The Role of Grass vs. Exogenous Abrasives in the Paleodietary Patterns of North American Ungulates
- 1Department of Biology, Bay Path University, Longmeadow, MA, United States
- 2ICREA, Barcelona, Spain
- 3Institut Català de Paleoecologia Humana i Evolució Social (IPHES), Tarragona, Spain
- 4Universitat Rovira i Virgili (URV), Àrea de Prehistòria, Tarragona, Spain
- 5Department of Ecology and Evolutionary Biology, Brown University, Providence, RI, United States
- 6School of Earth Sciences, University of Bristol, Bristol, United Kingdom
Equids have often been discussed regarding tooth morphological change due to the evolution of highly hypsodont teeth over time, the hyper-grazing habits of modern horses, and an older view that the acquisition of hypsodonty and the widespread appearance of grasslands were synchronous. Many more recent studies, however, have reported asynchrony in the origin of hypsodonty and the widespread appearance of grasslands and have considered exposure to exogenous grit as important evolutionary drivers of hypsodonty in ungulates. We tracked changes in crown height (hypsodonty index), relative abrasion (mesowear), and food and grit scar topography on dental enamel (microwear) to examine the relative contributions of grass vs. grit as a driving force in ungulate tooth changes during the evolution of North American Equidae compared to four North American ruminant artiodactyl families (Camelidae, Antilocapridae, Dromomerycidae, and Merycoidodontidae). We mirror other studies by finding that the overall pattern of the timing of the attainment of hypsodonty is inconsistent with grazing as the main impetus for the “Great Transition” within equids nor within the artiodactyl families as highly hypsodont ungulates post-date the spread of widespread grasslands. Mesowear closely mirrored hypsodonty trends in all families. Microwear patterns, particularly high degrees of enamel pitting (particularly large pits) and unusually coarse scratch textures in all five families, are consistent with exposure to exogenous grit as the main driver of hypsodonty acquisition prior to the consumption of significant levels of grass. Equidae exhibited a wider array of dietary behavior than the other families through most of their evolutionary history. Even so, grass was a much more common dietary item for equids than for the other families, and when combined with exogenous grit, which was more accelerated from the early Miocene onward based on more pitting and coarser scratch textures, may explain the more extreme acquisition of hypsodonty in equids compared to the artiodactyl families studied and set the stage for the Equidae alone to become hypergrazers in the Recent.
Introduction
Horse evolution has often been presented as a standard example of evolution. This proclivity is not surprising given: (1) well-documented evolutionary changes, particularly in the late early Miocene, (i.e., greater body size, somewhat higher-crowned cheek teeth, and more cursorially-adapted limbs) that have been perceived for a long time to echo higher-latitude environmental shifts through time (i.e., adaptations to shifts from living in tropical forests to eventual occupation of open grasslands) (Janis, 2007) and (2) the expansive fossil record of North American horses (especially in the Miocene) where the bulk of horse evolution and diversification (Equidae) occurred despite several successive dispersals to the Old World (MacFadden, 1992; Janis, 2007). Despite these dispersals to the Old World, the Equidae apparently evolved in isolation in North America from the middle of the Eocene through the late Oligocene (MacFadden, 1992). During the mid-Cenozoic, horses were very widespread in North America—reaching their maximum diversification and abundance in the late Miocene with individual fossil localities often accommodating up to eight equid species (MacFadden, 1992; Hulbert, 1993).
Because food acquisition is so imperative to an animal's survival, paleontologists have long been interested in adaptations that facilitate acquiring and comminuting food items as well as those that help to deal with an enhanced rate of tooth wear induced by food that is abrasive (e.g., grass) or due to exogenous substances possibly adhering to food (e.g., grit). Hummel et al. (2011) tested the relationship of total silica ingested (from plant phytoliths and exogenous grit) and hypsodonty by investigating the correlation between fecal silica content and hypsodonty and demonstrated a considerable influence of ingested silica on hypsodonty in large herbivores. The evolution of high-crowned teeth (i.e., hypsodonty) has particularly intrigued paleontologists (Osborn, 1910; Stirton, 1947; Simpson, 1953; White, 1959; Webb, 1983; Fortelius, 1985; Janis, 1988; Solounias et al., 1994; MacFadden, 2000a,b; Williams and Kay, 2001; Mihlbachler and Solounias, 2006; Strömberg, 2006; Jardine et al., 2012; Lucas et al., 2014). The potential correlation of crown height with habitat, climate, and dietary shifts has also been the subject of intensive study (Webb, 1983; Janis, 1988, 2008; Janis et al., 2000, 2002, 2004; Williams and Kay, 2001; Semprebon and Rivals, 2010; Mihlbachler et al., 2011) as the obtainment of high crowned dentitions had been considered generally as a potential response to a shift in foraging from closed habitats to more open ones and/or from feeding on browse to feeding on grass in the early Miocene (Osborn, 1910; Scott, 1937; Simpson, 1944; Stirton, 1947; Webb, 1977, 1983; Stebbins, 1981; Janis, 1984, 1993; Webb and Opdyke, 1995). Thus, hypsodonty was in the past mostly associated with grass consumption because of the large number of silica-rich phytoliths found in grasses which were presumed to impose accelerated wear of mammalian teeth.
Two important caveats have been considered in recent studies when evaluating the veracity of this old evolutionary “story”: Firstly, was the appearance of hypsodonty in the Miocene truly synchronous with the appearance of grasslands? Secondly, is dietary preference operating alone in determining the degree of hypsodonty acquisition? Recent studies have revealed a lack of synchronicity between the appearance of hypsodonty in the Miocene and the appearance of grasslands. Some of the latest evidence comes from recent research on phytoliths (Strömberg, 2004, 2005, 2011; Strömberg et al., 2016) that has revealed that grass was available for forage in the North American Great Plains region by ~22 million years ago (earliest Miocene), but possibly by even about 26 million years ago (latest Oligocene– Strömberg, 2011) about 6 million years before the appearance of the genus Merychippus. Merychippus represents the first hypsodont horse even though some members of the genus Parahippus showed a slight enhancement in crown height and evidence of profound dental wear (MacFadden and Hulbert, 1988; Strömberg, 2006; Damuth and Janis, 2011; Mihlbachler et al., 2011). Merychippus represents an important milestone in the evolution of horses due to its relatively high crowned cheek teeth, but also due to the first appearance of well-developed cementum between lophs and with the similar positioning of its tooth cusps to modern horses. A change in the shape of the angle of the jaw, indicating a more horizontally-oriented angle of insertion of the masseter muscle was also seen in Merychippus (Stirton, 1947; Simpson, 1951; Turnbull, 1970; MacFadden and Hulbert, 1988; Bernor et al., 1989, 1997; Prothero and Schoch, 1989; Hulbert and MacFadden, 1991; MacFadden, 1992; Spaan et al., 1994). Even so, highly hypsodont equines did not appear until about 14 Ma (late middle Miocene), well after the apparent availability of open grasslands (Damuth and Janis, 2011), a trend echoed by certain artiodactyl clades such as antilocaprids (pronghorns) and camelids that also developed hypsodonty in the late middle Miocene. In addition, a moderate amount of hypsodonty was attained by some artiodactyls in the Oligocene and many families of mostly burrowing rodents at least 7 million years earlier (Jardine et al., 2012). However, these hypsodont artiodactyls (stenomyline camelids, leptauchinine oreodonts, and hypisodine hypertragulids) went extinct in the late Oligocene/early Miocene not giving rise to later hypsodont artiodactyls.
Another important factor to consider is that researchers have suspected for some time that grass phytoliths may not have been the only evolutionary driver in the development of tooth morphological change [e.g., Janis (1988) and Fortelius et al. (2002)]. Janis (1988) has long hypothesized that grit consumption might also be an evolutionary driver through her observations that the degree of hypsodonty in ungulates might be more due to habitat preferences than to dietary preferences. Janis (1988) showed that ground-feeding ungulates in open habitats are significantly more hypsodont than closed habitat ungulates regardless of their preferences for food (see Figure 1). Janis (1988) also noted that ungulates that feed on dicotyledonous material above ground have the lowest hypsodonty values and suggested that dust and grit which accumulates on food consumed in open habitats is more important for determining hypsodonty in ungulates than dietary fiber levels. Others have also considered grit and soil as possibly more important agents of abrasion than grass phytoliths (Stirton, 1947; Janis, 1988; Williams and Kay, 2001; Janis et al., 2002) or ingested soil (Damuth and Janis, 2011).
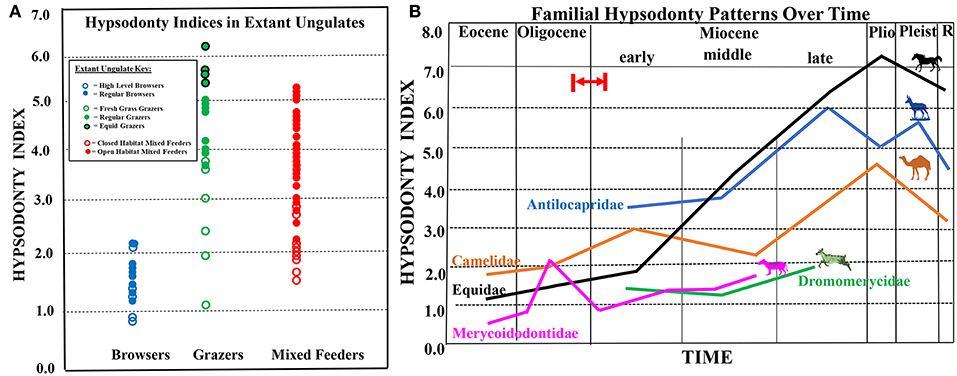
Figure 1. (A) Extant ungulate m3 hypsodonty indices (data from Janis, 1988; modified from Semprebon and Rivals, 2010). Extant ungulates are represented by circles (closed circles = regular browsers, grazers, and mixed feeders; closed circles with black outlines represent equid grazers; open circles = high-level browsers, fresh grass grazers, and closed habitat mixed feeders). (B) Composite figure showing hypsodonty trends through time for equids (black line), antilocaprids (blue line), camelids (orange line), dromomerycids (green line), and oreodonts (pink line). Red arrow and red lines indicate probable timing of first availability of grass in the North American Great Plains Region by ~22 million years ago (earliest Miocene), but possibly by even about 26 million years ago (latest Oligocene) based on phytolith data from (Strömberg, 2011). Please note that hypsodont equids will likely always have higher hypsodonty indices than ruminants on a similar diet, due to differences in digestive physiology and ingestive mastication.
Hoffman et al. (2015) examined the possibility of a “grit effect” experimentally and highlights as supporting evidence: (1) the asynchronous timing of the expansion of grasslands in North America and the acquisition of hypsodonty in Glires and ungulates (Jardine et al., 2012), (2) the coincidence of the finding of more hypsodonty and hypselodonty (ever-growing teeth) in herbivorous mammals from South America from the middle Eocene (40 Ma) to the early Miocene (20 Ma) with an interval of dry and open environments which lacked grasslands but were exposed to recurrent volcanic ashfall (Strömberg et al., 2013; Dunn et al., 2015), and (3) exogenous grit as an agent to producing microwear in ungulates like the extant Antilocapra americana, Camelus bactrianus, Camelus dromedarius, and Vicugna vicugna which live in semi-arid or arid habitats have been reported to have coarser microwear scars (i.e., coarse scratch textures, higher average pit numbers and gouging) relative to their counterparts in more humid places (Solounias and Semprebon, 2002). Hoffman et al. (2015) assessed the contribution of exogenous grit to enamel microwear by using a new technique for molding live animals representing the first ungulate controlled feeding experiment (in-vivo) using abrasives of different sizes. This study (2015) found a significant “grit effect” with medium sized silica particles (i.e., an increased abundance of pits [but not more scratches] which resulted from fracturing of sand grains through masticatory movements). Smaller particles of the fine sand treatment proved harder to break apart in this study and did not show a significant grit effect.
Jardine et al. (2012) carried out a study of crown height changes in herbivorous species (i.e., Glires and large mammals) of the Great Plains region of the United States to better understand the importance of grass vs. grit as drivers of the attainment of hypsodonty. This study focused on examining when hypsodonty evolved in these forms relative to the spread of grasslands and determining how widely distributed hypsodonty was among taxa. Jardine et al. (2012) found that the timing of the expansion of grasslands in North America was asynchronous with the timing of the acquisition of hypsodonty in Glires and ungulates. Jardine et al. (2012) also documented that many artiodactyl and perissodactyl families were exclusively low-crowned with the exception of the Antilocapridae (pronghorns), Camelidae (camels), Merycoidodontidae (oreodonts), Equidae (horses), and Rhinocerotidae (rhinoceroses) which were the only families that attained hypsodont or highly hypsodont dentitions. They also reported that these high-crowned families (except for pronghorns which appeared in the early Miocene as immigrants) have molars that change from brachydont to hypsodont or highly hypsodont and range through most of the time series. They (2012) describe: (1) a “first wave” of high-crowned taxa represented by leptauchenine oreodonts and stenomyline camelids in the Oligocene (with hypertraguline traguloids showing some degree of hypsodonty), (2) the appearance of the first highly hypsodont ungulate in the early Miocene (He1) (e.g., the latest surviving stenomyline camelids, and (3) a “second wave” of hypsodonty in the early Miocene (He1) in horses, rhinos, and pronghorns, but highly hypsodont taxa not appearing until the late middle Miocene (Barstovian) (including the first of the hypsodont later camelids).
In this study, we examine the relative contributions of grass vs. grit as a driving force in ungulate tooth evolutionary changes using a combination of dietary proxies (hypsodonty index, mesowear, and microwear) with different temporal resolution capabilities to investigate the amounts of different levels of abrasion imposed on molar teeth over evolutionary time and the potential causes of this abrasion. For example, hypsodonty is an evolutionary adaptation developed over deep time which makes a tooth more durable as an adaptation to resist augmented tooth wear incurred during mastication (Janis and Fortelius, 1988). Thus, a hypsodont tooth is not worn away as quickly because there is more tooth material present (Janis, 1988). Thus, hypsodonty is a reflection of selective pressures over time imposed by high levels of dental abrasion incurred by a species' lineage (Damuth and Janis, 2011).
Mesowear assesses gross molar wear by assessing lateral cusp shape and involves ecological time, in that, dental wear that accumulates on molars during an individual's lifespan is explored. Thus, mesowear should be sensitive to intrinsic, abrasive elements in plants but also to grit encountered on food or during the process of feeding (but does not tease apart relevant contributions of each).
Microwear examines shorter-term microscopic tooth wear imposed upon dental enamel by the last meals partaken by these animals just before dying, and as such, can elucidate daily, seasonal, or regional alterations in diet not possible with hypsodonty, mesowear or other gross craniodental methods. Importantly, microwear (Hoffman et al., 2015) shows a habitat effect (i.e., grit signature) as well as dietary food discrimination. In particular, dietary discrimination of modern ungulates using microwear is dependent upon relative numbers of scratches whereas scratch textures (i.e., widths) and degree of large pitting have been shown to track relative dietary abrasion regardless of dietary category (Semprebon, 2002; Solounias and Semprebon, 2002; Semprebon et al., 2004; Hoffman et al., 2015). Thus, browsers subsisting on relative soft foods such as leaves that occupy arid and open habitats show higher levels of pitting (especially large pits) and coarser scratch textures than leaf browsers that occupy relatively closed habitats (Semprebon, 2002). Coarser scratches are found in modern grazers that consume dry grass as opposed to fresh or moist C3 grass (and more large pits and gouges) and closed habitat and moist C3 grass mixed feeders have finer scratches and fewer large pits and gouges than open habitat mixed feeders (Semprebon, 2002). Solounias and Semprebon (2002) and Semprebon et al. (2004) have reported that those extant ungulate taxa that either encounter exogenous abrasives or consume fruit have large percentages of individuals displaying large pits in their enamel. However, frugivory produces large pits that are crater-like, deeply etched into the enamel and with very symmetrical, and well defined, and round border outlines. Exogenous abrasives produce a more superficial chipping effect on enamel where large pits are relatively shallow and with irregular borders.
