- 1Department of Biology, University of British Columbia – Okanagan, Kelowna, BC, Canada
- 2Summerland Research and Development Centre, Agriculture and Agri-food Canada, Summerland, BC, Canada
- 3Department of Plant Sciences, University of Saskatchewan, Saskatoon, SK, Canada
Although plants are known to have a strong influence on soil biota, the effect of groundcover vegetation in perennial cropping systems on soil fungi has been little explored. We surveyed extensively managed vineyards to determine how plant community functional characteristics, soil factors, and irrigation management related to the abundance of two guilds of soil fungi that may play a role in plant-soil feedback (entomopathogenic fungi represented by Beauveria bassiana, and the pathogenic species complex, Ilyonectria spp.). We found that plant community characteristics were related to fungal abundance for both fungi assayed. Beauveria bassiana increased with native species, annual plants, and legumes consistently across sampling periods. Ilyonectria spp. increased with the abundance of forbs and exotic species, though only the relationship with forbs was consistent across sampling periods. Both fungal guilds increased with increasing soil organic matter. The use of dual or sprinkler irrigation systems also increased B. bassiana and Ilyonectria spp. in vineyard soils. Overall, groundcover vegetation played a significant role in driving abundance of these important groups of soil fungi. Groundcover management may therefore be a viable tool to manipulate soil fungi with the potential for improving ecosystem services such as conservation biological control of soil dwelling insect pests and deterring pathogens in perennial cropping systems.
Introduction
Perennial agriculture is characterized by crop rows alternating with drive rows to facilitate field and tractor work. This means that much of the land area in a perennial cropping system is not actually planted to crop plants, but subjected to floor management practices. Depending on regional climate patterns, pest pressure, nutrient challenges, and aesthetics, different strategies are used to manage drive rows. Perhaps the most common approach is the maintenance and management of vegetation in the drive row using cover crops or groundcovers that provide a host of ecosystem services, such as improved carbon sequestration, pest control, and soil fertility (Winter, 2018). With increasing interest in the linkage between soil microbial diversity and ecosystem functioning (Bardgett and van der Putten, 2014) and the potential for groundcover vegetation to affect crop plant health through plant-soil feedbacks (Vukicevich et al., 2018), a logical question is: how does groundcover vegetation affect key groups of soil biota?
Plants alter the spatial distribution of soil resources through rhizodeposition (Badri and Vivanco, 2009) and litter decomposition (Fanin et al., 2014), creating unique nutrient rich patches that vary with plant species (Broeckling et al., 2008; De Deyn et al., 2011). In fact, specific plants have been used by farmers for millennia to affect changes in populations of soil microorganisms, e.g., through crop rotations (Bullock, 1992). Because rotation of the crop plant is not possible in perennial vineyards and orchards, the drive row then provides an opportunity to introduce plant diversity and subsequently soil microbial diversity to the system (Vukicevich et al., 2016). Cover crops or permanent groundcovers could have particularly pronounced effects on important crop pathogens and mutualists as evidenced by the strong effects of plant identity on soil fungi (De Bellis et al., 2007; De Deyn et al., 2011; Corneo et al., 2013; Lankau and Lankau, 2014; Detheridge et al., 2016).
One beneficial group of soil fungi in agriculture is the entomopathogenic (EP) fungi. EP fungi, typified by the well-studied and commercially sold Beauveria bassiana and Metarhizium anisopliae, are naturally common in soils where they are responsible for regulation of insect pest communities and appreciated as biocontrol agents (Shah and Pell, 2003; Pell et al., 2010). Living in close association with plants (Moonjely et al., 2016), they are even able to transfer N from infected insects to a plant host in return for plant carbon (Behie et al., 2012, 2017). They have also been implicated in plant protection from soilborne disease (Ownley et al., 2010). Because they may show rhizosphere specificity to some degree (Hu and St. Leger, 2002; Behie et al., 2015) and are preferentially associated with certain types of habitats (Meyling et al., 2009), vineyard groundcover management might also affect the abundance of these beneficial fungi thereby promoting positive plant-soil feedback through regulation of soil dwelling insect herbivores and decreases in plant disease.
