- 1Oregon Institute of Marine Biology, University of Oregon, Charleston, OR, United States
- 2Friday Harbor Laboratories, University of Washington, Friday Harbor, WA, United States
Sea urchins are ecosystem engineers of nearshore benthic communities because of their influence on the abundance and distribution of macroalgal species. Urchins are notoriously inefficient in assimilation of their macroalgal diets, so their fecal production can provide a nutritional subsidy to benthic consumers that cannot capture and handle large macroalgae. We studied the assimilation of macroalgal diets by urchins by analyzing the profiles of trophic biomarkers such as fatty acids (FAs). We tracked macroalgal diet assimilation in both Strongylocentrotus droebachiensis and S. purpuratus. Juvenile S. droebachiensis and adult S. purpuratus were maintained for 180 and 70 days, respectively, on one of three monoculture diets from three algal phyla: Nereocystis luetkeana, Pyropia sp., or Ulva sp. We then analyzed FA profiles of the macroalgal tissue fed to urchins as well as urchin gonad, gut, digesta, and egesta (feces) to directly evaluate trophic modification and compare nutritional quality of urchin food sources, urchin tissues, and fecal subsidies. In the S. purpuratus assay, there were significantly more total lipids in the digesta and egesta than in the algae consumed. The FA profiles of urchin tissues differed among urchin species, all diets, and tissue types. Despite these differences, we observed similar patterns in the relationships between the urchin and macroalgal tissues for both species. Egesta produced by urchins fed each of the three diets were depleted with respect to the concentration of important long chain polyunsaturated fatty acids (LCPUFAs), but did not differ significantly from the source alga consumed. Both urchin species were shown to synthesize and selectively retain both the precursor and resulting LCPUFAs involved in the synthesis of the LCPUFAs 20:4ω6 and 20:5ω3. S. droebachiensis and S. purpuratus exhibited consistent patterns in the respective depletion and retention of precursor FAs and resulting LCPUFAs of Pyropia and Ulva tissues, suggesting species level control of macroalgal digestion or differential tissue processing by gut microbiota. For both S. droebachiensis and S. purpuratus, macroalgal diet was a surprisingly strong driver of urchin tissue fatty acids; this indicates the potential of fatty acids for future quantitative trophic estimates of urchin assimilation of algal phyla in natural settings.
Introduction
Lipids are dense sources of metabolic energy that provide building blocks of cellular membranes and are involved in intracellular signaling and localized hormone control (Christie and Han, 2010). Fatty acids (FAs) from multiple lipid classes are integral in membrane function, energy storage, and many physiological processes (Arts et al., 2009). Fatty acids that cannot be synthesized by animals de novo in biologically relevant amounts and must be obtained from dietary sources are generally referred to as “essential fatty acids” (EFAs; Arts et al., 2001). Marine primary producers, particularly macroalgae and phytoplankton, are rich sources of lipids including polyunsaturated fatty acids (PUFAs) that are important for growth and reproduction of marine organisms (Dalsgaard et al., 2003; Wacker and Von Elert, 2004). Specifically, long chain polyunsaturated fatty acids (LCPUFAs, more than 20 carbon atoms), many of which are considered to be EFAs for consumers, have been identified as a key dietary component supporting growth and have been used to evaluate food quality (Sargent et al., 1999; Bell and Sargent, 2003; Winder et al., 2017).
Many FAs have been used as dietary biomarkers or tracers to explore trophic relationships in aquatic, pelagic, and benthic consumers (reviewed by Dalsgaard et al., 2003; Müller-Navarra, 2008; Kelly and Scheibling, 2012). However, dietary FAs are often modified by the consumer, either by elongation, desaturation, or de novo synthesis of FAs from precursors present in dietary tissues (Cook et al., 2007; González-Durán et al., 2008; Brett et al., 2016). Our understanding of FA trophic modification by marine invertebrates is still developing, and sea urchins are an interesting case study for this question because they have been studied for the sake of aquaculture (Castell et al., 2004). Additionally, when a certain EFA is limited in food sources, many consumers, including sea urchins, can selectively store the EFAs for later use, and as a result the FAs observed in consumer tissues often do not directly reflect their food sources (Castell et al., 2004; González-Durán et al., 2008).
Organismal FA composition can also vary seasonally and spatially, complicating the use of FA as biomarkers (Hughes et al., 2005; Dethier et al., 2013). FA profile variation in producers and consumers may be influenced by local abiotic conditions such as nutrient availability, temperature, salinity, and light (Galloway and Winder, 2015). However, more variation in FA profiles is explained by phylogeny, reflecting relatedness, than by abiotic conditions in aquatic ecosystems (Galloway and Winder, 2015). Because multivariate FA profiles can be reliably associated with a variety of primary producers and consumers, the use of FAs as biomarkers is supported despite local seasonal and site-specific variation (Galloway et al., 2012, 2013; Kelly and Scheibling, 2012; Dethier et al., 2013).
Sea urchins are ecologically and economically important organisms that can exert a strong influence on community composition primarily through grazing pressure (Steneck et al., 2002; Pearse, 2006). Urchins are known to transform significant amounts of kelp-derived biomass into particulate organic matter (e.g., Koike et al., 1987) through their fecal production (Sauchyn and Scheibling, 2009; Sauchyn et al., 2011), which enhances growth of associated microbes. Moreover, urchins are notoriously inefficient assimilators of their diet (Vadas, 1977), such that a large portion (e.g., 40–80%) of the macronutrients from Strongylocentrotus droebachiensis algal diets are left intact in the resulting feces (Mamelona and Pelletier, 2005). Urchin trophic modification of macroalgal tissue may represent a trophic hub between benthic and pelagic systems (Hughes et al., 2011). Urchins can produce tissues of high nutritional quality, as measured by content of key FAs, as a subsidy to larval pelagic and benthic consumers (direct predation on gonad tissue) while generating lower quality tissues for benthic detritivores (fecal material; Hughes et al., 2011).
Urchin gonad material (uni) is an important fishery (Andrew et al., 2003). Aquaculture studies have extensively investigated ratios of lipids in urchin diets that maximize the incorporation of essential FAs into gonad production and growth (Castell et al., 2004; Cook et al., 2007) for the sake of improved uni production. In pursuit of ideal FA ratios in diets, researchers have noted that urchins are capable of de novo synthesis of novel FAs, including non-methylene interrupted dienes (NMID; Takagi et al., 1980; Castell et al., 2004; González-Durán et al., 2008). This capability exists in multiple species of urchin including S. droebachiensis and is likely the result of Δ5 desaturase activity, which is also involved in the synthesis of arachidonic acid (ARA, 20:4ω6) from 20:3ω6 and of eicosapentaenoic acid (EPA, 20:5ω3) from 22 carbon chain monounsaturated fatty acids (MUFAs; Takagi et al., 1980).
To better understand dietary FA trophic transfer in urchins and the universality of the role that urchin digestion of macroalgae plays in either enhancing or depleting the nutritional quality of the material egested, we performed controlled laboratory feeding assays with two species of urchin, S. droebachiensis and S. purpuratus. S. droebachiensis experiments were conducted with newly settled juveniles, and S. purpuratus experiments used field-collected adults. We addressed four primary objectives: (1) Assess similarities and differences in the total lipid and FA content of tissues of urchins maintained on controlled algal diets; (2) Evaluate whether urchins alter the nutritional quality of their own tissue and of egested material by quantifying the concentrations of total lipid and long-chain polyunsaturated fatty acid (LCPUFA) of gonad, gut, alga consumed, digesta, and egesta tissues; (3) Evaluate if the urchins selectively retain LCPUFAs and their precursors; (4) test whether urchin tissue FAs can reliably be used to differentiate phylum level differences in their known algal diets.
Materials and Methods
Strongylocentrotus droebachiensis Feeding Assays
In autumn 2016, 240 recently settled S. droebachiensis (1.95 ± 0.19 mm, mean test diameter ± SD) resulting from in vitro breeding at Friday Harbor Laboratories (FHL: 48°32.7′N, 123°0.8′W) were raised on monoculture macroalgal diets for 180 days (September 2016–March 2017). Multiple juvenile urchins (N = 15) were maintained in replicate (N = 4 per diet treatment) clear, lidless, flow-through plastic bins (20 × 20 cm). Bins were kept in seawater tables at FHL on San Juan Island, WA using ambient seawater from Friday Harbor. Replicate bins were randomly assigned to one of three diet treatments, representing a species from Ochrophyta (Nereocystis luetkeana—Nereocystis throughout), Rhodophyta (Pyropia sp.—Pyropia throughout), or Chlorophyta (Ulva sp.—Ulva throughout). These algae are regularly available to urchins as attached benthic species or as drift in the intertidal and shallow subtidal, and their fatty acid (FA) signatures have clear phylogenetic resolution at the phylum level (Galloway et al., 2012). Algae were collected from the FHL property, either as drift (Nereocystis) or growing in the intertidal (Ulva and Pyropia). During the experimental period, seawater temperature declined (13–8°C) and there were some fluctuations in salinity (25–30 ppt) at the algal collection sites. Urchins were fed ad libitum; uneaten food was removed several times a week as needed and bins were cleaned regularly to prevent waste accumulation and diatom growth. Egesta were collected weekly and immediately stored at −20°C for later FA analyses. At the termination of the experimental period, urchins were euthanized and dissected to isolate urchin gonad, gut, and digesta (Hakim et al., 2015).
