenger pigeons in the early twentieth century undoubtedly influenced associated species and ecosystems as interactions stemming from the pigeons disappeared suddenly. Here, we strive to better understand what was probably one of the most significant of these interactions—that between passenger pigeons and seed bearing trees. Using the band-tailed pigeon (Patagioenas fasciata) and the rock dove (Columba livia) as physical and ecological proxies, we evaluated passenger pigeon dietary range and potential to disperse seeds. Our findings suggest that the passenger pigeon's dietary range, observed historically to be taxonomically broad, was constrained to certain seed sizes due to bill gape size. In addition, we conclude that the digestive process invariably destroyed consumed seeds but the potential for a nutrition/dispersal mutualism might still have existed via regurgitation and post-mortem release of crop contents. Our results highlight the range of ecological interactions that can be lost with species' extinction and the inherent challenge of understanding the consequences of those interactions.
Introduction
For tens of thousands of years prior to their extinction in the early twentieth century, passenger pigeons (Ectopistes migratorius) were the most abundant bird species in North America, moving nomadically, consuming mast, and fruit crops throughout eastern forests in megaflocks containing tens of millions to billions of individuals (Greenberg, 2014; Murray et al., 2017). Various hypotheses have been advanced for how these megaflocks shaped the distribution and abundance of tree species (Webb, 1986; Ellsworth and McComb, 2003); however, most of these hypotheses lack convincing evidence and long-term eco-evolutionary forces imposed by passenger pigeons lack attention almost entirely.
The ways in which passenger pigeons shaped whole ecosystems and/or individual species within forest communities depend on the nature of the bird's relationships with those species. Arguably the most significant interactions were between the pigeons and their primary prey—masting and seed bearing trees (Webb, 1986; Ellsworth and McComb, 2003). Historic observations and analyses of crop/gut contents indicate that passenger pigeon's dietary range included most if not all species of seed-bearing trees (Cottam and Knappen, 1939; Schorger, 1955; Greenberg, 2014). However, the degree to which passenger pigeons functioned as seed predators (consumers that destroy seeds) vs. seed dispersers (mutualists that disperse seeds) is unknown. These differing ecological roles have distinct evolutionary implications for the forest ecosystem both before and after the passenger pigeon's extinction.
The negative impacts of consumers on their prey (e.g., reduced fitness) can lead to an evolutionary arms race of defense and resistance, whereas positive effects (e.g., dispersal) may select for mutualisms (Ehrlich and Raven, 1964; Howe and Smallwood, 1982; Hererra, 1985; Thompson, 1994) In the case of mast bearing trees, responses to negative impacts (the destruction of seeds) include predator satiation, asynchronous masting, alteration of germination timing, changes in seed size, and chemical defenses (Janzen, 1971; Loehle, 1987; Vander Wall, 2001). The adaptive response by trees to seed predation depends on the number and size of seeds eaten. Due to their great abundance, passenger pigeons are commonly assumed to have consumed seed crops in their entirety, although this assumption neglects the morphological constraint of bill gape size. For avian consumers like passenger pigeons that must swallow their food whole, maximum gape size constrains dietary range (Wheelwright, 1985). While the gape size of passenger pigeons likely posed no limitations from small seed bearing plants, it may have influenced the species' impacts on large seed-bearing plants such as northern red oak, Quercus rubra, and American chestnut, Castanea dentata; although both species are noted in the historical record of passenger pigeon diet, acorns were commonly eaten whereas accounts of chestnut consumption are exceptionally rare (Cottam and Knappen, 1939; Schorger, 1955).
Fecal transport and caching are common modes of dispersal by seed predators (e.g., rodents and jays), each providing the quantity and quality of dispersal typical to many plant/consumer mutualisms (Schupp, 1993; Vander Wall, 2001). Pigeons do not cache seeds for later consumption. Studies of digestion in frugivorous or granivorous pigeons reveal that some species disperse seeds via fecal transport while others destroy them (Blockstein et al., 1987; Lambert, 1989; McConkey et al., 2004). For generalist wood pigeons that consume both fruits and seeds (i.e., European wood pigeon, Columba palumbus, band-tailed pigeon, Patagioenas fasciata, and the passenger pigeon), digestive impacts have not been determined (Webb, 1986). Post-mortem dispersal of undigested crop contents is another potential means of seed dispersal by pigeons (Bucher and Bocco, 2009). Given the abundance of passenger pigeons, which persisted for tens of thousands of years (Murray et al., 2017), post-mortem dispersal could have played a role in plant range expansion or redistribution following altered environmental conditions (Webb, 1986), possibly leading to mutualistic coevolution.
We conducted the following measurements and experiments in an effort to better understand the passenger pigeon's dietary ecology: first, we modeled the potential gape sizes of the passenger pigeon and screened mast crops samples to identify possible seed size refuges; second, we used feeding trials to test for the passage of viable seeds through the band-tailed pigeon digestive tract; and third, we conducted a field study of post-mortem seed dispersal via crop contents using acorns and rock pigeon, Columba livia, carcasses to explore the possibility of a dispersal mutualism between passenger pigeons and seed bearing plants.