In addition to beneficial soil fungi, generalist soil borne plant pathogens that harm woody perennial crops may build up on certain alternate host plants. For example, Agustí-Brisach et al. (2011) found Ilyonectria spp., the causal agent of black foot disease of grape, living in various asymptomatic common vineyard weeds. Because some groundcover plants may be good hosts for these pathogens, there is potential for spillover onto grapevine roots that occupy the same soil space. The perceived benefit of increased microbial diversity through enhanced vegetative diversity may be negated if these generalist pathogens accumulate in non-crop vegetation and promote establishment of disease in vines. On the other hand, certain groundcover plants have been seen to decrease the prevalence of these fungi and improve replant outcomes in crops such as apple (Manici et al., 2015). The choice of groundcover may therefore contribute to negative plant-soil feedback on vines if it promotes pathogens like Ilyonectria spp. or minimize negative feedbacks if it deters these fungi.
Understanding how to manage groundcover vegetation for positive plant-soil feedback via increasing beneficial fungi while deterring pathogenic fungi could improve the sustainability of perennial crop production (Vukicevich et al., 2016). Though some work has shown that vegetation management can increase overall microbial biomass and activity in vineyard soils (Ingels et al., 2005; Whitelaw-Weckert et al., 2007; Steenwerth and Belina, 2008), how groundcovers may change key fungal guilds over time remains largely unknown.
We sampled groundcover plant communities and associated soils from vineyards in the Okanagan valley, British Columbia. This provided a variety of groundcover management practices that already exist in vineyards in this region to test for effects on soil microbes in real world cropping scenarios. Across these vineyards, we studied how groundcover vegetation affects the abundance of the common EP fungus, B. bassiana, and plant pathogenic Ilyonectria spp. We used a model-selection approach to identify which plant community characteristics, irrigation techniques, and soil physicochemical factors affected the abundance of each of these fungal guilds as measured by digital droplet PCR (ddPCR) assays. Although there are no published studies that look specifically at how plant functional characteristics affect these groups of soil fungi, we made some predictions based on a review of related literature (Vukicevich et al., 2016). Specifically, we predicted that native plants might increase the abundance of EP fungi (Meyling et al., 2009). Legumes in groundcovers might also be expected to increase EP fungi (Shapiro-Ilan et al., 2012). We expected that Ilyonectria spp. might accumulate under legumes (Benitez et al., 2016), but might be less abundant with grasses as grasses seem to be less suitable hosts compared to other vineyard floor vegetation (Agustí-Brisach et al., 2011).
Methods
Field Sites
All five field sites were located in the southern Okanagan Valley (British Columbia, Canada) (from 49°33′52.44″N, 119°38′19.55″W south to 49° 4′44.02″N, 119°30′41.78″W). This region receives on average ~320 mm of precipitation annually in the form of snow in winter months and occasional rainfall in spring, summer, and fall. We chose vineyard sites that had different vegetation management schemes within the same vineyard block, i.e., alternating rows or randomized complete block designs. Sites were chosen independent of soil type or management practices, which were controlled for statistically. Site characteristics, including groundcover schemes and irrigation types, are given in Table 1.
Plant Community Assessment
We quantified plant communities using quadrats measuring 25 × 50 cm. Four quadrats were evenly spaced throughout four rows of each management scheme (in the case of alternating rows at sites BM, MH, and BC) or four replicate plots (in the case of randomized complete block designs at sites PME and PCH) making for 16 quadrats per management scheme at each site at each sampling period. Nearly 800 plant communities and concomitant soil samples were analyzed in total over the course of this study. Details of sample collection at each site are given in Supplementary Materials. Vines adjacent to quadrat placement were marked to facilitate sample collection from the same location at each sampling event. Visual estimation of percent coverage of the quadrat by each plant species was used as a proxy for relative abundance.
Soil Collection and Processing
We collected three soil cores (2.5 × 20 cm) per quadrat, pooled them in sealed plastic bags and kept them on ice for transport to a −20°C freezer. Samples were then weighed, oven dried at 60°C for 72 h to ensure DNA extraction from equal quantities of soil in each sample, and sieved to 2 mm to remove most roots and rocks and homogenize samples. Dried samples were stored at −20°C until use.
Soil Physicochemical Factors
As soil abiotic factors may also be important determinants of fungal abundance in soils, a composite sample of post-processed (after drying and sieving) soil for each treatment was sent to Zenalytic Laboratories (Kelowna, BC) for analysis of organic matter (by loss on ignition) (Davies, 1974), Total N (Kjeldahl, 1883), Extractable P (Mehlich-3 ICP)(Mehlich, 1984), and pH (1:1 in water) (Jackson, 1956).
