- 1Environmental Sustainability Research Centre, Brock University, St. Catharines, ON, Canada
- 2Department of Geography and Tourism Studies, Brock University, St. Catharines, ON, Canada
Previous research suggests climate warming during the current century is likely to lead to an increase in the frequency and severity of wildfire. Recent wildfire seasons in northern Canada generally support these studies, with some of the worst fire seasons on record occurring during the past decade. While we can readily track the spatial and temporal distribution of these events during recent decades using satellite-derived data, these records of past fire activity are relatively short. Proxy records of past fire activity are needed to fully understand how fire regimes may be shifting in response to changing climatic conditions. A high-resolution fire record for the full Holocene was developed using a 539.5-cm sediment core collected from a small lake in southwest Yukon Territory, Canada. Macroscopic charcoal was counted throughout the core at contiguous 0.5-cm intervals. The core was also analyzed for loss-on-ignition and magnetic susceptibility. Fossil pollen preserved in the lake sediment was analyzed to determine vegetation change throughout the Holocene. Macroscopic charcoal analysis indicates an active fire history throughout the record, with 91 fires recorded during the Holocene. Results suggest the fire regime in this region responds to both top-down (climate) and bottom-up (vegetation) factors. Fire return intervals changed in response to shifts in precipitation and temperature as well as the expansion of lodgepole pine into the region. The shifts in precipitation and temperature were attributed to the oscillation of the Aleutian Low pressure system and fluctuations in climate associated with the Medieval Climate Anomaly and the Little Ice Age.
Introduction
The circumpolar boreal forest is the largest forest ecosystem on the globe, comprising 17% of the earth's terrestrial surface (Stocks, 2004). These forests are valuable both economically and as part of the global carbon cycle, storing as much as 30% of global terrestrial carbon.
Fire is one of the most important disturbance factors in the boreal forest (Johnson, 1992; Payette, 1992), shaping the forest in terms of age, composition, and diversity (Weber and Stocks, 1998). Recent studies by Johnstone and Chapin (2006) and Johnstone et al. (2010) indicate that contemporary fire activity in Yukon is altering vegetation assemblages and changing the composition of some forest ecosystems from conifer-dominated systems to forests dominated by deciduous species. Such changes will lead to feedbacks in these systems as fuel loads and flammability of those fuels change.
Fire models suggest that the frequency and severity of forest fires across the boreal biome will likely increase during the current century as global temperatures continue to rise (Flannigan et al., 2005, 2009; Spracklen et al., 2009). In the Yukon specifically, temperatures have increased 2°C over the last 50 years, with an additional increase of 2°C projected over the next 50 years (Streicker, 2016). In addition to the rising temperatures, other changes in climate are also being observed. Lightning ignitions represent the majority of the area burned across the boreal forest and lightning ignitions have been increasing since 1975 (Veraverbeke et al., 2017). Veraverbeke et al. (2017) found that a record number of lightning ignitions coincided with increased fire activity in the Northwest Territories in 2014 and Alaska in 2015. Similarly, the large fire year of 2004 in Yukon was attributed to unusual lightning activity (Yukon Wildland Fire Management., 2005). Additional information about the long-term occurrence of wildfire across the boreal biome is needed to refine predictions and improve planning and management of these forests during a period of rapid climatic and environmental change.
Wildfire has an important role in shaping the boreal ecosystem throughout northwest Canada, however records of past fire occurrence and severity are geographically sparse and temporally short. Records of past wildfire occurrence in Yukon extend back to AD 1946 (Weber and Stocks, 1998). Therefore, it is difficult to assess natural fire regimes and develop management policies based on these temporally-short wildfire records when fire return intervals in the boreal forest typically range from 50 to >400 years (Bourgeau-Chavez et al., 2000; MacDonald, 2003; Stocks, 2004). Although archived fire data is lacking in Yukon, fire reconstruction studies using macroscopic charcoal found in lake sediments can extend the temporal coverage of past wildfire activity. Lakes are ideal reservoirs of ecological information as they often persist on the landscape for millennia and accumulate material from both the terrestrial and aquatic environments (Smol, 2009). However, there have been few paleolimnological studies focused on past wildfire activity in Yukon that have been undertaken at high temporal-resolution in combination with well-dated sequences (Gajewski et al., 2014). Detailed analyses are needed to elucidate whether the frequency of wildfire and the range of natural variability are changing as the global climate system continues to warm.
Here, we reconstruct fire history from a small kettle lake in southwest Yukon. More specifically, we aim to identify the natural range of variability of fire frequency in this region as well as associated drivers. Results of this study will (1) establish the long-term fire history for the area in terms of total number of fires during the Holocene; (2) determine the mean fire return interval (mFRI) for the Holocene; (3) determine if vegetation change, such as the arrival of lodgepole pine (Pinus contorta), altered fire frequency in the past; and (4) examine the major climatic drivers of fire in the area and their impact on fire regimes. Results of this research will provide forest managers with a detailed, long-term record of past wildfire activity for southwest Yukon, which will be useful to help assess risk more accurately as well as for fire modeling and prediction.
Study Site
Physical Environment
Spindly Pine Lake (unofficial name) is a kettle lake with a maximum depth of at least 8 m and a surface area of 1.12 ha (Figures 1A–C). The lake is located ~60 km south of Whitehorse, Yukon (60°18′6.03″N, 134°59′40.33″W; 787 m a.s.l.) and lies in the glaciated area of the southwest Yukon (Scudder, 1997). The lake was formed as a result of retreating glaciers from the Cordilleran ice sheet. Spindly Pine Lake is a closed basin, lacking both inflowing and outflowing streams (Figure 1).
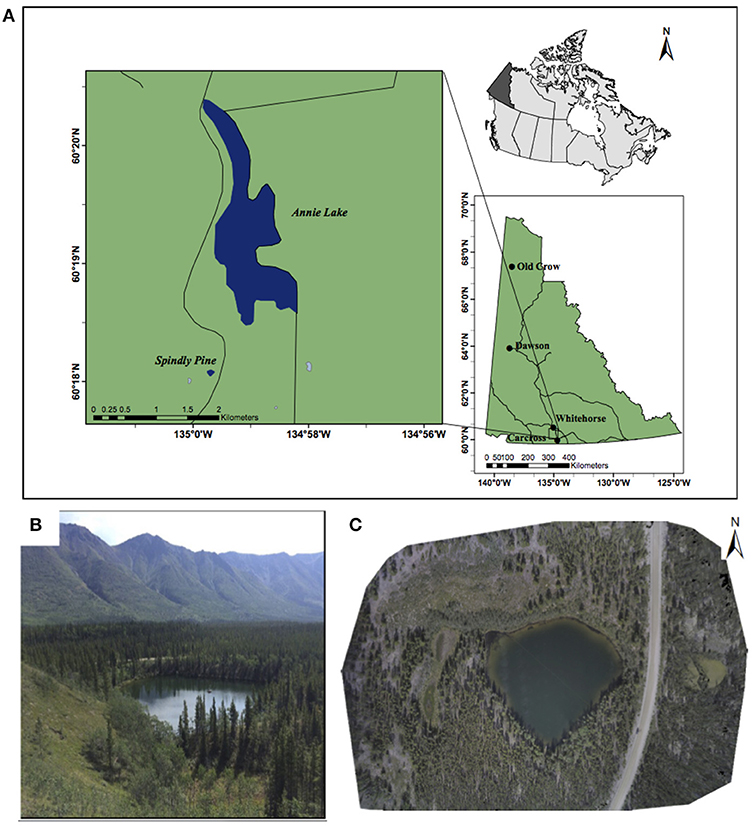
Figure 1. (A) Location of Spindly Pine Lake, Yukon. (B) Low-oblique photograph of Spindly Pine Lake showing the closed basin and lack of inflowing/outflowing streams; (C) Photograph mosaic of the Spindly Pine lake catchment, acquired using an unmanned aerial vehicle.
Climate
Spindly Pine is located within the southern lakes ecoregion of the Boreal Cordillera ecozone. The arid climate of this ecoregion is controlled by the rain shadow of the St. Elias coastal mountains (Smith et al., 2004), which create a dry and cool climate. Precipitation typically ranges from 200 to 325 mm per year, with ~30–50 percent occurring in the summer. Snow can fall at anytime during the year, but snow cover generally persists from late October until mid-April. The average annual temperature is −2°C. Winters are cold with average January temperatures of −21°C and summers are short and cool (average July temperature of 12–14°C) (Smith et al., 2004). However, the past few years (2012–2016) have been warmer with an average annual temperature of 1.1°C (Government of Canada., 2017).
Atmospheric pressure systems typically move across Yukon from west to east. The Aleutian Low pressure system (AL) impacts climate in Yukon as it oscillates between an eastern and western position (Trenberth and Hurrell, 1994; Spooner et al., 2003; Anderson et al., 2005). When the AL intensifies, it shifts to the east bringing warm, moist air over the coastal regions of western North America in a meridional pattern (Mock et al., 1998). The strong southerly winds associated with the AL pressure system move up the coast bringing intense precipitation for these coastal areas. However, due to the rain shadow effect of the St. Elias Mountains, the interior of Yukon experiences dry conditions with limited precipitation. When the AL weakens, the area of low-pressure shifts to the west over the north Pacific Ocean and a zonal flow pattern develops. This zonal flow pattern brings moist air across the mountain barriers and into the interior of Yukon (Anderson et al., 2005). Therefore, a strong AL positioned over the northeast Pacific Ocean is often associated with low precipitation and dry conditions in southern Yukon, while a weak AL positioned over the northwest Pacific Ocean is associated with a zonal flow pattern and increased precipitation in the interior of Yukon.
Glacial History and Geologic Setting
The Yukon Southern Lakes ecoregion is overlain with glacial till, glaciofluvial gravels and glaciolacustrine clay and silt deposits from the McConnell glaciation (Smith et al., 2004). The McConnell glaciation covered this region between 26,000 and 10,000 years ago (Jackson et al., 1991). The bedrock in the area is mainly coarse-grained metamorphoric and granitic rock. Dominant soils are alkaline eutric brunisols, and are typically sandy and well-drained. In exposed cutbanks throughout southern Yukon, a 2–5 cm thick layer of tephra is often visible (Smith et al., 2004). This tephra is the White River Ash (WRA) from Mount Churchill and was deposited ~1,170 year BP (Davies et al., 2016).