The purpose of this work is twofold: (1) to examine the changing paleodiet of Eocene to Recent Quaternary equids from North America using three different dietary proxies with different temporal resolutions (hypsodonty, mesowear, and microwear) and (2) to compare this pattern to that of four North American artiodactyl families to gain insight as to the impact of differing amounts of dietary abrasion encountered by food and grit on shaping these families through time.
Materials and Methods
Data from North American equids ranging from the Eocene (early Uintan) through the Pleistocene (Rancholabrean) were obtained from (Semprebon et al., 2016) (microwear; N = 1203) and (Mihlbachler et al., 2011) (mesowear; N = 6498). These data were compared to published microwear data on extant ungulates (Solounias and Semprebon, 2002) and microwear and mesowear data from North American Miocene through Pleistocene antilocaprids (data from Semprebon and Rivals, 2007), Eocene through Pleistocene camelids (data from Semprebon and Rivals, 2010), early Miocene through late Miocene dromomerycids (microwear and mesowear data Semprebon et al., 2004), and early Miocene through late Miocene merycoidodontids (unpublished microwear and mesowear “score” data from GS; published mesowear and hypsodonty data from Mihlbachler and Solounias, 2006). Unpublished hypsodonty data was obtained for all five fossil families from Christine Janis. Details regarding taxa and localities represented may be found in the above publications. These data were evaluated to compare the paleoecology of these North American through time.
All microwear data was obtained by a single, trained observer (G.S.) using a light stereomicroscope at 35× magnification following technique regime of Semprebon (2002) and Solounias and Semprebon (2002) and Semprebon et al. (2004). The tooth and area studied were the same for all families. The average number of pits (rounded features) vs. average number of scratches (elongated features) per taxon were counted within a 0.16 mm2 area using an ocular reticle. Results were compared to an extensive extant ungulate database (Semprebon, 2002; Solounias and Semprebon, 2002) to determine browser vs. grazer dietary categories. Large pits were scored as either present (i.e., more than four large pits per microscope field) or absent (within the 0.16 mm2 area) and if gouges were present and the percentage of individuals within each taxon with these variables was calculated. Scratch textures were qualitatively recorded as being either mainly fine, mainly coarse, or a mixture of fine and coarse textural types following procedures for recognizing these textural differences outlined in Solounias and Semprebon (2002) and Semprebon et al. (2004). A scratch width score (SWS) was attained by ascribing a score of 0 to molars with mostly fine scratches, 1 to molars possessing a mix of fine and coarse textures, and 2 to those with mostly coarse scratches. An average of individual scores for a taxon was obtained to arrive at the average scratch width score. Mixed feeders were distinguished from browsers and grazers based on calculations of the percentage of raw scratches per taxon falling into a low raw scratch range of 0–17 scratches as discriminating patterns are discernible among these three extant trophic groups (Semprebon, 2002; Semprebon and Rivals, 2007).
Mesowear for all families was obtained by modifying the mesowear technique of Fortelius and Solounias (2000) which examines attritional tooth wear due to tooth-on-tooth contact wear vs. abrasional wear due to food-on-tooth contact (i.e., mesowear). As in the traditional mesowear method, mesowear data was collected by observing molar cusps macroscopically in buccal view and assessing cusp sharpness (i.e., sharp, round, blunt) and degree of occlusal relief (high or low) (Fortelius and Solounias, 2000). However, because cusp shape and occlusal relief are not independent variables and also because assigning tooth wear into mesowear categories is dependent upon an individual observer's judgment without standardization of actual boundaries defining various shape categories, mesowear was treated as a single variable and cusp apices were assigned to stages along a continuum ranging from the sharpest cusps with the highest relief to the bluntest cusps with the lowest relief comparing fossil teeth to a mesowear “ruler” (Mihlbachler et al., 2011) which was devised using seven modern Equus tooth cusps, representing a range from sharp cusps with high relief (stage 0) to blunt cusps and no relief (stage 6). Stage 7 was assigned when cusps had a negative relief (i.e., the apex of the cusp was convex). A mesowear score was determined by calculating the average mesowear value from each fossil tooth sample. With this scoring technique, higher scores reflect relatively more abrasion vs. attrition. Lower scores reflect more attrition vs. abrasion. An individual with mostly high relief and sharp cusps would have a score near 0 (e.g., low abrasion browsers), whereas, an individual with blunt cusps and low relief such as extreme grazers with high abrasion diets would have a score of 6. Other individuals would have scores falling in between these two extremes (e.g., coarser browsers, mixed feeders, and non-extreme grazers).
Extant ungulate data for hypsodonty was obtained from Janis (1988) and fossil merycoidodontids from Mihlbachler and Solounias (2006). Unpublished data for fossil equids were provided to us from Christine Janis. Hypsodonty was measured as the crown height (distance from the base of the crown to the tip of the protoconid) divided by the labio-lingual width of the third, lower and unworn molar provided the crown height ratio (m3 ratio). Molar width was measured between the protoconid and the entoconid (occlusal surface). Mihlbachler and Solounias (2006), however, used upper third molars for merycoidodontids.
Results
Figure 1A shows extant ungulate m3 hypsodonty indices (data from Janis, 1988) which will serve as a comparative framework and context for understanding fossil indices. Extant ungulate hypsodonty indices are represented by circles (for extant browsers: open circles represent high-level browsers (i.e., those browsers that invariably feed above ground level), whereas closed circles represent regular browsers (those browsers that feed both above the ground and at ground level); for extant grazers: open circles represent fresh-grass grazers, whereas closed circles represent regular grazers and closed circles with a black outline represent equid grazers; for extant mixed feeders: open circles represent closed habitat mixed feeders, whereas closed circles represent open habitat mixed feeders). Figure 1A shows that lower crown heights are found in those extant taxa that either feed less close to the ground, on fresh grass, or in closed habitats. This underscores the likely influence that exposure to grit has played on tooth crown height over time.
Figures 2, 3 and 1B show graphical representations of hypsodonty indices through time in five North American ungulate families. Figure 2A shows that North American basal Eocene equids (hyracotherines) and Eocene and Oligocene Mesohippus and Miohippus have low crowned teeth (i.e., are brachydont)—at the level of extant regular browsers (Figure 1A). A slight increase in crown height (i.e., mesodonty) is observed in the early Miocene in some parahippine-grade taxa but Merychippine-grade and “Derived Equinae” begin a relatively progressive increase in hypsodonty level in the middle Miocene (consistent with the crown heights of some closed- and open-habitat mixed feeders), the latter continuing that trend through the Pliocene and Pleistocene when they finally attain crown heights similar to extant open-habitat mixed feeders and regular grazers.
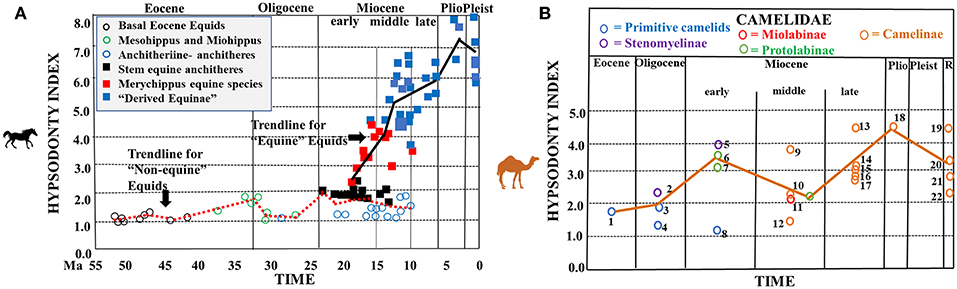
Figure 2. Hypsodonty indices of fossil equids and camelids. (A) Hypsodonty indices of North American fossil equids and Recent Old World equids (modified from Figure 2 in Damuth and Janis, 2011). Key to taxa: open circles = basal Eocene equids (Epihippus, Hyracotherium, Orohippus); Mesohippus and Miohippus, and Anchitherini; (Anchitherium, Hypohippus, Kalobatippus, Megahippus); squares = stem equine anchitheres (Archaeohippus, Desmatippus, Parahippus); Merychippus equine species; and “Derived Equinae.” Average modern (Recent) Equus hypsodonty indices from Janis (1988). The red, dotted line represents the hypsodonty index trendline for non-equine equids; the black, solid line represents the hypsodonty index trendline for equine equids (B). Hypsodonty indices of North American fossil camelids through time (brown, solid line represents the trendline through time -modified from Figure 4A in Semprebon and Rivals, 2010). Key to taxa: blue circles = primitive camelids (1 = Poebrotherium eximus, 3 = Poebrotherium wilsoni, 4 = Poabromylus kayi, 8 = Paratylopus cameloides); purple circles = Stenomyelinae (2 = Pseudolabis dakotensis, 5 = Stenomylus hitchcocki); red circles = Miolabinae (11 = Miolabis longiceps); green circles = Protolabinae (6 = Protolabis sp., 7 = Michenia sp.); orange circles = Camelinae (9 = Procamelus sp., 10 = Aepycamelus sp., 12 = Aepycamelus stocki, 13 = Procamelus occidentalis, 14 = Megatylopus gigas, 15 = Procamelus sp., 16 = Hemiauchenia sp., 17 = Aepycamelus sp., 18 = Camelops mexicanus, 19 = Lama vicugna, 20 = Lama guanicoe, 21 = Camelus bactrianus, 22 = Camelus dromedaries) Locality information for numbered taxa as in Table 1 in Semprebon and Rivals (2010). Please note that hypsodont equids will likely always have higher hypsodonty indices than ruminants on a similar diet, due to differences in digestive physiology and ingestive mastication.
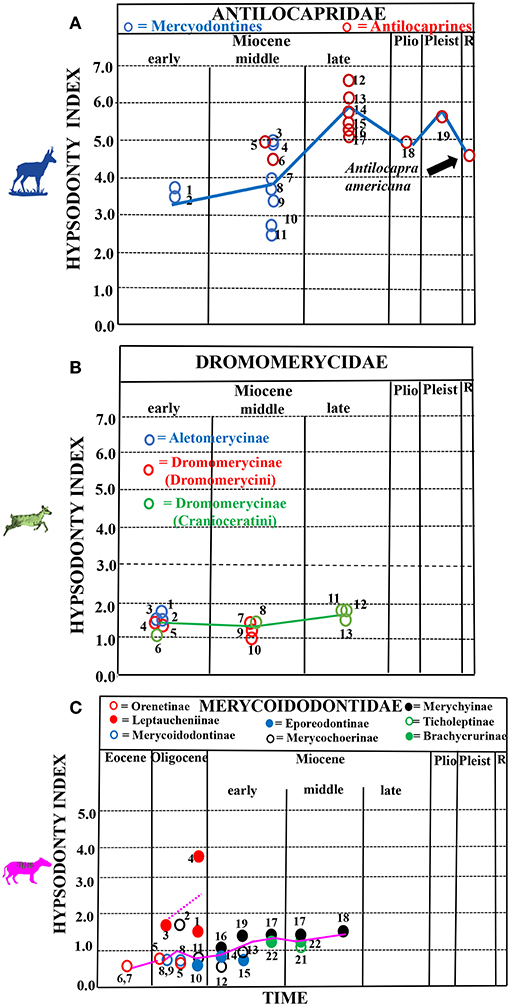
Figure 3. Hypsodonty indices of fossil pronghorns, dromomerycids and oreodonts through time. (A) North American fossil pronghorns through time. Key to taxa: blue circles = merycodontines (1 = Paracosoryx wilsoni, 2 = Paracosoryx alticornis, 3 = Cosoryx furcatus, 4 = Cosoryx cerroensis, 7 = Meryceros nenzelensis, 8 = Ramoceros ramosus, 9 = Ramoceros sp., 10 = Merycodus sabulonis, 11 = Merriamoceras coronatus); red circles = antilocaprines 12 = Hexabelomeryx sp., 13 = Ilingoceros sp., 14 = Texoceros guymonensis, 15 = Osbornoceras osborni, 16 = Plioceras dehlini, 17 = Hexabelomeryx sp., 18 = Capromeryx sp., 19 = Stockoceros sp., 20 = Antilocapra americana (Recent)]. Blue solid line = hypsodonty index trendline. Unpublished fossil data from Christine Janis; Recent data from Janis (1988). (B) Hypsodonty indices of North American fossil dromomerycids through time (Unpublished fossil data from Christine Janis). Key to taxa: blue circles = Aletomerycinae (1 = Aletomeryx marslandensis, 2 = Aletomeryx scotti, 3 = Sinclairomeryx riparius); red circles = Dromomerycinae (Dromomerycini) (4 = Subdromomeryx scotti, 5 = Barbouromeryx trigonocorneus, 7 = Dromomeryx whitfordi, 9 = Rakomeryx sinclairi, 10 = Drepanomeryx sp.); green circles = Dromomerycinae (Cranioceratini) (6 = Procranioceras skinneri, 8 = Bouromeryx sp., 11 = Pediomeryx hemphilliensis, 12 = Pediomeryx hamiltoni, 13 = Cranioceras granti). Green, solid line = hypsodonty index trendline. (C) Hypsodonty data from North American fossil oreodonts through time (modified from Figure 2 from Mihlbachler and Solounias, 2006). Key to taxa: closed red circles = Leptaucheniinae (1 = Leptauchenia major, 2 = Leptauchenia decora, 3 = Leptauchia sp., 4 = Sespia nitida); open red circles = Orenetinae (5 = Oreonetes gracilis, 6 = Oreonetes chadronensis, 7 = Merycoidodon culbertsoni, 8 = Merycoidodon major); open blue circles = Merycoidodontinae (9 = Merycoidodon bullatus); solid blue circles = Epororeodontinae (10 = Eporeodon occidentalis, 14 = Merycoides harrisonensis, 15 = Merycoides longiceps); open black circles = Merychochoerinae (11 = Merychochoerus superbus, 12 = Merychochoerus chelydra, 13 = Merychochoerus sp.); solid black circles = Merychyinae (16 = Merychyus crabilli, 17 = Merychyus relictus, 18 = Merychyus medius, 19 = Merychyus elegans; open green circles = Ticholeptinae (20 = Ticholeptis zygomaticus); solid green circles = Brachycrurinae (22 = Brachycrus laticeps. Locality information for numbered taxa as in Table 1 in Mihlbachler and Solounias, 2006). Pink, solid line = hypsodonty trendline for non-leptauchinines; dotted, pink line represents the trend for the relatively hypsodont leptauchinines.
Figure 2B shows that camels appear in the Eocene and Oligocene (e.g., Poebrotherium) with hypsodonty indices that are somewhat greater than equids from those time periods and similar to those of either extant browsers or closed-habitat mixed feeders. Crown height increased in the early Miocene (particularly in stenomylines) to the level of some extant fresh grass grazers and open habitat mixed feeders. Crown heights were lower in the middle Miocene due to the extinction of some of the more hypsodont earlier forms (e.g., stenomylines) but then were higher beginning in the late Miocene in the camelines (no Pleistocene m3 hypsodonty data were available) only to decrease slightly in the Recent. The highest hypsodonty levels were attained by the stenomylines and camelines—often approaching that of extant open-habitat mixed feeders and regular grazers.
Figure 3A shows that the cheek teeth of antilocaprids were relatively hypsodont even in the earliest immigrant forms that appear in the late early Miocene—the “merycodontines”—and more hypsodont than the early Miocene camels, dromomerycids, and equids (Figure 1B)—at the level of some extant open-habitat mixed feeders and fresh-grass grazers. Like all three other North American families studied here, pronghorns show an increase in hypsodonty level in the late Miocene (Figure 1B). Hypsodonty levels remain high through the Pleistocene but decreased in the Recent.
Figure 3B shows that dromomerycids appear in North America in the early Miocene. While the more derived and cursorial aletomerycines appear with mesodont crown heights at this time almost at the level of some parahippine-grade equid taxa, the majority of dromomerycines (Dromomerycini and early Cranioceratini) have low-crowned teeth (similar to modern browsers) from the early-middle Miocene. Crown height decreases slightly in the middle Miocene and then increases (though not significantly) in later Cranioceratini to the level of some extant closed-habitat mixed feeders until they go extinct (in the early Pliocene). Hypsodonty data may be found in Supplementary Table 1.