Strongylocentrotus purpuratus Feeding Assays
Forty-two relatively small, but unknown age, adult S. purpuratus (5.5 ± 0.6 cm, mean test diameter ± SD), were collected by hand from tide pools at Cape Arago, Oregon (43°20′ 24′′ N, 124°19′48′′ W) in August 2016. Urchins were collected from two low intertidal pools with similar temperature (13.1 and 13.0°C) and salinity (30.2 and 30.6 ppt). Urchins were immediately transported to the Oregon Institute of Marine Biology (OIMB) and haphazardly assigned individually to 4.7 L flow-through containers filled with 1 μm-filtered seawater. Filtered seawater was successfully used to impede diatom growth in the experimental chambers, which could have confounded the controlled feeding experiment if the urchins grazed on this alternative resource. Each container had both an airstone and constant filtered seawater flow. Urchins were given 2 weeks to purge their guts to remove remnants of previous diets and to acclimatize to laboratory conditions prior to the start of experimental feeding trials. During this period, fecal material was removed daily to avoid fouling. At the end of the acclimatization period, each urchin was randomly assigned to a Nereocystis, Pyropia, or Ulva diet treatment. A representative subset of the urchins maintained for the acclimatization period were dissected at the end of the acclimatization period to determine the amount of gonad tissue present at the start of the experiment.
Urchins were fed 6.0 g (± 0.1 g) of their designated alga every other day. Fresh macroalgae were collected weekly from OIMB property, either from a nearshore Nereocystis bed or intertidally (Pyropia and Ulva). The macroalgal collection site is adjacent to the seawater intake for OIMB and experiences fluctuations in seawater temperature (12–14°C) and salinity (30–33 ppt). Macroalgae were maintained in ambient flow-through seawater tables in the lab prior to presentation to urchins. No macroalgae were kept for longer than 1 week, as aging has been shown to affect kelp FA (Raymond et al., 2014). To prepare macroalgae, thalli were rinsed briefly in freshwater to dislodge mobile epibionts, e.g. amphipods, gastropods, or isopods, then inspected for sessile epibionts, which were removed if present. Cleaned macroalgae were spun dry and briefly blotted before one final inspection immediately prior to recording wet weights. Before new food was added to urchin containers, egesta (fecal material) and uneaten macroalgal thalli were removed. At three time points over the course of the 70 day experimental period, we collected and rinsed macroalgal thalli and egesta for later FA extraction and analysis. Adult S. purpuratus were euthanized and dissected as described for S. droebachiensis.
Fatty Acid Analysis
At the termination of the 180 and 70 day experimental periods, S. droebachiensis and S. purpuratus (respectively) were carefully dissected to isolate gonad, gut, and gut contents (digesta) from all other tissues. The same analysts performed all extractions on both species to maintain methodological consistency. Isolated tissues were briefly rinsed with freshwater and drained before placing samples in labeled plastic microcentrifuge tubes and frozen at −20°C. All tissues collected for total lipid and FA analysis were freeze dried for a minimum of 48 h (or until tissues were dry) within 3 weeks of collection. Following freeze-drying, each tissue type was carefully homogenized and extracted for total lipids as described by (Parrish, 1999). Briefly, homogenized tissue samples were digested for a minimum of 12 h in 2 ml chloroform and then sonicated, vortexed, and centrifuged three times in a 4:2:1 chloroform:methanol:0.9% NaCl solution. Following each of these cycles, the chloroform layers containing organics were removed and pooled. From this pooled solution, subsamples were removed for total lipid analysis by gravimetry (Kainz et al., 2017). The pooled chloroform solutions containing the organics were evaporated to dryness under N2 flow and the organics were re-suspended in a toluene and 1% sulfuric-acid methanol solution for 16 h at 50°C to trans-esterify FA methyl esters (FAME). Following transesterification, FAME mixtures were allowed to cool before neutralizing the acid with 2% KHCO3. The neutralized FAME solution was then diluted with 5 ml of hexane, and the mixture was briefly vortexed and centrifuged before carefully removing the FAME layer. The hexane addition, vortex, centrifugation and removal was repeated once more. Following the second addition of hexane, the pooled hexane solution was evaporated to dryness under N2 flow and the FAME were re-dissolved in 1.5 ml hexane in 2 ml glass vials for gas chromatography.
FAME were analyzed using a gas chromatograph equipped with a mass spectrometer (GC-MS, Model QP2020, Shimadzu) with a DB-23 column (30 × 0.25 mm × 0.15 μm, Agilent, Santa Clara, CA, USA) using helium as a carrier gas. A split/splitless injector was used to inject 1 μl of sample for analysis. The following heating program, based on Taipale et al. (2013), was used: 50°C was maintained for 1 min, then the temperature was increased at a rate of 10°C min−1 to 140°C, after which the warming rate was adjusted to 1.40°C min−1 to 190°C and held for 3 min, followed by 5°C min−1 to 220°C, and finally heated at 13°C min−1 to 240 and held for 2 min. The FA were identified from relative retention times and specific ions and then quantified using peaks of the major ions (Taipale et al., 2016). Concentrations of FAs were calculated based on calibration curves (Pearson correlation coefficient ≥ 0.99) of serial dilutions of known standard FAME mixtures (GLC standard mixture 566C, Nu-Chek Prep, Elysian, MN, USA). The FA concentrations were then standardized against the dry weight of the tissue extracted to obtain the concentrations in μg mg−1 dry weight. FA proportions represent the percentage of the sum of the total weight of FAs identified.
Statistical Analysis
We used the Shapiro–Wilks test to evaluate the normality of the total lipid content of gonad, gut, alga, digesta, and egesta tissues from both feeding assays. We then separately analyzed the total lipid of the alga tissue fed to S. droebachiensis and to S. purpuratus to evaluate differences in monoculture diets on which urchins were maintained, using a 2-way (diet × urchin species/location) analysis of variance (ANOVA, α ≤ 0.05) on untransformed data in R (R Core Team, 2015). Additionally, we used a 2-way ANOVA (diet × tissue) to evaluate the total lipid content based on diet consumed and tissue analyzed.
To evaluate the multivariate FA profiles, we used multiple routines in Primer v6.1.13 (Clarke and Gorley, 2006) with the PERMANOVA+ v.1.0.3 add-on (Anderson et al., 2008). Differences in the untransformed FA profiles of S. droebachiensis and S. purpuratus tissues were analyzed using a 3-way factorial permutational multivariate analysis of variance (PERMANOVA, α ≤ 0.05), with 9999 permutations with Type III sums of squares, mixed factors, and Euclidian distance for all tissues. The results were not sensitive to arcsine square-root transformations of FA data; we therefore report the untransformed results. To visualize the relationships between urchin species, diets, and tissue types we used non-metric multidimensional scaling (nMDS) plots. To identify the FAs contributing to differences between species, diets, and tissues we calculated the similarity percentage (SIMPER). Vector overlays of the FA identified by SIMPER analyses were then mapped onto a subset of the nMDS graphics to visualize relationships between diets for the gonad and gut (collectively referred to throughout as urchin tissues) and diet alga, digesta, and egesta (collectively referred to throughout as macroalgal tissues).
To evaluate the nutritional quality differences in tissues analyzed, we used a 2-way ANOVA of the ΣLCPUFAs, defined here as the sum of arachidonic (ARA, 20:4ω6), eicosapentaenoic (EPA, 20:5ω3), and docosahexanoic (22:6ω3) acid (Arts et al., 2001; Hughes et al., 2011). We also used 2-way ANOVAs to evaluate the mean content of EPA and ARA and precursor FAs including α-linoleic acid (LIN, 18:2ω6) and combined α-linolenic acid (ALA, 18:3ω3) and stearidonic acid (SDA, 18:4ω3) in urchin and macroalgal tissues.