Molecular Analysis
A 0.5 g subsample was then taken for DNA extraction and quantification of fungi. Genomic DNA was extracted from bulk soil using the FastPrep spin kit for soils (MP Biomedical, Carlsbad, CA) following the manufacturer's instructions. Quantification of target fungal groups was then performed using digital droplet PCR (ddPCR). Each protocol was optimized through the use of dilution series and melt curve analysis in qPCR and annealing/extension temperature gradients with both positive pure culture controls and positive environmental samples in ddPCR.
To quantify the EP fungus we used the primer set BB.fw/BB.rv (Landa et al., 2013), which targets B. bassiana at the species level. The following was used in a 20 μL final reaction volume: 10 μL QX200 ddPCR EvaGreen supermix (BioRad, Livermore, CA), 250 nM each primer, 2 μL DNA template, and 7 μL nuclease-free water. Droplets were prepared using BioRad droplet generation cartridges and a QX100 droplet generator. Reaction conditions consisted of initial denaturing at 95°C for 10 min followed by 40 cycles of denaturing at 95°C for 30 s, annealing/extension at 56°C for 2 min, 4°C for 5 min, and 90°C for 5 min. To quantify the plant pathogenic Ilyonectria spp. we used the primer set YT2F (Tewoldemedhin et al., 2011) and CYLR (Dubrovsky and Fabritius, 2007), which targets Ilyonectria spp. (species complex) that cause black foot disease of grape. This primer set has been used to evaluate abundance of Ilyonectria spp. (including I. macrodidyma and I. liriodendri) in nursery soils (Agusti-Brisach et al., 2014) as well as I. macrodidyma, I. pauciseptatum, Cylindrocarpon destructans, and I. liriodendri in diseased apple roots (Tewoldemedhin et al., 2011). PCR trials using this primer set in our lab also indicate positive amplification for I. torresensis, I. europaea, I. ianthothele, I. gamsii, Dactylonectria pauciseptum, Clyindrocarpon cylindroides, and C. olidum, all of which are known to cause black foot disease of grape (Úrbez-Torres et al., 2015). The same recipe and reaction conditions were used as described above except for the annealing/extension step was 60°C for 1 min.
After PCR, droplets were read for fluorescence in a QX200 droplet reader (BioRad, Livermore, CA). Only samples with >10,000 droplets were used for analysis. Raw amplitude and cluster data was exported from Quantasoft version 1.7 (BioRad, Livermore, CA) and the open source software “ddPCRquant” (Trypsteen et al., 2015) was used to determine amplicon concentration of each sample.
Data generated during this project can be viewed on the Open Science Framework, following this link: https://osf.io/cxesk/?view_only=f513573264b141389af7cf23e67200b0.
Data Analysis
Quantification of Plant Community Characteristics
To assess the effect of groundcover vegetation on abundance of our two fungal guilds, we first calculated the abundance and species richness of the plant community, as well as the abundance of different functional traits within the community. Plant abundance was calculated as the total % cover of all vascular plants, whereas species richness was the sum of the number of species. For functional traits, we focused on life history strategy (annual, annual/biennial, biennial, biennial/perennial, and perennial), origin (native/exotic), mycorrhizal status (±) and plant functional group (grass, forb, or legume). For the life history strategies, we coded the different strategies ordinally by increasing length (1 = annual, 2 = annual/biennial, etc.), and calculated the community weighted mean using the R package “FD” (Laliberté and Legendre, 2010). We also coded origin and mycorrhizal status ordinally (0 = native, 1 = exotic, and 0 = non-mycorrhizal, 1 = mycorrhizal) and calculated community weighted means, resulting in indices representing the weighted abundance of exotic and mycorrhizal plants within each quadrat. Exotic plants included both seeded exotic groundcover species as well as exotic weedy species. For plant functional groups, we did not recode the groups as there was no obvious order among them. Instead, we calculated the community weighted mean of % cover of each category; however, forb abundance was not used in subsequent models to avoid extreme collinearity among the indicators.
Determination of Effects Using Model Selection
To determine which factors affect the abundance of fungal taxa, we used mixed models in the R package lme4 (Bates et al., 2015) along with model selection. We used separate mixed models for each fungal group (B. bassiana, and Ilyonectria spp.). Within the mixed models, we included irrigation type, soil characteristics (phosphorus, pH, and organic matter) and plant community characteristics (total cover, species richness, plant life history strategy, mycorrhizal status, origin, and functional groups) as fixed effects. Given that microbial abundance was quantified in multiple seasons and years, we also included interaction terms between each of these predictors and the sampling period. As random effects, we included block nested within site and quadrat nested within block to account for spatial structuring of samples and inherent site differences.