Figure 3C shows that Merycoidodontids (“oreodonts”) appear in North America in the Eocene (Oreonetinae and Merycoidodontinae) with low molar crown heights typical of extant regular browsers. As in fossil equids and camelids, the oreodonts begin to increase their crown height in the Oligocene [though the highly hypsodont Sepsia nitida (Leptaucheniinae) and the somewhat hypsodont Leptauchenia major (Leptaucheniinae) skew the trend curve up in Figure 3C as other merycoidodontids are not highly hypsodont at this time]. In the early Miocene, the dip in the graph (Figure 3C) is due to the extinction of the hypsodont leptauchinine clade followed by a small trend toward increasing crown height into the middle Miocene. Hypsodonty data for merycoidodontids is from Mihlbachler and Solounias (2006).
Figure 1B displays relative hypsodonty trends through time of all five North American fossil ungulate families studied. Figure 1B shows that merycoidodontids and dromomerycids never attained levels of hypsodonty approached by antilocaprids, camelids and equids with the exception of Sepsia nitida (merycoidodontid). Also, equid hypsodonty eventually (in the late Miocene) far surpasses camelids and antilocaprids and equids alone remain at the crown height level of regular grazers from the late Miocene to the Recent.
Figures 4, 5 represent familial mesowear and microwear patterns plotted along a time axis. Symbols used to represent mesowear scores specify the dietary category assigned to each taxon based on microwear analysis (circles = leaf-dominated browsers, stars = regional or seasonal mixed feeders, squares = grazers).
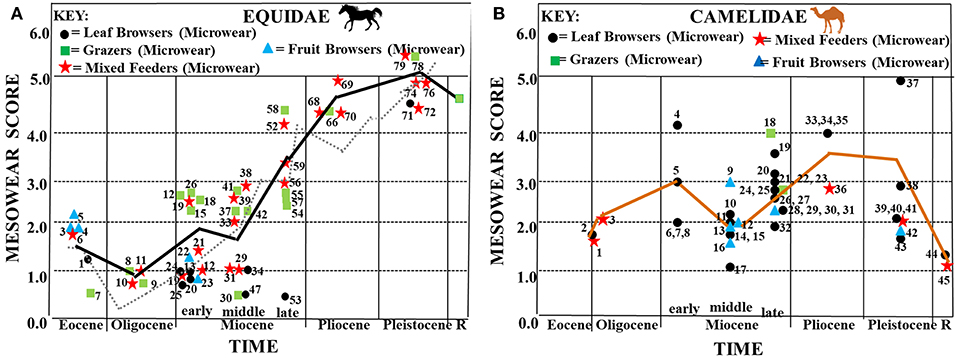
Figure 4. Synthesis of mesowear and microwear results for fossil equids (A) and camelids (B) plotted along a time axis. (A) Average mesowear scores for each equid taxon (mesowear scores from Mihlbachler et al., 2011) are shown plotted along a horizontal axis representing geological time. Symbols used for each mesowear score represent dietary assignment assessed via microwear (data from Semprebon et al., 2016). Key to microwear dietary assignment: circles = browsers, squares = grazers, stars = seasonal or regional mixed feeders. Black trend line = mesowear trend line using the same taxa from the same localities that had both mesowear and microwear data available (N = 72). Gray dotted line = mesowear trend line for those taxa with only mesowear scores available (N = 6,498). Key to taxa: 1 = Basal Eocene equid—Species A, 2 = Basal Eocene equid—Species B, 3 = Basal Eocene equid—Species C, 4 = Basal Eocene equid—Species D, 5 = Basal Eocene equid—Species E, 6 = Mesohippus sp., 7 = Miohippus obliquidens, 8 = Mesohippus bairdii, 9,11 = Mesohippus sp., 10 = Mesohippus westoni, 12, 16, 17 = Parahippus nebrascensis, 13 = Kalobatippus agatensis, 14 = Kalobatippus sp., 15, 18, 30, 31 = Parahippus sp., 19 = Parahippus leonensis, 20, 21 = Parahippus pawniensis, 22 = Archaeohippus blackbergi, 23 = Archaeohippus penultimus, 24 = Hypohippus sp., 25 = Kalobatippus sp., 26 = Merychippus primus, 27 = Acritohippus tertius, 28 = Parahippus avus, 29 = Parahippus integer, 32, 33, 53 = Hypohippus sp., 34, 35, 43 = Megahippus sp., 36 = “Merychippus” goorisi, 37 = Merychippus insignis, 38 = Scaphohippus intermontanus, 39 = Acritohippus isonesus, 40 = Acritohippus tertius, 41 = Calippus proplacidus, 42 = Protohippus perditus, 44, 45 = “Merychippus” calamarius, 46 = Cormohipparion quinni, 47 = Megahippus matthewi, 48 = Pseudhipparion retrusum, 49 = Protohippus supremus, 50 = Cormohipparion occidentale, 51, 54 = Calippus martini, 52 = Pseudhipparion hessei, 55 = Cormohipparion occidentale, 56 = Hipparion tehonense, 57, 60 = Dinohippus leidyanus, 58 = Dinohippus sp., 59 = Dinohippus interpolatus, 61 = Dinohippus sp., 62 = Nannipus aztecus, 63 = Pseudhipparion simpsoni, 64 = Neohipparion eurystyle, 65 = Cormohipparion emsliei, 66, 67 = Equus simplicidens, 69, 71, 72, 74, 78 = Equus sp., 70 = Nannipus peninsulatus, 73 = Equus (Hemionus) sp. “B,” 75 = Equus calobatus, 76 = Equus complicatus, 77 = Equus fraternus, 79 = Equus pacificus. Key to localities as in Semprebon et al. (2016) Table 1. (B) Synthesis of mesowear and microscopic microwear results for fossil camelids plotted along a time axis. Average mesowear scores for each taxon are shown plotted along a horizontal axis representing geological time. Symbols used for each mesowear score represent dietary assignment assessed via microwear (microwear and mesowear data from Semprebon and Rivals, 2010). Key for microwear dietary assignment: circles = browsers, squares = grazers, stars = seasonal or regional mixed feeders. Orange trend line = mesowear trend line using the same taxa from the same localities that had both mesowear and microwear data available (N = 45). Key: 1, 2 = Poebrotherium sp., 3 = Poebrotherium wilsoni, 4 = Stenomylus hitchcocki, 5, 9, 15, 16 = Aepycamelus sp., 6 = Michenia sp., 7, 8, 17 = Protolabis sp., 10 = Paramiolabis singularis, 11 = Aepycamelus proceras, 12, 13, 20, 26, 27, 29 = Procamelus sp., 14 = Miolabis princetonianus, 18, 19, 21, 22, 33, 34 = Megatylopus sp., 23 = Machaerocamelus sp., 24 = Procamelus occidentalis, 25, 30, 31, 32, 36 = Hemiauchenia sp., 28 = Megacamelus sp., 35 = Gigantocamelus spatula, 37, 38, 39 = Camelops sp., 40 = Hemiauchenia macrocephala, 41 = Camelops nevadanus, 42, 43 = Palaeolama mirifica, 44 = Camelus dromedarius, 45 = Lama vicugna. Key for localities as in Semprebon et al. (2016)—Table 1.
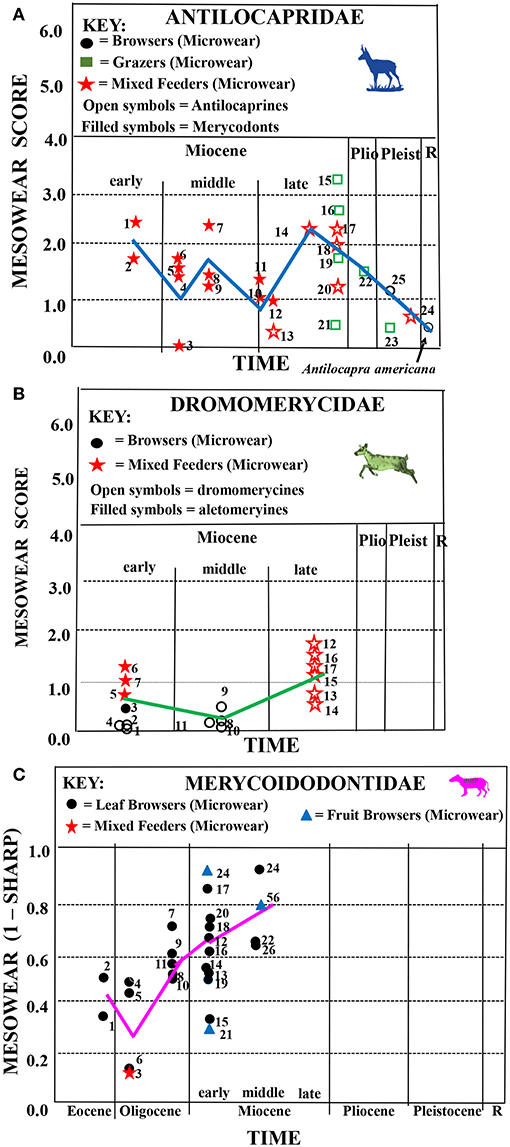
Figure 5. Synthesis of mesowear and microscopic microwear results for fossil antilocaprids, dromomerycids and merycoidodontids plotted along a time axis. (A) Antilocaprids: average mesowear scores for each taxon are shown plotted along a horizontal axis representing geological time. Symbols used for each mesowear score represent dietary assignment assessed via microwear (microwear and mesowear data from Semprebon and Rivals, 2007). Key for microwear dietary assignment: circles = browsers, squares = grazers, stars = seasonal or regional mixed feeders. Blue trend line = mesowear trend line using the same taxa from the same localities that had both mesowear and microwear data available (N = 26). Key: “Merycodontinae”−1 = Paracosoryx dawesensis, 2 = Paracosoryx wilsoni, 3, 4 = Merriamoceros coronatus, 5 = Paracosoryx alticornis, 6 = Merycodus sabulonis, 7 = Meryceros joraki, 8 = Ramoceros sp., 9 = Paracosoryx alticornis, 10, 11 = Cosoryx furcatus, 12 = Cosoryx cerroensis. Antilocaprinae—13 = Proantilocapra platycornea, 14 = Plioceros dehlini, 15 = ?Hexobelomeryx, 16 = Ilingoceros alexandrae, 17 = Osbornoceros osborni, 18 = Texoceras guymonensis, 19 = “Plioceros” texanus, 20 = cf. Sphenophalos, 21 = Ilingoceros alexandrae, 22 = Tetrameryx sp., 23 = Hayoceros falkenbachi, 24 = Stockoceros onusrosagris, 25 = Capromeryx furcifer. Key to localities as in Semprebon and Rivals (2007)—Table 2. (B) Dromomerycids: synthesis of mesowear and microscopic microwear results for fossil dromomerycids plotted along a time axis. Average mesowear scores (previously unpublished) for each taxon are shown plotted along a horizontal axis representing geological time. Symbols used for each mesowear score represent dietary assignment assessed via microwear (microwear data from Semprebon et al., 2004). Key for microwear dietary assignment: circles = browsers, squares = grazers, stars = seasonal or regional mixed feeders. Green trend line = mesowear trend line using the same taxa from the same localities that had both mesowear and microwear data available (N = 14). Key: 1 = Barbouromeryx sp., 2 = Bouromeryx pawniensis, 3 = Dromomeryx (Subdromomeryx) scotti, 4 = Procranioceras skinneri, 5 = Aletomeryx gracilis, 6 = Aletomeryx scotti, 7 = Sinclairomeryx riparius, Dromomeryx whitfordi, 9, 10 = Drepanomeryx (Matthomeryx) sp., 11 = Rakomeryx sinclairi, 12 = Cranioceras clarendonensis, 13 = Cranioceras unicornis, 14 = Pediomeryx hamiltoni, 15,16 = Pediomeryx hemphilliensis, 17 = Pediomeryx (P.) sp. (C) Merycoidodontids: synthesis of mesowear and microscopic microwear results for fossil merycoidodontids plotted along a time axis. Mesowear is represented as the proportion of cusps in a taxon that are not sharp (i.e., are rounded or even blunt) and were obtained from Mihlbachler and Solounias (2006) and derived in this study be subtracting the proportion of sharp cusps from 1.00. Mesowear for each taxon is plotted along a horizontal axis representing geological time. Symbols represent dietary assignment assessed via microwear (unpublished microwear data from GS). Key for microwear dietary assignment: circles = browsers, squares = grazers, stars = seasonal or regional mixed feeders. Pink trend line = mesowear trend line using the same taxa from the same localities that had both mesowear and microwear data available (N = 26). Key: 1 = Orenetes chadronensis (Oreonetinae), 2 = Merycoidodon culbertsoni (Merycoidodontinae), 3 = Leptauchenia sp. (Leptaucheniinae) (L. decora), 4 = Merycoidodon bullatus (Merycodoidodontinae), 5 = Merycoidodon major (Merycodoidodontinae), 6 = Orenetes gracilis (Oreonetinae), 7 = Sepsia nitida (Leptaucheniinae), 8 = Leptauchenia major, (Leptaucheniinae), 9 = Eporeodon occidentalis (Eporeodontinae), 10 = Merycochoerus superbus superbus (Merycochoerinae), 11 = Merycochoerus superbus (Merycochoerinae), 12 = Hypsiops breviceps breviceps (Merycochoerinae), 13 = Merychyrus crabilli (Merychyinae), 14 = Merycoides harrisonensis harrisonensis (Eporeodontinae), 15 = Merycochoerus chelydra carrikeri (Merycochoerinae), 16, 17 Merychyus elegans arenarum (Merychyinae), 18 = Merychochoerus sp. (Merycochoerinae), 19 = Merychyus sp. (Merychyinae), 20 = Merychyus relictus (Merychyinae), 21 = Brachycrus laticeps buwaldi (Brachycrurinae), 22 = Merychyus relictus (Merychyinae), 23 = Brachycrus laticeps buwaldi (Brachycrurinae), 24 = Ticholeptus zygomaticus (Ticholeptinae), 25 = Brachycrus laticeps siouense (Brachycrurinae), 26 = Merychyus medius medius (Merychyinae).
Equidae
Mesowear
Figure 4A shows that basal equids start off in the Eocene with slightly rounded cusps, but cusp apices gain in sharpness as the Eocene progresses. Mesowear values were lowest in the earliest Oligocene, suggesting very low abrasion feeding behavior at that time although this is followed by a change in the mesowear trend toward increased abrasion—however, abrasion is still fairly low. In the early Miocene, there is an increase in abrasion (higher mesowear values) and this increased abrasion sustained the progression toward a higher level of abrasion which started after the Eocene–Oligocene Transition. This trend toward greater abrasion continued into the Pliocene and Pleistocene. These mesowear results are mostly consistent with microwear dietary assignments. However, Miohippus obliquidens (Eocene), Mesohippus bairdii (Oligocene), Mesohippus sp. (Oligocene) and Kalobatippus sp. (middle Miocene) have unimodal, high scratch counts typical of extant grazers yet low mesowear scores typical of relatively low abrasion. When equid mesowear is compared to that of the other ungulate families studied (Figure 6), it is apparent that of those taxa that survived beyond the Miocene (i.e., equids, camelids, and antilocaprids), only the equids continued the trend toward higher dietary abrasion into the Pleistocene and Recent.