Methods
Morphometric and Gape Analyses
The band-tailed pigeon is the passenger pigeon's closest living relative. The species falls along a basal branch of Patagioenas, which is sister to Ectopistes (Johnson et al., 2010; Fulton et al., 2012). Band-tailed pigeons, which are common in forests of western and southwestern North America (Bucher, 1992; Sanders, 2015), are physically and ecologically similar to passenger pigeons. Both species consume seed and fruits from the same genera/families of plants (Cottam and Knappen, 1939; Neff, 1947; Mathewson, 2005; Greenberg, 2014). Band-tailed pigeons have minutely larger skull dimensions, but size measurements overlap considerably between the species (Figure 1). We thus assume the band-tailed pigeon to be a suitable morphological and physiological proxy for studying the dietary ecology of the passenger pigeon.
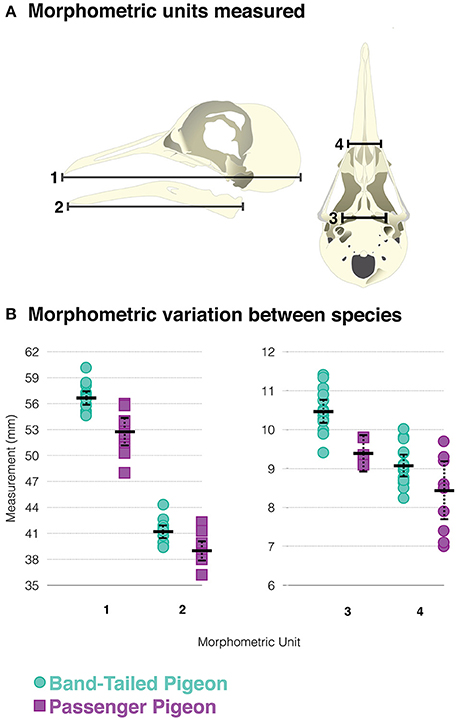
Figure 1. (A) Diagram of dry skull morphometric measurements used to compare passenger pigeons and band-tailed pigeons. (B) Comparison of passenger pigeon and band-tailed pigeon morphometric variation. Black bar = mean, dotted-lines delineate 95% confidence interval.
Pigeons swallow seeds whole, the maximum size of which is limited by bill gape. To estimate the passenger pigeon's potential dietary range in seed size, we constructed two-dimensional models of bill structure (two-dimensional cutouts from a paper-based material, 1.5 mm in thickness, which provided enough stiffness to block passage of seeds too large to pass through the gape) and determined consumable seed size as those small enough to pass through the models. In pigeons, the mid-region of each ramus of the lower mandible is flexible (Figure 2A), which allows gape to expand significantly beyond the resting state (Burton, 1974; Bout and Zweers, 2001). Maximum gape size cannot be determined from preserved specimens, which lack flexibility. To estimate maximum gape size from preserved specimens, we determined the ratio of expanded to resting gape measurements in 10 recently deceased band-tailed pigeons [obtained from the California Department of Fish and Wildlife (CDFW), Rancho Cordova, CA] and multiplied resting gape by this ratio. To verify that the gape kinetics of one pigeon species can be used to model the gape kinetics of another species, we measured the resting and expanded gapes of seven mourning doves (Zenaida macroura) and nine collared doves (Streptopelia decaocto)—also obtained from the CDFW Region 2 Wildlife Laboratory—and one mourning dove from the Alpine Animal Hospital, Palo Alto, CA. All pigeon species exhibited similar gape ratios of expanded to resting states (Supplementary Data and Statistics). We constructed three gape models: a maximum gape model extrapolated from our kinetic measurements (Supplementary Data and Statistics, Figure S1), and a minimum gape model which allowed acorns of ≤ 12.9 mm diameter to pass based on the average acorn size consumed by wild band-tailed pigeons recorded by Fry and Vaughn (1977); and an intermediate gape model representing the midpoint of the maximum and minimum models (Figure 2B).
