- 1Department of Biological Sciences, Macquarie University, Sydney, NSW, Australia
- 2Hawai'i Institute of Marine Biology, University of Hawai'i, Kaneohe, HI, United States
- 3Department of Biological Sciences, Florida International University, North Miami, FL, United States
- 4School of Biological Sciences and Australian Research Council Centre of Excellence for Coral Reef Studies, The University of Queensland, St. Lucia, QLD, Australia
- 5Department of Watershed Sciences and Ecology Center, Utah State University, Logan, UT, United States
- 6Remote Sensing Research Centre, School of Earth and Environmental Sciences, The University of Queensland, St. Lucia, QLD, Australia
- 7Research Institute for the Environment and Livelihoods, Charles Darwin University, Darwin, NT, Australia
How species interactions shape habitat structure is a longstanding question in ecology. A curious phenomenon reflecting ecological self-organization around reef habitat structures exists on coral reefs: large-scale (hundreds to hundreds of thousands of m2) halo-like patterns surrounding patch reefs, i.e., individual coral reefs that are often separated by seagrass or macroalgal meadows. These “halos,” long known to occur in various locations worldwide, reflect a distinct band of unvegetated sediments surrounding coral patch reefs. However, the full suite of mechanisms controlling them have never been rigorously explored, perhaps due to the common assumption dating back nearly 50 years that they arise solely from reef-based herbivory patterns shaped by anti-predator behavior. Here we provide empirical evidence from a set of halos within Australia's Great Barrier Reef that risk-averse foraging and a previously unrecognized functional group contribute to halo formation, demonstrating that these halos cannot be explained by any one mechanism in isolation. Our results show that halos are a more complex ecological phenomenon than previously assumed by the majority of studies of halos. Specifically, risk-averse grazing by herbivores is likely a key mechanism behind the formation of halos, as generally assumed, but bioturbators also play a central role. This knowledge furthers our understanding of how small-scale species interactions can structure habitat at landscape scales. These large-scale habitat features are important because they affect at least one important ecosystem function, carbon storage, and potentially others (e.g., biological nutrient transfer). These results also raise the question of whether other self-organized ecological patterns may be more nuanced than is currently assumed. This study capitalizes on recent advances in high resolution satellite imagery accessibility that allow ecologists to measure landscape-scale habitat features nearly everywhere on land and in shallow seas. Our results suggest that halos may hold potential as the basis for a tool for remotely observing ecological interactions and measuring large-scale ecosystem change on coral reefs.
Introduction
Ecologists have long been fascinated by the diverse ways in which species interactions can shape the habitats in which they live. From early studies in California grasslands (Bartholomew, 1970) to more recent studies of self-organized landscape patterns arising from different ecological processes across terrestrial (Getzin et al., 2016; Tarnita et al., 2017) and marine (Ruiz-Reynés et al., 2017) ecosystems, studies of how interactions among species shape their physical habitat abound. In many of these cases, vegetative patterns in particular result from the interplay of organisms' abundance and/or behavior with existing physical habitat structure. Self-organized patterns deriving from small-scale species interactions have recently been shown to increase ecosystem resilience to perturbations in marine systems (de Paoli et al., 2017). Understanding how species interactions shape their physical environment thus has increasing relevance for ecological systems in the Anthropocene.
A striking example of repeated habitat features over large spatial scales of hundreds to hundreds of thousands of m2 can be seen from high spatial resolution (in this case, ~2 m pixel−1) aerial and satellite imagery of tropical coasts. These halo-like patterns occur where an absence of seagrass or benthic algae leaves a distinct band of un-vegetated sediments surrounding coral patch reefs. Patch reefs are individual coral reefs that are often separated by seagrass or algal meadows and most often cover spatial extents of tens to hundreds of m2. These halo-like patterns, long known to occur in a handful of locations (e.g., Randall, 1965; Ogden, 1976) and often referred to as grazing halos (hereafter halos), occur in coral reefs worldwide (E. Madin, pers. obs; Precoda, pers. obs; C. Roelfsema, pers. obs.). However, the exact mechanisms controlling this common tropical and sub-tropical vegetation pattern have never been conclusively established, despite a common assumption dating back nearly 50 years that they arise from risk-averse herbivory (Randall, 1965; Ogden et al., 1973; Armitage and Fourqurean, 2006; Madin et al., 2011).
Halos offer a unique window into understanding how self-organized species interactions around existing habitat features can re-structure habitat at landscape scales (Rietkerk and van de Koppel, 2008). Ogden et al. (1973) pioneering work in the US Virgin Islands, in which halos disappeared after herbivorous sea urchins were experimentally removed, indicated that herbivory was sufficient to form halos and was necessary for their maintenance. Most studies of halos have implicitly or explicitly assumed that herbivory is the direct mechanism causing halos (Ogden et al., 1973; Sweatman and Robertson, 1994; Valentine and Heck, 2005; Armitage and Fourqurean, 2006; Valentine et al., 2007; Madin et al., 2011; Downie et al., 2013; Turgeon et al., 2014) and that predation risk is the indirect mechanism behind halos, i.e., predators create landscapes of risk that maintain spatially-constrained foraging patterns by herbivores (Randall, 1965; Ogden et al., 1973; Macintyre et al., 1987; Valentine et al., 2007; Madin et al., 2011; Downie et al., 2013; Atwood et al., 2018). However, Alevizon (2002) described halos around patch reefs in the Bahamas that had no apparent herbivore assemblage, calling the former assumption into question. These contrasting findings suggest that different systems may have different mechanisms behind halo formation, or that these mechanisms are more complex than has generally been assumed.
Understanding the key mechanisms behind halo formation, and the degree to which halos share common characteristics and mechanisms across systems, could help scientists and managers understand large-scale ecosystem changes on coral reefs. However, in order to take advantage of what halos can tell us about how an ecosystem is operating or potentially changing, we first need clarity on the particular mechanisms at play. Specifically, understanding which functional groups and/or processes lead to these landscape-scale habitat patterns in a given system may allow us to detect or infer ecosystem change if and when halos change in their occurrence and/or size.
To address this need, we used underwater and satellite remote imaging technologies to (1) quantify spatial characteristics of halos around a suite of Indo-Pacific patch reefs and (2) document species presence and interactions within these halos. Our unique approach of applying high-resolution satellite imagery to quantify halo characteristics allows us to do so at scales not accessible with traditional in-situ monitoring methods. This approach further provides a proof of concept for using this technology to quantify changes in “footprints” of species interactions on shallow seabed habitat over large spatial scales.