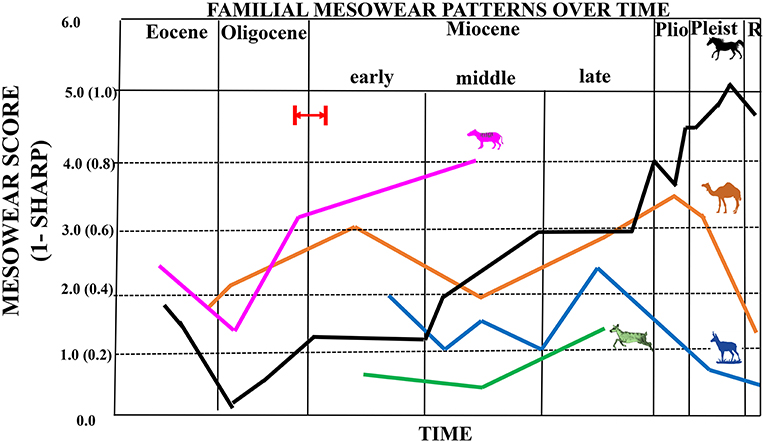
Figure 6. Composite figure showing mesowear trends through time for equids (black line), antilocaprids (blue line), camelids (orange line), dromomerycids (green line), and oreodonts (pink line). Equid, camelid, antilocaprid, and dromomerycid trends reflect a graphical display of average mesowear scores (scores that combine cusp shape with occlusal relief) for taxa in a particular time period (0–6); merycoidodontid trend reflects cusp shape only (i.e., the proportion of cusps that are not sharp, 0–1.0). Red arrow and red lines indicate probable timing of first availability of grass in the North American Great Plains Region by ~22 million years ago (earliest Miocene), but possibly by even about 26 million years ago (latest Oligocene) based on phytolith data from Strömberg (2011).
Microwear
The most basal early Eocene equids have microwear consistent with frugivory (e.g., many large, symmetrical puncture-like pits, scratch textures coarser than extant leaf browsers, as well as somewhat rounded gross cusp morphology) but a shift toward more leaf browsing and relatively fine scratch textures (Figure 7) (and sharper cusps) in the middle Eocene correlates with mesowear which shows less of a degree of dietary abrasion incurred toward the approach of the Eocene-Oligocene boundary. Microwear results in the early Miocene are consistent with the mesowear trend toward more abrasion at this time [i.e., scratch textures became coarser and gouging and large pitting in dental enamel increased (Figure 8)—even in browsing forms]. Microwear also shows grazing or mixed feeding in parahippine-grade taxa at this time which would add to the increased abrasion trend.
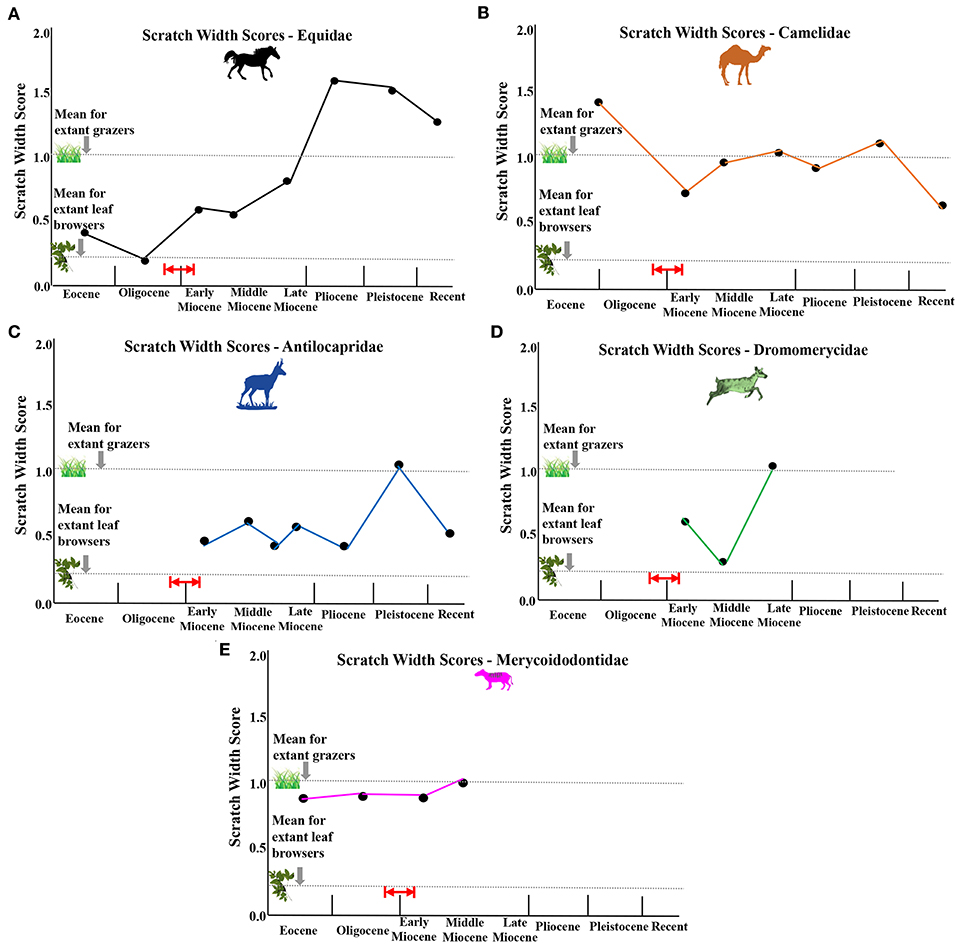
Figure 7. Average scratch width scores (sws) for each fossil family plotted over evolutionary time. (A) equids, (B) camelids, (C) antilocaprids, (D) dromomerycids, (E) merycoidodontids. Red arrow and red lines indicate probable timing of first availability of grass in the North American Great Plains Region by ~22 million years ago (earliest Miocene), but possibly by even about 26 million years ago (latest Oligocene) based on phytolith data from Strömberg (2011).
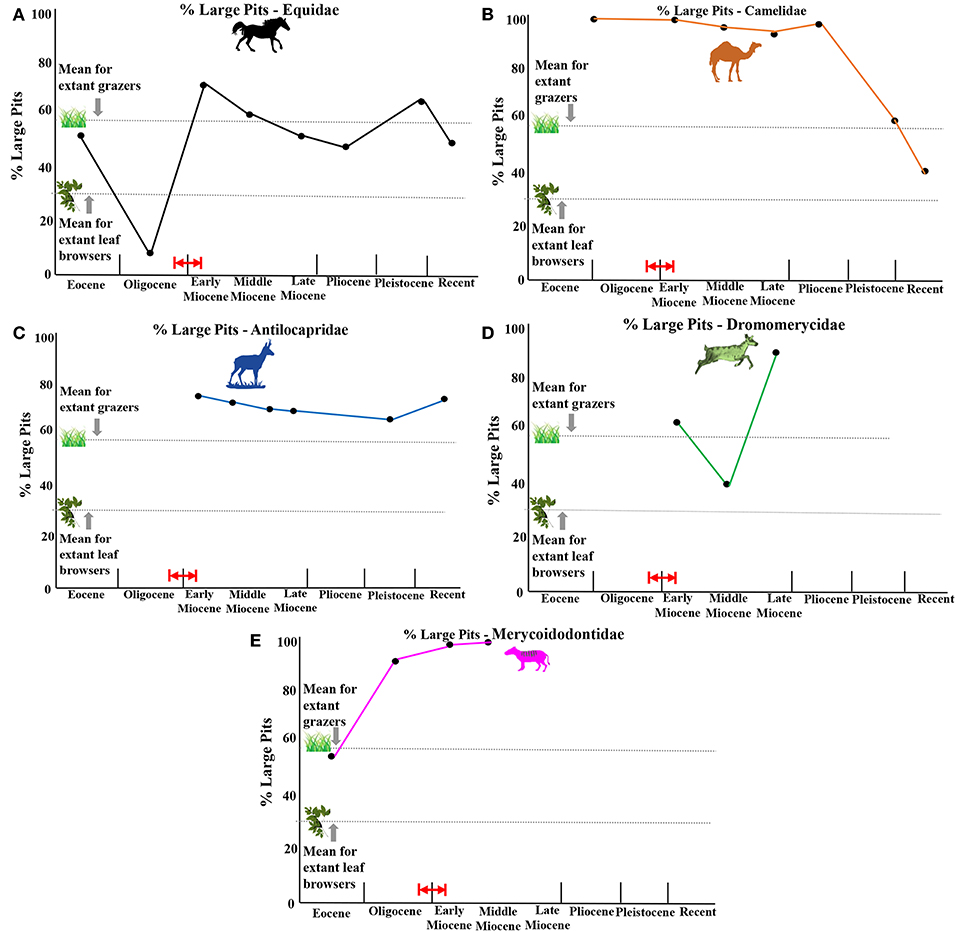
Figure 8. Average percentages of individuals per taxa in each time period that display large enamel pits (averages for extant ungulates from Semprebon, 2002). (A) equids, (B) camelids, (C) antilocaprids, (D) dromomerycids, (E) merycoidodontids. Red arrow and red lines indicate probable timing of first availability of grass in the North American Great Plains Region by ~22 million years ago (earliest Miocene), but possibly by even about 26 million years ago (latest Oligocene) based on phytolith data from Strömberg (2011).
Unlike the camels, pronghorn, and dromomerycids (Figure 6), equid mesowear continued the trend in the middle Miocene toward greater abrasion that began after the Eocene-Oligocene Transition. The percentages of large pits in enamel (Figure 8) remained at a level seen in most extant grazers from the early Miocene onward. Most of this greater abrasion seen in the middle Miocene was due to the dietary patterns of the derived Equinae which were mostly grazing on more abrasive grasses with coarser scratch textures (Figure 7), whereas the merychippine-grade taxa were mostly mixed feeders on relatively low abrasion grasses and the Anchitherinae sensu stricto (e.g., Archaeohippus, Hypohippus, Kalobatippus, and Megahippus) had low abrasion browsing patterns. In the late Miocene, the relatively rare Anchitherinae sensu stricto continued their low abrasion browsing, while the derived Equinae continued coarser mixed-feeding or grazing and showed more pitting in dental enamel compared to the middle Miocene. This trend toward feeding on a coarser type of food persisted into the Pliocene as microwear shows a continuation of grazing as well as mixed feeding although total pit counts were higher than at any other time and scratch textures (Figure 7) were generally coarser. These results concur with the increased mesowear scores (higher abrasion) observed in mesowear at this time.
The trend toward higher microwear pit counts, that began in the late Miocene and Pliocene continued into the Pleistocene, with the greatest amount of pitting in Pleistocene species which is concordant with the high abrasion patterns seen in mesowear at this time although most Equus taxa exhibited dietary flexibility—alternating between browse and grass and scratch textures diminished slightly (Figure 7) and pitting of enamel surfaces decreased in the Recent.
Camelidae
Mesowear
Figure 4B shows increasing mesowear scores (a rise in abrasion) from the late Eocene/early Oligocene to the early Miocene. Camelid dietary abrasion decreased in the middle Miocene but then changed to more abrasion through the late Miocene, Pliocene and most of the Pleistocene. In the late Pleistocene, a large shift toward less abrasion occurred that continued into the Recent. When camelids are compared to the other ungulate fossil families studied (Figure 6), the relatively high abrasion pattern begun in the late Miocene (which is equivalent to many extant mixed feeders and grazers) is reversed toward relatively low abrasion in the later Pleistocene into the Recent (unlike the pattern in equids).
Microwear
Unlike equids, that begin the Eocene as browsers in closed habitats, late Eocene-early Oligocene camels show microwear evidence of some mixed feeding and significantly more pitting. This is congruent with camelids occupying relatively more open habitats than the equids at this time, a pattern consistent with the much longer legs and higher mesowear scores found in early camels than in the other families studied here. Scratch textures in the Eocene-Oligocene camelids are also relatively coarse (Figure 7) contributing to the relatively high abrasion indicated by heavy pitting and fairly high mesowear scores.
Early Miocene fossil camels have microwear results indicating browsing but with heavy enamel pitting consistent with the higher mesowear scores at this time (especially Stenomylus hitchcocki). Scratch textures (Figure 7) become finer (decreased scratch widths) in the early Miocene which is consistent with a switch from some grass consumption in the Eocene/Oligocene to pure browsing in the Early Miocene. A somewhat lower abrasion browsing pattern (fewer pits overall) is seen in the middle Miocene which correlates with the decrease in mesowear seen then—although scratch textures increase slightly (Figure 7). The increased mesowear scores in the late Miocene are accompanied by microwear results indicating a shift toward some mixed feeding and occasional grazing in addition to browsing with heavy pitting of enamel. The relatively high mesowear scores in the Pliocene and Pleistocene camels match the heavy enamel pitting found in the browsing, mixed feeding and grazing taxa from this time period as well as an increase in scratch widths (Figure 7). The modern camelids (Recent) have lower mesowear scores than the fossil camelids even though hypsodonty levels are different (i.e., Lama vicugna has more hypsodont dentition than Camelus dromedarius but both have similar mesowear scores), suggesting that fossil camels have a more abrasive diet than the modern camels studied. Scratch widths (Figure 7) also decrease in the Recent further substantiating that modern camels encounter less abrasion in their diets than fossil forms. Interestingly, camelids display many individuals in each taxon with large pits in their enamel (Figure 8) for most of their evolutionary history only declining in the Pleistocene and Recent even though most of them have microwear patterns similar to modern dirty browsers (i.e., a grit effect).
Antilocapridae
Mesowear
Figure 5A shows that antilocaprids begin the Miocene with somewhat rounded cusps and then shift toward higher mesowear scores indicating more abrasive diets starting in the late Hemphillian (late Miocene–Pliocene). After this, they reverse toward a less abrasive diet starting in the Pliocene and continuing through the Pleistocene into the Recent. Antilocaprids (like camelids) had relatively long legs and were rather hypsodont when they first appeared and were well-suited to exploit open habitats. When antilocaprids are compared to the other fossil ungulate families studied here (Figure 6), it is apparent that they followed a similar pattern to that seen in fossil camelids in terms of reducing overall abrasion as they moved into the Recent [although pronghorns apparently reduced their level of abrasion a bit earlier (i.e., in the Pliocene)] than camelids.
Microwear
Like the camelids but unlike equids, the earliest and more primitive merycodonts have microwear results consistent with browsing and grazing on a seasonal or regional basis but with far less pitting overall than camelids and finer scratch textures (narrower scratches) (Figure 7) which explains the relatively low mesowear scores in antilocaprids (less abrasion) at this time. Like the other families studied here, overall abrasion increased (Figure 6) in the late Miocene (i.e., mesowear) when the more derived antilocaprines apparently engaged in more grass consumption although scratch textures (Figure 7) indicate a diet that was not highly abrasive. As seen in the camelids and dromomerycids (but not in equids), antilocaprids decreased dietary abrasion (Figure 6) in the Pliocene and Pleistocene into the Recent (the modern Antilocapra americana eats mostly low-level browse in an open habitat). This decrease in abrasion is consistent with the decrease in scratch widths in the Recent seen in Figure 7. Interestingly, like camelids, antilocaprids have relatively large numbers of individuals per taxon that display large pits in their enamel (Figure 8), about the level of extant grazers, for most of their evolutionary history regardless of dietary assignment via microwear.
Dromomerycidae
Mesowear
Figure 5B shows that the dromomerycids have relatively low abrasion mesowear scores in the early Miocene which dip down further in the middle Miocene and then begin to climb in the late Miocene to the level of some extant mixed feeders. Dromomerycids experienced the lowest levels of abrasion throughout their evolutionary history when compared to abrasion levels (mesowear) in the other families studied here (Figure 6).
Microwear
The early Miocene low abrasion mesowear results parallel microwear results as the earliest dromomerycids (Dromomerycinae—Dromomerycini) have microwear similar to fine browsers [i.e., many finely-textured scratches and relatively few pits (including little or no large pits)]. In the late early Miocene, the Aletomerycinae appear with limb proportions suggestive that they occupied open/ecotonal habitats and microwear indicating seasonal or regional mixed feeding. Aletomyerycinae start off in the late early Miocene with fairly high scratch textures. The relatively large percentages of individuals displaying large pits (Figure 8) in the late early Miocene are confined to the more open country and mesodont Aletomerycinae. From the early-middle Miocene, the majority of dromomerycids were low abrasion browsers (scratches were also relatively fine and narrow—Figure 7) but in the late Miocene, increased mesowear scores are mirrored by the more derived Cranioceratini (subfamily Dromomerycinae) which show evidence of alternating between browsing and grazing but with many individuals displaying large pits (Figure 8) and a prodigious level of enamel surface gouging as well many coarse scratches (Figure 7) compared to what is typically found in extant mixed feeders (i.e., a grit effect).