Results
Based on the increase in test diameter (mm), juvenile S. droebachiensis grew an average of 873 ± 195% over 180 days, to an average test diameter of 19 ± 4 mm (mean ± standard deviation, SD) and an average wet weight of 2.77 ± 1.38 g. Growth of S. droebachiensis was extensive due to the fact that initial S. droebachiensis were newly settled larvae at the start of the experiment (initial test diameter 1.95 ± 0.19 mm). S. purpuratus also grew in their experiment, resulting in an average test size of 5.9 ± 0.6 cm, a total of 4.8 ± 6.9% change in diameter, and a 13.5 ± 9.5% increase in the mean wet weights across all diet treatments with a final mean gonad index of 0.12 ± 0.03. The mean gonad index of S. purpuratus dissected at the beginning of the experiment was 0.02 ± 0.01 (gonad wet weight: whole body wet weight), resulting from the presence of gonad material in 8 of the 10 urchins dissected. A total of 37 FAs were identified in S. droebachiensis and S. purpuratus tissues and their associated diets, digesta, and egesta, with 32 FAs contributing ≥1% to any of the tissues analyzed (Supplementary Tables 1–6). The total lipids extracted from the algae fed to urchins at both locations exhibited significant differences based on macroalgal species (2-way ANOVA, F = 31.52, df = 2, p < 0.001), location (F = 63.57, df = 1, p < 0.001), and the interaction between diet algae and location (F = 40.32, df = 2, p < 0.001). The most total lipid was available in Ulva tissues fed to S. droebachiensis at FHL and the least total lipid was available in Pyropia fed to S. droebachiensis as well as Pyropia and Ulva fed to S. purpuratus (Figure 1). The FA contents of algae consumed by S. droebachiensis and S. purpuratus were more similar for the Ulva fed to urchins in both experiments, with greater separation between the FA profiles of the Nereocystis and Pyropia consumed (Figure 2).
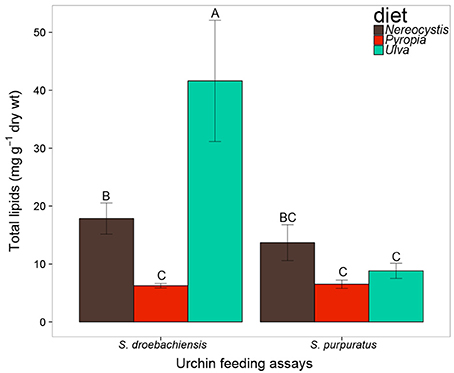
Figure 1. Total lipid (mg g−1 dry tissue weight) of macroalgae fed to S. droebachiensis and S. purpuratus. Bars with the same letter are not significantly different (2-way ANOVA, Tukey HSD, p < 0.05). Concentrations represent mean ± standard deviation, SD).
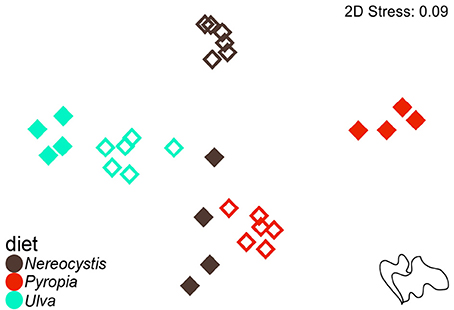
Figure 2. Non-metric multidimensional scaling (nMDS) plot of Euclidean distances between the proportions of FA (n = 37) of macroalgal monoculture diets (Nereocystis, Pyropia, or Ulva) fed to S. droebachiensis (filled symbols) and S. purpuratus (open symbols).
The total lipids in tissues of juvenile S. droebachiensis were not significantly different based on urchin diet (Table 1, 2-way ANOVA, F = 1.02, df = 2, p = 0.37) and there was no interaction between urchin diet and total lipids in tissues (F = 2.06, df = 8, p = 0.55) but there were lower levels of total lipid in the macroalgal tissues compared to the urchin tissues (F = 19.03, df = 4, p < 0.001). In contrast, the tissues of adult S. purpuratus differed in total lipid based on urchin diet (Table 1, 2-way ANOVA, F = 6.17, df = 2, p < 0.001) and tissue type tested (F = 236.77, df = 4, p < 0.001) with no interactions between these factors (F = 1.51, df = 8, p = 0.17). For S. purpuratus, tissues from urchins fed Pyropia differed from those fed Nereocystis (Tukey HSD, p < 0.001), and the total lipids in the macroalgal tissue were lower than the digesta, gut, and gonad tissues (Tukey HSD, p < 0.001), but not different from the egesta (Tukey HSD, p = 0.08). There also were no differences between the total lipids of the egesta compared to the digesta (Tukey HSD, p = 0.98) or the gut compared to the gonad (Tukey HSD, p = 0.77).
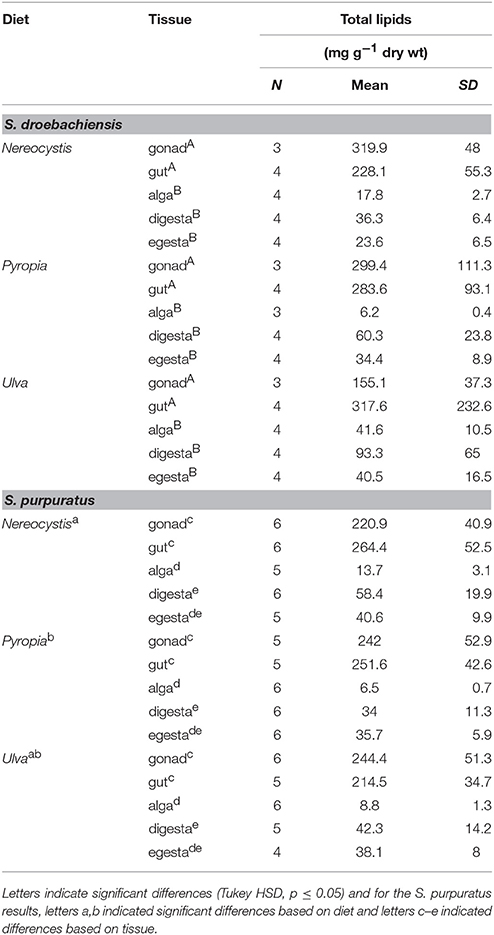
Table 1. Total lipid (mean ± SD) of tissues from S. droebachiensis and S. purpuratus feeding assays.
The multivariate FA profiles of both urchin species were significantly different for all three factors tested (urchin species, macroalgal diet, urchin tissue type) and there were significant interactions between all factors (Table 2). SIMPER analysis (Table 3) identified FAs contributing to the differences between urchin species. There were high proportions of 16:0 and 20:5ω3 in both urchin species (Table 3). However, S. droebachiensis were characterized by 16:1ω7, 18:3ω3, and 16:4ω3 while the distinguishing FAs for S. purpuratus were 18:4ω3, 18:1ω7, and 14:0. We found 20:2 Δ5,11 NMID in the urchin gut and gonad tissues of both urchin species but this FA was not detected in the algae consumed or the associated digesta and egesta (Supplementary Tables 1–6). Despite significantly different FA profiles among urchins, both exhibited similar distributional patterns with respect to the relative relationships between the urchin and macroalgal tissues (Figures 3, 4).
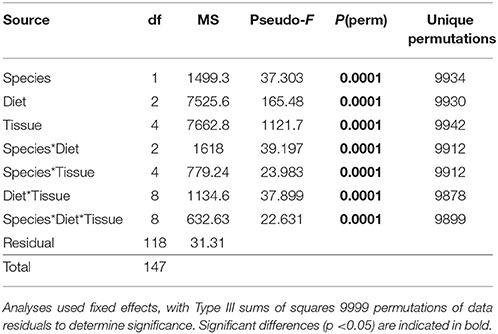
Table 2. Results of PERMANOVA analysis of proportions of identified FA contributing ≥ 1% of identified FA for S. droebachiensis and S. purpuratus (data untransformed, Euclidean distance) in a 3-way design with factors of species, diet, and tissue.
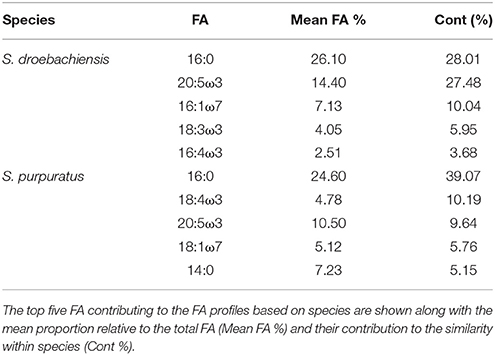
Table 3. SIMPER results for analysis of combined FA of tissues from S. droebachiensis (N = 12) and S. purpuratus (N = 18).