To reduce the complexity of these models, we used a combination of model and variable selection. First, we ran all possible combinations of the models using the dredge function in the R package MuMIn (Barton, 2017). These models were then ranked by their AICc score relative to the most parsimonious model (ΔAICc). Models with a ΔAICc score > 2 were considered uninformative and not considered further (Burnham and Anderson, 2002). Using this subset of models, we weighted each variable using the sums of the ΔAICc scores for the models in which they were included using the model.avg function in “MuMIn.” Variables with a weight > 0.7 were considered important and included in the final model. This procedure was repeated separately for each microbial group. Outputs from the model.avg function listing the average importance of all variables tested across all models run using the dredge function are given in Tables S1, S2. For the final models, we also estimated R2 values, partitioned between the fixed effects and fixed plus random (Nakagawa and Schielzeth, 2013), as implemented in MuMIn.
To aid the interpretation of the effects of sampling periods, we calculated the estimated marginal means for each sampling period using the R package “emmeans” (Lenth, 2018). Additionally, we used the “emtrends” function within this package to compare the slopes between sampling periods in cases where there were significant interactions with the continuous predictors. To enable comparison among indicators, each indicator variable was scaled to a mean of zero and divided by the standard deviation prior to calculating the trends.
Results are organized into two categories based on the two individual models (B. bassiana, and Ilyonectria spp.) and then based on three subcategories (“plant effects,” “soil effects,” and “irrigation and time effects”) for ease of interpretation and discussion. Full results for each model can be obtained from the Open Science Framework by following this link: https://osf.io/7qctx/?view_only=e0c567cad4f74a57a81185640b93d62a.
Results
Beauveria bassiana
The final model for B. bassiana included sample period, irrigation type, plant life history strategy, exotic species, legumes, grasses, organic matter, and soil P, as well as sampling period interaction terms with irrigation type, grasses, legumes, and soil P (Table 2). The fixed effects in this model explained 19% of the variation in B. bassiana abundance, while 54% of the variation was explained by fixed plus random effects (vineyard site and spatial structuring of sampling). Sampling period affected the abundance of B. bassiana [F(3, 717) = 21.93, P < 0.001], with abundance increasing in spring 2017 compared to spring 2016.
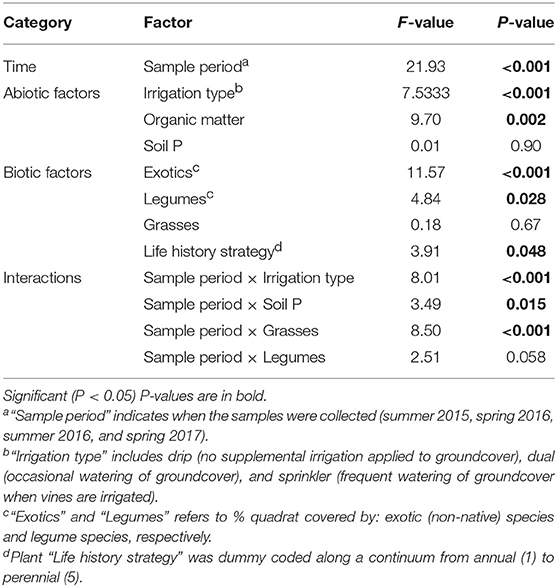
Table 2. Effect of sampling date and biotic and abiotic factors in the model of Beauveria bassiana abundance as tested using Satterwaite type III approximation for degrees of freedom (Model AIC: 2,166, R2fixed = 0.19).
Plant Effects on Beauveria bassiana
The abundance of B. bassiana was consistently related to plant life history strategy, proportion of exotic species, and legumes (Table 2). B. bassiana decreased with average plant lifespan [F(1, 586) = 3.91, P = 0.048], increased with the proportion of native plant species [F(1, 670) = 11.57, P < 0.001], and increased with legume cover [F(1, 667) = 4.84, P = 0.03] (Figure 1A). These plant effects were consistent across the experiment, i.e., did not depend on sampling period. The effect of grass cover was not significant overall, but was positively associated with B. bassiana abundance in spring of 2017.