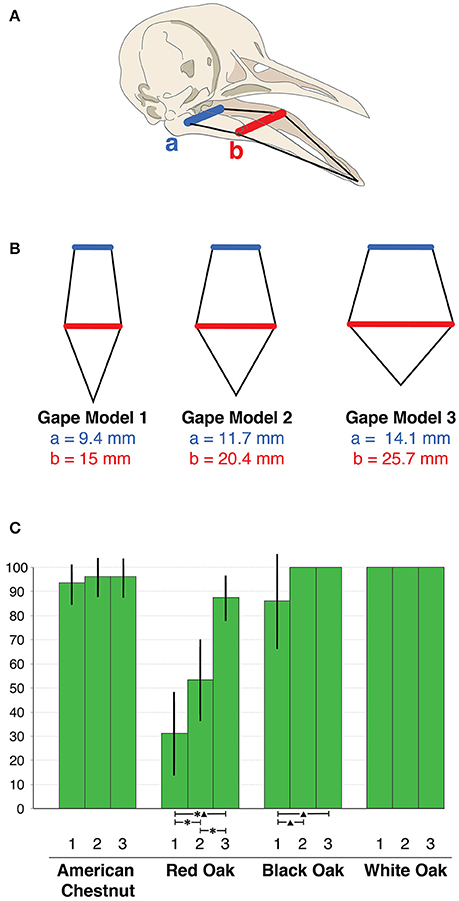
Figure 2. (A) Diagram of a columbid skull showing the constant dimensions of the lower mandibular rami (black lines) and the expandable dimensions of the lower mandibular gape (quadrate expansion: blue line a; mid-rami inflection zone: red line b). (B) The three gape models are shown to relative scale with actual measurements of expandable dimensions listed. (C) Each green bar displays the mean percentage of mast crop samples passing through each gape model for each tree species – gape models indicated on x axis. 95% confidence intervals displayed and statistically different means (p > 0.05) between gape model pairs indicated by asterisks for one-way ANOVA and a triangle for Kruskall-Wallis tests.
Passenger pigeons were documented to consume seeds of many large seed bearing tree species, particularly multiple oak species, which vary considerably in size within and between taxa (Aizen and Woodcock, 1992; Figure S2). We collected seed samples from several large seed-bearing tree species known or suspected to have been consumed by passenger pigeons, including northern red oak (935 acorns from six sites), black oak (Quercus velutina) (415 acorns from four sites), white oak (Quercus alba) (618 acorns from three sites), and American chestnut (83 chestnuts from four sites). The American chestnuts used in this study were from pure American chestnuts from trees derived from stands established prior to the species' virtual extinction, not recently established hybrid or transgenic trees. Acorn caps were removed as passenger pigeons are reported to have done this before consumption (Gibbs, 1892). We then recorded the proportion of collected seeds that successfully passed through each model.
We compared the results of seed passage through each gape model for each mast-crop data set (Supplementary Data and Statistics). Assuming a normal seed-size distribution, we performed One-way ANOVA followed by Bonferoni's multiple comparisons test using GraphPad Prism version 5.00 for Windows, GraphPad Software, San Diego California USA, www.graphpad.com. To compensate for potential non-normal distribution, we performed a Kruskal-Wallis test with Dunn's multiple comparison test using the same software.
Seed Digestion
We next performed an experiment to measure the proportion of consumed seeds that remained viable after passage through the pigeon digestive system. We obtained four captive-bred adult band-tailed pigeons (two males and two females) from Exotic Wings International (Hemet, California), which we housed in separate box-aviaries (Figure S3). During a 3 month acclimatization period, we fed the pigeons a base diet of ZuPreem® fruit blend pellets and a custom mixed grain/seed feed. Although the custom mix was occasionally replaced by commercial seed mixes during the experimental period due to availability constraints, the nutrient composition of the base diet was not significantly altered. We examined guano collected from each bird microscopically (40X magnification) to confirm that each bird was free from parasites and that the experimental aviary environment induced no dietary stress. To mimic the common aviary environment and allow continued exchange of microbiota among individual birds, we randomly rearranged water dishes among the four box-aviaries every 2 days. This experimental design was intended to allow each bird to serve as an independent replicate of the same physiological environment for seed digestion. Finally, we measured the mass of guano produced by each bird over a 6-day interval prior to experimentation, which we then compared to that produced during the experimental period to ensure that the experimental diet did not inhibit natural food consumption. The experiment was performed in compliance with the approved University of California Santa Cruz IACUC protocol SHAPB1506.
We purchased germination-competent seeds of northern red oak, white pine (Pinus strobus), beech (Fagus grandifolia), red maple (Acer rubrum), yellow birch (Betula alleghaniensis), and American elm (Ulmus americana) from commercial vendors (see Supplementary Information for sources). All of these seeds reportedly were consumed by passenger pigeons (Cottam and Knappen, 1939; Schorger, 1955; Greenberg, 2014) and represent a broad diversity of seed size, shape, and composition (factors that may affect digestion).
After the 3-month aviary acclimatization period, we performed four sequential trials in which we hand-fed seeds to each bird using the following experimental design: One male and one female were fed a combination of all seed types at once for all four trials. The other male and female were fed one seed-type per trial in randomized order. This experimental design was chosen in order to account for potential effects that digestion of one seed type on the digestion of a different seed type when consumed together or in sequence. The number of seeds fed ranged from 3 to 5 for each seed type when fed in combination and 3 to 20 when fed individually. This number was based on total seed volume, with the aim to prevent possible complications during hand-feeding, such as aspiration. We chose to hand-feed subjects to ensure the consumption of controlled numbers of seeds between replicates. Seeds were unaltered except in the case of red maple, for which we removed the wing to facilitate hand feeding; this did not affect the integrity of the seed coat encapsulating the embryo.
We collected guano from each box-aviary for 36 h following each hand-feeding trial. We examined the guano macro- and microscopically for potentially viable seeds.