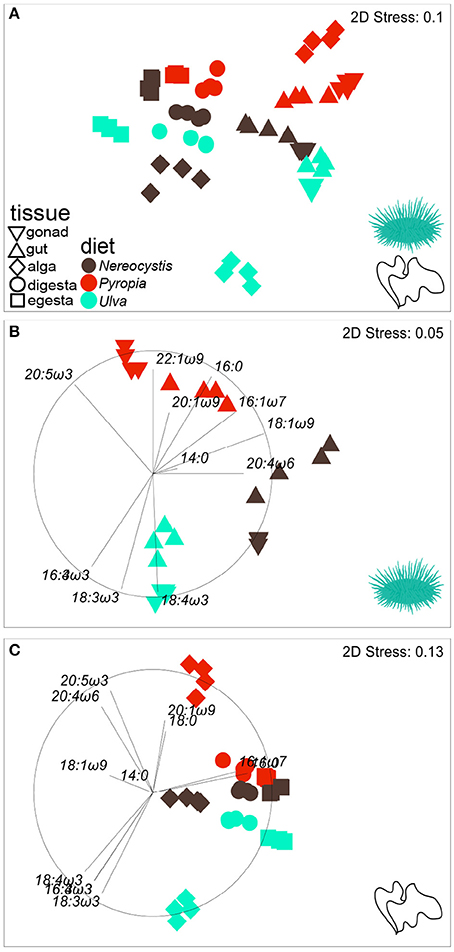
Figure 3. nMDS plot (Euclidean distance) showing the proportions of FA (n = 37) in tissues from S. droebachiensis fed monoculture diets of Nereocystis, Pyropia, or Ulva.Tissues from the feeding assay at FHL (A) are included along with subsets representing (B) urchin tissues and (C) macroalgal tissues. Vectors indicate the top five FAs identified by SIMPER analyses that distinguish FA profiles between tissues and diets (Tables 3–5, Supplementary Tables 7–11) and are included to visualize how FAs contribute to the relative distributions of (B) urchin and (C) macroalgal tissues.
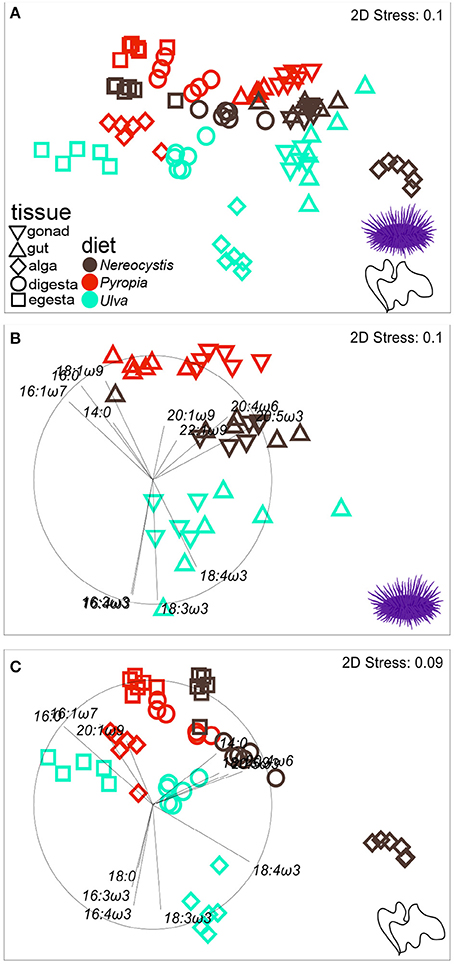
Figure 4. nMDS plot (Euclidean distance) showing proportions of FA (n = 37) in tissues from S. purpuratus fed monoculture diets of Nereocystis, Pyropia, or Ulva.Tissues from the feeding assay at OIMB (A) are included along with subsets representing (B) urchin tissues and (C) macroalgal tissues. Vectors indicate the top five FAs identified by SIMPER analyses that distinguish FA profiles between tissues and diets (Tables 3–5, Supplementary Tables 7–11) and are included to visualize how FAs contribute to the relative distributions of (B) urchin and (C) macroalgal tissues.
The gut and gonad from each species tended to group together by dietary treatment, and tissues from S. droebachiensis (Figure 3A) are grouped tighter than tissues from S. purpuratus (Figure 4A). Because there were significant differences between urchin species, we performed additional SIMPER analyses separately for S. droebachiensis and S. purpuratus to identify the FAs characterizing diets and tissues (Figures 3, 4, Tables 4, 5; Supplementary Tables 7–11). Both 16:0 and 20:5ω3 were present in high proportions in all three diets for both urchin assays (Table 4). There was more overlap between the FA profiles of S. droebachiensis and S. purpuratus fed Nereocystis compared to those fed Pyropia or Ulva (Figures 3A, 4A). The urchin and macroalgal tissues associated with the Ulva diet were separated from the other diets by 16- and 18-ω-3 PUFAs (Figures 3, 4, Table 4).
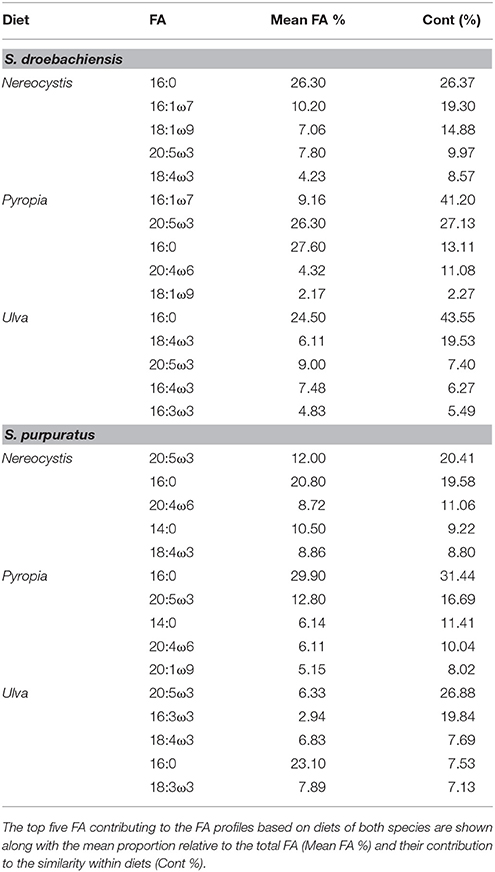
Table 4. SIMPER results for analysis of combined FA of tissues from S. droebachiensis (N = 12) and S. purpuratus (N = 18).
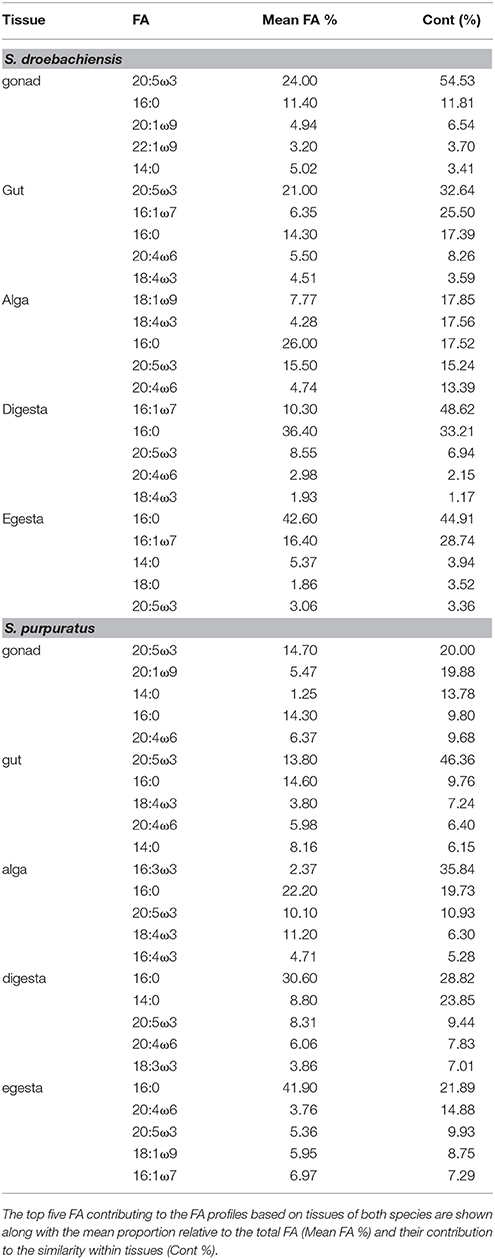
Table 5. SIMPER results for analysis of combined FA of tissues from S. droebachiensis (N = 12) and S. purpuratus (N = 18).
Urchin tissues were dominated by many of the same FAs that were prominent contributors to the Nereocystis, Pyropia, and Ulva diets, particularly the presence of 20:5ω3 (Figures 3B, 4B, Table 5), which was found in high proportions in all urchin and macroalgal tissues investigated (Table 5). The presence of 20:5ω3 and 20:1ω9 were important in distinguishing Pyropia-derived tissues from urchin tissues from other macroalgal treatments, particularly gonad, gut, as well as Pyropia-alga tissues (Figures 3, 4). The SIMPER analysis identified 18:3ω3 as a prominent component of Nereocystis tissue (Table 5), which separated Nereocystis from the other macroalgal tissues (Figures 3C, 4C).