Vegetation
The main conifers growing in this region include white spruce (Picea glauca), black spruce (Picea mariana), lodgepole pine (Pinus contorta) and subalpine fir (Abies lasiocarpa) at higher elevations (Smith et al., 2004). Lodgepole pine is the dominant tree species as it regenerates quickly after fire. Common hardwoods include Alaska birch (Betula spp.) and trembling aspen (Populus tremuloides) with understory shrubs of alder (Alnus crispa), willow (Salix spp.), and shrub birch (Betula glandulosa). Spindly Pine Lake is primarily surrounded by lodgepole pine, with white spruce also being abundant around the lake. Black spruce is absent. A stand of aspen is also present on a steeper south-facing slope of the lake close to the water's edge (Figure 1C).
Methodology
A continuous sediment record from the sediment-water interface down to 5.4 m below the sediment water interface was collected from Spindly Pine Lake. Sediment cores were collected from the lake center (Courtney Courtney Mustaphi et al., 2015). The watery surface sediments were collected undisturbed using a Glew gravity coring system with internal diameter of 7.6 cm (Glew et al., 2001). Deeper sediments were collected using a modified Livingstone piston core with an internal diameter of 5 cm (Wright et al., 1984). The piston core overlapped the gravity core to ensure a continuous record. The surface core was collected in August 2016 and the piston core in April 2006. Piston cores were extruded horizontally in the field, wrapped in cellophane and aluminum foil and stored at ~4°C until they were analyzed. The surficial sediment core measured 48 cm in length and was subsampled in the field at 0.5 cm intervals.
The timing of the last fire around “Spindly Pine Lake” was estimated based on the age of trees within the catchment. Tree cores were collected in August 2016 from 1 white spruce and 21 lodgepole pine trees surrounding the lake. Cores were collected with a Haglof increment borer with an internal diameter of 4.3 mm. The samples were collected just above the root collar to ensure an accurate date of establishment for each tree. A total of 22 trees were sampled and aged using standard dendrochronological techniques (Speer, 2010). Cores were mounted on wood holders, sanded with progressively finer grit sandpapers and visually cross-dated. Dated samples were grouped into 5-year bins to determine the timing of the most recent stand establishing fire.
Laboratory Methods
Piston cores were split lengthwise and sub-sampled at contiguous 0.5 cm intervals. Suitable samples for radiocarbon dating (macrofossils and bulk sediment) were removed and sent to the A.E. Lalonde AMS Laboratory at the University of Ottawa, Ottawa, Canada for 14C dating using accelerator mass spectrometry (AMS). Ten 14C dates, calibrated using IntCal13 (Reimer et al., 2013) and the White River ash deposit, were utilized to develop the age-depth model using the R Studio package Bacon V 2.2 (Blaauw and Christen, 2013). For depths throughout the core that were lacking macrofossils suitable for dating, bulk sediment samples were used instead. However, bulk sediment can often provide incorrect age estimates due to the freshwater reservoir effect (Patterson et al., 2017). To account for old carbon offsets associated with these samples, a bulk sediment sample was dated directly after the WRA to determine the offset between the bulk sediment age and the known age of the WRA deposit. The calculated offset was then applied to subsequent bulk samples to provide more realistic age estimates for the bulk sediment dates (Patterson et al., 2017). However, it is acknowledged that the offset may change throughout the core.
Given the time between collection of the Livingstone cores and their analysis, care was taken to ensure that any changes in core length were accounted for. Extruded cores were measured in the field at the time of collection and marked on the cellophane and aluminum foil at that time. At the time of subsampling in 2016, these measurements were checked again and verified to ensure the Livingstone core segments had not decreased in length due to dewatering. In all instances, except for the very top Livingstone core segment, there was no change in core length and appearance. In the topmost segment, core length did not change according to our measurements, but rather the core top decreased in volume and became somewhat tapered. This was confirmed with the examination of macroscopic charcoal, which indicated three peaks that were identifiable in both the piston and surface sediment cores (Figure S1). The three peaks in the surface core occurred at ~37, 41, and 43 cm, respectively. These same three peaks are noted in the Livingstone piston core at 10, 14, and 16 cm, respectively. The spacing between these peaks (~6 cm in both cores) was identical, indicating the Livingstone piston core had not decreased in length. The remaining Livingstone cores at greater depth showed no similar changes in volume that were noted in the uppermost section and their lengths at the time of subsampling matched those that were recorded in the field at the time of core collection.
A multi-proxy approach was used for this study to determine the timing of past fire events. Macroscopic charcoal, loss-on-ignition (LOI), magnetic susceptibility (MS), and tree cores were used to build a record of fire activity, while pollen analysis was used to determine past changes in vegetation. LOI and MS analyses were conducted on both cores at 0.5 cm resolution. LOI was conducted on 1 cm3 subsamples using the methods of Heiri et al. (2001). Magnetic susceptibility was measured using a Bartington MS3 magnetic susceptibility system and a MS2B sensor. The overlap between the Glew gravity core and the Livingston piston cores was determined using LOI, MS and the macroscopic charcoal profiles.
Analysis of subfossil pollen was conducted every 25 cm throughout the combined sediment record following the methods of Faegri et al. (1989). Two Lycopodium spore tablets of known quantity (Batch No. 483216; χ = 18,582 spores per tablet ± 3,820) were added to every 1 cm3 sediment sample before the chemical processing to enable calculation of pollen and stomata concentrations (Stockmarr, 1971). The sediment matrix was digested using standard acid digestion for palynological studies, including 10% hydrochloric acid, 10% potassium hydroxide, 50% hydrofluoric acid and acetolysis. Sediments were also fine sieved to remove clay and silt particles using a 7 μm Nitex® cloth (Cwynar et al., 1979). Coarse sieving was not performed on any of the samples to ensure subfossil stomata were not removed (Pisaric et al., 2003). A minimum of 300 pollen grains was counted for each sample at 400 × magnification using a Nikon Eclipse 80i microscope. Pollen identifications were made based on a modern reference collection at the Brock University Water and Environment Laboratory and several published keys (McAndrews et al., 1973; Bassett et al., 1978; Faegri et al., 1989; Moore et al., 1991). For this study, shrub birch and tree birch and white spruce and black spruce were combined in the pollen counts. Stomata were counted for each sample and identified using the key developed by Hansen (1995). Stomata were enumerated on the same slides used for pollen identification. Stomata data are presented as presence/absence data. TILIA v2.0.41 was used to generate the pollen diagrams and CONISS was used to conduct a constrained cluster analysis to determine the pollen zones (Grimm, 1987).
Macroscopic charcoal analysis was conducted at contiguous 0.5 cm intervals using 1 cm3 subsamples. The charcoal preparation and analysis followed the wet sieving method and included deflocculating and bleaching the samples for at least 24 h before being washed through a 150 μm mesh sieve (Whitlock and Larsen, 2001; Schlachter and Horn, 2010). The remaining material was collected in a petri dish and enumerated. Charcoal morphology was also recorded based on categories derived in (Courtney Mustaphi and Pisaric, 2014).
The peak detection of macroscopic charcoal was analyzed using the software package CHARAnalysis verison 1.1 (Higuera, 2009). CHAR data were resampled to a median sampling interval of 10 years based on sedimentation rates from the Bacon output. CHARAnalysis identified and separated the slowly varying background CHAR (bCHAR) from local peaks (CPeaks) that represent fire events (Higuera et al., 2010). The bCHAR was found using a robust LOWESS smoothing filter, with a 500-years window. The CPeaks were defined locally using the non-transformed, residual model (NR), where the bCHAR was subtracted from the resampled CHAR. The threshold applied was a Gaussian mixture model with a 99th-percentile noise cut off (Higuera et al., 2010). The minimum count analysis was set to 0.05 indicating that the charcoal 75 years before a peak needed < 5% probability of coming from the same Poisson distribution to be considered a statistically significant peak (Higuera, 2009). A smoothed window of 1,000 years was then used to determine fire frequency based on the CPeak within the 1000-year periods. A signal-to-noise index (SNI) was used to determine the suitability of the record for peak detection analysis. The SNI is a way to quantify the separation between the large peaks and the slowly varying background noise. Kelly et al. (2011) suggest that a charcoal record with a SNI > 3 is suitable for peak detection analysis.
Results
The piston and gravity cores were matched by comparing several different proxies between the separate cores to determine the depth of overlap in order create a continuous record (Figure S1). The macroscopic charcoal records from the short gravity core and the first drive of the piston core show a clear overlap. The top of the piston core aligns with a depth of 27.5 cm in the gravity core based on the charcoal data (see Supplementary Material). The overlap between the gravity core and the piston cores resulted in a total adjusted core length of 539.5 cm.
Chronology
Results from AMS radiocarbon dating on 10 samples are summarized in Table 1. These dates and the accepted age of the WRA were used to build the age-depth model (Figures 2A–D). The base of the Spindly Pine Lake sediment core was deposited at ~12,450 year BP. The core top, representing the sediment-water interface, was intact and preserved during core collection. Therefore, the Spindly Pine Lake sediment core provides a continuous record of the full Holocene epoch in southern Yukon.
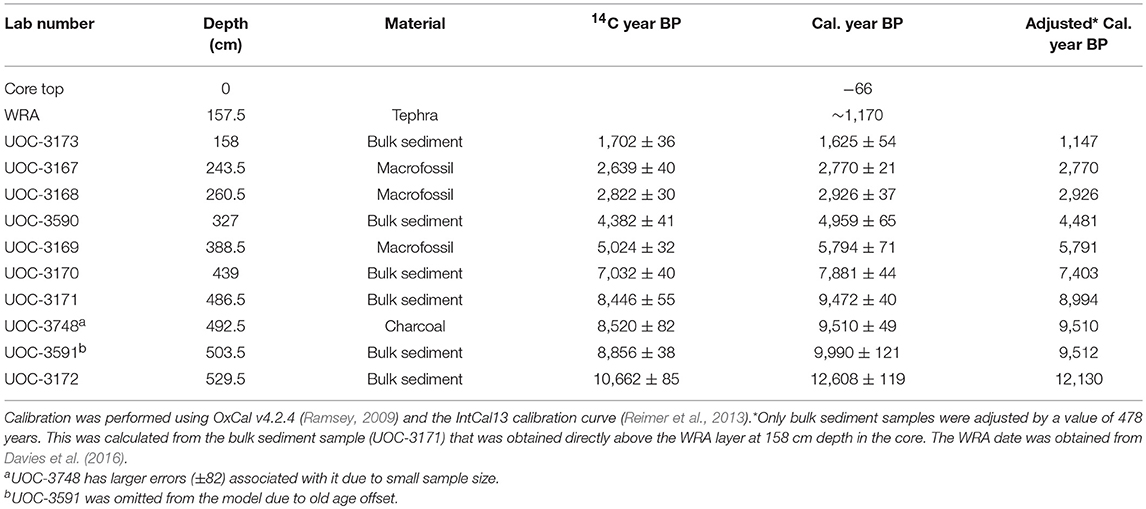
Table 1. Radiocarbon results on bulk sediment and macrofossil samples analyzed at the A.E. Lalonde AMS Laboratory in Ottawa, Canada.