Merycoidodontidae
Mesowear
Figure 5C shows mesowear trends through time in fossil merycoidodontids. Mesowear was obtained from Mihlbachler and Solounias, 2006. In this study, mesowear was graphically portrayed as the inverse proportion of sharp cusp apices (1-sharp) rather than via comparison with the mesowear ruler which gives a mesowear score that is a combination of cusp shape and occlusal relief. Even so, higher values represent more abrasion wear and can be used to demonstrate changes in abrasion over evolutionary time. Figure 5C demonstrates that early merycoidodontids (Eocene and early Oligocene) display low abrasion (similar to extant browsers) but this trend reverses beginning in the late Oligocene and continues as an increasing abrasion trend into the middle Miocene with some taxa showing abrasion similar to that of extant mixed feeders and some non-extreme grazers. It is not possible to compare merycoidodontid mesowear in terms of magnitude of abrasion to other fossil ungulate families studied herein (Figure 6) because mesowear scoring systems were somewhat different although overall relative similarities and differences in trends can be discerned.
Microwear
Figure 5C shows that merycoidodontids relied mainly on a browsing dietary strategy throughout their evolutionary time. Even so, scratch textures (Figure 7) and percentages of individuals displaying large pits in their enamel (Figure 8) are well above typical modern leaf browsers. The higher crowned Leptauchiniinae (Figure 3C) are interesting in that Leptauchenia decora (early Oligocene) apparently engaged in seasonal or regional mixed feeding while the highly hypsodont Sepsia nitida apparently browsed (late Oligocene). Miocene merycoidodontids (most taxa) have microwear patterns that demonstrate committed leaf browsing or an alteration between leaves and fruit (Brachycrus species).
Figure 8 depicts a summary of the average percentage of large pits found in the enamel of the five fossil ungulate families relative to each other through evolutionary time shown in relation to the mean score for extant browsers (typically with low percentages of large pits) and extant grazers (typically with higher percentages of large pits). A preponderance of large pits reflects either an increased amount of fruit and/or seed consumption (puncture-like, symmetrical large pits) or an increased exposure to grit coating food substances—the latter presumably due to feeding close to the ground (Solounias and Semprebon, 2002). Equidae (Figure 8A) begin the Eocene with increased large pits due mostly to frugivory (see Figure 4A) and then decrease the amount toward the Eocene-Oligocene transition as more leaf browsing and low abrasion grass consumption is apparent. During the early Miocene, large pitting increases to the level of extant ungulate grazers and remains at this relatively high level until the recent (grit effect).
The Camelidae (Figure 8B) display very high levels of large pits from the Eocene through the Pliocene (above the mean of extant grazers even though most of them are clearly browsing) and then show a rather precipitous decline. Antilocaprids (Figure 8C) show a very consistent level of large pitting (above the level of extant grazers—even though most of them are mixed feeding and eventually browsing). The dromomerycid large pit mean percentage is high in the early Miocene but this is mostly skewed high due to the large percentages of large pits found in the more open habitat, mixed feeding aletomerycines. The majority of dromomerycines (Figure 8D) (browsers) in the early Miocene have relatively low numbers of large pits. What is striking is the extreme level of large pitting encountered in the enamel of the late Miocene Cranioceratini before they go extinct in the Pliocene. The merycoidodontines (Figure 8E) show large percentages of individuals with large pits within taxa on average from their beginning in the Eocene and increasing to levels comparable to what is seen in the camelids.
Figures 9–13 summarize microwear average scratch vs. pit results for each family by plotting each family in the same time period and arranging results in chronologic order (data are shown in Supplementary Tables 1,2). Data in Figures 9–13 are plotted using Gaussian confidence ellipses (p = 0.95) on the centroid as a reference for extant browser (B) and grazer (G) data which were also adjusted by sample size. Data are from Semprebon (2002) and Solounias and Semprebon (2002). Dietary assignments given to mixed feeders which may fall within the browsing average scratch/pit ecospace (browse-dominant mixed feeders), grazing ecospace (grass-dominant mixed feeders), or in the gap between browsers and grazers (fairly equal browsing and grazing behavior) are based on raw scratch distributions as described in the relevant publications noted in figure captions from which data were obtained. The designation of fruit browser was based on percentages of large pits, puncture-like large pits, and coarser scratch textures than leaf browsers (see relevant publications noted in figure captions for details). Figure 9 shows average pit vs. average scratch numbers for Eocene-Oligocene fossil equids, camelids, and merycoidodontids. Most fossil equids (Figure 9A) apparently engaged in both leaf and fruit browsing but Miohippus obliquidens, Mesohippus bairdii, and Mesohippus sp. displays unimodal and high scratch results (but finely textured unlike modern grazers) which is incongruent with the gross morphology of their teeth, their mesowear, and low overall rate of wear and may thus possibly reflect an abrasive element in their diet other than grass. Camelids and merycoidodontids were engaging in an unusual type of browsing and mixed feeding – exceptionally high pitting characteristic of open habitat “dirty” browsers and mixed feeders (i.e., significant grit effect).
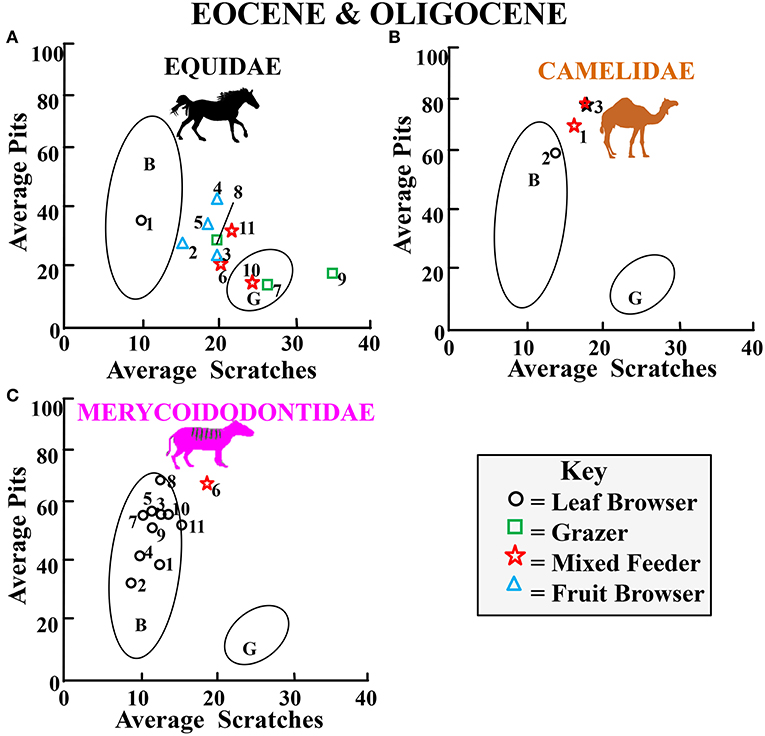
Figure 9. Bivariate plot showing results for the average number of pits vs. the average number of scratches per taxon for Eocene and Oligocene fossil equids (A), camelids (B), and merycoidodontids (C) plotted in reference to extant leaf dominated ungulate browsers (B), and extant grazers (G) at 35 times magnification (extant comparative data from Semprebon, 2002 and Solounias and Semprebon, 2002). Gaussian confidence ellipses (p = 0.95) on the centroid are indicated for the extant leaf browsers and grazers (convex hulls) adjusted by sample size. Key to Equidae: (as in Figures 3, 4) Semprebon et al. (2016)−1 = Species A, 2 = Species B, 3 = Species C, 4 = Species D, 5 = Species E, 6 = Mesohippus sp., 7 = Miohippus obliquidens, 8 = Mesohippus bairdii, 9 = Mesohippus sp., 10 = Mesohippus westoni, 11 = Mesohippus sp. Key to Camelidae: 1,2 = Poebrotherium sp., 3 = Poebrotherium wilsoni. Key to Merycoidodontidae: 1 = Orenetes gracilis chadronensis, 2 = Merycoidodon culbertsoni, 3 = Merycoidodon bullatus, 4 = Orenetes gracilis, 5 = Merycoidodon major, 6 = Leptauchenia decora, 7 = Sepsia nitida, 8 = Leptauchenia major, 9 = Eporeodon occidentalis, 10 = Merycochoerus superbus superbus, 11 = Merycochoerus superbus.
For most time periods, the four families occupied mostly disparate niches with the exception of the late Miocene when all four families had a number of taxa engaging in varying levels of grass consumption—although the camelids (Figure 9B) were engaging in mainly browsing at this time as they apparently did for most of their evolutionary history. The camelids also were committed “dirty browsers” through most of their evolution—showing relatively extremely high total pit counts consistent with exposure to grit. None of the relatively low-crowned dromomerycids (Figures 10B, 11D, 12D) were pure grazers at any time in their past whereas the other families that had mesodont and hypsodont representatives engaged in grazing or alternating between grass and browse. In addition, equids appear to have consistently showed the greatest overall flexibility in their dietary behavior. Contrary to what is often assumed, Plio-Pleistocene equids were not restricted in their dietary regimes at this time but show a level of dietary breadth that may reflect more seasonal variation in diet.
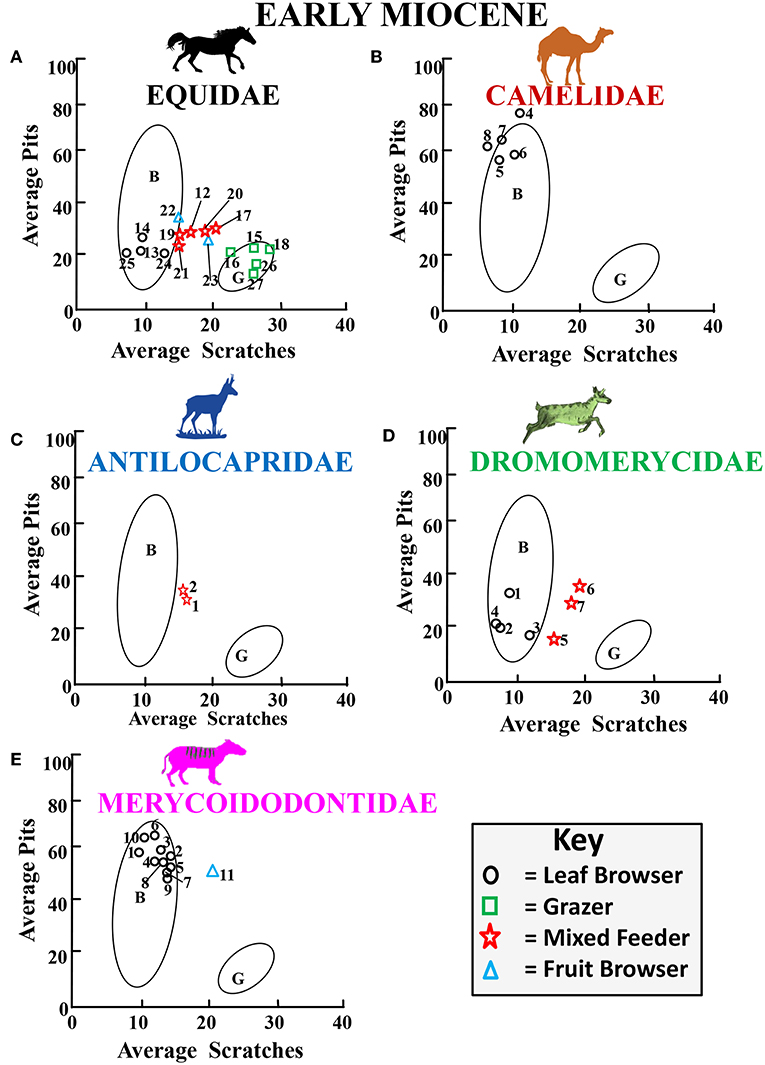
Figure 10. Bivariate plot showing results for the average number of pits vs. the average number of scratches per taxon for Early Miocene fossil equids (A), camelids (B), antilocaprids (C), dromomerycids (D) and merycoidodontids (E) plotted in reference to extant leaf dominated ungulate browsers (B), and extant grazers (G) at 35 times magnification (extant comparative data from Semprebon, 2002; Solounias and Semprebon, 2002). Gaussian confidence ellipses (p = 0.95) on the centroid are indicated for the extant leaf browsers and grazers (convex hulls) adjusted by sample size. Key to Equidae: 12,16, 17 = Parahippus nebrascensis, 13 = Kalobatippus agatensis, 14, 25 = Kalobatippus sp., 15, 18 = Parahippus sp., 19, 21 = Parahippus leonensis, 20 = Parahippus pawniensis, 22 = Archaeohippus blackbergi, 23 = Archaeohippus penultimus, 24 = Hypohippus sp., 26 = Merychippus primus, 27 = Acritohippus tertius. Key to Camelidae: 4 = Stenomylus hitchcocki, 5 = Aepycamelus sp., 6 = Michenia sp., 7, 8 = Protolabis sp. Key to Antilocapridae: 1 = Paracosoryx dawsensis, 2 = Paracosoryx wilsoni. Key to Dromomerycidae: 1 = Barbouromeryx sp., 2 = Bouromeryx pawniensis, 3 = Dromomeryx (Subdromomeryx) scotti, 4 = Procranioceras skinneri, 5 = Aletomeryx gracilis, 6 = Aletomeryx scotti, 7 = Sinclairomeryx riparius. Key to Merycoidodontidae: 1 = Hypsiops breviceps breviceps, 2 = Merychyus crabilli, 3 = Merycochoerus chelydra carrikeri, 4 = Merycoides harrisonensis harrisonensis, 5 = Merychyus elegans arenarum, 6 = Merychyus elegans minimus, 7 = Merychyus elegans elegans, 8 = Merychochoerus sp., 9 = Merychyus sp., 10 = Merychyus relictus, 11 = Brachycrus laticeps buwaldi.