Overall, the LCPUFAs in S. droebachiensis tissues exhibited similar trends to those observed in S. purpuratus tissues (Figure 5). The LCPUFAs in gut and gonad tissues were higher than in the macroalgal, digesta, or egesta tissues (Figure 5). There were significant differences in the concentration of LCPUFAs in the tissues from S. droebachiensis assays as a result of diet (2-way ANOVA, F = 21.70, df = 2, p < 0.001) and tissue (F = 70.83, df = 4, p < 0.001), and there was an interaction between these factors (F = 11.38, df = 8, p < 0.001). The LCPUFAs were higher (Tukey HSD, p < 0.05) in the gonad tissue of S. droebachiensis fed Nereocystis or Pyropia than all other tissues quantified (Figure 5). LCPUFA concentrations differed based on diet (2-way ANOVA, F = 12.40, df = 2, p < 0.001) and tissue (F = 174.19, df = 4, p < 0.001), and there was an interaction between factors (F = 5.62, df = 8, p < 0.001). As with S. droebachiensis, the highest concentrations of LCPUFAs were found in the gonad tissues of S. purpuratus fed Nereocystis and Pyropia (Tukey HSD, p < 0.05), however these concentrations did not differ from the gut tissue of S. purpuratus fed Nereocystis (Tukey HSD, p ≥ 0.05, Figure 5B).
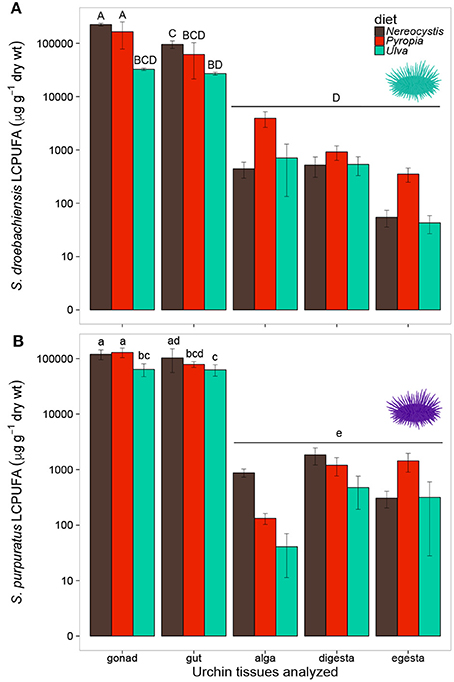
Figure 5. Concentrations (μg g−1 dry tissue weight) of long chain polyunsaturated fatty acids (LCPUFA—sum of ARA, EPA, and DHA) of tissues (gonad, gut, alga, digesta, egesta) collected from feeding assays with (A) S. droebachiensis and (B) S. purpuratus. Bars with the same letter are not significantly different (2-way ANOVA, Tukey HSD, p < 0.05). Concentrations are plotted on a logarithmic scale and error bars represent ± standard deviation, SD.
Similar to LCPUFA analysis, there were significant differences in LIN (18:2ω6), ARA (20:4ω6), ALA + SDA (18:3ω3 + 18:4ω3), and EPA (20:5ω3) based on urchin diet, tissue, and the interaction between both factors (Table 6). To visualize these relationships between the mean concentrations of precursor and resultant LCPUFAs, we plotted the concentrations of LIN against ARA (Figure 6) and concentrations of ALA + SDA against EPA (Figure 7). For all comparisons, the gonad and gut tissues of S. droebachiensis and S. purpuratus concentrations of LIN, ARA, ALA + SDA, and EPA were greater than the concentrations in the macroalgal tissues (Figures 6, 7). In S. droebachiensis, the macroalgal tissues exhibited intermediate FA concentrations of LIN and ARA relative to the digesta and egesta (lower relative concentrations) and the gut and gonad (higher relative concentrations) tissues associated with Pyropia and Ulva (Figure 6A). There is a similar pattern between the concentrations of the precursors ALA + SDA and resultant EPA in S. droebachiensis tissues (Figure 7A) with a slightly more pronounced increase in EPA in digesta along with a decrease in ALA + SDA (Figure 7A). In the S. purpuratus assays, Pyropia and Ulva macroalgal tissues had the lowest relative LIN and ARA concentrations, while Nereocystis macroalgal tissues had intermediate LIN and ARA concentrations compared to digesta and egesta tissues (Figures 6B, 7B). This same trend is also reflected in the comparison of ALA + SDA and EPA for S. purpuratus (Figure 7B). PUFAs (ARA and EPA) tended to be increased in digesta tissue and reduced in egesta compared to precursor FAs (Figures 6, 7).
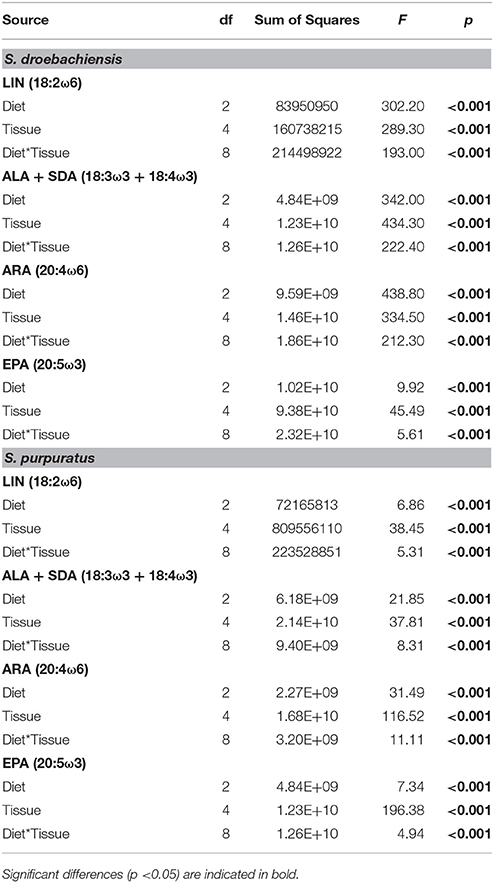
Table 6. Results from 2-way analysis of variance (ANOVA) for the concentration (μg g−1 dry tissue weight) of precursor (LIN and ALA + SDA) of the corresponding most abundant (ARA and EPA, respectively) long chain polyunsaturated fatty acids (LCPUFA) identified in tissues from S. droebachiensis and S. purpuratus assays.
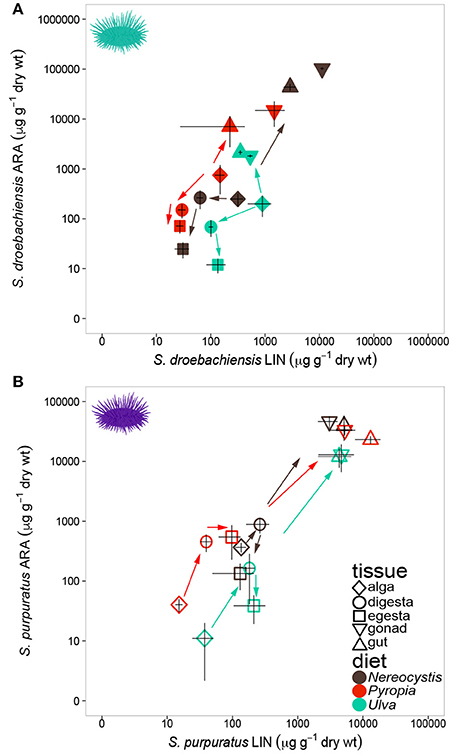
Figure 6. Concentrations (mean ± SD, μg g−1 dry weight of precursor FA 18:2ω6 (LIN) of tissues and 20:4ω6 (ARA) in tissues collected from feeding assays with (A) S. droebachiensis and (B) S. purpuratus. Arrows indicate urchin modification of diet algae as they are digested and subsequently incorporated into new urchin tissue. Concentrations are plotted on a logarithmic scale.
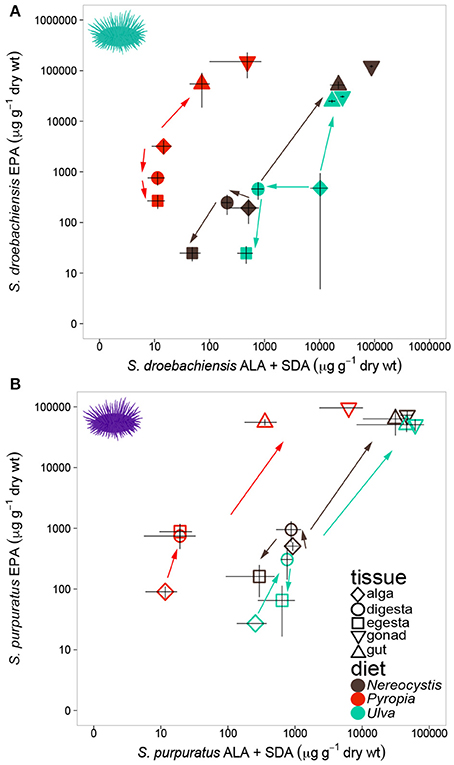
Figure 7. Concentrations (mean ± SD, μg g−1 dry weight) of precursor FAs 18:3ω3 (ALA) and 18:4ω3 (SDA) and 20:5ω3 (EPA) in tissues collected from feeding assays with (A) S. droebachiensis and (B) S. purpuratus. Arrows indicate urchin modification of diet algae as they are digested and subsequently incorporated into new urchin tissue. Concentrations are plotted on a logarithmic scale.