Methods
Characterizing Halos From Remote Sensing Imagery
Halos were visually identified and manually digitized from high spatial resolution remote sensing images of the sheltered lagoonal habitat (~27 km2) adjacent to Heron Island, Australia with pixel size varying between 1 and 4.0 m. These images were acquired as part of ongoing benthic habitat mapping research on Heron Reef (Roelfsema et al., 2018) and included a series of multi-year, sequential remote sensing images of Heron lagoon from satellites Worldview 2/3, Quickbird 2, and Ikonos and an airplane for CASI imagery. A subset of satellite imagery representing lagoonal area was extracted using a boundary previously identified as part of a geomorphic zonation map (Phinn et al., 2012). When halos were present, 24 reefs (including the same 22 patch reefs used for in-situ surveys) and their halos were outlined in 11 lagoon satellite images spanning 2002 to 2014. Halos were present in fewer than half of the images for any given reef (Table S1). Given the thin, wispy, and often sparse nature of the benthic algae assemblage within Heron lagoon, it is unclear if halos were not present in the years they did not appear in satellite imagery, or rather if the level of background algae was too sparse to enable detection from the imagery. Halo width was calculated by subtracting the radius of a circle with the same area as the reef from the radius of a circle with the area enclosed by the outer edge of the halo as discerned by a single observer for all halos. Further details of halo measurement are in Supplementary Information. Reef and halo polygons were measured using QGIS 2.14.8-Essen (QGIS Development Team, 2016).
In-situ Observation of Species Interactions and Algal Patterns
Underwater remote video (i.e., cameras recording in the absence of divers) was used to monitor fish and invertebrate behavior and abundance at 22 patch reefs within the Heron reef lagoon system (Figure S1) in May of 2013. This habitat is typically shallower than 5 m and features patch reefs isolated by expanses of bare or algae-covered sand. Benthic algae in Heron lagoon is a mixed-species assemblage, comprised in our study area largely of Enteromorpha sp. (synonymized now under Ulva; Chlorophyta), Hincksia sp. (Phaeophyta), Cladophora sp. (Chlorophyta), and benthic micro algae (BMA) mats. Video surveys were conducted during both daytime and dusk/nighttime with GoPro underwater video cameras (www.gopro.com) deployed in a line outward from patch reefs, with an average of 201 min per video (Figure S1). To estimate the percent cover of benthic algae at different distances from the reef, benthic quadrats were laid near each camera station and points on the substrate were manually classified from images as sand or algae. Table S2 provides a summary of the data collected.
After assigning species-level identifications from remote videos (see Supplementary Information), fishes were grouped into the following functional categories: (i) herbivores that primarily feed on plant material and detritus, which included all surgeonfishes, rabbitfishes, parrotfishes, chubs, and unicornfishes; (ii) piscivorous top predators that feed mainly on other fishes and have few or no predators themselves, which included all sharks; (iii) piscivorous mesopredators, which included barracuda, jacks/trevally and groupers, that feed mainly on other fishes and are potentially preyed upon by the top predators; and (iv) invertivores that feed largely on benthic invertebrates, disturb sediment and dislodge associated benthic algae through bioturbation, which included grunts (which includes sweetlips), snappers, and emperors. Only macro-invertebrates (sea cucumbers, sea urchins, and cephalopods) and sea turtles that could be reliably identified were censused from our videos and included in our dataset. Sea urchins, turtles, and cephalopods were observed in only a handful of instances, precluding analysis of their occurrence data.
Daytime and dusk/night fish presence (percent of total video seconds) and bite rate (both aggregate and per capita) were calculated for trophic groups and selected genera. Sea cucumbers were counted and their percent of total video seconds recorded.
Results
Characterizing Halos From Remote Sensing Imagery
Halo widths in Heron lagoon were determined in two ways: first, by averaging the halo widths over remotely sensed images at the patch reefs where the surveys were conducted (Figure 1A), and second, by averaging benthic algal cover in 2013 from in-situ quadrats at the same reefs (Figure 1B; details in next section). Our remotely sensed outlines provide a binary metric of halo presence (i.e., an area is either inside or outside of the halo boundary) and indicated that halos at these reefs were generally no larger than ~15 m wide (Figure 1A).
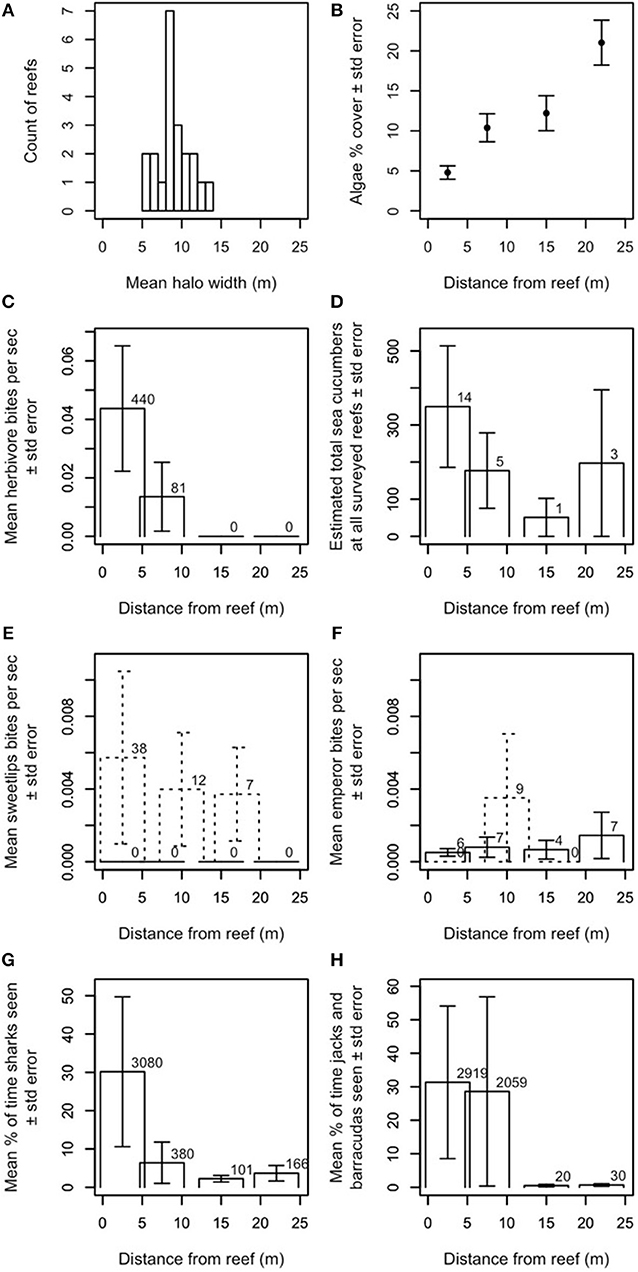
Figure 1. Ecological characteristics of halos and contributors to halo formation. (A) Approximate widths of halos at Heron Island, measured from satellite images, and averaged over time. Twenty-one reefs are included because three of the 24 reefs used in the study never had measureable halos in the satellite images. (B) Average benthic algal cover in 2013 surrounding the 22 study reefs where in-situ data could be collected relative to distance from the reef. (C) Mean aggregate bite rate of herbivorous fishes by distance from the reef (number above bar indicates N bites observed). Herbivorous fishes were seen in our dusk/night dataset, but were never observed feeding. (D) Estimated total number of sea cucumbers at all surveyed reefs by distance from the reef (number above bar indicates N individuals observed). Daytime observations are shown. Dusk/night observations yielded too few observations to report statistically (i.e., one sea cucumber in the band from −0.3 to 5.3 m from the edge of the reef and three sea cucumbers in the band from 7.2 to 12.8 m from the edge of the reef). (E,F) Mean aggregate bite rate for (E) sweetlips and (F) emperors, by distance from the reef (number above bar indicates N bites observed). Solid lines indicate daytime observations; dashed lines indicate dusk/night observations. Panels (C,E–H) show data averaged over all available (daytime N = 22, dusk/night N = 6–9) reefs at Heron. All bite rates are calculated relative to the total length of the video recordings at each distance from the reef. (G,H) Mean percent of time spent for reef-associated piscivores (G) sharks (genera Carcharinus, Galeocerdo, Negaprion, Triaenodon) and (H) jacks and barracuda (genera Caranx, Carangoides, Sphyraena), by distance from the reef. Percents are calculated relative to the total length of the video recordings at each distance from the reef and number above bar indicates N seconds individuals of each group were observed. Only one shark was observed off the reef at dusk/night; no jacks or barracudas were observed off the reef at dusk/night. None of the system's large reef-based piscivores (genera Plectropomus, Cephalopholis, Gymnothorax) were seen off the reef during daytime observations or anywhere during dusk/night observations. Y-values in panels (C–H) are extrapolated to cover entire area at that distance (see Supplementary Information for details).