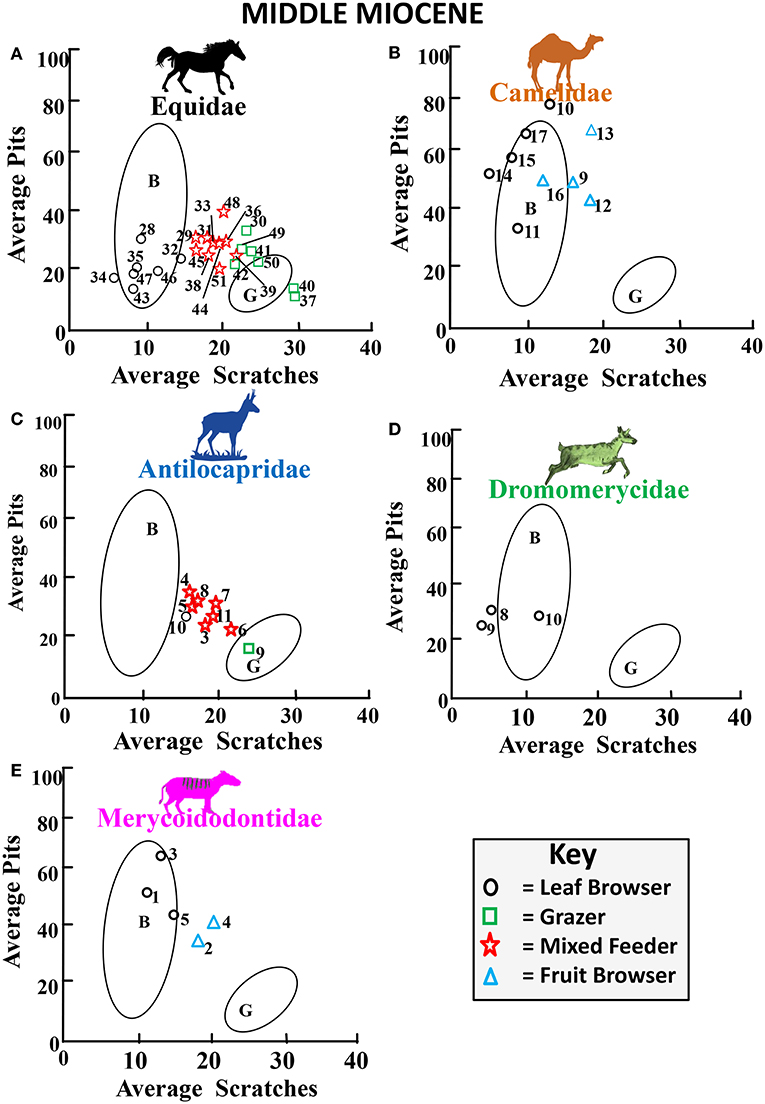
Figure 11. Bivariate plot showing results for the average number of pits vs. the average number of scratches per taxon for Middle Miocene fossil equids (A), camelids (B), antilocaprids (C), dromomerycids (D), and merycoidodontids (E) plotted in reference to extant leaf dominated ungulate browsers (B), and extant grazers (G) at 35 times magnification (extant comparative data from Semprebon, 2002 and Solounias and Semprebon, 2002). Gaussian confidence ellipses (p = 0.95) on the centroid are indicated for the extant leaf browsers (B) and grazers (G) (convex hulls) adjusted by sample size. Key to Equidae: 28 = Parahippus avus, 29 = Parahippus integer, 30, 31 = Parahippus sp., 32, 33 = Hypohippus sp., 34, 35, 43 = Megahippus sp., 36 = “Merychippus” goorisi, 37 = Merychippus insignis, 38 = Scaphohippus intermontanus, 39 = Acritohippus isonesus, 40 = Acritohippus tertius, 41 = Calippus proplacidus, 42 = Protohippus perditus, 44, 45 = “Merychippus” calamarius, 46 = Cormohipparion quinni, 47 = Megahippus matthewi, 48 = Pseudhipparion retrusum, 49 = Protohippus supremus, 50 = Cormohipparion occidentale, 51 = Calippus martini. Key to Camelidae: 9, 15, 16 = Aepycamelus sp., 10 = Paramiolabis singularis, 11 = Aepycamelus proceras, 12, 13 = Procamelus sp., 14 = Miolabis princetonianus, 17 = Protolabis sp. Key to Antilocapridae: 3, 4 = Merriamoceros coronatus, 5 = Paracosoryx alticornis, 6 = Merycodus sabulonis, 7 = Meryceros joraki, 8 = Ramoceros sp., 9 = Paracosoryx alticornis, 10, 11 = Cosoryx furcatus. Key to Dromomerycidae: 8 = Dromomeryx whitfordi, 9, 10 = Drepanomeryx (Matthomeryx) sp. Key to Merycoidodontidae: 1 = Merychyus relictus, 2 = Brachycrus laticeps buwaldi, 3 = Ticholeptus zygomaticus, 4 = Brachycrus laticeps siouense, 5 = Merychyus medius medius.
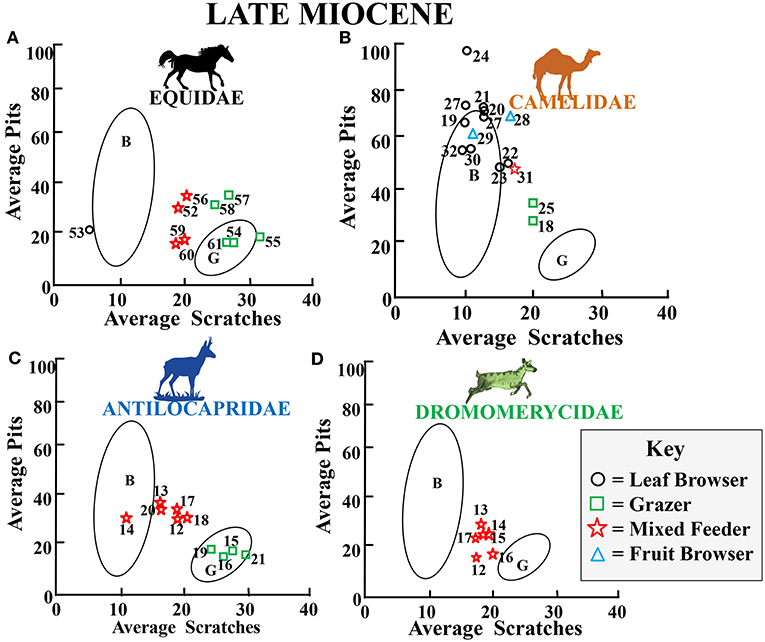
Figure 12. Bivariate plot showing results for the average number of pits vs. the average number of scratches per taxon for Late Miocene fossil equids (A), camelids (B), antilocaprids (C), and dromomerycids (D) plotted in reference to extant leaf dominated ungulate browsers (B), and extant grazers (G) at 35 times magnification (extant comparative data from Semprebon, 2002 and Solounias and Semprebon, 2002). Gaussian confidence ellipses (p = 0.95) on the centroid are indicated for the extant leaf browsers (B) and grazers (G) (convex hulls) adjusted by sample size. Key to Equidae: 52 = Pseudhipparion hessei, 53 = Hypohippus sp., 54 = Calippus martini, 55 = Cormohipparion occidentale, 56 = Hipparion tehonense, 57, 60 = Dinohippus leidyanus, 58, 61 = Dinohippus sp. 59 = Dinohippus interpolatus. Key to Camelidae: 18, 19, 21, 22 Megatylopus sp., 20, 27, 29 = Procamelus sp., 23 = Machaerocamelus sp., 24 = Procamelus occidentalis, 25, 30, 31, 32 = Hemiauchenia sp., 28 = Megacamelus sp. Key to Antilocapridae: 14 = Plioceros dehlini, 13 = Proantilocapra platycornea, 12 = Cosoryx cerroensis, 15 = ?Hexobelomeryx sp., 16, 21 = Ilingoceros alexandrae, 17 = Osbornoceros osborni, 18 = Texoceras guymonensis 19 = “Plioceros” texanus, 20 = cf. Sphenophalos. Key to Dromomeryidae: 12 = Cranioceras clarendonesis, 13 = Cranioceras unicornis, 14 = Pediomeryx hamiltoni, 15 = Pediomeryx hemphilliensis (Guymon Area), 16 = Pediomeryx hemphilliensis (Coffee Ranch), 17 = Pediomeryx sp.
Figure 10 shows average pit vs. average scratch numbers for early Miocene fossil equids, camelids, antilocaprids, dromomerycids, and merycoidodontids. Once again, equids (Figure 10A) show a diverse array of dietary behavior with some forms engaging in leaf browsing, some in fruit browsing, some alternating between browse and grass, and some grazing. Camelids (Figure 10B) and merycoidodontids (Figure 10D) continue mainly dirty browsing while antilocaprids (Figure 10C) are mixed feeders and dromomerycids (Figure 10D) are either leaf browsers or mixed feeders.
Figure 11 shows comparative average pit vs. average scratch numbers for middle Miocene fossil families. The equids (Figure 11A) show fairly balanced numbers of browsers, mixed feeders and grazers, while the camelids (Figure 11B) and merycoidodontids (Figure 11E) continue dirty browsing and some fruit browsing. Dromomerycids (Figure 11D) concentrated on pure browsing at this time, while antilocaprids were mostly alternating between browse and mixed feeding but also engaging in limited browsing and grazing.
Figure 12 shows average pit vs. average scratch numbers for late Miocene fossil families. All four families depicted in Figure 12 show shifts toward more grass consumption than what was seen earlier in the Miocene. Only the camelids (Figure 12B) continue with mostly dedicated browsing (although high abrasion, dirty browsing). Equid (Figure 12A) mixed feeders are now closer to the grazing ecospace indicating more grass-dominated mixed feeding than antilocaprids (Figure 12C) or dromomerycids (Figure 12D). Also, equids and antilocaprid mixed feeders and equid mixed feeders and grazers show more pitting than earlier in the Miocene, indicating exposure to more grit during feeding.
Figure 13 shows average pit vs. average scratch numbers for Pliocene and Pleistocene fossil families. In the Pliocene and Pleistocene, there is a continuation of relatively high abrasion (i.e., heavy pitting) mixed feeding and grazing in equids (Figures 13A,D), high abrasion browsing and grazing in camelids (Figures 13B,E) and relatively lower abrasion browsing and grazing in antilocaprids (Figures 13C,D).
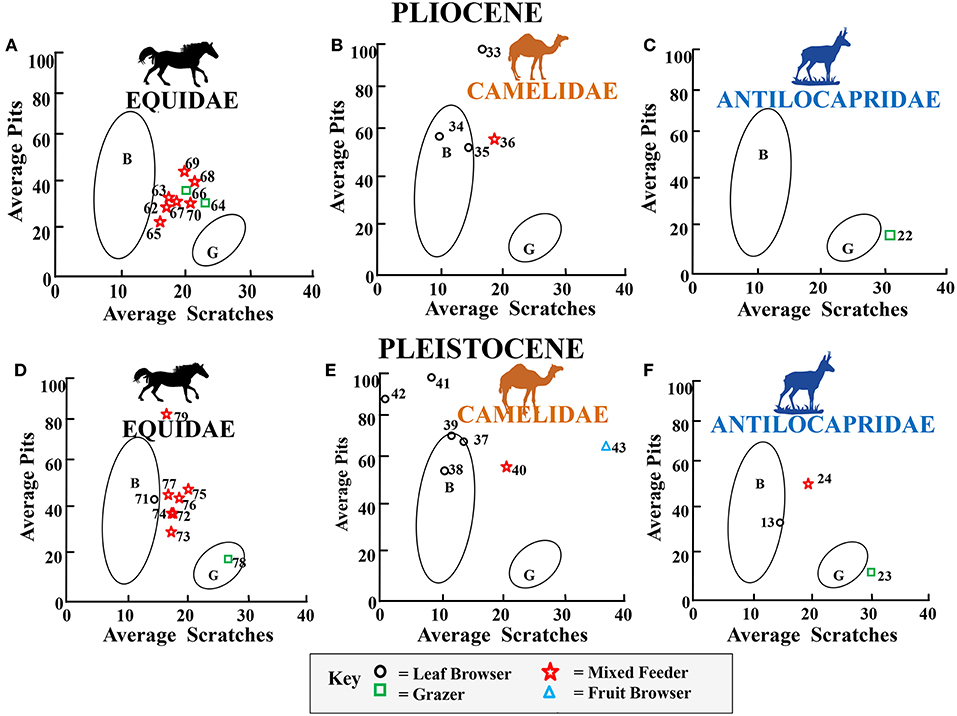
Figure 13. Bivariate Plot showing results for the average number of pits vs. the average number of scratches per taxon for Pliocene and Pleistocene fossil equids (A,D), camelids (B,E), and antilocaprids (C,F) plotted in reference to extant leaf dominated ungulate browsers (B), and extant grazers (G) at 35 times magnification (extant comparative data from Semprebon, 2002 and Solounias and Semprebon, 2002). Gaussian confidence ellipses (p = 0.95) on the centroid are indicated for the extant leaf browsers (B) and grazers (G) (convex hulls) adjusted by sample size. Key to Pliocene Equidae: = 62 = Nannipus aztecus, 63 = Pseudhipparion simpsoni, 64 = Neohipparion eurystyle, 65 = Cormohipparion emsliei, 66, 67 = Equus simplicidens, 68, 69 = Equus sp., 70 = Nannipus peninsulatus. Key to Pliocene Camelidae: 33, 34 = Megatylopus sp., 35 = Gigantocamelus spatula, 36 = Hemiauchenia sp. Key to Pliocene Antilocapridae: 22 = Tetrameryx sp. Key to Pleistocene Equidae: 71, 74, 78 = Equus sp., 72 = Equus sp. A, 73 = Equus (Hemionus) sp. “B,” 75 = Equus calobatus, 76 = Equus complicatus, 77 = Equus fraternus. Key to Pleistocene Camelidae: 37 = Camelops sp., 38, 39 = Camelops sp., 40 = Hemiauchenia macrocephala, 41 = Camelops nevadanus, 42, 43 = Palaeolama mirifica. Key for to Pleistocene Antilocapridae: 13 = Capromeryx furcifer, 23 = Hayoceros falkenbachi, 24 = Stockoceros onusrosagris.
Discussion
In this study, we examined the relative contributions of exogenous abrasives as a driving force in ungulate tooth evolutionary changes using a combination of dietary proxies (hypsodonty index, mesowear and microwear) with different temporal resolution capabilities to shed light on the amounts of different levels of abrasion imposed on molar teeth over evolutionary time and the potential causes of this abrasion.
The evolution of the Equidae has provided much fuel for the paleoecology fire. The rather dramatic craniodental and locomotory modifications of the late early Miocene radiation of horses (Equinae) prompted Simpson (1951) to call this the time of “the great transformation”. Such changes (e.g., changes in craniodental proportions, cementum-covered, and hypsodont dentition, locomotory changes, and increased body size) were once considered as an indication that savannas spread during this time period, an hypothesis supported by the fact that crown height generally correlates with diet and habitat among extant ungulates.
To add to the growing body of knowledge that has been accumulating that challenges this historical hypothesis, we also investigated whether the appearance of hypsodonty in the Miocene was truly synchronous with the appearance of grasslands and also whether exogenous grit could be a contributing factor to increased exposure to abrasion and subsequent crown height augmentation in fossil horses and other ungulates using three paleodietary techniques.
To this end:
1. We tracked crown height (hypsodonty indices) through time for five North American families – Equidae, Camelidae, Antilocapridae, Dromomerycidae, and Merycoidodontidae.
2. We tracked level of abrasion incurred by individuals in each family through time via assessing cusp shape and occlusal relief (mesowear) and compared it to hypsodonty trends.
3. We reconstructed dietary behavior through time using enamel microwear to track grazing behavior and encroachment on food items by exogenous grit.
The older ideas regarding the correlation between the appearance of grasslands and hypsodonty in North America was fueled by the radiation of the genus Merychippus which represented a more hypsodont horse than earlier forms, as well as the earliest member of the subfamily Equinae which were highly hypsodont at around 17.5 Ma (late early Miocene) (Damuth and Janis, 2011), although a small increase in crown height was seen in the first appearance of Parahippus at about 23 Ma (Jardine et al., 2012). Merychippus, though more hypsodont than Parahippus, is not a highly hypsodont horse and plant phytolith evidence suggests that extensive grasslands were present in North America much earlier than the radiation of Merychippus.
Figure 1B shows that in equids, highly hypsodont equines appeared only about the late middle Miocene (roughly 14 million years ago), well after the projected availability of pervasive open grasslands by 22 Ma (earliest Miocene) (Strömberg, 2004, 2005) or even 26 Ma (latest Oligocene) (Strömberg, 2011), although many early Miocene forms were rather hypsodont. Antilocaprids and camelids also developed hypsodonty in the late middle Miocene. Merycoidodontids attained a modest degree of hypsodonty in the Oligocene. Figure 1B also shows that it is within the Equidae that the highest levels of hypsodonty were obtained through time of the families studied here.
Jardine et al. (2012) (Figure 21A) demonstrated with their regional-scale study of tooth height changes of US Great Plains fossil herbivores that both artiodactyls and perissodactyls show a gradual changeover from brachydont to highly hypsodont forms, but that perissodactyls have a greater proportion of highly hypsodont taxa than artiodactyls. Jardine et al. (2012) also showed that within Perissodactyla, it is the Equidae rather than the Rhinocerotidae that make up a higher proportion of hypsodont forms, and only the Equidae that evolve highly hypsodont forms. Jardine et al. (2012) also found that many families of artiodactyls and perissodactyls were entirely low crowned (≤mesodont), and within the Artiodactyla, only antilocaprids (which appear as mesodont immigrants in the early Miocene), camelids and merycoidodontids attained hypsodont or highly hypsodont dentitions. The first artiodactyls to become hypsodont are the leptauchenine oreodonts and the stenomyline camelids (Jardine et al., 2012). The obvious question is why? Strömberg et al. (2016) investigated functions of phytoliths in vascular plants and rejected the traditional hypothesis that phytoliths in grasses evolved as part of a defense against large herbivorous mammals. Also, Müller et al. (2014) point out that although phytoliths abrade enamel, grit probably induces more wear on teeth in the habitats where most grazers seek food (Madden, 2014). Our results are consistent with these hypotheses as they indicate that a grit effect was likely an important first driver of the acquisition of hypsodonty in the families studied here. For example, very heavily pitted enamel surfaces with many large pits and relatively coarse scratch textures are found in leptauchenine oreodonts and stenomyline camels—results typical of modern ungulates today living in open and arid habitats and exposed to high levels of exogenous grit. Of the three leptauchenine oredonts studied here, two have microwear consistent with “dirty” browsing and Leptauchenia decora has microwear consistent with “dirty” seasonal or regional mixed feeding. Our microwear results for L. decora indicate that some forest fragmentation was most likely apparent in the Oligocene which is consistent with Retallack's research on plains paleosols (Retallack, 1992, 2004) that showed evidence of more open environments with the potential of feeding closer to the ground, as evidenced by mixed feeding in L. decora and the camelid Probrotherium sp.