Discussion
We observed clear correspondences between the fatty acid composition of the diets urchins consumed and the new tissues they produced. One reason we pursued these experiments with two urchin species, and over relatively long durations, is that there have been very few controlled feeding trials with urchins fed different macroalgal diets, with mixed results reported in literature on urchin incorporation of dietary FA. Past feeding experiments with S. droebachiensis have shown, for example, that they have considerable ability to modify their dietary FA over long experiments (14 week duration; Kelly et al., 2008), or that they do not incorporate their dietary FA signal into their tissues in very short experiments (3 week duration; Wessels et al., 2012). A long (17 week) feeding experiment with adult red urchins (Mesocentrotus franciscanus) showed that urchin FAs were strongly differentiated in the gonad tissues of urchins fed two kelp species (Raymond et al., 2014). Our results show that when fed for a sufficiently long period (e.g., 25 weeks in S. droebachiensis, 10 weeks in S. purpuratus), both urchins' multivariate FA compositions are strongly affected by their diets; in fact, both species can be differentiated based on whether they were fed brown, red, and green algae (NMDS and PERMANOVA results). Interestingly, this was the case for both the gonad and gut tissues. To our knowledge, this is the first experiment that has evaluated multiple urchin tissue types in multiple urchins after controlled feeding trials with different algae.
Some differences in urchin tissue FAs may have resulted from the total lipids and FAs available in local algae fed to urchins at the two experimental sites. It is likely that macroalgal tissue consumed by urchins at FHL (inland marine waters of Washington) and OIMB (southern coastal Oregon) experienced different abiotic conditions, resulting in some of the differences in lipid and FA content observed. FA biosynthesis is subject to environmental conditions that also influence photosynthesis, including but not limited to light, temperature, nutrient, and salinity (reviewed by Galloway and Winder, 2015). Here, we observed greater similarity between the FA profiles of Ulva tissues from the two areas, with less overlap between Nereocystis and Pyropia fed to urchins in both feeding trials. Some variation between study sites may arise from our inability, without molecular identification, to guarantee that we were working with the same Pyropia and Ulva species at each study site. Despite the observed differences in lipid and FA content, there was sufficient similarity between monoculture diets of the same phylum from FHL and OIMB to support new tissue growth with significantly different FA profiles.
Remarkably, even though certain key LCPUFA content declined in egesta, the total lipid content tended to increase from macroalgal tissue to digesta to egesta, suggesting that the nutritional quality of macroalgal tissues was modified by urchin digestion. This result is consistent with other studies, using different metrics, that have shown a general increase in the nutritional value or energy content of urchin fecal material derived from macroalgae (Mamelona and Pelletier, 2005) or seagrass (Koike et al., 1987), as a consequence of microbial enrichment of the substrate. Increased lipid content in the egesta across all diets was a trend, albeit insignificant, in the juvenile S. droebachiensis experiment, and was significant with the adult S. purpuratus. Differences in the total lipid content of macroalgal tissues and the resulting digesta and egesta is likely to be influenced by the urchin gut microbiome, which is thought to play a role in carbohydrate, amino acid, and lipid metabolism (Hakim et al., 2016) and can be directly influenced by immediate culture environment (Hakim et al., 2015). It is therefore possible that some of the differences we observed in urchin digestion of Nereocystis, Pyropia, and Ulva between our experiments are driven not just by the difference in FA metabolism between urchin species, but also by variations in the local microbiota at Friday Harbor, WA and Charleston, OR. This may in part explain the consistent patterns in the relative depletion of LIN and ARA the digesta and egesta of S. droebachiensis for Pyropia and Ulva compared to increased in concentration of LIN, ARA, ALA + SDA, and EPA in the S. purpuratus assay as tissues were digested (e.g., Figures 6, 7). Local microbiota availability and retention in urchin gut tissue may have contributed to the increased ARA and EPA concentrations in digesta and subsequent depletion in egesta of Nereocystis tissues. Microbial abundance on macroalgal tissue can influence the biochemistry of macroalgae consumed (Sosik and Simenstad, 2013) and could contribute to differences in urchin gut microbiota (Hakim et al., 2015, 2016). Conspecific urchin microbiome comparisons between these regions could be an interesting area of future investigation.
Urchin tissues from each diet treatment exhibited parallel differences to those that distinguish brown, red, and green algae from one another (Galloway et al., 2012; Kelly and Scheibling, 2012). The FA profiles of both urchin species displayed elevated levels of FAs previously associated with the same macroalgal phyla, even though tissues were not characterized by all of the same FAs (Kelly and Scheibling, 2012). As a result, urchins fed the same macroalgal diet grouped more closely together in the nMDS, with the tightest groupings among S. droebachiensis fed monoculture diets from which all tissues were generated. Convergence on similar profiles between the two assays occurred despite S. purpuratus having a unquantified amount of “legacy” FAs in soft tissues from previous in situ diets. The substantial growth we quantified in both species (e.g., in wet weight) suggests that urchins rapidly generated new tissues from experimental diets. This higher abundance of food in the laboratory may have over-ridden some of the within-species or site variation we would have otherwise expected due to season or sex (Dethier et al., 2014; Zárate et al., 2016). Urchin “tissue turnover” time is still unknown, and thus the ideal experimental duration for tracking macroalgal diets into urchin tissues can be estimated using growth rate as a proxy, until future experiments quantify tissue turnover rates. However, it appears that urchin tissue turnover is slow enough that experiments of several weeks (e.g., Wessels et al., 2012) are insufficient to allow urchin tissues to incorporate the dietary FA biomarker signal. Urchins, like other animals, can elongate and modify (i.e., biosynthesize) their dietary precursor FA to achieve heightened concentrations of key PUFA (Floreto et al., 1996; Cook et al., 2000; Castell et al., 2004). For example, the urchin Psammechinus miliaris has the ability to synthesize small amounts of EPA (20:5ω3) from ALA (18:3ω3) among other PUFA modifications (Bell et al., 2001). It is presently unknown whether the patterns we observed here, resulting from very regimented and controlled diets offered in the lab, would transfer to wild urchins that are presumably integrating a range of macroalgal diets over the course of the year.
The higher concentrations of LCPUFAs observed in urchin tissues compared to macroalgal tissues highlight the urchins' ability for de novo synthesis and selective retention of some FAs (Cook et al., 2000; Bell et al., 2001). This is particularly evident in the S. droebachiensis experiment, in which juvenile urchins generated nearly all of their tissues from a monoculture diet, as compared with the S. purpuratus experiment, which started with adult urchins with significant “legacy FA” from their previous diets in nature. We documented relatively high proportions of 20:2 Δ5, 11 NMID in the gonad and gut tissue of S. droebachiensis and S. purpuratus, as high as 8.38 ± 0.83 and 9.68 ± 0.94% (gonad, gut of S. purpuratus fed Pyropia, Supplementary Table 5). González-Durán et al. (2008) documented similar proportions of 20:2 Δ5, 11 NMID in urchin gonad (5.9%) and gut (8.61%) while Castell et al. (2004) documented much higher synthesis in tissues of S. droebachiensis fed formulated diets, and demonstrated that urchins can synthesize 20:2 Δ5, 11 NMID from either 20:1ω7 or 20:1ω9. González-Durán et al. (2008) hypothesized that when there is an EFA deficiency, taxa such as echinoids may utilize NMIDs in place of some EFAs to maintain physiological functions.
S. droebachiensis and S. purpuratus also synthesized and retained LCPUFAs including ARA and EPA in gonad and gut tissues. These elevated LCPUFAs can become available to pelagic and benthic consumers upon gamete release (Hughes et al., 2011; Fuiman et al., 2015), subsidizing marine consumers. FA profiles of gonad tissue and larvae subsequently released from mature gonads share similar concentrations of these important LCPUFAs (Hughes et al., 2011). However, Hughes et al. (2011) only took FAs into account to evaluate nutritional quality. In the present study, we demonstrated that even though there were no significant differences in the FA profiles between algae consumed and egested, the urchins increased the nutritive quality of egesta in the form of increased total lipid content. This has significant implications for marine food webs, because increased lipid content in a food source is likely to benefit consumers, and suggests a mechanism for why macrophyte-derived carbon (generally) and kelp-derived carbon (more specifically) may be a valuable trophic subsidy (Sauchyn and Scheibling, 2009; Sauchyn et al., 2011; Britton-Simmons et al., 2012; Lowe et al., 2015) to benthic organisms that might not otherwise utilize these sources directly.