Post-Mortem Seed Dispersal
We next performed a field-based experiment to assess whether post-mortem scavenging of pigeon carcasses might have resulted in the dispersal of viable seeds. Seeds stored in the crop are not digested and any seed stored in the crop upon death may be dispersed. Although any seed type can be used to test the potential of dispersal, we chose acorns for this experiment for ease of observation and to relate our work to previous speculations of passenger pigeon/prey relationships, which most often focus on oak species. We purchased 20 fresh whole rock pigeon carcasses from Palmetto Pigeon Plant, Sumter, South Carolina. We filled the crop of each bird with 10 germination competent acorns (purchased as described above). Two birds each were placed at 10 locations in mixed hardwood forests of Southern Worcester County, Massachusetts (centered near 42.0936°N, −71.7167°W; Figure S4; Table S4). Prior to carcass placement, we swept away forest floor debris to remove non-experimental acorns. At each site, one bird was wrapped in chicken wire caging material and secured to nearby saplings to prevent excessive disturbance by scavengers; the second bird was staked out to allow scavenger consumption but prevent the carcass from being dragged away (Figure S3B). Leaf litter was then spread over the sites to match the surrounding forest floor. We visited each site periodically over 98 days and recorded carcass decay, carcass disturbance, and release and condition of experimental acorns. At the end of the observation period, we dissected the undisturbed carcasses and examined their remaining crop contents.
Results
Consumable Seeds by Gape Modeling Analysis
The proportion of consumable seeds varied by tree species and gape model (Figure 2C). Even the smallest gape model indicates that passenger pigeons were able to consume the full size range of white oak seeds obtained from our sampled localities. In contrast, between 13 and 69% of the red oak acorns were too large to be eaten by passenger pigeons. Seed size distributions for black oak and American chestnut are intermediate between red and white oak, thus affording a possible refuge in size for a small proportion of the seeds produced by black oak and chestnut.
Seed Digestion
We fed a total of 289 seeds to the four band-tailed pigeons, none of which remained intact in guano (Table 1). Three of the four pigeons regurgitated seeds during the first two trials (seven acorns and one beechnut in total), including the two birds that were fed only acorns (Tables S1–S3). Because all regurgitation events occurred during the first two of four trials, we hypothesize that regurgitation reflected adjustment to the experimental diet, and that these numbers do not reflect rates of regurgitation in the wild.
No signs of indigestion (such as diarrheic guano) were observed during the experimental period. Hourly guano production (g/h) during the experiment was not statistically different from guano production outside of the experimental period (p > 0.05, Supplementary Data and Statistics). The band-tailed pigeon guano contained gastroliths, amorphous food items (possibly partially digested seeds or feathers), and acorn seed coat fragments (Figure S5). Seed coats from the base diet were rare.
Post-Mortem Seed Dispersal
Four of 10 caged rock pigeon carcasses remained undisturbed for the 98-day observation period (Table S6). Scavengers removed the pigeon carcasses from their caging at six sites, and at one site consumed portions of the carcass through the caging. Scavengers removed eight of the staked birds within the first 14 days (Table S5). We observed scattered feathers at many sites, suggesting that at least partial consumption by the scavengers took place on site prior to carcass removal. At seven of the ten sites, we found intact experimental acorns among the scattered feathers or partial carcasses, indicating that some acorns had been released from the crop (Figure 3A). This result is similar to that of Bucher and Bocco (2009) for eared doves. Two acorns germinated at two sites, but both germinations arrested without producing seedlings. Insect boring on the experimental acorns was also observed at two sites. Most of the experimental acorns disappeared, probably taken with the carcass or consumed after release. No acorn dispersal was observed for the four caged carcasses that remained undisturbed. We recovered only a single damaged acorn from a disturbed caged carcass (Figure S6); all other experimental acorns appear to have decomposed. All carcasses had decomposed completely by day 35, leaving only feathers and bones (Figure 3B).