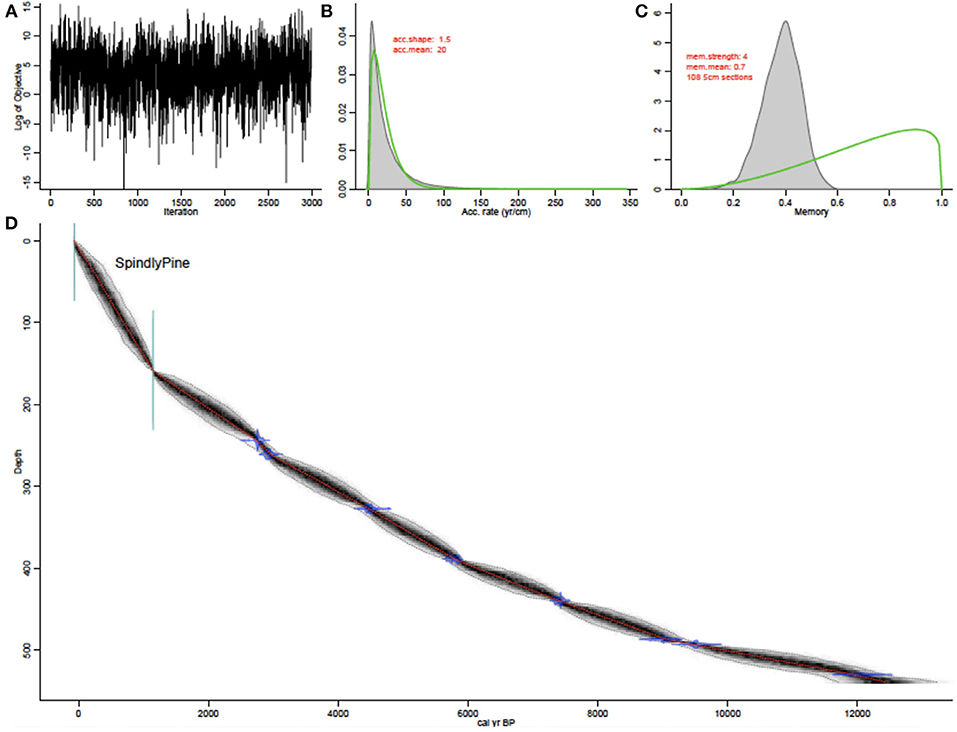
Figure 2. (A) Markov chain Monte Carlo iterations of the BACON output; (B) Distribution of accumulation rates; (C) Memory/autocorrelation, demonstrating how the accumulation rate at depth in the core is dependent on nearby depths. Low memory indicates changing sedimentation rates throughout the core, while high memory indicates a more smooth, and consistent depositional history; (D) Age depth model created with BACON V 2.2 in R Studio. The horizontal green lines represent known dates, blue lines represent the 14C dates and the red line is the model of best fit.
The chronology indicates changing sedimentation rates throughout the record. In the early Holocene, sedimentation rates were slow at 0.16 mm/year. Sedimentation rates increased throughout the record to 0.45 mm/year in the middle Holocene and reach their most rapid rates in the late Holocene sediments at 1.35 mm/year. Therefore, each 0.5 cm sampling interval used for macroscopic charcoal, LOI and MS represents ~6–7 years of deposition during the late Holocene, ~10 years throughout the middle Holocene and ~20 years in the early Holocene when sedimentation rates were slower. A second model was run in CHARAnalysis to address any concern of bias in the record due to changing sedimentation rates. However, the results of this model suggest that the change in sedimentation rates did not impact the CHARAnalysis results. A detailed explanation of this procedure is included in the Supplementary Material.
Sedimentology
The magnetic susceptibility values were low throughout the majority of the record, typically < 10 × 10−6 SI (Figure 3). Small, occasional peaks could have resulted from erosion events in the catchment. The large peak of 238 × 10−6 SI at 158 cm represents the WRA deposit. Smaller peaks between 158 and ~170 cm represent samples where the denser WRA material has migrated downcore into the less dense gyttja below, similar to processes described by Beierle and Bond (2002). MS reached a maximum value of 368 × 10−6 SI at the bottom of the core (539.5-cm). Sediment at this depth abruptly transitions from gyttja to silty clay, which was likely deposited at the termination of the last glaciation and during the formation of Spindly Pine Lake.
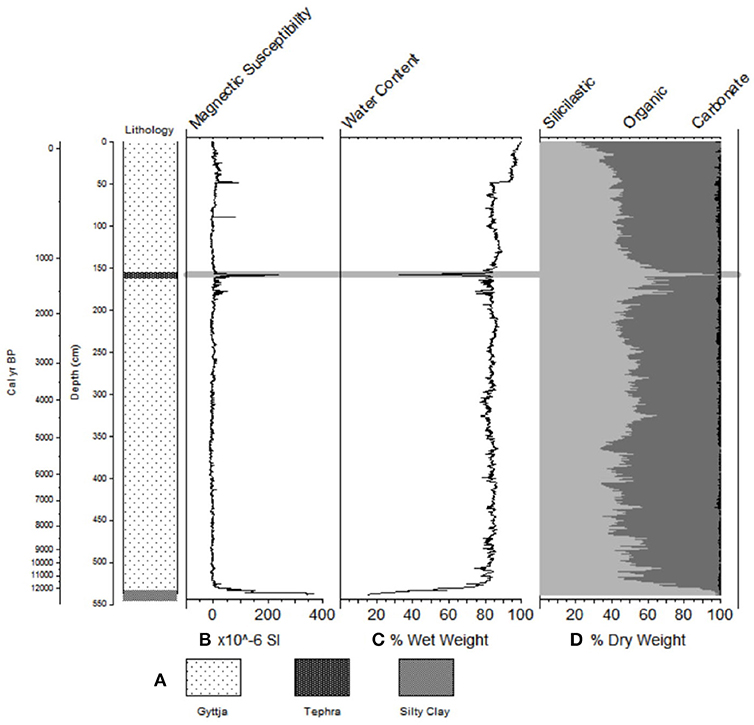
Figure 3. Sedimentology of the sediment record for Spindly Pine Lake, including (A) lithology, (B) magnetic susceptibility, (C) water content, (D) loss-on-ignition. The gray horizontal line represents the tephra deposit. Loss-on-ignition shows the dry weight (%) of organic, carbonate and siliciclastic material.
Water content is high throughout the sediment core, typically ranging from 80 to 84% and reaching 92–99% at the sediment water interface. Water content is comparably lower at 158 cm (~32%) in association with the WRA deposit and in the basal sediments of the core (~14%) where silty clays are dominant. The sudden drop in water content at 48 cm is attributed to water loss during storage of the piston core, which was collected ~10 years before analysis, rather than a change in sedimentological factors. LOI is relatively high (50–60%) throughout the record, confirming the lake sediment is dominated by gyttja. Steep declines in organic content, to ~3%, occur in association with the WRA deposit at 158 cm and the silty glacial clay at the base of the sediment core. The organic content increases to its maximum values (60–80%) in the uppermost part of the sediment profile (13 cm to the surface). Carbonate content is low throughout the record (< 5%), with a maximum value of ~10% at 116.5 cm depth in the core.
Fire History
Analysis of the full record from Spindly Pine Lake indicates macroscopic charcoal concentrations ranged from 0 to 441 pieces cm−3 and averaged 25.5 pieces cm−3. CHAR ranged from 0 to 15 pieces cm−2 year−1 with a mean of 1 piece cm−2 year−1 (Figure 4A). Periods with no charcoal present occurred in the early Holocene (~12,450–11,100 year BP), when the sediment record was dominated by silty clay. The highest macroscopic charcoal concentrations in the entire record occurred at ~6,000 year BP (398 cm) and contained 441 pieces of charcoal per cm3. Based on the macroscopic charcoal record, a total of 91 fire events were identified between ~12,449 year BP and AD 2016 (Figure 4B). The Weibull fitted FRI distributions passed a one-sample Kolmogorov–Smirnov goodness of fit test with ρ >0.05, indicating that the FRIs are normally distributed. The mFRI is 120 years with a 95% confidence interval of 100–142 years (Figure 4C). Fire frequency ranged from 0 to 14 fires per 1,000 years (Figure 5C) with periods of decreased fire frequency occurring between 7,000 and 6,000 year BP and 2,500–1,250 year BP. A significant increase in fire frequency occurred at ~1,000 year BP. The global SNI value for the record is 6.83, and the local SNI is only < 3 in the early Holocene when there was no fire activity. After 10,500 year BP the local SNI is >3 with a maximum value of 15.3 (Figure 5D), suggesting the record is suitable for peak detection analysis.