Additionally, a comparison of halo widths and patch reef sizes within the Heron lagoon showed a positive relationship between halo width and patch reef area (Figure S2). However, visual inspection of analogous data from other locations globally shows no clear relationship, suggesting that this Heron lagoon reef pattern is not ubiquitous (K. Precoda, unpublished data).
In-situ Observation of Species Interactions and Algal Patterns
Our in-situ benthic quadrats across the halos, which provide a metric of spatial changes in benthic algal cover at standardized points up to 30 m from the reef edge (i.e., both within the halo and beyond the halo boundary in the algal meadows; Figures 1B, S3), indicated that algal cover increased most dramatically between 15 and 25 m from reef edges. Halo boundaries were observed up to 14 m from reef edges, with a mean of ~9 m (Figure 1A). Together with our remotely sensed data, these data suggest that (a) halo boundaries in this system are visible in satellite imagery where algal percent cover roughly exceeds 10–12%, and (b) algal coverage increases up to (at least) 25 m from reef edges.
Our video surveys showed that most activity by herbivores and large bioturbating fishes occurred at distances shorter than or comparable to typical halo widths in Heron lagoon. Herbivores' mean aggregate bite rate dropped precipitously by about 10 m from the reef [Figure 1C; families Acanthuridae, Siganidae, Labridae (Scarinae only; formerly family Scaridae), and Kyphosidae]. Sea cucumbers (class Holothuroidea), which bioturbate the substrate while scavenging for detritus, had higher densities farther from patch reefs and were most commonly found ~20–25 m from reefs (Figure 1D). The large bioturbating sweetlips (family Haemulidae) foraged roughly evenly from the reef edge up to ~20 m from reefs (Figure 1E). Bioturbating emperors (family Lethrinidae) had the highest aggregate bite rate at ~10 m from reef edges, but foraged at lower rates out to ~25 m from reefs (Figure 1F). Stingrays (family Dasyatidae), shovelnose rays (family Rhinobatidae), and eagle rays (family Myliobatidae) were all occasionally observed. Two of the shovelnose rays in the field of view (FOV) at 12.2–17.8 m were feeding and thus bioturbating the substrate; others were passing and were not observed to bioturbate the substrate (Figure S4). Rays were seen passing by at all camera distances from the reef; the three rays that were feeding or resting on the substrate were observed between 7.2 and 17.8 m.
The outer boundary of where herbivores were observed was ~10 m from patch reef edges, with the vast majority of feeding activity, and highest per-capita bite rate no farther than 5 m (Figure 1C).
Large predatory fishes (sharks, family Carcharhinidae; jacks, family Carangidae; and barracudas, family Sphyraenidae) were found at all distances surveyed from patch reefs (i.e., up to 25 m from reef edges) during daytime observations, but their activity was concentrated primarily within ~5–10 m (Figures 1G,H) from the reef's edge. Sharks exhibited a smaller, secondary peak in activity at ~20–25 m from the reef's edge (Figure 1G).
Spatial patterns of foraging away from a central location such as patch reefs could be related to competition among reef-based foragers (e.g., mobile herbivores). Specifically, higher herbivore densities on the reef could necessitate more distant foraging to meet nutritional requirements if sufficient food resources are not present on or within the reef matrix. We therefore examined whether densities of the herbivorous species observed foraging in the halo zone were related to either the maximum excursion distance away from patch reefs (Figure 2A) or to halo width (Figure 2B), and whether herbivore density increased with increasing reef area (Figure S5). In each case, no pattern was apparent. A rank-order, tie-corrected Spearman correlation produced rho = 0.35 (Figure 2A) and rho = 0.25 (Figure 2B). We used a permutation test (100,000 permutations) to calculate p-values and obtained p = 0.06 (Figure 2A) and p = 0.15 (Figure 2B), suggesting that while we detected no statistically significant effects of density-dependence (Figure 2A), a non-significant trend toward density-dependent foraging herbivore distances may exist. Multiple models confirmed that regardless of which, if any, outliers were excluded from analysis, the slope of the relationship between reef area and herbivore abundance was statistically indistinguishable from zero.
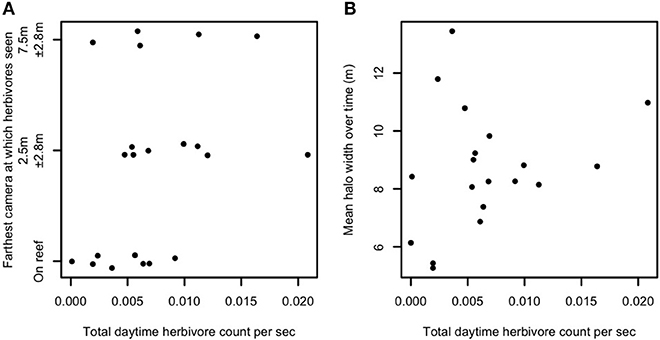
Figure 2. Relationship between herbivore abundance, behavior, and halo width. Herbivore abundance per unit time against (A) field of view (FOV) of farthest camera visited by herbivores (vertically jittered for better visibility) and (B) time-averaged halo width, in Heron lagoon. Twenty-one patch reefs are shown in (A), as no herbivores were seen on one patch reef which had video cameras. Nineteen patch reefs are shown in (B), as no halos were measured around three patch reefs which had video cameras. Y-axis values in (A) represent the range of horizontal distance along the benthos from the edge of each patch reef that was within each camera's FOV. Herbivores in this plot include all species observed feeding in any of the halos (i.e., Acanthurus spp., Siganus spp., Zebrasoma veliferum, and Naso unicornis). Abundances are extrapolated to estimate total abundance at each reef.