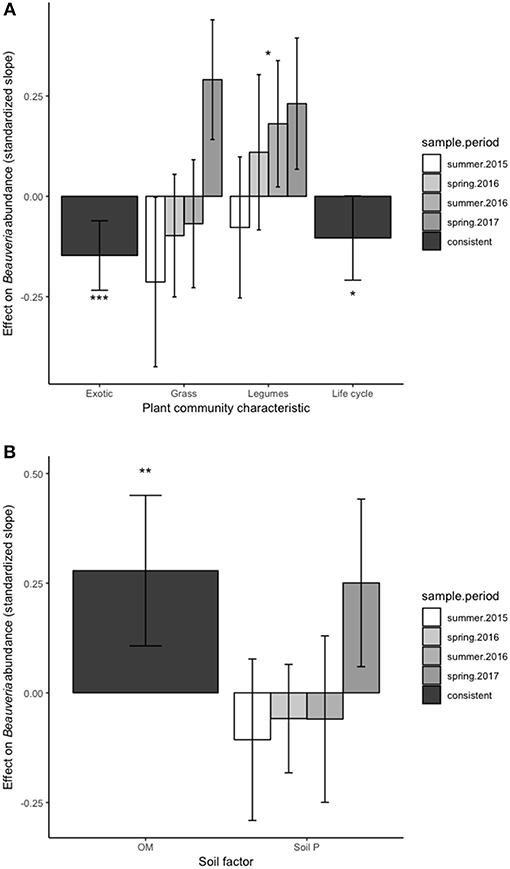
Figure 1. Effect of plant community characteristics (A) and soil properties (B) on Beauveria bassisana abundance. Positive values indicate a positive relationship (standardized slope) between a given factor and B.bassiana at that sampling point while negative values represent a negative relationship. A single bar for a given factor means that sampling time did not change the relationship between B. bassiana and that factor, i.e., effects were consistent across time. The presence of bars for each sampling time indicates that the relationship between B. bassiana and a given factor depended on sampling time. Height of the bar indicates relative strength of each effect. Error bars represent 95% confidence limits. Error bars that do not cross 0 on the y-axis indicate significantly positive or negative relationships between soil factors and B. bassiana abundance at a given sample period. “*,” “**,” and “***” indicate overall significant (P < 0.05, 0.01, and 0.001, respectively) positive or negative effects of a factor on B. bassiana abundance.
Soil and Irrigation Effects on Beauveria bassiana
Soil organic matter had a consistently positive effect on B. bassiana [F(1, 662) = 9.70, P = 0.002] (Figure 1B). Soil P had no effect overall, but depended on sampling period [F(3, 733) = 3.49, P = 0.015] with a positive relationship seen in spring 2017 (Figure 1B).
Irrigation type was also related to B. bassiana abundance with dual and sprinkler irrigation increasing Beauveria compared with drip irrigation [F(2, 689) = 7.53, P < 0.001], but effects were inconsistent among sampling periods [F(6, 719) = 8.01, P < 0.001] (Figure 2).
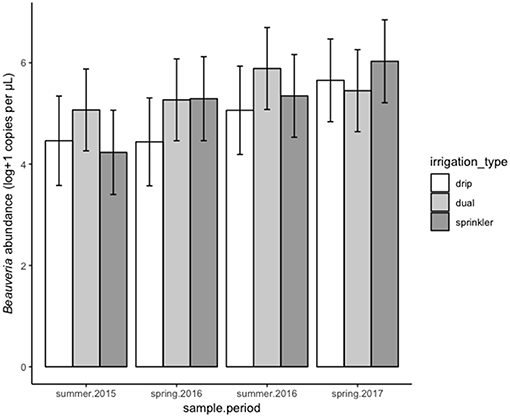
Figure 2. Effect of irrigation type (shades) on Beauveria bassiana abundance across all sites at four sampling periods. Error bars indicate 95% confidence limits based on likelihood of these estimates within the final model, i.e., accounting for all other factors included in the model.
Ilyonectria spp.
The final model for Ilyonectria spp. included sample period, irrigation type, organic matter, soil P, total plant cover, exotic plant cover, and grass cover, as well as interaction terms with sample period for all factors except grass cover and soil P. Fixed effects in this model explained 38% of the variation, with fixed plus random effects explaining 45%. Ilyonectria spp. abundance varied with sampling period [F(3, 568) = 12.21, P < 0.001], with a lower amount of Ilyonectria spp. detected in spring 2016 compared to the other sampling periods (Table 3).