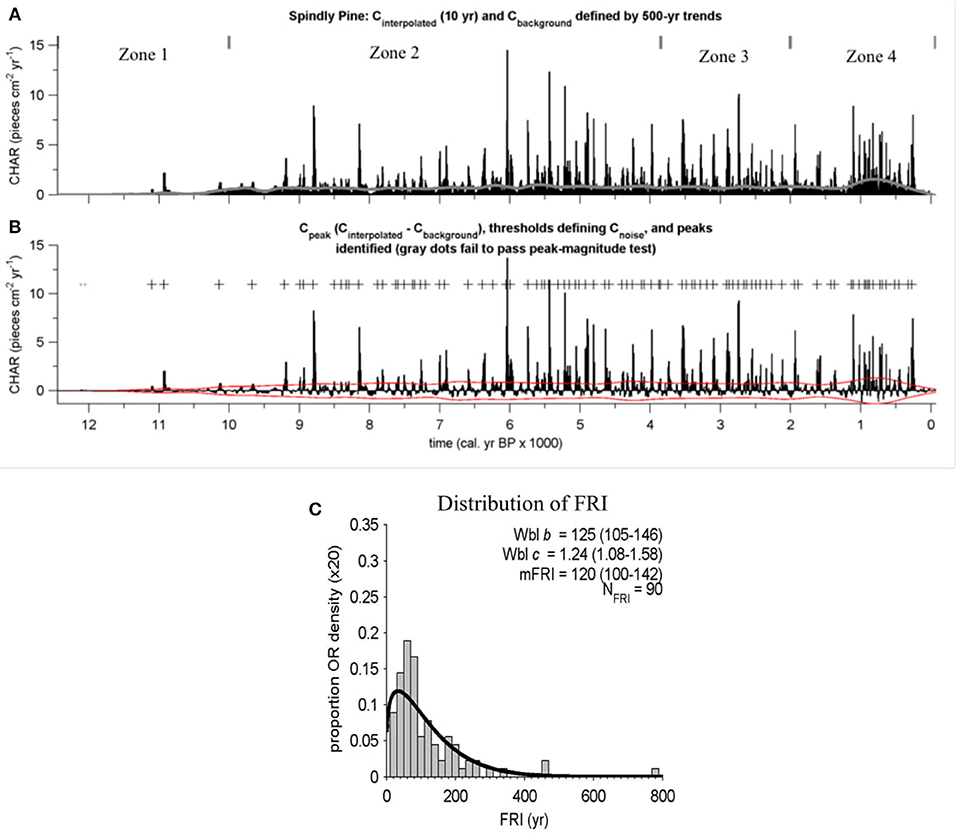
Figure 4. (A) Charcoal accumulation (CHAR) in pieces cm−2 year−1; gray line represents background charcoal levels; (B) Peak identification with plus (+) symbols representing peaks/fire events and gray dots representing peaks that failed to pass the peak-magnitude test; (C) Distribution of fire return intervals (20-year bins) and Weibull model estimates (b and c parameters with 95% CI) The four zones plotted in (A) are based on constrained cluster analyses in CONISS using pollen percentage data.
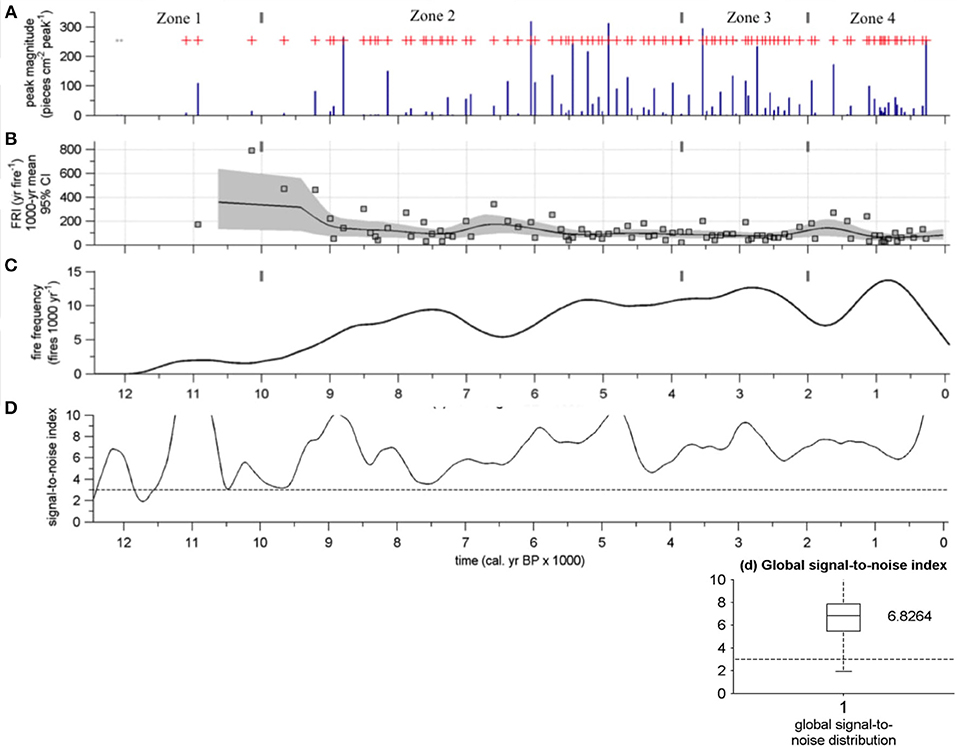
Figure 5. Mean fire return interval and confidence intervals for the four different pollen zones. Distribution of fire return intervals (20-year bins) and Weibull model estimates (b and c parameters with 95% CI).
The mFRI (120 years) for the entire Holocene is similar to other long-term estimates (Higuera et al., 2009; Edwards et al., 2015) ranging between ~100–300 years. However, the FRI distribution (Figure 4C) indicates the area around Spindly Pine Lake has also burned more frequently in the past, with numerous occurrences of two or more fires within a century. The FRIs varied throughout the record with longer FRIs in the early Holocene and shorter FRIs in the late Holocene (Figure 5B). The FRIs were also calculated for the four different pollen zones (Figure 6). Zone 1 had the least amount of fire activity with only 3 fires occurring within the ~2,500 years period between 12,450 year BP and 10,000 year BP. The time between fires for this zone was 170 and 790 years, however, due to the small number of fires in Zone 1 CHARAnalysis could not calculate full fire statistics for pollen Zone 1. Zone 2 (10,000 year BP to 3,850 year BP) had the longest mFRI (124 years) of the three zones for which fire statistics were determined. This zone had slightly longer FRIs, but was the most similar to the long-term Holocene average. Fire activity increased during Zone 3 (3,850 year BP to 2,000 year BP) with a mFRI of 85 years. This was significantly different from Zone 2 and the shortest mFRI for the four pollen zones identified in the sediment record. Fire activity declined only slightly during pollen zone 4 (2,000 year BP to AD 2016), with a mFRI of 88 years.
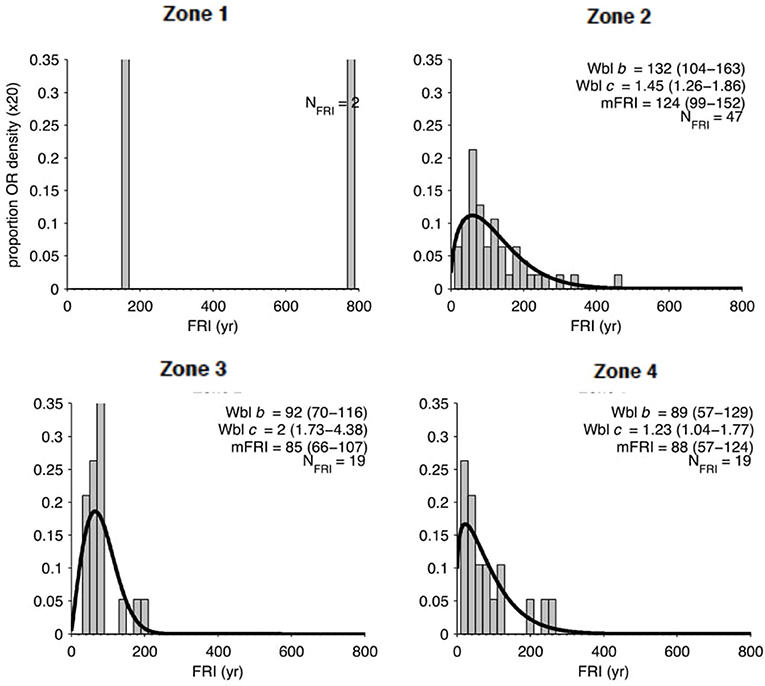
Figure 6. (A) Identified charcoal peaks represented by the red plus symbol and the peak magnitude in dark blue; (B) Fire return interval with 95% confidence intervals; (C) Smoothed fire frequency (fires per 1,000 years); (D) Local signal-to-noise index throughout the record with the global signal-to-noise index below.
Analysis of charcoal morphotypes (Figure 7) indicates the type of charcoal that was produced by fires throughout the Holocene was generally consistent throughout the record. The most prominent types found are the wood categories A3 and B2, described in Courtney Mustaphi and Pisaric (2014). Charcoal morphotype A3 represented ~40–50% of the charcoal pieces examined on average. Charcoal morphotype B2 represented ~20–30%. During the mid-Holocene period from ~5,000 year BP to 2,000 year BP, charcoal morphology shifts slightly and becomes less A3 and B2 dominant, with an increase in A2, B1, B5, B6, C1, C5, and C7. The largest change in relative percent occurred in A3, dropping ~25%. From ~5,000 year BP to 2,000 year BP there is a larger variety of material being burned compared to the rest of the record. Further, between ~5,000 and ~2,000 year BP, there are many high magnitude peaks, including some of the largest peaks in the record, suggesting more severe fires and more material burned.