Alternately, foragers' spatially constrained movement could be the consequence of consumption by predators, where foragers that venture farther from the reef's shelter are more likely to be consumed and thus fewer individuals are found in more distant areas. We therefore quantified attacks by predators on prey. We observed no successful attacks by predators on any forager (e.g., herbivore or invertivorous bioturbator) in 395 h of daytime footage (with 311 of these in the halo zone and algal meadow) and 52 h of dusk/night footage.
A third possibility is that predator presence, even in the absence of frequent consumption of prey, is sufficient to constrain foragers' excursions to areas adjacent to and near reef shelter. We therefore examined the relationship between mobile (i.e., non-cryptic) predator presence and aggregate herbivore bite rate (Figure 3) and per-capita bite rates by herbivores, sweetlips, and emperors (Figures 4A–C) as a function of distance from reef shelter. Figure 3 suggests that both shark and mesopredator presence may be inversely correlated with aggregate herbivore bite rate and that overall foraging by herbivores may be suppressed when predation risk is higher. Per-capita bite rates by herbivores, sweetlips, and emperors each peaked away from the reef's edge, with herbivores peaking between 4.7 and 10.3 m (Figure 4A), sweetlips peaking between 7.2 and 12.8 m (Figure 4B), and emperors peaking between 12.2 and 17.8 m from reef's edge (Figure 4C).
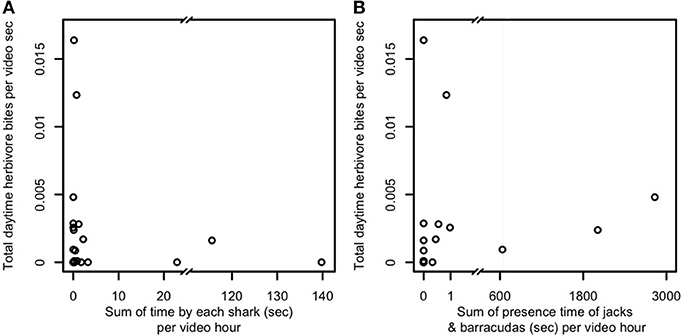
Figure 3. Relationship between predator presence and herbivore bite rate for (A) sharks and (B) jacks and barracudas. Predator presence is measured as total time predators were observed at the study's 22 focal patch reefs in Heron lagoon (no herbivores were seen on one patch reef; sharks were observed around seven patch reefs and jacks and barracudas around eight). When multiple predators were present, the time each individual was observed was summed to obtain total presence time. Presence time is normalized per hour of video time. Herbivores in this plot include all species observed. Because of the variation of one to three orders of magnitude in predator presence time, because predators were observed at only a minority of patch reefs, and for lack of an a priori model that is sufficiently detailed to explain bite rate observations over such a wide range of predator presence times, we present here the raw data for examination.
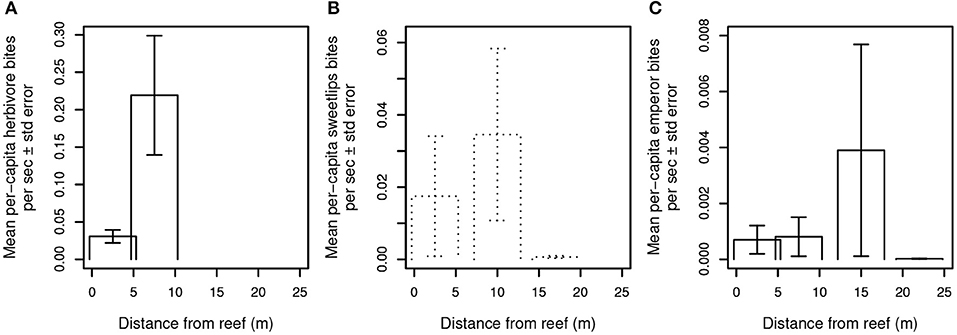
Figure 4. Per capita bite rates of herbivores and bioturbating fishes. Bite rates are calculated per second of video footage for (A) herbivores, (B) sweetlips, and (C) emperors. Daytime observations are indicated with solid lines and dusk/night with dotted lines. No herbivores were observed in the dusk/night videos, and no feeding by sweetlips occurred in the daytime videos. In the dusk/night videos, emperors were seen taking a total of nine bites at the intermediate-distance camera on a single patch reef, and a total of three non-feeding emperors were recorded at the near and far cameras on other reefs.
Discussion
The longstanding question in ecology of how species interactions self-organize to shape habitat structure continues to spark interest across a vast array of ecosystem types (Rietkerk and van de Koppel, 2008). Understanding the interplay between established physical structure (in this case, coral patch reefs), species behaviors and interactions, and vegetative patterns is of particular relevance in the current era of rapid biological and environmental change (Hobbs et al., 2009). This study sheds light on the mechanisms underlying one well-known example of ecological self-organization around reef habitat structures: coral reef halos. We do so by documenting the species abundance, activity, and behavioral relationships at a set of Indo-Pacific patch reefs surrounded by concentric, vegetation-free halo patterns and using remote sensing imagery to quantify halo sizes averaged over time. Collectively, these results provide evidence that individual-level species interactions likely scale up to generate landscape-level patterns in habitat structure on scales visible from high-spatial-resolution (~2 m pixel size) satellites. By empirically demonstrating that risk-averse herbivory and a novel functional group—bioturbators—can contribute to the formation of halos, these findings shed new light on the prevailing assumption within marine ecology (e.g., Randall, 1965; Ogden et al., 1973) regarding the mechanisms underlying self-organized halos (Figure 5). In so doing, this study also highlights the utility of applying high-resolution, emergent imaging technologies toward uncovering answers to classic questions in ecology.