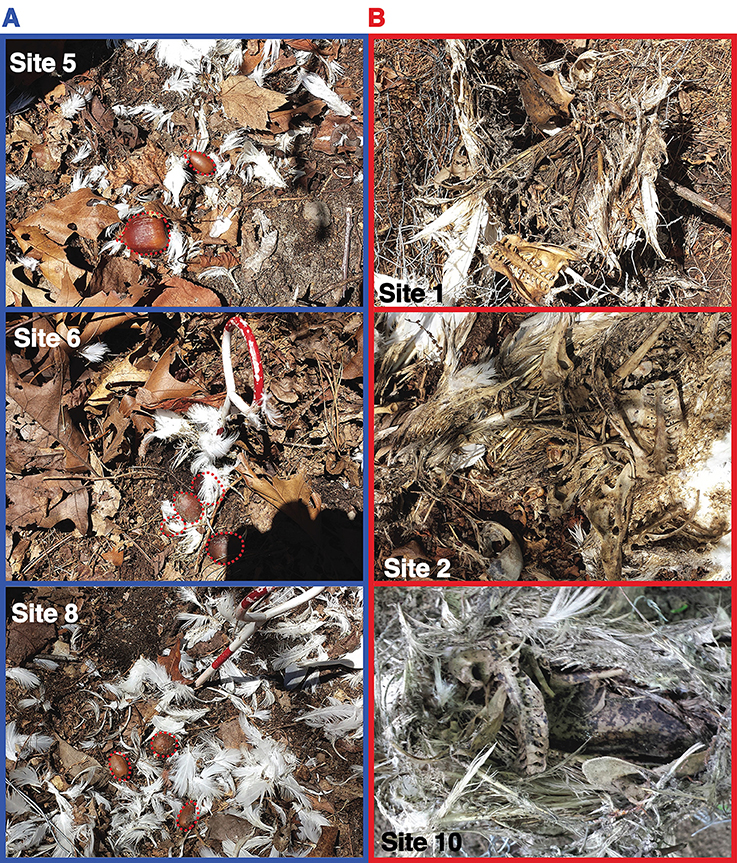
Figure 3. (A) Examples of post-mortem released acorns from staked carcasses in blue inset images. Acorns are outlined in dashed red-line. (B) Examples of caged-carcass decay in red inset images.
Discussion
Until their rapid demise in the nineteenth century, hundreds of millions to perhaps billions of passenger pigeons inhabited deciduous forests of northeastern North America (Greenberg, 2014). This vast population is thought to have persisted for at least several tens of thousands of years (Murray et al., 2017). Such abundance over so long a time period leads almost inevitably to the conclusion that passenger pigeons figured prominently in the workings of eastern deciduous forest ecosystems. Although the precise nature of the processes and their ecological and evolutionary outcomes remain uncertain, it is easy to imagine passenger pigeon impacts as consumers of the species they ate; as a nutritional resource to the species that ate them; as competitors or mutualists with other species; and as vectors of disease and the movement of nutrients and other materials within and among forest communities.
Our focus here has been on passenger pigeons as consumers and seed producing trees as their prey, a decision based in part on the fundamental importance of consumer–prey interactions to living nature; in part on the reportedly vast numbers of passenger pigeons and the almost inescapable conclusion that these birds were important consumers; and in part to previous hypotheses concerning the consumer-prey interactions between passenger pigeons and seed-producing trees.
Many seed producing tree species in northeast North America do so by masting. Masting, while costly in terms of energy and material investment, also can provide counteracting benefits by satiating seed consumers (Janzen, 1971). Passenger pigeons, as the most abundant seed consumer in eastern deciduous forests, may thus have figured into the evolution of masting cycles. Similarly, the pulsed food resources provided by masting trees may have figured into the ecology and evolution of passenger pigeons.
We have shown that viable seeds do not pass through a band-tailed pigeon's digestive system, thus suggesting that the interaction between closely related passenger pigeons and seed-bearing trees was antagonistic rather than mutualistic. Although this conclusion is supported from a digestive perspective, other components of passenger pigeon foraging and food handling behavior, many of which are not possible to explore experimentally with an extinct species, will also influence their potential effectiveness as seed dispersers (Howe and Smallwood, 1982; Schupp et al., 2010). Our studies of seed-engorged pigeon carcasses establish that viable seeds remaining in the birds' crops following death could have been dispersed by predators and scavengers, even if the deceased bird fell directly beneath the seed's parent tree. Observations from our digestive study also show that seeds that are regurgitated soon after consumption remain viable. If we extrapolate from historical records of passenger pigeon foraging behavior to known seed dispersal pathways (Schorger, 1955; Schupp et al., 2010), it is reasonable to hypothesize that passenger pigeons were most effective as seed dispersers during foraging/nomadic migrations, when seeds may have been released either via regurgitation or post-mortem over areas in which secondary consumers could have enhanced dispersal (i.e., diplochory). Although the probability of a given seed being dispersed in such ways by an individual pigeon was likely very low, the great abundance of pigeons, the extreme longevity of trees after reaching seed-bearing age, and the vast number of seeds produced through repeated masting events over a tree's lifetime combine to cloud the nature of basic ecological interactions between passenger pigeons and trees.
Estimates of passenger pigeon bill gape width and seed size indicate that the seeds of some tree species (i.e., red oaks, black oak, and American chestnut) had a partial refuge in size from passenger pigeon consumption whereas others (i.e., white oak) did not. This varying feature of seed morphology among tree species might have influenced tree fitness, especially if large flocks of foraging pigeons were capable of consuming all or most of an individual tree's mast set during any particular year (possible given the great abundance of passenger pigeons). Under this circumstance, trees producing even a small proportion of unconsumable seeds may have realized an advantage in fitness over those that did not, despite the greater cost of making larger seeds.
Shifts in seed size have been noted to occur rapidly after the extinction of avian seed dispersers (Galetti et al., 2013). If passenger pigeons influenced the fitness of trees through size-selective predation on their seeds, the size of seeds produced by particular trees might well have undergone evolutionary responses to the absence of abundant passenger pigeons during the past 130 years, in turn influencing seed dispersal distance, germination rate, and the foraging ecology of other seed-eating species.