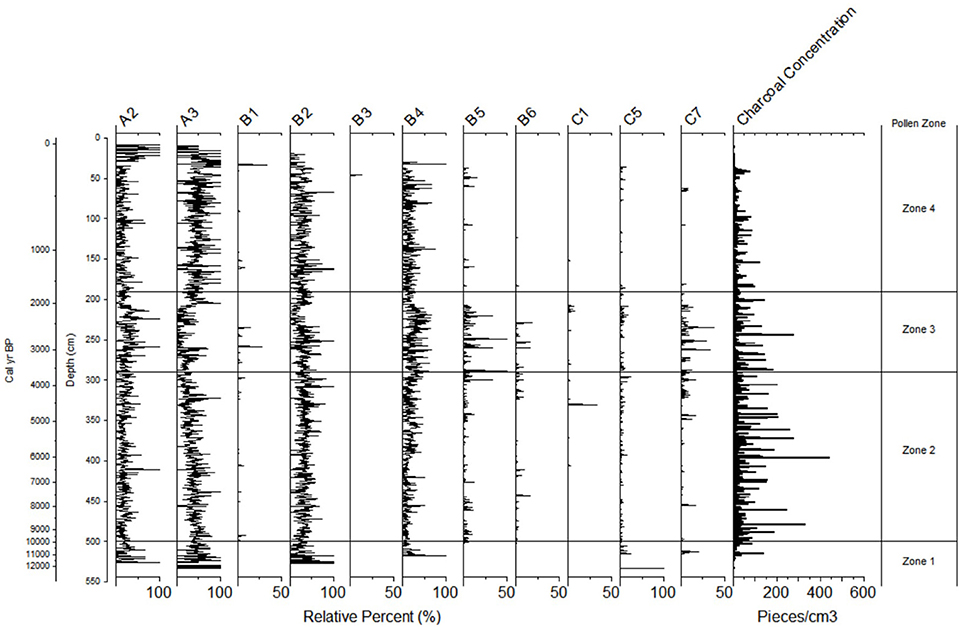
Figure 7. Charcoal morphology for Spindly Pine Lake based on categories derived by Courtney Mustaphi and Pisaric (2014).
Dendrochronology
Dendrochronological samples of fire scars (Figure 8) collected from trees surrounding Annie Lake, a larger lake < 1 km east of Spindly Pine Lake, show evidence of 3 recent fire events (Robillard, 2012). These fires occurred in AD 1725, 1833, and 1892, indicating the last fire in the catchment occurred ~125 years ago. Dates of establishment (5-year bins) based on tree cores collected from mature lodgepole pine and white spruce trees around Spindly Pine Lake suggests the current canopy established at ~ AD 1915. This suggests the current forest around Spindly Pine Lake could represent post fire establishment after the AD 1892 fire. Interestingly, a single lodgepole pine tree from this site was dated to 179 years old, suggesting this tree survived the 1892 fire and perhaps represents regrowth following the 1833 fire.
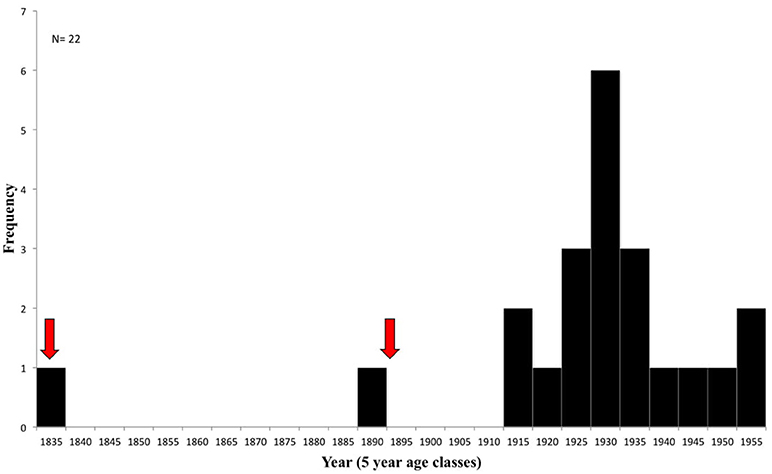
Figure 8. Relative abundance pollen diagram for Spindly Pine Lake. CONISS was used to determine significant changes in vegetation, identifying 4 main pollen zones. Stomata are shown as presence or absence. A total of 300 pollen grains were counted for all depths.
Vegetation
Analysis of pollen samples identified four main vegetation zones during the Holocene based on the Spindly Pine Lake sediment record (Figure 9). These are summarized as follows.
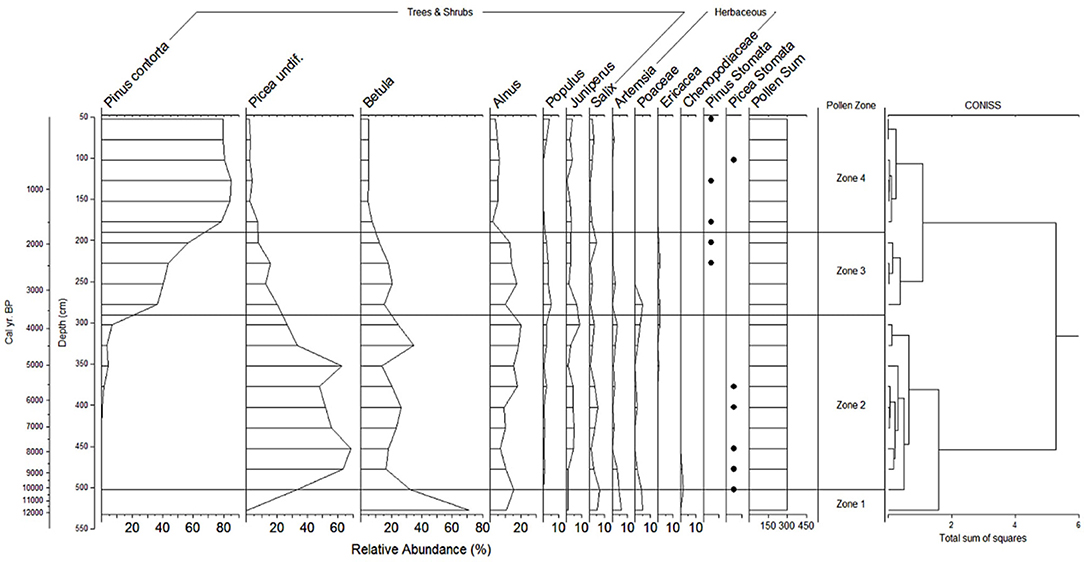
Figure 9. Histogram showing Spindly Pine Lake stand establishment (5-year bins). Recent fires occurred in AD 1725, 1833, and 1892 and are represented by a red arrow. The recent fires in the catchment were determined by dating fire scars on lodgepole pine trees collected from three sites in the area surrounding Annie Lake, located within 1 km of Spindly Pine Lake (Robillard, 2012). At each site, fire scar dates represent the year of fire based on multiple trees at each site.
Zone 1: ~12,450 year BP to 10,000 year BP (539.5–500 cm) Zone 1 represents the onset of the Holocene at Spindly Pine Lake. Pollen from Zone 1 is dominated by shrubs, in particular Betula which accounts for ~70% of the pollen rain. Alnus pollen contributes ~10% of the pollen rain in Zone 1. Salix, Artemisia and Poaceae are also relatively abundant, with each of these pollen types accounting for ~5% of the pollen rain in Zone 1. Picea pollen is relatively low in Zone 1, but increases rapidly at the transition between pollen zones 1 and 2. Picea stomata are absent in Zone 1.
Zone 2: ~10,000 year BP to 3,850 year BP (500–300 cm) Picea pollen reaches maximum values of ~60% in Zone 2. Picea pollen increases from the bottom of Zone 2 at ~10,000 year BP before decreasing to ~25% at the top of Zone 2 (~4,000 year BP). Picea stomata occur throughout the zone, indicating that spruce trees were growing in the vicinity of Spindly Pine Lake since 10,000 year BP. During the transition between Zone 1 and 2, Betula decreases to ~35% and remains between ~20–30% throughout Zone 2. Alnus remains relatively abundant in Zone 2, increasing slightly to ~15%. Pinus contorta pollen is absent throughout the majority of Zone 2. Lodgepole pine appears at the top of Zone 2 in relatively low abundance, accounting for < 5% of the pollen rain.
Zone 3: ~3,850 year BP to 2,000 year BP (300–200 cm) Zone 3 is marked by the sustained occurrence of lodgepole pine pollen in the sediment record. Lodgepole pine pollen increases throughout Zone 3 from ~5% (~3,850 year BP) to a maximum of 55% of the pollen rain, at the top of Zone 3 (~2,000 year BP). Pinus stomata are present near the end of Zone 3 at ~2,500 year BP, when Pinus pollen reaches ~40% of the pollen rain, marking the arrival of lodgepole pine in the area around Spindly Pine Lake. Picea pollen continues to decrease in Zone 3 from ~25% at the start of the zone (~3,850 year BP) to ~10% at the top of the zone (~2,000 year BP). Betula remains abundant throughout Zone 3, accounting for ~20% of the pollen rain with a slight decrease to ~15% at the top of Zone 3. Alnus pollen was consistent at ~15% throughout Zone 3.
Zone 4: ~2,000 year BP to AD 2016 (200 cm to core top) Zone 4 is characterized by the continued expansion of lodgepole pine in the area. Pine dominates this period, increasing to ~80% of the pollen rain throughout Zone 4 (~2,000 year BP to 2016 AD). Pinus stomata are also present throughout the uppermost pollen zone, suggesting lodgepole pine was an important component of the vegetation cover surrounding Spindly Pine Lake during this period. Picea pollen continued to decrease at the start of Zone 4 to ~5%, where it remains at present. Picea stomata were present throughout Zone 4, indicating the local presence of spruce at Spindly Pine Lake. This is supported by current observations of spruce on the landscape around Spindly Pine Lake. Betula and Alnus decrease in Zone 4, accounting for ~5% of the pollen rain, respectively.
Discussion
A 539.5 cm sediment core was collected from a small lake in southwest Yukon and analyzed for pollen, stomata, macroscopic charcoal, and sedimentological parameters. Based on the occurrence of glacial silt at the base of the sediment core, 10 radiocarbon dates and one tephra deposit, the record provides an uninterrupted record of environmental and climate change in this region from the end of the last glaciation (McConnell) to the present. The ice sheet began to retreat prior to ~13,000 year BP and deglaciation was complete prior to ~10,000 year BP (Jackson et al., 1991). The study region was likely one of the last areas to become ice-free during the Cowley stage, one of the later stages in the McConnell deglaciation (Bond, 2004).
The pollen record for this study provides a reference for species abundance with the main objective of identifying the arrival of lodgepole pine in this region and the effects pine has on fire frequency. Several pollen records have been developed for other lakes across southern Yukon (MacDonald and Cwynar, 1985; Cwynar and Spear, 1995; Edwards et al., 2015). These records were developed with higher resolution and corroborate the major vegetation changes that are noted in the Spindly Pine Lake record during the Holocene, including the timing of arrival of lodgepole pine. Therefore, the interpretation of the Spindly Pine Lake pollen record is supported, as well as informed, by these records.