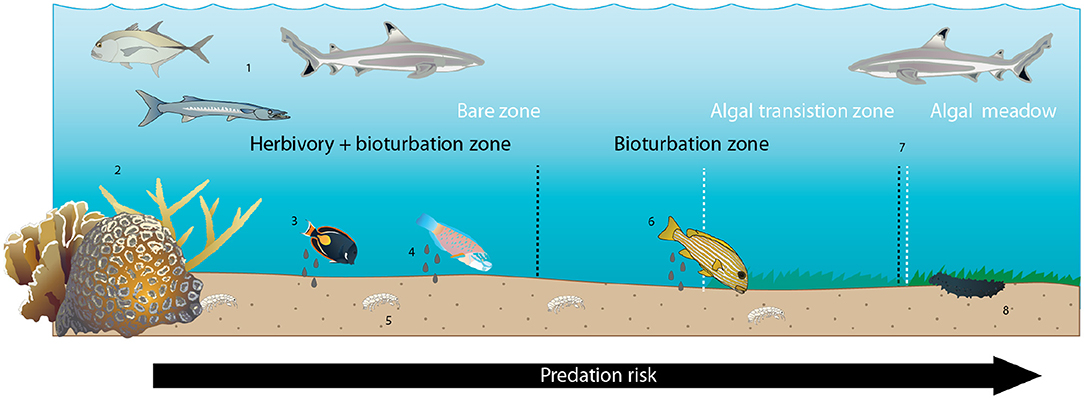
Figure 5. Conceptual diagram of proposed and hypothesized drivers of halos at Heron Island. Zones indicated by white text and dashed lines are benthic algal zones; zones indicated by black text and dashed lines are ecological process zones. Numbers below refer to numbered icons that reflect patterns and processes known, observed in this study, or assumed to occur. (1) Predators generate predation risk through the lagoon seascape. (2) Coral patch reefs provide shelter from predators for smaller-bodied potential prey, creating a gradient of predation risk that increases with distance from patch reef shelter. (3) Herbivorous prey species graze benthic algae in a distance-sensitive manner, preferentially foraging in the immediate vicinity of the patch reef. (4) Foraging fishes defecate where they forage, creating elevated detrital input in the vicinity of the patch reef. (5) Some small benthic and infaunal invertebrates preferentially inhabit areas of higher fish activity, presumably due to elevated detrital resources, though others do not. (6) Larger-bodied bioturbating fishes preferentially forage in the vicinity of the reef, presumably due to elevated infaunal prey surrounding the reef and/or increased predation risk farther from the reef. (7) Declining net herbivory + bioturbation at increasing distances from the reef leads to increasing coverage of benthic algae over this gradient. (8) Larger-bodied scavenging invertebrates (sea cucumbers) preferentially forage near the reef and in the algal meadow beyond the halo boundary, presumably due to elevated faecal- and algal-derived detrital food availability, respectively.
Our finding that herbivores rarely (if ever) forage as far as the halo boundary in our study location, but that bioturbators behave in a manner consistent with halo formation by reaching this boundary, adds a new dimension to a long-assumed suite of species interactions behind these large-scale habitat features. Specifically, bioturbation by sweetlips and emperors may explain the discrepancy in Heron lagoon between the outer boundary of where herbivores were observed (~10 m from patch reef edges, with the vast majority of feeding activity and highest per-capita bite rate no farther than 5 m; Figure 1C) and where some halo boundaries were observed [up to 14 m from reef edges, with a mean of ~9 m (Figure 1A), with increasing algal coverage up to 25 m (Figure 1B)]. Since halos were first documented in the Caribbean over 50 years ago (Randall, 1965), most studies have assumed that herbivory was the sole direct species interaction underlying them (Ogden et al., 1973; Sweatman and Robertson, 1994; Valentine and Heck, 2005; Armitage and Fourqurean, 2006; Valentine et al., 2007; Madin et al., 2011; Downie et al., 2013; Turgeon et al., 2014), with predation risk assumed to be an indirect species interaction governing herbivore foraging patterns (Randall, 1965; Ogden et al., 1973; Macintyre et al., 1987; Valentine et al., 2007; Madin et al., 2011; Downie et al., 2013; Atwood et al., 2018). Indeed, algal transplant assays conducted elsewhere (Valentine et al., 2008) and within a subset of the patch reefs studied here (Madin et al., 2011) demonstrate that grazers will forage into the halo in a distance-sensitive manner to graze on algae when it is artificially made available, supporting this assumption. Despite this common assumption, however, previous studies have also pointed to a range of other mechanisms as possible drivers of halos, such as local water movement due to current eddies (Steiner and Willette, 2014), sediment deposition (Garrett et al., 1971), incidental bioturbation via refuge-seeking animals (Glynn, 1985), nutrient toxicity from enhanced nutrient levels surrounding patch reefs (Alevizon, 2002), and spatially constrained bioturbation (Alevizon, 2002; Steiner and Willette, 2014). Although these mechanisms are not mutually exclusive, and one or more could theoretically be at work, all but foraging by herbivorous and bioturbating fishes were found to be insufficient in isolation to explain halos found in our and/or other study systems (Supplementary Information). To our knowledge, no previous studies have shown bioturbator foraging patterns that are consistent with halo formation. Our results suggest that herbivory is a key mechanism in forming halos within Heron lagoon to a maximum of ~10 m from patch reefs, and that bioturbators are likely key to extending halo boundaries beyond this range (Figure 5; see Supplementary Information).
Our observations of bioturbating fishes (sweetlips and emperors) foraging in a distance-sensitive manner relative to patch reefs (Figures 1E,F) are important for two reasons. First, one of these groups (emperors) is heavily targeted by fisheries within the Great Barrier Reef (Castro-Sanguino et al., 2017), and both are common targets in other reef systems globally, particularly where other fish stocks have been previously reduced by fishing. Removing these groups from an ecosystem may therefore have consequences for habitat structure, specifically formation of halos, and subsequently sedimentary carbon storage (Atwood et al., 2018). Second, using halos as indicators of species interactions, an idea proposed as an alternative to the high cost and limited feasibility in remote locations of traditional in-situ monitoring practices (Madin et al., 2011), will only be possible if we fully understand the suite of interactions and other conditions leading to their occurrence.
In line with the conclusions of recent studies quantifying coral reef predator effects on patterns of prey foraging (Rizzari et al., 2014; Gil et al., 2017; Rasher et al., 2017; Atwood et al., 2018), the most parsimonious explanation for the patterns we document of spatially constrained foraging is that predation risk limits foraging excursions of herbivorous and potentially also invertivorous (bioturbating) fishes, though other mechanisms may also be involved. Figure 3 suggests that herbivores may collectively forage at lower rates in the presence of large, mobile predators. Spatially-constrained foraging in the presence of predators is consistent with risk allocation theory (Lima and Dill, 1990; Brown, 1999) and is expected under central place foraging (Orians and Pearson, 1979). More specifically, predation risk is known to constrain the upper bound, but not necessarily the lower bound, of risky prey fish behaviors such as foraging (Madin et al., 2010b). This is because prey under low risk are able to engage in risky behaviors, but will not necessarily do so at all times, whereas prey under high risk must balance risk-taking with predator avoidance, leading to a consistently constrained upper bound of foraging distances. The wedge-shaped distribution of points in Figures 3A,B is consistent with this expectation. Additionally, herbivores' per-capita bite rate peaked away from the reef's edge between 4.7 and 10.3 m (Figure 4A). This finding is consistent with those of both Catano et al. (2016) and Gil et al. (2017) from other coral reef locations that found reduced aggregate herbivory, yet higher individual bite rates, in riskier habitats. Analogous patterns of spatially constrained foraging by herbivores, detritivores, and planktivores in another reef system have been found through a combination of theoretical and empirical work to be the result of predation risk (Madin et al., 2010a,b). None of the possible explanations other than predation risk would be expected to lead to the spatially constrained foraging pattern we observed in herbivores (Table S3). Despite the fact that herbivores' potential predators spend greater amounts of time near patch reefs than farther away (Figures 1G,H), coinciding with areas frequented by herbivores, this area contains a means to avoid or escape interactions with those predators, i.e., the reef matrix. The lack of physical refuge within the bare halo and the algal meadows beyond, combined with the composition of Heron lagoon's predator assemblage (i.e., including many large, mobile predators) means that although predator encounters in those areas are less frequent, such encounters increase in risk for herbivores the farther they are from the reef refuge (Figure 5; Gil et al., 2017). When feeding in sand areas adjacent to patch reefs—where predators are most abundant—herbivores reduce encounter probability with predators through tidally-driven temporal behavioral adjustments (Atwood et al., 2018).