Other potential responses to passenger pigeon seed predation are asynchronous masting and changes in the timing of germination. After noting an overlap in white oak dominance in regions of spring-time pigeon occurrence, Ellsworth and McComb (2003) hypothesized that the autumn germination of white oaks allowed them to escape spring time predation by pigeons, further allowing white oaks to dominate over red oaks in northern areas commonly occupied by passenger pigeons during spring months. These authors interpreted a shift in dominance from white to red oak after the passenger pigeon's extinction as further evidence in support of their hypothesis. This hypothesis rests on the assumption that no red oak acorns could ascape seed predation; however neither of the above patterns are consistent with our finding that some 13–69% of red oak mast crops would have evaded passenger pigeon predation by way of their refuge in seed size. Moreover, expansion of red oaks in the early twentieth century has been attributed to the overall change in populations of seed predators (e.g., turkey, deer, pigeons), selective logging, fire suppression (Abrams, 2003; Dey, 2014), and the loss of physical disturbance by way of limb breakage and guano production with the passenger pigeon's demise (Novak, 2016).
The apparent rarity of chestnut consumption by passenger pigeons (Cottam and Knappen, 1939; Schorger, 1955) is perplexing given the reportedly great historical abundance of American chestnut trees (Russell, 1987), the chestnut's nutritional content and palatability (Steele et al., 2005), a pattern of regular annual seed set by chestnuts (Diamond et al., 2000); and our data establishing that the majority of American chestnut seeds were small enough to be consumed by pigeons. Perhaps the chestnut's spiny seed capsule deterred passenger pigeons from feeding on chestnuts, leading in turn to the differing seed production patterns between oaks (which mast episodically) and chestnuts (which mast annually) and a comparative advantage between oaks and chestnuts that might have shifted from the latter to the former with extinction of the passenger pigeon?
The influences of passenger pigeon predation on oaks might also have varied geographically. A comparison of our results to those reported by Aizen and Woodcock (1992) suggest that black oak, scarlet oak, tukey oak, northern red oak, shumard oak, swamp white oak, chestnut oak, bur oak, and white oak all produce acorns that are large enough to escape predation by passenger pigeons, whereas all white oak acorns sampled in our study were small enough to be eaten by passenger pigeons (Figure S7). These differing results make sense in light of within-species geographic variation of seed sizes, which Aizen and Woodcock found decreases from southern to northern latitudes for most oak species. The mast crop samples used in our study were all obtained between 38° and 48° latitude and are similar in size to Aizen and Woodcock reported size range for that same latitude (Figure S8). Overall, Aizen and Woodcocks' data would suggest that black oak, white oak, and red oak all produce seeds large enough to evade passenger pigeon predation, but moreso in southern latitudes. In general red oak trends to exhibit a greater advantage over white oak throughout their overlapping latitudinal ranges (Figure S9). Further sampling of greater geographic ranges and other large seed-bearing oak species will help to clarify the historic impacts of passenger pigeons throughout their former range.
Much of the preceding discussion is necessarily speculative. Nonetheless, seed predation by passenger pigeons probably influenced the size and number of seeds produced, the timing of seed production and release, seed quality as a nutritional resource to pigeons and other seed predators, and the manners in which life history characteristics influenced the distribution and abundance of various tree species in eastern deciduous forests before and after the passenger pigeon's demise. We urge that future research on the ecological dynamics and evolution of forest ecosystems be undertaken with these processes in mind.
Conclusions
Our results provide new insights into the diet of passenger pigeons and related impacts on the structure and dynamics of eastern North American forests. In agreement with previous hypotheses, we find that passenger pigeons were primarily seed predators (Janzen, 1971; Ellsworth and McComb, 2003) although they may also have contributed to seed dispersal (Webb, 1986; Aizen and Patterson, 1990). We also identify physical limitations of passenger pigeon gape that would have affected their ability to consume and disperse the largest seeds of eastern North American tree species. Given the historical abundance of passenger pigeons, our results are useful for interpreting their ecological impacts on North American trees, understanding the potential co-evolutionary consequences of these interactions, and better understanding possible influences of the species' extinction.
Ethics Statement
This study was carried out in accordance with the recommendations of the UCSC Institutional Animal Use and Care Committee; protocol SHAPB1506.
Author Contributions
BN, JE, and BS conceived the research design, interpreted results, and wrote the manuscript. BN collected morphometric data and carried out gape model and digestive experiments. EN assisted in collecting gape kinetic data and handling subjects for digestive experiments, screened guano for parasites, and monitored subject health. HS conducted post-mortem seed dispersal experiments.
Funding
Funding was provided by the Founding Funders, Angel Funders, and many supporters of Revive & Restore (http://reviverestore.org/our-supporters/).
Conflict of Interest Statement
BN was employed by Revive & Restore, a non-profit conservation organization. The authors declare that this study received funding from Revive & Restore. The funder was not involved in the study design or collection, analysis, or interpretation of the data.