Early Holocene: ~12,450 Year BP to ~8,000 Year BP
After deglaciation, Betula shrubs dominated the area. Conifer species were absent during this period (~12,450 year BP to 10,000 year BP) as Picea had not yet migrated into the region. Results from this study indicate fire activity was low and intermittent following deglaciation with low fire frequency (< 3 fires per 1,000 years). Fire activity increased after 9,500 year BP (~8 fires per 1,000 years). The increase in fire activity was synchronous with the expansion of Picea into the area at ~10,000 year BP, replacing Betula as the dominant species. The arrival of Picea across the region led to an increase in biomass and fuel accumulation. The higher biomass on the landscape was driven by large scale warming across the Northern Hemisphere driven by orbital variations and changing receipts of solar radiation. These top-down controls on vegetation abundance probably drove this early-Holocene increase in fire activity. The arrival of spruce in the area is supported by the occurrence of spruce stomata at the start of Zone 2. Although white spruce (Picea glauca) and black spruce (Picea mariana) were not differentiated for this study, Cwynar and Spear (1995) show that white spruce was responsible for the majority of the spruce pollen in southwest Yukon at this time.
Middle Holocene: ~8,000 Year BP to ~3,000 Year BP
Spruce pollen was most abundant in the record during the middle Holocene between ~8,000 year BP and 4,500 year BP; following this the abundance of spruce pollen began to decline (Figure 9). Initially, during this decline there was an increase in the abundance of Betula, although this was short-lived until ~3,300 year BP when lodgepole pine began to advance into the region. As lodgepole pine migrated north, lodgepole pine pollen increased in abundance and stomata of lodgepole pine were noted in the sediment record in Zone 3.
A decrease in fire frequency during the middle Holocene occurred between 7,000 and 6,000 year BP when fire frequency decreased to ~5–6 fires per 1,000 years with longer FRIs of ~170 years. An increase in black spruce and green alder in southern Yukon between 6,500 year BP and 6,000 year BP is noted from several studies (Keenan and Cwynar, 1992; Cwynar and Spear, 1995) and probably represented a transition to a cooler and wetter climate. These cool and wet conditions may have suppressed fire activity in the region, leading to lower fire frequencies in the mid-Holocene. After ~6,000 year BP, fire frequency increased again to ~10 fires per 1,000 years.
The middle Holocene can be characterized as a high-severity fire regime as it may have contained many of the largest fires throughout the entire record based on the number of particles entering the lake. Fire activity increased throughout the middle Holocene, increasing to as many as 10 fires per 1,000 years with a FRI of ~95 years at ~5,500 year BP. The fire with the largest peak magnitude in the record occurred at ~6,000 year BP with an influx of 441 charcoal pieces per cm3. This large influx of charcoal, indicating high-severity, followed an extended period of low fire activity in the charcoal record, which could have led to a build up of fuel as a result of the lower fire frequency. Climate may be an important driver of this change, with the shift to a cooler and wetter climate. This high-severity fire regime also occurred during a period when spruce forests dominated.
Late Holocene: ~3,000 Year BP to Present
During the late Holocene the expansion of lodgepole pine into southern Yukon was well-underway. Lodgepole pine populations expanded rapidly via local population growth, which can be triggered by fire (Edwards et al., 2015). At Spindly Pine Lake, the expansion of lodgepole pine was likely a response to lodgepole pine extending its range following the end of the last glaciation and high fire activity at ~3,000 year BP, which could have opened up the regional spruce dominated forests and assisted in lodgepole pine becoming established following its arrival in the region. The timing of the regional pine expansion occurred at ~3,000 year BP, however pine established locally around Spindly Pine Lake at ~2,500 year BP as indicated by the occurrence of pine stomata in the sediment record. This is consistent with other studies, including MacDonald and Cwynar (1985), which suggest that pine arrival at nearby Kettlehole Pond occurred at 2,490 year BP.
Fires became more frequent after the expansion of lodgepole pine (~3,000 year BP), highlighting an important transition from predominantly top-down fire controls to the bottom-up changes associated with the arrival of lodgepole pine in this region. The mFRI throughout Zone 4 was 88 years (Figures 5B and 6). However, a period of decreased fire activity occurred between 2,500 and 1,000 year BP. During this time FRIs lengthened to a mFRI of ~150 years, and several fires during this period had time between fires that exceeded 200 years. This decline was attributed to Neoglacial cooling as well as wetter conditions, which is supported by isotopic data derived from a lake sediment record from nearby Jellybean Lake (Anderson et al., 2005; Figure 10). The Neoglacial cooling occurred near the end of this period (~1,400 year BP to ~1,100 year BP), resulting in cooler temperatures and regional glacial advances (Anderson et al., 2005; Davis et al., 2016). During the period from 2,500 to 1,000 year BP the Aleutian Low pressure system is also believed to have been in a prolonged westerly and weak position (Figure 10). This allowed for more precipitation to be carried into central Yukon via this zonal pattern. By comparing the AL with precipitation:evaporation ratios derived from δ18O, Anderson et al. (2007) found the westerly and weak position of the AL correlates with wetter conditions in southwest Yukon. Conversely, the more easterly and stronger AL is associated with drier conditions across southern Yukon. The isotopic data and inferences about the position and strength of the AL derived from them, suggests the period from 2,500 to 1,000 year BP was dominated by a westerly positioned and relatively weak AL, leading to wetter conditions and lower fire activity in the region.
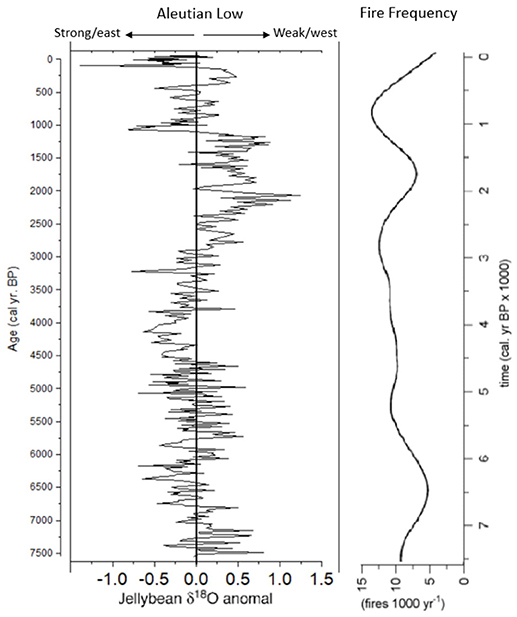
Figure 10. Reconstruction of Aleutian Low strength/position using δ18O from Jellybean Lake (Anderson et al., 2005) with fire frequency from Spindly Pine Lake.
Following the period from 2,500 to 1,000 year BP, an increase in fire frequency occurred at ~1,000 year BP. This represented the most active period in terms of fire events throughout the entire Holocene. Fire frequency increased to ~13 fires per 1,000 years at ~1,000 year BP with short FRIs of ~70–80 years. From ~1,000 year BP to 650 year BP, there were numerous instances when multiple fires occurred within a century resulting in the shortest FRIs (~20–30 years) of the entire record. During this time the AL switched from westerly and weak to easterly and strong (Figure 10), leading to a more meridional pattern and limiting the amount of precipitation moving into central Yukon. In addition to the shift in the position of the AL, this period also coincides with the Medieval Climate Anomaly (MCA; Mann et al., 2009), a period of warmth in the late Holocene that is comparable to current conditions. Therefore, the increased fire activity may be a result of the combined effects of warm and dry conditions from the MCA and contemporaneous changes associated with the AL.
Fire activity decreased again between 600 year BP to the present. This decrease in fire activity coincides with a shift in the AL to a westerly and weak position (Figure 10) and the onset of the Little Ice Age (LIA; Luckman, 2000; Mann et al., 2009). The LIA led to cooling across many parts of the northern hemisphere, including western Canada, and led to regional glacial advances. The cooler conditions of the LIA likely suppressed fire activity through a number of different mechanisms including decreased biomass accumulation and higher fuel moisture levels due to lower evaporation in response to cooler conditions.
The changes in fire frequency that occurred in association with a shifting AL, the MCA and LIA suggest that top-down controls (i.e., climate) are important drivers of fire in this region. Similarly, the significant shift in mFRI when lodgepole pine expanded into southwest Yukon highlights the importance that bottom-up controls (i.e., vegetation) also have on fire occurrence in southwest Yukon.
Current Conditions
Tree establishment dates and fire scar data from directly around Spindly Pine Lake and nearby Annie Lake indicate it has been a minimum of 125 years since the last fire in the Spindly Pine Lake catchment. The limited fire activity in the modern record, relative to Zone 4, is likely a result of current fire management and prevention practices that contribute to fire suppression. Comparison of the time since the last fire in the catchment (125 years) with the mFRI for the entire record (120 years), suggests the area around Spindly Pine Lake is overdue for a large fire. However, the current forest composition is an important consideration as the FRIs did change throughout the record. The current vegetation around the lake is representative of that which occurred throughout Zone 4, with lodgepole pine as the dominant species. Therefore, the mFRI for Zone 4 (88 years) is probably a more accurate comparison than the average mFRI for the entire Holocene and further highlights that this part of the southern Lakes region of Yukon is overdue for a large stand-replacing fire event. The risk is heightened due to the fact that fine fuels have now had more than a century to accumulate on this landscape in the absence of fire, exceeding not only the Holocene mFRI, but also the normal fire activity of the past several thousand years when boundary and vegetation conditions have been similar to those at the present time. Further complicating these fire conditions is the ongoing warming that has occurred in this region throughout the twentieth and into the twenty-first century. Over the past 50 years temperatures have increased by 2°C in Yukon and they are projected to increase another 2°C over the next 50 years (Streicker, 2016). In addition, in the North Pacific Ocean, the AL has been in a prolonged easterly position (Figure 10). Several studies (McCoy and Burn, 2005; Flannigan et al., 2009, 2013) have predicted a change in the fire regime in response to changing climatic conditions, with increased temperatures leading to higher fire frequency and severity. The fire record from Spindly Pine Lake, especially at ~1,000 year BP, is a useful comparison to portend future fire scenarios for this region. At ~1,000 year BP vegetation and boundary conditions, including the position of the AL (Figure 10), were similar to present conditions. The Spindly Pine fire record indicates these conditions led to some of the highest fire activity recorded in the Spindly Pine Lake charcoal record during the Holocene. Similarly, the largest fire event(s) based on influx of charcoal particles at ~6,000 year BP, occurred after a period of prolonged absence of fire on the landscape and an easterly positioned AL. Similar conditions are present at the current time across southern Yukon. In addition, continued and predicted warming due to climate change will further exacerbate the potential for large fires in southern Yukon in the coming decades.