Bioturbating fishes such as emperors and sweetlips are also at greater risk of predation with increasing distance from reef shelter, but to a lesser extent than smaller herbivores (~25–70 cm adult TL max; smaller for juveniles) given bioturbators' larger (~85–100 cm TL max) body size. Similar to herbivores, per-capita bite rates for both of these families peaked away from the reef's edge, with sweetlips peaking between 7.2 and 12.8 m (Figure 4B) and emperors peaking between 12.2 and 17.8 m from reef's edge (Figure 4C). Again, this pattern is consistent with that which would be expected under a gradient of predation risk on coral reefs (Catano et al., 2016; Gil et al., 2017). Specifically, in risky, exposed areas, prey must divide their time between vigilance and taking bites from the substrate (which precludes vigilance), resulting in a higher per capita bite rate due to the need to acquire sufficient energetic resources in a shorter amount of time. However, these patterns may also be explained by changes in the quantity or quality of invertebrates, or by differences in small-scale abiotic factors (such as water motion). Bioturbating sweetlips were observed foraging exclusively at night and emperors foraged during both day and night, whereas large potential predators of these fishes (i.e., sharks) were observed exclusively during daytime. This temporal separation precludes analysis of the relationship between shark presence and these bioturbators' activity, yet it suggests that adaptive temporal niche partitioning may be occurring—a well-known risk-mitigating strategy in marine animals (Heithaus et al., 2012; Rasher et al., 2017; Atwood et al., 2018). It is also possible that bioturbating fishes' spatially constrained foraging may be a response to food distribution or parasite avoidance (Table S3). Ollivier et al. (2018) found that overall community composition of benthic (infaunal) invertebrates varied significantly with distance from Heron lagoon patch reef edges, but that taxon-specific abundance patterns were variable and did not indicate clear trends in any particular taxa relative to distance from reefs.
Bioturbating sea cucumbers cannot necessarily detect nor move rapidly away from predators, nor are they likely prey for the dominant fish predators we observed. While sea cucumber diets are known to include both plant/algae and animal-based detrital food sources, stable isotope analyses from another location and species have shown that benthic macro- and micro-algae constitute the bulk of their diets (i.e., 30.9–67.4%; Sun et al., 2013). Algal-derived detrital food availability for these scavengers presumably increases with distance from patch reefs due to the higher standing stock and lower consumption by herbivores of algae farther from patch reefs. Food availability is therefore a likely explanation for sea cucumbers' distribution. Because sea cucumbers move extremely slowly relative to fishes and have relatively low densities per reef in our system, their net effect on algae removal via bioturbation may be more limited than that of more mobile fishes. This may help explain why algal meadows persist in the areas of highest sea cucumber density (Figure 1).
In summary, we propose that predation risk is likely a key mechanism leading to the spatially constrained foraging patterns of herbivores and potentially bioturbating fishes that create halos, and that a secondary effect of these patterns is spatially structured sea cucumber foraging (Figure 5).
The use of observational data necessarily results in limitations to what can be concluded. For example, attribution of causality based on correlational data alone is difficult, particularly with low samples sizes such as in the case with our dusk/night surveys. However, when considered in conjunction with previous results from the literature and the expectations generated from competing hypotheses (Table S3), our observational data allow us to rule out a number of possible mechanisms (Sagarin and Pauchard, 2010) for the spatial foraging patterns we observed. As with many observational approaches applied to ecological questions on large spatial and temporal scales, these results provide insights that could not be generated from more definitive, yet smaller-scale, manipulative studies (Sagarin and Pauchard, 2010).
Additional research into the suite of species interactions that collectively govern the formation and regulation of halos is necessary to understand this well-known tropical phenomenon. For example, disentangling the relative roles and importance of the various ecological players involved in the species interactions behind halos could potentially be achieved through the collection of both behavioral and species abundance data within “natural experiments,” where herbivore and/or predator populations have been altered by human actions such as fishing or marine reserves. Alternatively, large-scale, in-situ species manipulations and exclusions would lend valuable insight, though logistical constraints may render such studies impossible at the spatial and temporal scales required in some systems and settings. Where they are possible, however, doing so at multiple locations globally will lend the greatest insight into the mechanisms behind halos and will help us understand their potential as the basis of scientific and conservation tools. Importantly, smaller-scale experiments that generate time-series data on halo creation, and in particular how each functional group contributes to halo formation, would yield valuable insight.
Our finding that multiple types of small-scale species interactions may collectively structure habitat at landscape scales in a self-organized way (Rietkerk and van de Koppel, 2008) suggests that halos could serve as indicators of large-scale change within coral reefs. For example, human activities such as fishing that reduce particular functional groups (e.g., herbivorous or bioturbating fishes) might be expected to indirectly impact halo occurrence and/or width by virtue of reducing their role in halo formation. Such human activities have been shown in other systems to dramatically affect species interactions, in turn altering physical habitat over very large spatial and temporal scales (e.g., the classic killer whale/sea otter/urchin/kelp forest example; Estes et al., 1998). Changes to halo presence or size may also have further ecosystem-level effects. For example, halos may be hypothesized to affect ecosystem processes, such as coral recruitment, in systems where hard-bottom (e.g., “pavement”) substrate underlies bare halo zones (e.g., Downie et al., 2013) because herbivory is known to both promote (via reducing algae) and inhibit (via scraping of coral recruits) coral recruitment (Mumby, 2009). Similarly, halos have been shown to affect at least one ecosystem function, sedimentary carbon storage, through the same pathway of predator-prey interactions (Atwood et al., 2018). Further, benthic primary producers play a critical role in a wide range of other ecosystem processes and functions, such as provision of food and habitat for mobile species, stabilization of sediments, and nutrient cycling, among many others. Halos may therefore provide large-scale indicators of how some species densities, species interactions, ecosystem processes, and/or ecosystem functions are changing over space or time. Because of their relatively large spatial scale, remote methods of observation (e.g., satellites, drones, and other aerial tools) will provide a means of doing so that may not be otherwise feasible. The accessibility of these type of remote sensing data sets is rapidly increasing with “cube satellites” revisiting every place on earth daily with 3 m pixel resolution, providing an ideal tool to explore high temporal resolution patterns as well (Thompson et al., 2017). By coupling these new and emerging technologies with behavioral ecology, halos hold potential as a tool for remotely observing ecological interactions and assessing both natural and human-induced ecosystem change now and into the future.