Equids and antilocaprids also become somewhat hypsodont in the late early Miocene (Hemingfordian), and at this time both families experience high degrees of large pitting in their enamel and begin to show scratch textures regardless of dietary classification indicating heavy exposure to exogenous grit.
Our hypsodonty results (Figure 1B) are consistent with those of Jardine et al. (2012) that show that it is not until the middle Miocene (Barstovian) that highly hypsodont (HI >5) taxa first appear on the Great Plains. While some grass was likely consumed in the Oligocene based on our microwear results (e.g., early camels and pronghorns), the overall pattern in terms of timing of the attainment of hypsodonty is not congruent with a grass diet as the main impetus for the “Great Transition” in equids nor of crown height changes in the ungulate families studied here. Our results are more consistent with prior results that show that even after open grasslands became pervasive, forest cover was also available until the late Miocene and habitats remained heterogeneous (Strömberg, 2005; Strömberg and McInerney, 2011) – a finding consistent with the fact that most ungulates studied here were browsing or mixed feeding throughout the Miocene.
An excellent review of the evolution of grasses and grassland evolution was put forth by Strömberg (2011) where she pointed out that the evolution of hypsodonty was not synchronous with the spread of open and grass-dominated habitats and that in North America, hypsodonty appeared or became more prominent in faunas many million years after the expansion of open-habitat grasses (Strömberg, 2006; Strömberg et al., 2007). Our data confirm this assertion. Strömberg (2011) also confirmed that macrofossils demonstrate that while the earliest grasses on the North American continent are known from the earliest Eocene, the evolution of grasses in North America has proven to be a rather complex phenomenon in terms of how and when open and grass-dominant flora spread with different scenarios proposed by different lines of evidence.
These scenarios are summarized as follows: (1) Palynofloral and macrofossil data indicate that habitats that were mostly grass-free prevailed in the central parts of North America throughout the Oligocene, while habitats that were open and grass-dominated did not disseminate until the middle-late Miocene (e.g., Thomasson, 1990; Bolick et al., 1995). (2) Phytoliths (from the Great Plains region) reveal that some open-habitat type grasses permeated subtropical and closed forest by the earliest Oligocene but were minor elements there until the late Oligocene into the early Miocene when they began to expand. It was not until the latest Miocene that less patchy and more uniformly open grasslands seem to have spread based on work by Strömberg and McInerney (2011). (3) Paleosol data from studies by Retallack (1997, 2007) on the central Great Plains, northern Rocky Mountains, and Pacific Northwest suggests an expansion of more open habitats by the earliest Oligocene while C3-dominated grasslands were purported to have spread in the early Miocene in drier habitats.
Even so, Samuels and Hopkins (2017) have shown that smaller mammals such as rodents and lagomorphs correspond more closely with the timing of habitat changes than those studied here most likely because of their smaller ranges and shorter generation times. Figure 6 shows comparative familial mesowear patterns over time. These patterns closely mirror hypsodonty trends (Figure 1B) in terms of synchrony in the timing of the acquisition of increased dietary abrasion, as evidenced by higher mesowear scores when hypsodonty increases. An interesting difference, however, is that while antilocaprids attain relatively high hypsodonty indices in the late Miocene, the spike in their mesowear scores is relatively lower comparatively than what is observed in equids or camelids. This most likely reflects more intermittent exposure to abrasive elements via seasonal or regional mixed feeding than the high level of dirty browsing (reflected by extreme pitting and coarser scratches) seen in the camelids and more grazing seen in the equids. Antilocaprids also show finer (though still relatively coarse) scratch textures than equids or camelids further explaining their somewhat lower mesowear scores in the late Miocene and presaging their further drop in abrasion as they moved into the Recent and adopted a browse-dominant diet.
We need to insert a word of caution when comparing hypsodonty levels in equids and other ungulates (principally ruminant artiodactyls). Equids experience a greater degree of dental wear for any given diet than other ungulates due to both their digestive physiology and their feeding behavior: thus, even if only considering the relative height of a single tooth, an equid will be more hypsodont than other ungulates consuming the same diet. The living species of equids (all grazers) are not only more hypsodont than other ungulates in terms of measures of individual molars, they also have a greater total postcanine tooth volume (due to their large and molarized premolars, which are as hypsodont as the molars, in contrast to the partially molarized premolars of ruminants, which may be reduced in both size and number, and are not as hypsodont as the molars) (see Janis, 1988). A further additional factor is that equid postcanine teeth do not form roots when they are first laid down; root formation is delayed until around 2 years of age, with additional crown growth during this time, so height of an unerupted molar does not measure the total (functional) height of the tooth as it cannot capture the length of crown added before tooth closure. This dental condition is rare in hypsodont artiodactyls, but it has been reported in a few, such as Ovis, Capra, and Antilocapra (Webb and Hulbert, 1986; Ackermans et al., 2019). We do not know of a comprehensive survey of extinct hypsodont equids that might determine whether the Equus condition is seen throughout the clade, but open-rooted molars have been reported in specimens of the hipparionine equid Pseudhipparion simpsoni (but not in other species of this genus) (Webb and Hulbert, 1986).
Equids (at least those in the hypsodont subfamily Equinae) also have a greater amount of enamel in their postcanine dentition than most ruminant artiodactyls, due to complication of the occlusal enamel surface. Famoso et al. (2016) attribute this occlusal enamel complexity (OEC) to dental durability, noting that it is more extensive in the tribe Hipparionini than in the tribe Equini (of which Equus is the surviving genus): because hipparionines are generally less hypsodont than equinines, they conclude that OEC is an alternative mode of resisting dental wear to hypsodonty. But the issue is more complex than this: for example, high levels of OEC are seen in Pleistocene species of Equus whose mesowear is indicative of browsing (Juha Saarinen, personal communication). Similar OEC is seen in specialized grazing bovids, such as Bos (Bovini), Hippotragus (Hippotragini), and Connochaetes (Alcelaphini). For mechanical reasons, OEC can only be a feature of teeth that have a flat occlusal surface, and Sanson (2016) considers that this dental pattern is related to food manipulation on this surface. A flat occlusal surface in bovids is seen only among grazers, while it is a characteristic feature of the Equinae, even though other dental parameters (microwear, mesowear, hypsodonty index) indicate that many of these species were not grazers (especially among the Hipparionini). This is an interesting issue that requires further investigation; but the data suggest that OEC relates to other issues besides resisting rates of tooth wear.
The reason why equids require a greater tooth volume (i.e., greater resistance to tooth wear) than other ungulates on similar diets relates both to differences in digestive physiology and to food intake behavior. As originally noted by Janis (1988), the less efficient hindgut fermentation of perissodactyls means that they must consume more food per day than a similarly-sized ruminant artiodactyl eating a diet of similar fiber content, and hence equids encounter more total tooth wear per lifetime. Additionally, in contrast to both ruminant artiodactyls, and other perissodactyls such as rhinos, horses grind their food to a fine particle size on its initial ingestion, a behavior which must take a toll on their dentition (Clauss et al., 2015). Although fecal particle size is slightly smaller in ruminants than in horses, attesting to a greater total amount of oral preparation, the majority of this processing takes place during rumination when the food has been softened by the effects of fermentation. In addition, the rechewed food has the benefit of being “washed” in the rumen: abrasive particles such as sand sink to the bottom of the rumen and are not included in the regurgitated bolus (Dittman et al., 2017).
Figures 9–13 show comparative dietary assignments of fossil families in each time period based on average scratch and pit results and reveal some very interesting differences between these North American families. The Camelidae and Merycoidodontidae, though attaining hypsodonty in some forms, were committed browsers throughout most of their evolutionary history but with a type of browsing that incurred a large amount of abrasion (i.e., “dirty” browsing) right from the beginning. Solounias and Semprebon (2002) and Semprebon and Rivals (2007, 2010) have demonstrated a similar pattern of microwear in extant taxa that occupy open and arid habitats such as camels and pronghorns. Thus, any hypsodonty attained by these forms, due most likely to continuous exposure to exogenous grit, would allow them to forage on grass periodically, thus providing a mechanism to broaden their niche. Antilocaprids appear to have shifted their dietary behavior between grass and browse through much of their evolutionary history but returned to mainly browsing in the Recent. Lastly, it is clear that the Equidae exhibited a wider array of dietary behaviors than the other families through most of their evolutionary history (except in the Recent). Even so, grass apparently was a much more common dietary item for equids than for the other families, and when combined with exogenous grit, which was more accelerated in the late Miocene onward (more pitting and coarser scratch textures), may explain the more extreme acquisition of hypsodonty in equids compared to camels and antilocaprids, and set the stage for the Equidae alone of the families studied here to become hypergrazers in the Recent. Our results are compatible with those of Hummel et al. (2011) by stressing that total exposure to abrasive elements (both phytoliths in plant material and exogenous abrasives) should be considered when interpreting factors relating to the acquisition of hypsodonty.
We would also like to caution that much remains to be learned regarding the effects of extrinsic abrasive particles of different sizes (i.e., dust vs. grit). It is obvious that small particles would inflict smaller enamel scars than larger particles and that smaller particles should be more quickly obliterated by wear than larger scars which should persist for a longer period of time. It is intuitive that very small particles such as dust could collectively remove large amounts of tissue without leaving many scars that would be simultaneously detectable by any of these dietary methods. Consequently, the wear rate in open habitats is accelerated despite the fact that large pits do not override the dietary signal, it should be considered that very small particles could be a major but unrecognized wear agent.
Winkler et al. (2019) have proposed that the state of hydration of plant tissue may also affect dental abrasion based on a controlled feeding experiment using guinea pigs fed with different types of forage (in both a fresh and dried state). They stress that water content as well as phytolith content may affect plant abrasiveness. This is very likely why browsers and browse-dominated mixed feeders in open habitats (e.g., camels, pronghorns) tend to have such a clear browser signal in their mesowear, from high attrition in combination with (moderately) high wear rates (Fortelius and Solounias, 2000). Further research should help to elucidate how surface texture wear patterns (as per Winkler et al.) translate into absolute wear rates.
Conclusions
Documenting changes in crown height (hypsodonty index), relative abrasion (mesowear) and diagnostic food and grit scar topography on dental enamel (microwear) allowed for the discernment of the relative contributions of grass vs. exogenous abrasives as potential driving forces in ungulate tooth evolutionary changes during the evolution of North American Equidae, Camelidae, Antilocapridae, Dromomerycidae, and Merycoidodontidae. The timing of hypsodonty acquisition is not consistent with grazing as the main driver for crown height changes in the equids or the artiodactyl families studied, as highly hypsodont ungulates post-date the appearance of widespread grasslands. Synchrony in the timing of the acquisition of hypsodonty and increased dietary abrasion incurred during feeding as measured via mesowear was seen in all five fossil families. High degrees of enamel pitting (particularly large pits) and unusually coarse scratch textures in all five fossil families as measured via microwear are consistent with exposure to exogenous grit or soil ingestion as the main driver of hypsodonty acquisition prior to the consumption of significant levels of grass and consistent with recent experimental controlled feeding studies in ungulates. Camelids, dromomerycids, and merycoidodontids, though attaining varying levels of hypsodonty in some forms, were committed browsers throughout most of their evolutionary history, but with a type of browsing that incurred a large amount of abrasion (i.e., “dirty” browsing). Antilocapridae browsed and grazed throughout most of their evolutionary history, but demonstrated unusual levels of large pitting and relatively coarser scratch textures consistent with exposure to exogenous grit. Equidae exhibited a wider array of dietary behavior than the other families through most of their evolutionary history (except the Recent). Even so, grass was a much more common dietary item for equids than for the other families. This combined with exposure to exogenous grit, which was more accelerated from the early Miocene onward based on more pitting and coarser scratch textures, may explain the more extreme acquisition of hypsodonty in equids compared to the artiodactyl families studied, setting the stage for the Equidae alone to become hypergrazers in the Recent.
Data Availability
All datasets generated for this study are included in the manuscript, from indicated references and/or the supplementary files.
Author Contributions
GS conceived of the idea, collected mesowear, and microwear data, FR and GS analyzed the data with advice from CJ. All authors contributed to the writing of the manuscript.
Conflict of Interest Statement
The authors declare that the research was conducted in the absence of any commercial or financial relationships that could be construed as a potential conflict of interest.
Acknowledgments
Our gratitude is extended to the curators of the American Museum of Natural History Division of Paleontology and Division of Mammalogy, Vertebrate Paleontology Laboratory at the University of Texas at Austin, Florida Museum of Natural History, and Yale Peabody Museum of Natural History for access to and permission to cast specimens. We are thankful to our reviewers for their input and Patricia Drewniak and Emily Woodruff for assistance with molding and casting.
Supplementary Material
The Supplementary Material for this article can be found online at: https://www.frontiersin.org/articles/10.3389/fevo.2019.00065/full#supplementary-material
References
Ackermans, N. L., Clauss, M., Winkler, D. E., Shulz-Kornas, E., Kaiser, T. M., Müller, D. W. H., et al. (2019). Root growth compensates for molar wear in adult goats (Capra aegagrus hircus). J. Exp. Biol. A 331, 139–148. doi: 10.1002/jez.2248
Bernor, R. L., Tobien, H., Hayek, L.-A., and Mittmann, H. W. (1997). Hippotherium primigenium (Equidae, Mammalia) from the late Miocene of Howenegg (Hegau, Germany). Andrias 10, 1–230.
Bernor, R. L., Tobien, H., and Woodburne, M. O. (1989). “Patterns of Old World hipparionine evolutionary diversification and biostratigraphic extension,” in European Neogene Mammal Chronology, eds E. H. Lindsay, V. Fahlbusch, and P. Mein (New York, NY, Plenum Press), 263–319.
Bolick, M. R., Corner, R. G., and Voorhies, M. R. (1995). New pollen data from the Kilgore locality, Valentine Formation; additional evidence for savanna vegetation. Geol. Soc. Am. Prog. Abstr. 27:A–41.
Clauss, M., Steuer, P., Erlinghagen-Lückerath, K., Kaandorp, J., Fritz, J., Südekum, K.-H., et al. (2015). Faecal particle size: digestive physiology meets herbivore diversity. Comp. Biochem. Physiol. A 179, 182–191. doi: 10.1016/j.cbpa.2014.10.006
Damuth, J., and Janis, C. M. (2011). On the relationship between hypsodonty and feeding ecology in ungulate mammals, and its utility in palaeoecology. Biol. Rev. 86, 733–758. doi: 10.1111/j.1469-185X.2011.00176.x
Dittman, M. T., Kreuzer, M., Runge, U., and Clauss, M. (2017). Ingestive mastication in horses resembles rumination but not ingestive mastication in cattle and camels. J. Exp. Biol. A 327, 98–109. doi: 10.1002/jez.2075
Dunn, R. E., Strömberg, C. A. E., Madden, R. H., Kohn, M. J., and Carlini, A. A. (2015). Linked canopy, climate, and faunal change in the Cenozoic of Patagonia. Science 347, 258–261. doi: 10.1126/science.1260947
Famoso, N. A., Davis, E. B., Feranec, R. S., Hopkins, S. S. B., and Price, S. A. (2016). Are hypsodonty and occlusal enamel complexity evolutionarily correlated in ungulates? J. Mammal. Evol. 23, 43–47. doi: 10.1007/s10914-015-9296-7
Fortelius, M. (1985). Ungulate cheek teeth: developmental, functional, and evolutionary interrelations. Acta Zool. Fennica 180, 1–76.