The others authors declare that the research was conducted in the absence of any commercial or financial relationships that could be construed as a potential conflict of interest.
Acknowledgments
Data presented in this paper first appeared in the master's thesis of BN. We thank Sal Alvarez and Paul Marini for their guidance concerning band-tailed pigeon care. Paul Marini also liaised purchase of pigeon carcasses from Palmetto Pigeon Plant. Peter Capainolo and Brian Schmidt provided passenger pigeon skull measurements. We thank Carla Cicero and Krysta Rogers for access to specimens. Joseph Richinelli, John Bender, Gina Gilliland, Don Sparks, Gayle Brillhart, Bill Sayers, and Jeff and Cheryl Carpenter collected oak mast crop samples used in this study. William A. Powell and Andy Newhouse provided chestnuts from the SUNY ESF American Chestnut Restoration Project. We thank Mathew Bruce for consultation for statistical analyses. We thank Richard Ostfield, Ian Roderer, and Sylvia B. Shaw for comments on earlier drafts of the manuscript. Experimentation with live birds was preapproved by the UCSC Institutional Animal Use and Care Committee.
Supplementary Material
The Supplementary Material for this article can be found online at: https://www.frontiersin.org/articles/10.3389/fevo.2018.00020/full#supplementary-material
References
Abrams, M. D. (2003). Where has all the White Oak gone? Bioscience 53, 927–939. doi: 10.1641/0006-3568(2003)053[0927:WHATWO]2.0.CO;2
Aizen, M. A., and Patterson, W. A. (1990). Acorn size and geographical range in the North American oaks (Quercus L.). J. Biogeogr. 17, 327–332. doi: 10.2307/2845128
Aizen, M. A., and Woodcock, H. (1992). Latitudinal trends in acorn size in eastern North American species of Quercus. Can. J. Bot. 70, 1218–1222. doi: 10.1139/b92-153
Blockstein, D. E., Maxwell, B. D., and Fay, P. K. (1987). Dispersal of leafy spurge seeds (Euphorbia esula) by mourning doves (Zenaida macroura). Weed Sci. 35, 160–162.
Bout, R. G., and Zweers, G. A. (2001). The role of cranial kinesis in birds. Comp. Biochem. Physiol. A 131, 197–205. doi: 10.1016/S1095-6433(01)00470-6
Bucher, E. H. (1992). “The causes of passenger pigeon extinction,” in Current Ornithology, ed D. M. Power (New York, NY: Plenum Press), 1–36.
Bucher, E. H., and Bocco, P. J. (2009). Reassessing the importance of granivorous pigeons as massive, long-distance seed dispersers. Ecology 90, 2321–2327. doi: 10.1890/08-2077.1
Burton, P. J. K. (1974). Jaw and tongue features in psittaciformes and other orders with special reference to the anatomy of the tooth-billed pigeon (Didunculus sfrigirostris). J. Zool. 174, 255–276. doi: 10.1111/j.1469-7998.1974.tb03156.x
Cottam, C., and Knappen, P. (1939). Food of some uncommon North American birds. Auk 56, 138–169. doi: 10.2307/4078038
Dey, D. C. (2014). Sustaining oak forests in Eastern North America: regeneration and recruitment, the pillars of sustainability. For. Sci. 60, 926–942. doi: 10.5849/forsci.13-114
Diamond, S. J., Giles, R. H., Kirkpatrick, R. L., and Griffin, G. J. (2000). Hard mast production before and after the chestnut blight. South. J. Appl. For. 24, 196–201.
Ehrlich, P. R., and Raven, P. H. (1964). Butterflies and plants: a study in coevolution. Evolution 18, 586–608.
Ellsworth, J. W., and McComb, B. C. (2003). Potential effects of passenger pigeon flocks on the structure and composition of presettlement forests of Eastern North America. Conserv. Biol. 17, 1548–1558. doi: 10.1111/j.1523-1739.2003.00230.x
Fry, M. E., and Vaughn, C. E. (1977). Acorn selection by band-tailed pigeons. Calif. Fish Game 63, 59–60.