Author Contributions
EM conceived the study. EM and AH designed and led field studies. EM, AH, TA, and OL collected field data. OL processed and analyzed video imagery. CR provided satellite imagery. KP analyzed satellite imagery and performed data analyses. KP and EM wrote the first draft of the manuscript. All authors provided intellectual input and contributed to manuscript revisions.
Conflict of Interest Statement
The authors declare that the research was conducted in the absence of any commercial or financial relationships that could be construed as a potential conflict of interest.
Acknowledgments
We thank Guillermo Diaz-Pulido for algal specimen identification. EM was generously supported by the World Wildlife Fund's Kathryn S. Fuller Science for Nature Fund, a US National Science Foundation International Postdoctoral Fellowship (OISE-0853117), and several Google Earth Education Initiative in-kind grants. EM and AH were partly funded by Australian Research Council DECRA Fellowships (Fellowship DE120102614 to EM and Fellowship DE120102459 to AH). Remote sensing imagery funding came from an Australian Research Council Discovery Grant (DP0663 to S. Phinn and P. Mumby), the World Bank Global Environments Facility's Coral Reef Targeted Research program (to P. Mumby and S. Phinn), a CSIRO Collaborative Research Grant, the Remote Sensing Research Centre at the University of Queensland, and Digital Globe. This is contribution #124 from the Center for Coastal Oceans Research in the Institute for Water and Environment at Florida International University.
Supplementary Material
The Supplementary Material for this article can be found online at: https://www.frontiersin.org/articles/10.3389/fevo.2019.00102/full#supplementary-material
References
Alevizon, W. (2002). Enhanced seagrass growth and fish aggregations around Bahamian patch reefs: the case for a functional connection. Bull. Mar. Sci. 70, 957–966.
Armitage, A. R., and Fourqurean, J. W (2006). The short-term influence of herbivory near patch reefs varies between seagrass species. J. Exp. Mar. Biol. Ecol. 339, 65–74. doi: 10.1016/j.jembe.2006.07.013
Atwood, T. B., Madin, E. M. P., Harborne, A. R., Hammill, E., Luiz, O. J., Ollivier, Q. R., et al. (2018). Predators shape sedimentary organic carbon storage in a coral reef ecosystem. Front. Ecol. Evol. 6:110. doi: 10.3389/fevo.2018.00110
Bartholomew, B. (1970). Bare zone between california shrub and grassland communities: the role of animals. Science 170, 1210–1212. doi: 10.1126/science.170.3963.1210
Brown, J. S. (1999). Vigilance, patch use and habitat selection: foraging under predation risk. Evolu. Ecol. Res. 1, 49–71.
Castro-Sanguino, C., Bozec, Y.-M., Dempsey, A., Samaniego, B. R., Lubarsky, K., Andrews, S., et al. (2017). Detecting conservation benefits of marine reserves on remote reefs of the northern GBR. PLoS ONE 12:e0186146. doi: 10.1371/journal.pone.0186146
Catano, L. B., Rojas, M. C., Malossi, R. J., Peters, J. R., Heithaus, M. R., Fourqurean, J. W., et al. (2016). Reefscapes of fear: predation risk and reef heterogeneity interact to shape herbivore foraging behaviour. J. Anim. Ecol. 85, 146–156. doi: 10.1111/1365-2656.12440
de Paoli, H., van der Heide, T., van den Berg, A., Silliman, B. R., Herman, P. M. J., and van de Koppel, J. (2017). Behavioral self-organization underlies the resilience of a coastal ecosystem. Proc. Natl. Acad. Sci. U.S.A. 114:201619203. doi: 10.1073/pnas.1619203114
Downie, R. A., Babcock, R. C., Thomson, D. P., and Vanderklift, M. A. (2013). Density of herbivorous fish and intensity of herbivory are influenced by proximity to coral reefs. Mar. Ecol. Progr. Series 482, 217–225. doi: 10.3354/meps10250
Estes, J., Tinker, M., Williams, T., and Doak, D (1998). Killer whale predation on sea otters linking oceanic and nearshore ecosystems. Science 282, 473–476. doi: 10.1126/science.282.5388.473
Garrett, P., Smith, D. L., Wilson, A. O., and Patriquin, D. (1971). Physiography, ecology, and sediments of two Bermuda patch reefs. J. Geol. 79, 647–668. doi: 10.1086/627696
Getzin, S., Yizhaq, H., Bell, B., Erickson, T. E., Postle, A. C., Katra, I., et al. (2016). Discovery of fairy circles in Australia supports self-organization theory. Proc. Natl. Acad. Sci. 113, 3551–3556. doi: 10.1073/pnas.1522130113
Gil, M. A., Zill, J., and Ponciano, J. M. (2017). Context-dependent landscape of fear: algal density elicits risky herbivory in a coral reef. Ecology 98, 534–544. doi: 10.1002/ecy.1668
Glynn, P. (1985). El Nino-associated disturbance to coral reefs and post disturbance mortality by Acanthaster planci. Mar. Ecol. Progr. Series 26, 295–300. doi: 10.3354/meps026295
Heithaus, M. R., Wirsing, J. A., and Dill, L. M (2012). The ecological importance of intact top-predator populations: a synthesis of 15 years of research in a seagrass ecosystem. Mar. Freshwater Res. 63, 1039–1050. doi: 10.1071/MF12024
Hobbs, R. J., Higgs, E., and Harris, J. A. (2009). Novel ecosystems: implications for conservation and restoration. Trends Ecol. Evolu. 24, 599–605. doi: 10.1016/j.tree.2009.05.012
Lima, S. L., and Dill, L. M. (1990). Behavioral decisions made under the risk of predation: a review and prospectus. Can. J. Zool. 68, 619–640. doi: 10.1139/z90-092
Macintyre, I. G., Graus, R. R., Reinthal, P. N., Littler, M. M., and Littler, D. S. (1987). The barrier reef sediment apron: tobacco reef, belize. Coral Reefs 6, 1–12. doi: 10.1007/BF00302206
Madin, E. M. P., Gaines, S. D., Madin, J. S., and Warner, R. R. (2010a). Fishing indirectly structures macroalgal assemblages by altering herbivore behavior. Am. Nat. 176, 785–801. doi: 10.1086/657039
Madin, E. M. P., Gaines, S. D., and Warner, R. R. (2010b). Field evidence for pervasive indirect effects of fishing on prey foraging behavior. Ecology 91, 3563–3571. doi: 10.1890/09-2174.1
Madin, E. M. P., Madin, J. S., and Booth, D. J. (2011). Landscape of fear visible from space. Sci. Rep. 1:14. doi: 10.1038/srep00014
Mumby, P. J. (2009). Herbivory versus corallivory: are parrotfish good or bad for Caribbean coral reefs? Coral Reefs 28, 683–690. doi: 10.1007/s00338-009-0501-0
Ogden, J., Brown, R., and Salesky, N. (1973). Grazing by the echinoid Diadema antillarum philippi: formation of Halos around West Indian Patch Reefs. Science 182, 715–717. doi: 10.1126/science.182.4113.715
Ogden, J. C. (1976). Some aspects of herbivore-plant relationships on Caribbean reefs and seagrass beds. Aqua. Botany 2, 103–116. doi: 10.1016/0304-3770(76)90013-9
Ollivier, Q. R., Hammill, E., Booth, D. J., Madin, E. M. P., Hinchliffe, C., Harborne, A. R., et al. (2018). Benthic meiofaunal community response to the cascading effects of herbivory within an algal halo system of the Great Barrier Reef. PLoS ONE 13:e0193932. doi: 10.1371/journal.pone.0193932
Orians, G. H., and Pearson, N. E. (1979). “On the theory of central place foraging,” in Analysis of Ecological Systems, eds D. J. Horn, G. R. Stairs, and R. D. Mitchell (Columbus, OH: Ohio State University Press), 155–177.