Fortelius, M., Eronen, J., Jernavall, J., Liu, L., Pushkina, D., Rinne, J., et al. (2002). Fossil mammals resolve regional patterns of Eurasian climate change over 20 million years. Evol. Ecol. Res. 4, 1005–1016.
Fortelius, M., and Solounias, N. (2000). Functional characterization of ungulate molars using the abrasion-attrition wear gradient: a new method for reconstructing paleodiets Am. Museum Novit. 3301, 1–36.
Hoffman, J. M., Fraser, D., and Clementz, M. T. (2015). Controlled feeding trials with ungulates: a new application of in vivo dental molding to assess the abrasive factors of microwear. J. Exp. Biol. 218, 1538–1547. doi: 10.1242/jeb.118406
Hulbert, R. C. (1993). Taxonomic evolution in North American Neogene horses (Subfamily Equinae): the rise and fall of an adaptive radiation. Paleobiology 19, 216–234. doi: 10.1017/S0094837300015888
Hulbert, R. C., and MacFadden, B. J. (1991). Morphological transformation and cladogenesis at the base of the adaptive radiation of Miocene hypsodont horses. Am. Mus. Novit. 3000, 1–61.
Hummel, J., Findeisen, E., Südekum, K.-H., Ruf, I., Kaiser, T. M., Bucher, M., et al. (2011). Another one bites the dust: faecal silica levels in large herbivores correlate with high-crowned teeth. Proc. R. Soc. B Biol. Sci. 278, 1742–1747. doi: 10.1098/rspb.2010.1939
Janis, C. (1988). “An estimation of tooth volume and hypsodonty indices in ungulate mammals, and the correlation of these factors with dietary preference,” in Teeth revisited: Proceedings of the VIIth International Symposium on Dental Morphology, Mémoires du Muséum National d'Histoire Naturelle, (série C), eds D. E. Russell, J. P. Santoro, and D. Sigogneau-Russell (Paris: Muséum national d'Histoire naturelle),367–387.
Janis, C. M. (1984). “The significance of fossil ungulate communities as indicators of vegetation structure and climate,” in Fossils and Climate, ed P. J. Brenchley (New York, NY: John Wiley and Sons), 85–104.
Janis, C. M. (1993). Tertiary mammal evolution in the context of changing climates, vegetation, and tectonic events. Annu. Rev. Ecol. Syst. 24, 467–500. doi: 10.1146/annurev.es.24.110193.002343
Janis, C. M. (2007). “The horse series,” in Icons of Evolution, ed B. Regal (Westport, CT: Greenwood Press), 257–280.
Janis, C. M. (2008). “An evolutionary history of browsing and grazing ungulates,” in The Ecology of Browsers and Grazers, eds I. J. Gordon and H. H. T. Prins (Berlin: Springer-Verlag), 21–45.
Janis, C. M., Damuth, J., and Theodor, J. M. (2000). Miocene ungulates and terrestrial primary productivity: where have all the browsers gone? Proc. Natl. Acad. Sci. U.S.A. 97, 7899–7904. doi: 10.1073/pnas.97.14.7899
Janis, C. M., Damuth, J., and Theodor, J. M. (2002). The origins and evolution of the North American grassland biome: the story from hoofed mammals. Palaeogeogr. Palaeoclimatol. Palaeoecol. 177, 183–198. doi: 10.1016/S0031-0182(01)00359-5
Janis, C. M., Damuth, J., and Theodor, J. M. (2004). The species richness ofMiocene browsers, and implications for habitat type and primary productivity in the North American grassland biome. Palaeogeogr. Palaeoclimatol. Palaeoecol. 207, 371–398. doi: 10.1016/j.palaeo.2003.09.032
Janis, C. M., and Fortelius, M. (1988). On the means whereby mammals achieve increased functional durability of their dentitions, with special reference to limiting factors. Biol. Rev. 63, 197–230. doi: 10.1111/j.1469-185X.1988.tb00630.x
Jardine, P. E., Janis, C. M., Sahney, S., and Benton, M. J. (2012). Grit not grass: concordant patterns of early origin of hypsodonty in Great Plains ungulates and Glires. Palaeogeogr. Palaeoclimatol. Palaeoecol. 365–366, 1–10. doi: 10.1016/j.palaeo.2012.09.001
Lucas, P. W., van Casteren, A., Al-Fadhalah, K., Almusallam, A. S., Henry, A. G., Michael, S., et al. (2014). The role of dust, grit and phytoliths in tooth wear. Ann. Zool. Fenn. 51, 143–152. doi: 10.5735/086.051.0215
MacFadden, B. J. (1992). Fossil Horses: Systematics, Paleobiology, and Evolution of the Family Equidae. Cambridge: Cambridge University Press
MacFadden, B. J. (2000a). “Origin and evolution of the grazing guild in Cenozoic New World terrestrial mammals,” in Evolution of Herbivory in Terrestrial Vertebrates, H. D. Sues (Cambridge: Cambridge University Press), 223–244.
MacFadden, B. J. (2000b). Cenozoic mammalian herbivores from the Americas: reconstructing ancient diets and terrestrial communities. Annu. Rev. Ecol. Syst. 31, 33–59. doi: 10.1146/annurev.ecolsys.31.1.33
MacFadden, B. J., and Hulbert, R. C. (1988). Explosive speciation at the base of the adaptive radiation of Miocene grazing horses. Nature 336, 466–468. doi: 10.1038/336466a0
Madden, R. H. (2014). Hypsodonty in Mammals. Evolution, Geomorphology, and the Role of Earth Surface Processes. Cambridge, UK: Cambridge University Press.
Mihlbachler, M. C., Rivals, F., Solounias, N., and Semprebon, G. M. (2011). Dietary change and evolution of horses in North America. Science 331, 1178–1181. doi: 10.1126/science.1196166
Mihlbachler, M. C., and Solounias, N. (2006). Coevolution of tooth crown height and diet in oreodonts (Merycoidodontidae, Artiodactyla) examined with phylogenetically independent contrasts. J. Mammal. Evol. 13, 11–36. doi: 10.1007/s10914-005-9001-3
Müller, J., Clauss, M., Codron, D., Schulz, E., Hummel, J., Fortelius, M., et al. (2014). Growth and wear of incisor and cheek teeth in domestic rabbits (Oryctolagus cuniculus) fed diets of different abrasiveness. J. Exp. Zool. Part A 321, 283–298. doi: 10.1002/jez.1864
Prothero, D. R., and Schoch, R. M. (1989). “The origin and evolution of perissodactyls: a summary and synthesis,” in The Evolution of Perissodactyls, eds D. R. Prothero and R. M. Schoch (New York, NY: Oxford University Press), 504–529.
Retallack, G. J. (1992). “Paleosols and changes in climate and vegetation across the Eocene–Oligocene boundary,” in Eocene–Oligocene Climatic and Biotic Evolution, eds D. R. Prothero and W. A. Berggren (Princeton, NJ: Princeton University Press), 382–398.
Retallack, G. J. (1997). Neogene expansion of the North American prairie. Palaios 12, 380–390. doi: 10.2307/3515337
Retallack, G. J. (2004). Late Oligocene bunch grassland and early Miocene sod grassland paleosols from central Oregon, U. S. A. Palaeogeogr. Palaeoclimatol. Palaeoecology. 207, 203–237. doi: 10.1016/j.palaeo.2003.09.027
Retallack, G. J. (2007). Cenozoic paleoclimate on land in North America. J. Geol. 115, 271–294. doi: 10.1086/512753
Samuels, J. X., and Hopkins, S. B. (2017). The impacts of Cenozoic climate and habitat changes on small mammal diversity of North America. Glob. Planet. Change 149, 36–52. doi: 10.1016/j.gloplacha.2016.12.014
Sanson, G. (2016). Cutting food in terrestrial carnivores and herbivores. Interface Focus 6:20150109. doi: 10.1098/rsfs.2015.0109
Scott, W. B. (1937). A History of Land Mammals in the Western Hemisphere. New York, NY: The MacMillan Company.
Semprebon, G. M. (2002). Advances in the Reconstruction of Extant Ungulate Ecomorphology With Applications to Fossil Ungulates, Ph.D. Dissertation, University of Massachusetts, Amherst.
Semprebon, G. M., Godfrey, L. R., Solounias, N., Sutherland, M. R., and Jungers, W. L. (2004). Can low-magnification stereomicroscopy reveal diet? J. Hum. Evol. 47, 115–144. doi: 10.1016/j.jhevol.2004.06.004
Semprebon, G. M., and Rivals, F. (2007). Was grass more prevalent in the pronghorn past? An assessment of the dietary adaptations of Miocene to recent Antilocapridae (Mammalia: Artiodactyla). Palaeogeogr. Palaeoclimatol. Palaeoecol. 253, 332–347. doi: 10.1016/j.palaeo.2007.06.006
Semprebon, G. M., and Rivals, F. (2010). Trends in the paleodietary habits of fossil camels from the Tertiary and Quaternary of North America. Palaeogeogr. Palaeoclimatol. 295, 131–145. doi: 10.1016/j.palaeo.2010.05.033
Semprebon, G. M., Rivals, F., Solounias, N., and Hulbert, R.ichard, C. Jr (2016). Paleodietary reconstruction of fossil horses from the Eocene through Pleistocene of North America, Palaeogeography, Palaeoclimatology. Palaeoecology 442, 110–127. doi: 10.1016/j.palaeo.2015.11.004
Simpson, G. G. (1951). Horses: The Story of the Horse Family in the Modern World and Through Sixty Million Years of History. Oxford: Oxford University Press
Simpson, G. G. (1953). Evolution and Geography: an Essay on Historical Biogeography with Special Reference to Mammals. Eugene: Oregon State System of Higher Education.
Solounias, N., Fortelius, M., and Freeman, P. (1994). Molar wear rates in ruminants: a new approach. Ann. Zool. Fennici 31, 219–227.
Solounias, N., and Semprebon, G. (2002). Advances in the reconstruction of ungulate ecomorphology with application to early fossil equids. Am. Museum Novit. 3366, 1–49.
Spaan, A., Sondaar, P., and Hartman, W. (1994). The structure of the evolutionary process. Geobios 27, 385–390. doi: 10.1016/S0016-6995(94)80184-3
Stebbins, G. L. (1981). Coevolution of grasses and herbivores. Ann. Missouri Bot. Gardens 68, 75–86. doi: 10.2307/2398811
Stirton, R. A. (1947). Observation of evolutionary rates in hypsodonty. Evolution 1, 32–41. doi: 10.1111/j.1558-5646.1947.tb02711.x
Strömberg, C. A. E. (2004). Using phytolith assemblages to reconstruct the origin and spread of grass-dominated habitats in the great plains of North America during the late Eocene to early Miocene. Palaeogeogr. Palaeoclimatol. Palaeoecology. 207, 239–275. doi: 10.1016/j.palaeo.2003.09.028
Strömberg, C. A. E. (2005). Decoupled taxonomic radiation and ecological expansion of open-habitat grasses in the Cenozoic of North America. Proc. Natl. Acad. Sci. U.S.A. 102, 11980–11984.
Strömberg, C. A. E. (2006). Evolution of hypsodonty in equids: testing a hypothesis of adaptation. Paleobiology 32, 236–258. doi: 10.1666/0094-8373(2006)32[236:EOHIET]2.0.CO;2
Strömberg, C. A. E. (2011). Evolution of grasses and grassland ecosystems. Annu. Rev. Earth Planet. Sci. 39, 517–544. doi: 10.1666/09067.1
Strömberg, C. A. E., Dunn, R. E., Madden, R. H., Kohn, M. J., and Carlini, A. A. (2013). Decoupling the spread of grasslands from the evolution of grazer-type herbivores in South America. Nat. Commun. 4, 1–4. doi: 10.1038/ncomms2508
Strömberg, C. A. E., and McInerney (2011). The Neogene transition from C3 to C4 grasslands in North America: assemblage analysis of fossil phytoliths. Paleobiology 37, 50–71.
Strömberg, C. A. E., Stilio, V. S., and Di Song, Z. (2016). Functions of phytoliths in vascular plants: an evolutionary perspective. Funct. Ecol. 30, 1286–1297. doi: 10.1111/1365-2435.12692
Strömberg, C. A. E., Werdelin, L., Friis, E. M., and Sarac„, G (2007). The spread of grass-dominated habitats in Turkey and surrounding areas during the Cenozoic: phytolith evidence. Palaeogeogr. Palaeoclimat. Palaeoecol. 250, 18–49. doi: 10.1016/j.palaeo.2007.02.012
Thomasson, J. R. (1990). “Fossil plants from the Late Miocene Ogallala Formation of central North America: possible paleoenvironmental and biostratigraphic significance,” in Geologic Framework and Regional Hydrology: Upper Cenozoic Blackwater Draw and Ogallala Formations, Great Plains, ed T. C. Gustavson (Austin, TX: Bur. Econ. Geol. Univ. Texas Austin), 99–114.
Webb, S. D. (1977). A history of savanna vertebrates in the New World. Part I: North America. Annu. Rev. Ecol. Syst. 8, 355–380.
Webb, S. D. (1983). “The rise and fall of the late Miocene ungulate fauna in North America,” in Coevolution, ed M. H. Nitecki (Chicago, IL: University of Chicago Press), 267–306.
Webb, S. D., and Hulbert, R. C. Jr. (1986). “Systematics and evolution of Pseudhipparion (Mammalia, Equidae) from the late Neogene of the Gulf Coastal Plain and the Great Plains,” in Contributions to Geology, Special Paper 3 (Laramie: University of Wyoming), 237–272.
Webb, S. D., and Opdyke, N. D. (1995). “Global climatic influence on Cenozoic land mammal faunas,” in National Research Council Panel on Effects of Past Global Change on Life, Effects of Past Global Change On Life, eds J. P. Kennett and S. M. Stanley (Washington, DC: National Academies Press), 184–208.
White, T. E. (1959). “The endocrine glands and evolution, No. 3: Os cementum, hypsodonty, and diet,” in Contributions from the Museum of Paleontology 13. University of Michigan, pp. 211–265.
Williams, S. H., and Kay, R. F. (2001). A comparative test of adaptive explanations for hypsodonty in ungulates and rodents. J. Mammal. Evol. 8, 207–229. doi: 10.1023/A:1012231829141
Winkler, D. E., Schulz-Kornas, E., Kaiser, T. M., De Cuyperd, A., Clausse, M., and Tütken, T. (2019). Forage silica and water content control dental surface texture in guinea pigs and provide implications for dietary reconstruction. Proc. Natl. Acad. Sci. U.S.A. 116, 1325–1330. doi: 10.1073/pnas.1814081116
Keywords: ungulates, microwear, mesowear, hypsodonty, paleodiet
Citation: Semprebon GM, Rivals F and Janis CM (2019) The Role of Grass vs. Exogenous Abrasives in the Paleodietary Patterns of North American Ungulates. Front. Ecol. Evol. 7:65. doi: 10.3389/fevo.2019.00065
Received: 04 January 2019; Accepted: 21 February 2019;
Published: 19 March 2019.
Edited by:
K. Christopher Beard, University of Kansas, United StatesReviewed by:
Mikael Fortelius, University of Helsinki, FinlandNikos Solounias, New York Institute of Technology, United States
Copyright © 2019 Semprebon, Rivals and Janis. This is an open-access article distributed under the terms of the Creative Commons Attribution License (CC BY). The use, distribution or reproduction in other forums is permitted, provided the original author(s) and the copyright owner(s) are credited and that the original publication in this journal is cited, in accordance with accepted academic practice. No use, distribution or reproduction is permitted which does not comply with these terms.
*Correspondence: Gina M. Semprebon, gsempreb@baypath.edu