Conclusions
This study presents a full Holocene record of fire history conducted at sub-decadal to decadal resolution in concert with a robust age-depth model. Results suggest that climate has been an important top-down influence on fire regimes in this area, with the Aleutian Low pressure system in particular being an important climatic driver of fire across this region. Periods of increased fire frequency coincide with a strong and easterly positioned AL, while periods of decreased fire frequency coincide with a weak and more westerly positioned AL. In addition, large shifts in the fire regime also occurred in association with known changes in climate, most notably the MCA and the LIA.
Edwards et al. (2015) concluded that fire might have triggered a rapid expansion of lodgepole pine in southwest Yukon. The results of the current study support this suggestion. The Spindly Pine Lake pollen record includes an extended pine pollen tail, followed by a zone of pine expansion occurring synchronously with a shift to lower mFRIs. Prior to lodgepole pine becoming established in this region, the mFRI was ~124 years. Following the expansion of lodgepole pine the mFRI declined to ~85 years and has remained in this range since lodgepole pine became fully established and dominant across southern Yukon. It is important to recognize however, the important role that climate plays in controlling fire regimes in southwest Yukon, because even during the initial expansion of lodgepole pine, a prolonged period of weak and westerly positioned AL led to decreased fire activity.
Edwards et al. (2015) suggest that only one of their four study sites showed a statistically significant increase in mFRI after lodgepole pine expanded across the region. The charcoal record from Spindly Pine Lake seems to support the idea that bottom-up controls can be important, but the lack of response at their other study sites highlights the importance of site-to-site variation. Due to the natural variability of wildfire and the possible overprint of climate on fire regimes in this region, bottom-up controls may vary between individual sites. Future studies should increase the number of study sites across the region to better assess the synchrony of changing fire regimes in response to both bottom-up and top-down controls.
The highest magnitude fire in the Spindly Pine Lake charcoal record followed a period of decreased fire activity. This supports the notion that bottom-up controls of the fire regime, via the accumulation of fine fuels, can also be an important factor controlling fire regimes. The distributions of FRIs in this study are in agreement with previous estimates of FRIs for the boreal forest, albeit at the lower end of previous estimates. When factoring in different vegetation types, the estimates of mFRI decrease further depending on which conifer tree species are dominant. In particular, when species, such as lodgepole pine increase in importance and dominance on the landscape, fire return intervals shorten dramatically. This information is important for forest managers and planners involved in the assessment and management of fire in these fire-prone landscapes in southern Yukon. The results from this study suggest the landscape around Spindly Pine Lake is overdue for a fire event. Given the warming that has occurred across Yukon during the past 50 years, the susceptibility of the landscape to the occurrence of a major fire is further increased. Unfortunately, it is unlikely that the conditions around Spindly Pine Lake are anomalous across southern Yukon and certainly many other regions are probably also overdue and highly susceptible to future fire events as lodgepole pine forests continue to expand beyond the Spindly Pine region. The results from this study provide insights about past fire activity in southern Yukon during periods of warmer conditions and similar vegetation scenarios to those occurring today. These results suggest future fire scenarios in Yukon will be severe.
Author Contributions
MP and KT designed and supervised the paleoecological study. TP completed the laboratory work and the paleoecological analyses. MP and KT provided input to the interpretation of results. TP wrote the first draft of the manuscript. MP and KT contributed to the writing of the manuscript. All authors contributed to the revision of the manuscript, read and approved the submitted version.
Funding
Funding was provided by the Natural Sciences and Engineering Research Council (NSERC) of Canada Discovery and Discovery Northern Supplements programs to MFJP and KT, the Northern Scientific Training Program (NSTP) of Polar Knowledge Canada to TP, Ontario Graduate Scholarship (OGS) and Brock University Research Fellowship to TP. We acknowledge and thank the Brock University Library Open Access Publishing Fund for financial support.
Conflict of Interest Statement
The authors declare that the research was conducted in the absence of any commercial or financial relationships that could be construed as a potential conflict of interest.
Acknowledgments
Field assistance was provided by D. Hughes, R. Marcantonio, D. Youngblut, and K. Moser. We thank B. Hancock for logistical help and D. Iankoulov for technical support. The manuscript was improved based on the helpful and insightful comments from Dr. Mary Edwards and Dr. Thomas Minkley. Permission to conduct field work was granted by the Yukon Government Tourism and Culture Department under Yukon Scientists and Explorers Licence number 16-31S&E. This research was conducted within the traditional territory of the Carcross/Tagish First Nation and we acknowledge and thank them for their permission and support to conduct this research.
Supplementary Material
The Supplementary Material for this article can be found online at: https://www.frontiersin.org/articles/10.3389/fevo.2018.00209/full#supplementary-material
References
Anderson, L., Abbott, M. B., Finney, B. P., and Burns, S. J. (2005). Regional atmospheric circulation change in the North Pacific during the Holocene inferred fromlacustrine carbonate oxygen isotopes, Yukon Territory, Canada. Quat. Res. 64, 21–35. doi: 10.1016/j.yqres.2005.03.005
Anderson, L., Abbott, M. B., Finney, B. P., and Burns, S. J. (2007). Late Holocene moisture balance variability in the southwest Yukon Territory, Canada. Quat. Sci. Rev. 26, 130–141. doi: 10.1016/j.quascirev.2006.04.011
Bassett, I. J., Crompton, C. W., and Parmelee, J. A. (1978). An Atlas of Airborne Pollen Grains and Common Fungus Spores of Canada. Ottawa, ON: Printing and Publishing Supply and Services Canada.
Beierle, B., and Bond, J. (2002). Density-induced settling of tephra through organic lake sediments. J. Paleolimnol. 28, 433–440. doi: 10.1023/A:1021675501346
Bond, J. D. (2004). “Late Wisconsinan McConnell glaciation of the Whitehorse map area (105D), Yukon,” in Yukon Exploration and Geology, eds D. S. Emond and L. L. Lewis (Yukon Geological Survey), 73–88.
Bourgeau-Chavez, L. L., Alexander, M. E., Stocks, B. J., and Kasischke, E. S. (2000). “Distribution of forest ecosystems and the role of fire in the North American boreal region,” in Fire Climate Change Carbon Cycl. Boreal Forest, eds E. S. Kasischke and B. J. Stocks (New York, NY: Springer) 138, 111–131. doi: 10.1007/978-0-387-21629-4_7
Courtney Mustaphi, C., and Pisaric, M. F. (2014). A classification for macroscopic charcoal morphologies found in Holocene lacustrine sediments. Progr. Phys. Geogr. 38, 734–754. doi: 10.1177/0309133314548886
Courtney Mustaphi, J. C., Davis, E. L., Perreault, J. T., and Pisaric, M. F. (2015). Spatial variability H of recent macroscopic charcoal deposition in a small montane lake and implications for reconstruction of watershed-scale fire regimes. J. Paleolimnol. 54, 71–86. doi: 10.1007/s10933-015-9838-2
Cwynar, L., and Spear, R. (1995). Paleovegetation and paleoclimatic changes in the Yukon at 6 ka BP. Géogr. Phys. Quarter. 49, 29–35. doi: 10.7202/033027ar
Cwynar, L. C., Burden, E., and McAndrews, J. H. (1979). An inexpensive sieving method for concentrating pollen and spores from fine-grained sediments. Canad. J. Earth Sci. 16, 1115–1120. doi: 10.1139/e79-097
Davies, L. J., Jensen, B. J., Froese, D. G., and Wallace, K. L. (2016). Late Pleistocene and Holocene tephrostratigraphy of interior Alaska and Yukon: key beds and chronologies over the past 30,000 years. Quat. Sci. Rev. 146, 28–53. doi: 10.1016/j.quascirev.2016.05.026
Davis, E. L., Mustaphi, C. J. C., Gall, A., Pisaric, M. F., Vermaire, J. C., and Moser, K. A. (2016). Determinants of fire activity during the last 3500 yr at a wildland–urban interface, Alberta, Canada. Quarter. Res. 86, 247–259. doi: 10.1016/j.yqres
Edwards, M., Franklin-Smith, L., Clarke, C., Baker, J., Hill, S., and Gallagher, K. (2015). The role of fire in the mid-Holocene arrival and expansion of lodgepole pine (Pinus contorta var. latifolia Engelm. ex S. Watson) in Yukon, Canada . Holocene, 25, 64–78. doi: 10.1177/0959683614556389
Faegri, K., Kaland, P. E., and Krzywinski, K. (1989). Textbook of Pollen Analysis (No. Ed. 4). Chichester, UK; New York, NY: John Wiley and Sons Ltd.
Flannigan, M., Cantin, A. S., de Groot, W. J., Wotton, M., Newbery, A., and Gowman, L. M. (2013). Global wildland fire season severity in the 21st century. Forest Ecol. Manage. 294, 54–61. doi: 10.1016/j.foreco.2012.10.022
Flannigan, M., Stocks, B., Turetsky, M., and Wotton, M. (2009). Impacts of climate change on fire activity and fire management in the circumboreal forest. Global Change Biol. 15, 549–560. doi: 10.1111/j.1365-2486.2008.01660.x
Flannigan, M. D., Logan, K. A., Amiro, B. D., Skinner, W. R., and Stocks, B. J. (2005). Future area burned in Canada. Climatic Change 72, 1–16. doi: 10.1007/s10584-005-5935-y
Gajewski, K., Bunbury, J., Vetter, M., Kroeker, N., and Khan, A. H. (2014). Paleoenvironmental studies in southwestern Yukon. Arctic 67(Suppl),158–170. doi: 10.14430/arctic4349
Glew, J. R., Smol, J. P., and Last, W. M. (2001). “Sediment core collection and extrustion,” in Tracking Environmental Change Using Lake Sediments. Volume 1: Basin Analysis, Coring and Chronological Techniques, eds W. M. Last and J. P. Smol (Dordrect: Kluwer Academic Publishers). 73–105.