Fulton, T. L., Wagner, S. M., Fisher, C., and Shapiro, B. (2012). Nuclear DNA from the extinct passenger pigeon (Ectopistes migratorius) confirms a single origin of New World pigeons. Ann. Anat. 194, 52–57. doi: 10.1016/j.aanat.2011.02.017
Galetti, M., Guevara, R., Côrtes, M. C., Fadini, R., Von Matter, S., Leite, A. B., et al. (2013). Functional extinction of birds drives rapid evolutionary changes in seed size. Science 340, 1086–1090. doi: 10.1126/science.1233774
Hererra, C. M. (1985). Determinants of plant-animal coevolution: the case of mutualistic dispersal of seeds by vertebrates. OIKOS 44, 132–141. doi: 10.2307/3544054
Howe, H. F., and Smallwood, J. (1982). Ecology of seed dispersal. Annu. Rev. Ecol. Syst. 13, 201–228. doi: 10.1146/annurev.es.13.110182.001221
Janzen, D. H. (1971). Seed predation by animals. Annu. Rev. Ecol. Syst. 2, 265–492. doi: 10.1146/annurev.es.02.110171.002341
Johnson, K. P., Clayton, D. H., Dumbacher, J. P., and Fleischer, R. C. (2010). The flight of the passenger pigeon: phylogenetics and biogeographic history of an extinct species. Mol. Phylogenet. Evol. 57, 455–458. doi: 10.1016/j.ympev.2010.05.010
Lambert, F. (1989). Pigeons as seed predators and dispersers of figs in a Malaysian lowland forest. Ibis 131, 521–527. doi: 10.1111/j.1474-919X.1989.tb04788.x
Loehle, C. (1987). Tree life history strategies: the role of defenses. Can. J. For. Res. 18, 209–222. doi: 10.1139/x88-032
Mathewson, W. (2005). Band-tailed Pigeons: Wilderness Bird at Risk, 1st Edn. Portland, OR: Timber Press, Incorporated.
McConkey, K. R., Meehan, H. J., and Drake, D. R. (2004). Seed dispersal by Pacific Pigeons (Ducula pacifica) in Tonga, Western Polynesia. Emu 104, 369–376. doi: 10.1071/MU03060
Murray, G. G. R., Soares, A. E. R., Novak, B. J., Schaefer, N. K., Cahill, J. A., Baker, A. J., et al. (2017). Natural selection shaped the rise and fall of passenger pigeon genomic diversity. Science 358, 951–954. doi: 10.1126/science.aao0960
Neff, J. A. (1947). Habits, food, and economic status of the band-tailed pigeon. North Am. Fauna 58, 1–56. doi: 10.3996/nafa.58.0001
Novak, B. J. (2016). Deciphering the Ecological Impact of the Passenger Pigeon: A Synthesis of Paleogenetics, Paleoecology, Morphology, and Physiology. Dissertion, University Of California, Santa Cruz.
Russell, E. W. B. (1987). Pre-blight distribution of Castanea dentata (Marsh.) Borkh. Torrey Bot. Soc. 114, 183–190. doi: 10.2307/2996129
Sanders, T. A. (2015). Band-Tailed Pigeon Population Status. (Washington, DC: U.S. Department of the Interior, Fish and Wildlife Service, Division of Migratory Bird Management), 19.
Schorger, A. W. (1955). The Passenger Pigeon: Its Natural History and Extinction, 1st Edn. (Madison, WI: The University of Wisconsin Press), 424.
Schupp, E. W. (1993). “Quantity, quality, and the effectiveness of seed dispersal by animals,” in Frugivory and Seed Dispersal: Ecological and Evolutionary Aspects, eds T. H. Fleming and A. Estrada (Dordrecht: Springer), 15–29.
Schupp, E. W., Jordano, P., and Gómez, J. M. (2010). Seed dispersal effectiveness revisited: a conceptual review. New Phytol. 188, 333–353. doi: 10.1111/j.1469-8137.2010.03402.x
Steele, M. A., Mccarthy, B. C., and Keiffer, C. H. (2005). Seed dispersal, seed predation, and the american chestnut. J. Am. Chestnut Found. 19, 47–54.
Vander Wall, S. B. (2001). The evolutionary ecology of nut dispersal. Bot. Rev. 67, 74–117. doi: 10.1007/BF02857850
Webb, S. L. (1986). Potential role of passenger pigeons and other vertebrates rapid holocene migrations of nut trees. Quat. Res. 375, 367–375. doi: 10.1016/0033-5894(86)90096-7
Keywords: passenger pigeon, extinct, oak, American chestnut, seed predation, seed dispersal, coevolution, ecological interactions
Citation: Novak BJ, Estes JA, Shaw HE, Novak EV and Shapiro B (2018) Experimental Investigation of the Dietary Ecology of the Extinct Passenger Pigeon, Ectopistes migratorius. Front. Ecol. Evol. 6:20. doi: 10.3389/fevo.2018.00020
Received: 18 November 2017; Accepted: 16 February 2018;
Published: 05 March 2018.
Edited by:
Jennifer Leonard, Consejo Superior de Investigaciones Científicas (CSIC), SpainReviewed by:
Brenda C. McComb, Oregon State University, United StatesMekala Sundaram, Brown University, United States
Copyright © 2018 Novak, Estes, Shaw, Novak and Shapiro. This is an open-access article distributed under the terms of the Creative Commons Attribution License (CC BY). The use, distribution or reproduction in other forums is permitted, provided the original author(s) and the copyright owner are credited and that the original publication in this journal is cited, in accordance with accepted academic practice. No use, distribution or reproduction is permitted which does not comply with these terms.
*Correspondence: Ben J. Novak, YmVuQHJldml2ZXJlc3RvcmUub3Jn
†Present Address: Erika V. Novak, Geelong Animal Emergency, Geelong, VIC, Australia