There are multiple factors influencing FA profiles that could explain some of the differences we documented between S. droebachiensis and S. purpuratus assays. These include using two different urchin species (Dethier et al., 2013), under different local conditions (Hughes et al., 2005; Dethier et al., 2013), and with different prior in situ diets. Additionally, the feeding assays spanned different time periods (180 vs. 70 days) and used urchins at different life history stages (Hughes et al., 2006), which likely resulted in gonad tissues in different stages of gametogenesis (Zárate et al., 2016). The gonads present in the juvenile S. droebachiensis were unlikely to be mature and more likely represent their role in nutrient storage rather than reproduction. We did not quantify sex ratios, but in some cases females and males have different FAs (Hughes et al., 2006; Zárate et al., 2016). Despite these differences, particularly between urchin species and life stage, it is nonetheless clear that growing urchins, whether from increased tissue biomass, or in gonad development, incorporated multivariate FA profiles of the algae provided. The macroalgal diets selected here are known to have strong differences in their FA; future work could investigate whether it is possible to trace the FA of more closely related algae, e.g., different species of Laminariales (e.g., Raymond et al., 2014), into these urchin species.
Our results indicate that urchin digestion of different macroalgae following maintenance on the same monoculture diets generates similar changes in total crude lipid and FA content. The FA profiles of urchin tissues differ significantly from the FA profiles of the macroalgal diets. This is expected, as animal FA profiles will never completely align with those of macroalgal diets, even if they are strongly structured by them. Additionally, neither urchin species significantly altered the LCPUFA content of macroalgae consumed. However, we did find different patterns in S. droebachiensis and S. purpuratus processing of ARA and EPA precursors that may be related to differences in gut microbiota. We found that both urchin species selectively retained EFAs that were relatively deplete in diets and may have synthesized some of these EFAs from precursors along with 20:2 Δ5, 11 NMID. The similar patterns in trophic modification we documented suggest that it is feasible to develop FA resource libraries for future use in Bayesian mixing models for further evaluating in situ urchin trophic linkages (Galloway et al., 2014). Common resource libraries representing urchin trophic modification of macroalgae make it possible to estimate the diets of a wider array of urchins. We have also demonstrated that it is possible to obtain a good estimation of urchin modification of macroalgal tissue over a relatively short time period if macroalgae are made available in quantities to support new soft tissue generation. This rapid convergence of FA profiles despite a large quantity of legacy tissue from previous diets makes the development of resource libraries for benthic consumers more feasible.
Author Contributions
JS, AG, MD, and JK: experimental conception, writing; JS and JK: sample collection, experimentation, total lipid/FA extraction; JS and AG: statistical analysis.
Conflict of Interest Statement
The authors declare that the research was conducted in the absence of any commercial or financial relationships that could be construed as a potential conflict of interest.
Acknowledgments
The authors thank Sami Taipale and Martin Kainz for their generous advice and training in FA extraction and quantification protocols. We thank David Duggins for conceptual inspiration for the work which led to this project via funding from the National Science Foundation (NSF; BIO-OCE 0925718). Work completed at the Oregon Institute of Marine biology was supported by the start-up fund awarded to AG by the University of Oregon. We thank the Director of the Friday Harbor Labs for use of facilities; C. Feehan and B. Grauman-Boss for providing the tiny green urchins; D. Yiu for careful urchin husbandry.
Supplementary Material
The Supplementary Material for this article can be found online at: https://www.frontiersin.org/articles/10.3389/fevo.2018.00083/full#supplementary-material
References
Anderson, M., Gorley, R. N., and Clarke, R. K. (2008). PERMANOVA+ for PRIMER: Guide to Software and Statistical Methods (Plymouth: PRIMER-E Ltd).
Andrew, N., Agatsuma, Y., Ballesteros, E., Bazhin, A., Creaser, E., Barnes, D., et al. (2003). Status and management of world sea urchin fisheries. Oceanogr. Mar. Biol. Annu. Rev. 40, 343–425. doi: 10.1201/9780203180594.ch7
Arts, M. T., Ackman, R. G., and Holub, B. J. (2001). “Essential fatty acids” in aquatic ecosystems: a crucial link between diet and human health and evolution. Can. J. Fish. Aquat. Sci. 58, 122–137. doi: 10.1139/cjfas-58-1-122
Arts, M. T., Brett, M. T., and Kainz, M. J. (2009). Lipids in Aquatic Ecosystems. New York, NY: Springer.
Bell, J. G., and Sargent, J. R. (2003). Arachidonic acid in aquaculture feeds: current status and future opportunities. Aquaculture 218, 491–499. doi: 10.1016/S0044-8486(02)00370-8
Bell, M. V., Dick, J. R., and Kelly, M. S. (2001). Biosynthesis of eicosapentaenoic acid in the sea urchin Psammechinus miliaris. Lipids 36, 79–82. doi: 10.1007/s11745-001-0671-2
Brett, M. T., Eisenlord, M. E., and Galloway, A. W. E. (2016). Using multiple tracers and directly accounting for trophic modification improves dietary mixing-model performance. Ecosphere 7:e01440. doi: 10.1002/ecs2.1440.
Britton-Simmons, K. H., Rhoades, A. L., Pacunski, R. E., Galloway, A. W. E., Lowe, A. T., Sosik, E. A., et al. (2012). Habitat and bathymetry influence the landscape-scale distribution and abundance of drift macrophytes and associated invertebrates. Limnol. Oceanogr. 57, 176–184. doi: 10.4319/lo.2012.57.1.0176
Castell, J. D., Kennedy, E. J., Robinson, S. M. C., Parsons, G. J., Blair, T. J., and Gonzalez-Duran, E. (2004). Effect of dietary lipids on fatty acid composition and metabolism in juvenile green sea urchins (Strongylocentrotus droebachiensis). Aquaculture 242, 417–435. doi: 10.1016/j.aquaculture.2003.11.003
Christie, W. W., and Han, X. (2010). Lipid Analysis-Isolation, Separation, Identification and Lipidomic Analysis. Bridgwater: Oily Press.
Cook, E. J., Bell, M. V., Black, K. D., and Kelly, M. S. (2000). Fatty acid compositions of gonadal material and diets of the sea urchin, Psammechinus miliaris: trophic and nutritional implications. J. Exp. Mar. Biol. Ecol. 255, 261–274. doi: 10.1016/S0022-0981(00)00301-4
Cook, E. J., Hughes, A. D., Orr, H., Kelly, M. S., and Black, K. D. (2007). Influence of dietary protein on essential fatty acids in the gonadal tissue of the sea urchins Psammechinus miliaris and Paracentrotus lividus (Echinodermata). Aquaculture 273, 586–594. doi: 10.1016/j.aquaculture.2007.10.032
Dalsgaard, J., St. John, M., Kattner, G., Müller-Navarra, D., and Hagen, W. (2003). Fatty acid trophic markers in the pelagic marine environment. Adv. Mar. Biol. 46, 225–340. doi: 10.1016/S0065-2881(03)46005-7
Dethier, M. N., Brown, A., Burgess, S., Eisenlord, M. E., Galloway, A. W. E., Kimber, J., et al. (2014). Degrading detritus: changes in food quality of aging kelp tissue varies with species. J. Exp. Mar. Biol. Ecol. 460, 72–79. doi: 10.1016/j.jembe.2014.06.010
Dethier, M. N., Sosik, E., Galloway, A. W., Duggins, D. O., and Simenstad, C. A. (2013). Addressing assumptions: variation in stable isotopes and fatty acids of marine macrophytes can confound conclusions of food web studies. Mar. Ecol. Prog. Ser. 478, 1–14. doi: 10.3354/meps10310
Floreto, E. A. T., Teshima, S., and Ishikawa, M. (1996). The effects of seaweed diets on the growth, lipid and fatty acids of juveniles of the white sea urchin Tripneustes gratilla. Fish. Sci. 62, 589–593.