Government of Canada. (2017). Historical Climate Data. Available online at: http://climate.weather.gc.ca/climate_normals/results_1981_2010_e.html?stnID=1617andautofwd=1
Grimm, E. C. (1987). CONISS: a FORTRAN 77 program for stratigraphically constrained cluster analysis by the method of incremental sum of squares. Comput Geosci 13, 13–35. doi: 10.1016/0098-3004(87)90022-7
Hansen, B. C. (1995). Conifer stomate analysis as a paleoecological tool: an example from the Hudson Bay Lowlands. Can. J. Botany 73, 244–252. doi: 10.1139/b95-027
Heiri, O., Lotter, A., and Lemcke, G. (2001). Loss on ignition as a method for estimating organic and carbonate content in sediments: reproducibility and comparability of results. J. Paleolimnol. 25, 101–110. doi: 10.1023/A:1008119611481
Higuera, P. (2009). CharAnalysis 0.9: Diagnostic and Analytical Tools for Sediment Charcoal Analysis. User's Guide. Bozeman, MT: Montana State University.
Higuera, P. E., Brubaker, L. B., Anderson, P. M., Hu, F.S., and and Brown, T.A. (2009). Vegetation mediated the impacts of postglacial climate change on fire regimes in the south-central Brooks Range, Alaska. Ecol. Monogr. 79, 201–219. doi: 10.1890/07-2019.1
Higuera, P. E., Gavin, D. G., Bartlein, P. J., and Hallett, D. J. (2010). Peak detection in sediment–charcoal records: impacts of alternative data analysis methods on fire-history interpretations. Int. J. Wild. Fire 19, 996–1014. doi: 10.1071/WF09134
Jackson, L., Ward, B., Duk-Rodkin, A., and Hughes, O. (1991). The last Cordilleran ice sheet in southern Yukon Territory. Géogr. Phys. Quarter. 45, 341–354. doi: 10.7202/032880ar
Johnson, J. A. (1992). Fire and Vegetation Dynamics: Studies From the North American Boreal Forest. New York, NY: Cambridge University Press.
Johnstone, J. F., and Chapin, F. S. (2006). Fire interval effects on successional trajectory in boreal forests of northwest Canada. Ecosystems 9, 268–277. doi: 10.1007/s10021-005-0061-2
Johnstone, J. F., McIntire, E. J., Pedersen, E. J., King, G., and Pisaric, M. J. (2010). A sensitive slope: estimating landscape patterns of forest resilience in a changing climate. Ecosphere 1, 1–21. doi: 10.1890/ES10-00102.1
Keenan, T. J., and Cwynar, L. C. (1992). Late Quaternary history of black spruce and grasslands in southwest Yukon Territory. Can. J. Botany 70, 1336–1345. doi: 10.1139/b92-168
Kelly, R. F., Higuera, P. E., Barrett, C. M., and Hu, F. S. (2011). A signal-to-noise index to quantify the potential for peak detection in sediment-charcoal records. Quarter. Res. 75, 11–17. doi: 10.1016/j.yqres.2010.07.011
Luckman, B. H. (2000). The little ice age in the Canadian Rockies. Geomorphology 32, 357–384. doi: 10.1016/S0169-555X(99)00104-X
MacDonald, G. M., and Cwynar, L. C. (1985). A fossil pollen based reconstruction of the late Quaternary history of lodgepole pine (Pinuscontorta ssp. latifolia) in the western interior of Canada. Can. J. Forest Res. 15, 1039–1044. doi: 10.1139/x85-168
Mann, M. E., Zhang, Z., Rutherford, S., Bradley, R. S., Hughes, M. K., Shindell, D., et al. (2009). Global signatures and dynamical origins of the Little Ice Age and Medieval Climate Anomaly. Science 326, 1256–1260. doi: 10.1126/science.1177303
McAndrews, J. H., Berti, A. A., and Norris, G. (1973). Key to the Quaternary Pollen and Spores of the Great Lakes Region. Ottawa, ON: The Royal Ontario Museum.
McCoy, V. M., and Burn, C. R. (2005). Potential alteration by climate change of the forest-fire regime in the boreal forest of central Yukon Territory. Arctic 276–285. doi: 10.14430/arctic429
Mock, C. J., Bartlein, P. J., and Anderson, P. M. (1998). Atmospheric circulation patterns and spatial climatic variations in Beringia. Int. J. Climatol. 18, 1085–1104.
Moore, P. D., Webb, J. A., and Collison, M. E. (1991). Pollen Analysis. Hong Kong: Blackwell scientific publications.
Patterson, R. T., Crann, C. A., Cutts, J. A., Courtney Mustaphi, C. J., Nasser, N. A., Macumber, A. L., et al. (2017). New occurrences of the White River Ash (east lobe) in Subarctic Canada and utility for estimating freshwater reservoir effect in lake sediment archives. Palaeogeogr. Palaeoclimatol. Palaeoecol. 477, 1–9. doi: 10.1016/j.palaeo.2017.03.031
Payette, S. (1992). “Fire as a controlling process in the North American boreal forest,” in A Systems Analysis of the Boreal Forest. eds H. H. Shugart, R. Leemans, and G. B. Bonan (Cambridge: Cambridge University Press), 144–169.
Pisaric, M. F., Holt, C., Szeicz, J. M., Karst, T., and Smol, J. P. (2003). Holocene treeline dynamics in the mountains of northeastern British Columbia, Canada, inferred from fossil pollen and stomata. Holocene 13, 161–173. doi: 10.1191/0959683603hl599rp
Ramsey, C. B. (2009). Bayesian analysis of radiocarbon dates. Radiocarbon 51, 337–360. doi: 10.1017/S0033822200033865
Reimer, P. J., Bard, E., Bayliss, A., Beck, J. W., Blackwell, P. G., Ramsey, C. B., et al. (2013). IntCal13 and Marine13 radiocarbon age calibration curves 0–50,000 years cal BP. Radiocarbon 55, 1869–1887. doi: 10.2458/azu_js_rc.55.16947
Robillard, K. (2012). A 250-Year Record of Forest Fire History in Canada's Southwestern Yukon. [Undergraduate thesis]. Department of Geography and Environmental Studies, Carleton University, Ottawa, ON.
Schlachter, K. J., and Horn, S. P. (2010). Sample preparation methods and replicability in macroscopic charcoal analysis. J. Paleolimnol. 44, 701–708. doi: 10.1007/s10933-009-9305-z
Scudder, G. G. E. (1997). Environment of the Yukon. Insects of the Yukon. Ottawa, ON: Biological Survey of Canada (Terrestrial Arthropods), 13–57.
Smith, C. A. S., Meikle, J. C., and Roots, C. F. (2004). Yukon Southern Lakes. Ecoregions of the Yukon Territory: Biophysical Properties of Yukon Landscapes, (04-01), Summerland, BC: Agriculture and Agri-Food Canada. 207–218.
Smol, J. P. (2009). Pollution of lakes and Rivers: A Paleoenvironmental Perspective. Singapore: John Wiley and Sons.
Spooner, I. S., Barnes, S., Baltzer, K. B., Raeside, R., Osborn, G. D., and Mazzucchi, D. (2003). The impact of air mass circulation dynamics on Late Holocene paleoclimate in northwestern North America. Quarter. Int. 108, 77–83. doi: 10.1016/S1040-6182(02)00196-9
Spracklen, D. V., Mickley, L. J., Logan, J. A., Hudman, R. C., Yevich, R., Flannigan, M. D., et al. (2009). Impacts of climate change from 2000 to 2050 on wildfire activity and carbonaceous aerosol concentrations in the western United States. J. Geophys. Res. 114:D20. doi: 10.1029/2008JD010966
Stockmarr, J. (1971). Tablets With Spores Used in Absolute Pollen Analysis. Pollen et spores. 13, 615–621.
Stocks, B. J. (2004). Forest fires in the boreal zone: climate change and carbon implications. Int. For. Fire News 31, 122–131.
Streicker, J. (2016). Yukon Climate Change Indicators and Key Findings 2015. Northern Climate ExChange, Yukon Research Centre, Yukon College. p. 84.
Trenberth, K. E., and Hurrell, J. W. (1994). Decadal atmosphere-ocean variations in the Pacific. Climate Dynam. 9, 303–319. doi: 10.1007/BF00204745
Veraverbeke, S., Rogers, B. M., Goulden, M. L., Jandt, R. R., Miller, C. E., Wiggins, E. B., et al. (2017). Lightning as a major driver of recent large fire years in North American boreal forests. Nat. Climate Change 7, 529–534. doi: 10.1038/nclimate3329
Weber, M. G., and Stocks, B. J. (1998). Forest fires and sustainability in the boreal forests of Canada. Ambio 27, 545–550.
Whitlock, C., and Larsen, C. (2001). “Charcoal as a fire proxy,” in Tracking Environmental Change Using Lake Sediments. Volume 3: Terrestrial, Algal, and Siliceous Indicators, eds J. P. Smol, H. J. B. Birks and W. M. Last (Dordrecht: Kluwer Academic Publishers), 75–97.
Keywords: wildfire, charcoal, Yukon, paleoecology, lodgepole pine, climate change, Holocene
Citation: Prince TJ, Pisaric MFJ and Turner KW (2018) Postglacial Reconstruction of Fire History Using Sedimentary Charcoal and Pollen From a Small Lake in Southwest Yukon Territory, Canada. Front. Ecol. Evol. 6:209. doi: 10.3389/fevo.2018.00209
Received: 04 August 2018; Accepted: 27 November 2018;
Published: 19 December 2018.
Edited by:
Scott Andrew Mensing, University of Nevada, Reno, United StatesReviewed by:
Thomas A. Minckley, University of Wyoming, United StatesMary E. Edwards, University of Southampton, United Kingdom
Copyright © 2018 Prince, Pisaric and Turner. This is an open-access article distributed under the terms of the Creative Commons Attribution License (CC BY). The use, distribution or reproduction in other forums is permitted, provided the original author(s) and the copyright owner(s) are credited and that the original publication in this journal is cited, in accordance with accepted academic practice. No use, distribution or reproduction is permitted which does not comply with these terms.
*Correspondence: Michael F. J. Pisaric, bXBpc2FyaWNAYnJvY2t1LmNh