Phinn, S. R., Roelfsema, C. M., and Mumby, P. J. (2012). Multi-scale image segmentation for mapping coral reef geomorphic and benthic community zone. Int. J. Remote Sens. 33, 3768–3797. doi: 10.1080/01431161.2011.633122
QGIS Development Team (2016). QGIS Geographic Information System. Open Source Geospatial Foundation Project. Available online at: http://qgis.osgeo.org
Randall, J. E. (1965). Grazing effect on sea grasses by herbivorous reef fishes in the West Indies. Ecology 46, 255–260. doi: 10.2307/1936328
Rasher, D. B., Hoey, A. S., and Hay, M. E. (2017). Cascading predator effects in a Fijian coral reef ecosystem. Sci. Rep. 7:15684. doi: 10.1038/s41598-017-15679-w
Rietkerk, M., and van de Koppel, J. (2008). Regular pattern formation in real ecosystems. Trends Ecol. Evolu. 23, 169–175. doi: 10.1016/j.tree.2007.10.013
Rizzari, J. R., Frisch, A. J., Hoey, A. S., and McCormick, M. I. (2014). Not worth the risk: apex predators suppress herbivory on coral reefs. Oikos 123, 829–836. doi: 10.1111/oik.01318
Roelfsema, C., Kovacs, E., Roos, P., Terzano, D., Lyons, M., and Phinn, S. (2018). Use of a semi-automated object based analysis to map benthic composition, Heron Reef, Southern Great Barrier Reef. Remote Sens. Lett. 9, 324–333. doi: 10.1080/2150704X.2017.1420927
Ruiz-Reynés, D., Gomila, D., Sintes, T., Hernández-García, E., Marbà, N., and Duarte, C. M. (2017). Fairy circle landscapes under the sea. Sci. Adv. 3:e1603262. doi: 10.1126/sciadv.1603262
Sagarin, R., and Pauchard, A. (2010). Observational approaches in ecology open new ground in a changing world. Front. Ecol. Environ. 8, 379–386. doi: 10.1890/090001
Steiner, S. C. C., and Willette, D. A. (2014). Dimming sand halos around coral reefs in Dominica : new expansion corridors for the invasive seagrass Halophila stipulacea. ITME Res. Rep. 328, 1–3.
Sun, Z., Gao, Q., Dong, S., Shin, P. K. S., and Wang, F. (2013). Seasonal changes in food uptake by the sea cucumber Apostichopus japonicus in a farm pond: evidence from C and N stable isotopes. J. Ocean Univ. China 12, 160–168. doi: 10.1007/s11802-012-1952-z
Sweatman, H., and Robertson, D. R. (1994). Grazing halos and predation on juvenile Carribbean surgeonfishes. Mar. Ecol. Prog. Ser. 111, 1–6. doi: 10.3354/meps111001
Tarnita, C. E., Bonachela, J. A., Sheffer, E., Guyton, J. A., Coverdale, T. C., Long, R. A., et al. (2017). A theoretical foundation for multi-scale regular vegetation patterns. Nature 541, 398–401. doi: 10.1038/nature20801
Thompson, D. R., Hochberg, E. J., Asner, G. P., Green, R. O., Knapp, D. E., Gao, B.-C., et al. (2017). Airborne mapping of benthic reflectance spectra with Bayesian linear mixtures. Remote Sens. Environ. 200, 18–30. doi: 10.1016/j.rse.2017.07.030
Turgeon, K., Robillard, A., Grégoire, J., Duclos, V., and Kramer, D. L. (2014). Functional behavioral a reef fish connectivity from perspective: behavioral tactics for moving fragmented landscape. Ecology 91, 3332–3342. doi: 10.1890/09-2015.1
Valentine, J. F., and Heck, K. L. (2005). Perspective review of the impacts of overfishing on coral reef food web linkages. Coral Reefs 24, 209–213. doi: 10.1007/s00338-004-0468-9
Valentine, J. F., Heck, K. L., Blackmon, D., Goecker, M. E., Christian, J., Kroutil, R. M., et al. (2007). Food web interactions along seagrass-coral reef boundaries: effects of piscivore reductions on cross-habitat energy exchange. Mar. Ecol. Progr. Ser. 333, 37–50. doi: 10.3354/meps333037
Keywords: coral reef, reef fish, halo, behavior, self-organization, species interactions
Citation: Madin EMP, Precoda K, Harborne AR, Atwood TB, Roelfsema CM and Luiz OJ (2019) Multi-Trophic Species Interactions Shape Seascape-Scale Coral Reef Vegetation Patterns. Front. Ecol. Evol. 7:102. doi: 10.3389/fevo.2019.00102
Received: 04 October 2018; Accepted: 13 March 2019;
Published: 24 April 2019.
Edited by:
Evan Preisser, University of Rhode Island, United StatesReviewed by:
Hauke Reuter, Leibniz Centre for Tropical Marine Research (LG), GermanyShelby A. Rinehart, Hebrew University of Jerusalem, Israel
Copyright © 2019 Madin, Precoda, Harborne, Atwood, Roelfsema and Luiz. This is an open-access article distributed under the terms of the Creative Commons Attribution License (CC BY). The use, distribution or reproduction in other forums is permitted, provided the original author(s) and the copyright owner(s) are credited and that the original publication in this journal is cited, in accordance with accepted academic practice. No use, distribution or reproduction is permitted which does not comply with these terms.
*Correspondence: Elizabeth M. P. Madin, ZW1hZGluQGhhd2FpaS5lZHU=