Fuiman, L. A., Connelly, T. L., Lowerre-Barbieri, S. K., and McClelland, J. W. (2015). Egg boons: central components of marine fatty acid food webs. Ecology 96, 362–372. doi: 10.1890/14-0571.1
Galloway, A. W., Britton-Simmons, K. H., Duggins, D. O., Gabrielson, P. W., and Brett, M. T. (2012). Fatty acid signatures differentiate marine macrophytes at ordinal and family ranks. J. Phycol. 48, 956–965. doi: 10.1111/j.1529-8817.2012.01173.x
Galloway, A. W., and Winder, M. (2015). Partitioning the relative importance of phylogeny and environmental conditions on phytoplankton fatty acids. PLoS ONE 10:e0130053. doi: 10.1371/journal.pone.0130053
Galloway, A. W. E., Eisenlord, M. E., Dethier, M. N., Holtgrieve, G. W., and Brett, M. T. (2014). Quantitative estimates of isopod resource utilization using a Bayesian fatty acid mixing model. Mar. Ecol. Prog. Ser. 507, 219–232. doi: 10.3354/meps10860
Galloway, A. W. E., Lowe, A. T., Sosik, E. A., Yeung, J. S., and Duggins, D. O. (2013). Fatty acid and stable isotope biomarkers suggest microbe-induced differences in benthic food webs between depths. Limnol. Oceanogr. 58, 1451–1462. doi: 10.4319/lo.2013.58.4.1451
González-Durán, E., Castell, J. D., Robinson, S. M., and Blair, T. J. (2008). Effects of dietary lipids on the fatty acid composition and lipid metabolism of the green sea urchin Strongylocentrotus droebachiensis. Aquaculture 276, 120–129. doi: 10.1016/j.aquaculture.2008.01.010
Hakim, J. A., Koo, H., Dennis, L. N., Kumar, R., Ptacek, T., Morrow, C. D., et al. (2015). An abundance of Epsilonproteobacteria revealed in the gut microbiome of the laboratory cultured sea urchin, Lytechinus variegatus. Front. Microbiol. 6:1047. doi: 10.3389/fmicb.2015.01047
Hakim, J. A., Koo, H., Kumar, R., Lefkowitz, E. J., Morrow, C. D., Powell, M. L., et al. (2016). The gut microbiome of the sea urchin, Lytechinus variegatus, from its natural habitat demonstrates selective attributes of microbial taxa and predictive metabolic profiles. FEMS Microbiol. Ecol. 92:fiw146. doi: 10.1093/femsec/fiw146
Hughes, A. D., Catarino, A. I., Kelly, M. S., Barnes, D. K., and Black, K. D. (2005). Gonad fatty acids and trophic interactions of the echinoid Psammechinus miliaris. Mar. Ecol. Prog. Ser. 305, 101–111. doi: 10.3354/meps305101
Hughes, A. D., Cook, E. J., Orr, H., Kelly, M. S., and Black, K. D. (2011). The transformation of long chain polyunsaturated fatty acids in benthic food webs: the role of sea urchins. J. Exp. Mar. Biol. Ecol. 409, 229–234. doi: 10.1016/j.jembe.2011.08.027
Hughes, A. D., Kelly, M. S., Barnes, D. K., Catarino, A. I., and Black, K. D. (2006). The dual functions of sea urchin gonads are reflected in the temporal variations of their biochemistry. Mar. Biol. 148, 789–798. doi: 10.1007/s00227-005-0124-0
Kainz, M. J., Hager, H. H., Rasconi, S., Kahilainen, K. K., Amundsen, P.-A., and Hayden, B. (2017). Polyunsaturated fatty acids in fishes increase with total lipids irrespective of feeding sources and trophic position. Ecosphere 8:e01753. doi: 10.1002/ecs2.1753
Kelly, J. R., and Scheibling, R. E. (2012). Fatty acids as dietary tracers in benthic food webs. Mar. Ecol. Prog. Ser. 446, 1–22. doi: 10.3354/meps09559
Kelly, J. R., Scheibling, R. E., Iverson, S. J., and Gagnon, P. (2008). Fatty acid profiles in the gonads of the sea urchin Strongylocentrotus droebachiensis on natural algal diets. Mar. Ecol. Prog. Ser. 373, 1–9. doi: 10.3354/meps07746
Koike, I., Mukai, H., and Nojima, S. (1987). The role of the sea urchin, Tripneustes gratilla (Linnaeus), in decomposition and nutrient cycling in a tropical seagrass bed. Ecol. Res. 2, 19–29. doi: 10.1007/BF02348616
Lowe, A. T., Whippo, R., Galloway, A. W. E., Britton-Simmons, K. H., and Dethier, M. N. (2015). Sedentary urchins influence benthic community composition below the macroalgal zone. Mar. Ecol. 36, 129–140. doi: 10.1111/maec.12124
Mamelona, J., and Pelletier, E. (2005). Green urchin as a significant source of fecal particulate organic matter within nearshore benthic ecosystems. J. Exp. Mar. Biol. Ecol. 314, 163–174. doi: 10.1016/j.jembe.2004.08.026
Müller-Navarra, D. C. (2008). Food web paradigms: the biochemical view on trophic interactions. Int. Rev. Hydrobiol. 93, 489–505. doi: 10.1002/iroh.200711046
Parrish, C. C. (1999). “Determination of total lipid, lipid classes, and fatty acids in aquatic samples,” in Lipids in Freshwater Ecosystems, eds M. T. Arts, B. C. Wainman (New York, NY: Springer), 4–20.
Pearse, J. S. (2006). Ecological role of purple sea urchins. Science 314, 940–941. doi: 10.1126/science.1131888
Raymond, W. W., Lowe, A. T., and Galloway, A. W. E. (2014). Degradation state of algal diets affects fatty acid composition but not size of red urchin gonads. Mar. Ecol. Prog. Ser. 509, 213–225. doi: 10.3354/meps10888
R Core Team (2015). A Language and Environment for Statistical Computing. Vienna: R Foundation for Statistical Computing. Available online at: http://www.R-project.org/
Sargent, J., Bell, G., McEvoy, L., Tocher, D., and Estevez, A. (1999). Recent developments in the essential fatty acid nutrition of fish. Aquaculture 177, 191–199.
Sauchyn, L. K., Lauzon-Guay, J. S., and Scheibling, R. E. (2011). Sea urchin fecal production and accumulation in a rocky subtidal ecosystem. Aquat. Biol. 13, 215–223. doi: 10.3354/ab00359
Sauchyn, L. K., and Scheibling, R. E. (2009). Degradation of sea urchin feces in a rocky subtidal ecosystem: implications for nutrient cycling and energy flow. Aquat. Biol. 6, 99–108. doi: 10.3354/ab00171
Sosik, E. A., and Simenstad, C. A. (2013). Isotopic evidence and consequences of the role of microbes in macroalgae detritus-based food webs. Mar. Ecol. Prog. Ser. 494, 107–119. doi: 10.3354/meps10544
Steneck, R. S., Graham, M. H., Bourque, B. J., Corbett, D., Erlandson, J. M., Estes, J. A., et al. (2002). Kelp forest ecosystems: biodiversity, stability, resilience and future. Environ. Conserv. 29, 436–459. doi: 10.1017/S0376892902000322
Taipale, S. J., Hiltunen, M., Vuorio, K., and Peltomaa, E. (2016). Suitability of phytosterols alongside fatty acids as chemotaxonomic biomarkers for phytoplankton. Front. Plant Sci. 7:212. doi: 10.3389/fpls.2016.00212
Taipale, S., Strandberg, U., Peltomaa, E., Galloway, A. W. E., Ojala, A., and Brett, M. T. (2013). Fatty acid composition as biomarkers of freshwater microalgae: analysis of 37 strains of microalgae in 22 genera and in seven classes. Aquat. Microb. Ecol. 71, 165–178. doi: 10.3354/ame01671
Takagi, T., Eaton, C. A., and Ackman, R. G. (1980). Distribution of fatty acids in lipids of the common Atlantic sea urchin Strongylocentrotus droebachiensis. Can. J. Fish. Aquat. Sci. 37, 195–202. doi: 10.1139/f80-025.
Vadas, R. L. (1977). Preferential feeding: an optimization strategy in sea urchins. Ecol. Monogr. 47, 337–371.
Wacker, A., and Von Elert, E. (2004). Food quality controls egg quality of the zebra mussel Dreissena polymorpha: the role of fatty acids. Limnol. Oceanogr. 49, 1794–1801. doi: 10.4319/lo.2004.49.5.1794
Wessels, H., Karsten, U., Wiencke, C., and Hagen, W. (2012). On the potential of fatty acids as trophic markers in Arctic grazers: feeding experiments with sea urchins and amphipods fed nine diets of macroalgae. Polar Biol. 35, 555–565. doi: 10.1007/s00300-011-1101-3
Winder, M., Carstensen, J., Galloway, A. W. E., Jakobsen, H. H., and Cloern, J. E. (2017). The land-sea interface: a source of high-quality phytoplankton to support secondary production. Limnol. Oceanogr. 62, S258–S271. doi: 10.1002/lno.10650
Keywords: fatty acids, biomarkers, trophic ecology, egesta, macroalgae, trophic modification, PUFA
Citation: Schram JB, Kobelt JN, Dethier MN and Galloway AWE (2018) Trophic Transfer of Macroalgal Fatty Acids in Two Urchin Species: Digestion, Egestion, and Tissue Building. Front. Ecol. Evol. 6:83. doi: 10.3389/fevo.2018.00083
Received: 28 February 2018; Accepted: 29 May 2018;
Published: 19 June 2018.
Edited by:
Liliane Ruess, Humboldt-Universität zu Berlin, GermanyReviewed by:
Mickie Lynn Powell, University of Alabama at Birmingham, United StatesDavid Alonso, Consejo Superior de Investigaciones Científicas (CSIC), Spain
Copyright © 2018 Schram, Kobelt, Dethier and Galloway. This is an open-access article distributed under the terms of the Creative Commons Attribution License (CC BY). The use, distribution or reproduction in other forums is permitted, provided the original author(s) and the copyright owner are credited and that the original publication in this journal is cited, in accordance with accepted academic practice. No use, distribution or reproduction is permitted which does not comply with these terms.
*Correspondence: Julie B. Schram, anNjaHJhbUB1b3JlZ29uLmVkdQ==
†Present Address: Julia N. Kobelt, Department of Biology, Montclair State University, Montclair, NJ, United States