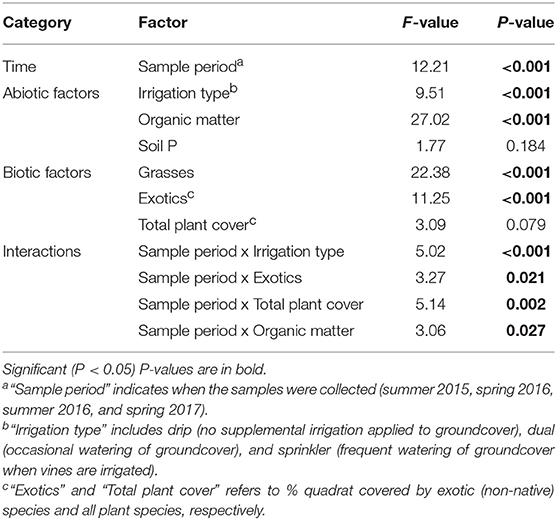
Table 3. Effects of sampling date and biotic and abiotic factors in the model of Ilyonectria spp. abundance as tested using Satterwaite type III approximation for degrees of freedom (Model AIC: 2,168, R2fixed = 0.38).
Plant Effects on Ilyonectria spp.
The abundance of Ilyonectria spp. was consistently negatively associated with grass cover [F(1, 531) = 22.38, P < 0.001] (Figure 3A). Overall, Ilyonectria spp. increased with exotic plant cover [F(1, 675) = 11.25, P < 0.001], but this relationship varied with sampling period (Figure 3A).
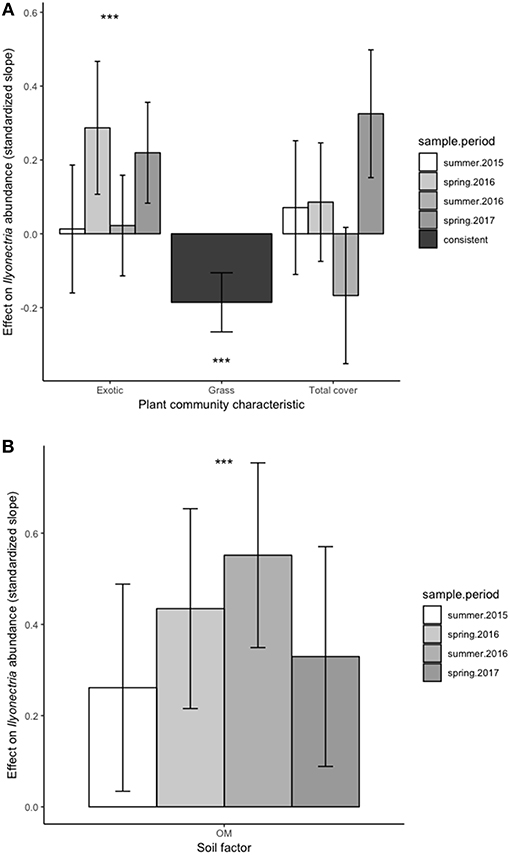
Figure 3. Effect of plant community characteristics (A) and soil properties (B) on Ilyonectria spp. abundance. Positive values indicate a positive relationship (slope) between a given factor and Ilyonectria spp. abundance at that sampling point while negative values represent a negative relationship. A single bar for a given factor means that sampling time did not change the relationship between Ilyonectria spp. and that factor, i.e., effects were consistent across time. The presence of bars for each sampling time indicates that the relationship between Ilyonectria spp. and a given factor depended on sampling time. Height of the bar indicates relative strength of each effect. Error bars represent 95% confidence limits. Error bars that do not cross 0 on the y-axis indicate significantly positive or negative relationships between soil factors and Ilyonectria spp. at a given sample period. ““***” indicate overall significant (0.001, respectively) positive or negative effects of a factor on Ilyonectria spp. abundance.
Soil and Irrigation Effects on Ilyonectria spp.
Soil organic matter was positively related [F(1, 47) = 27.02, P < 0.001] at all sampling periods despite a weak interaction with sampling period [F(3, 751) = 3.06, P = 0.03] (Figure 3B).
Irrigation type also affected Ilyonectria spp. abundance [F(2, 115) = 9.51, P < 0.001], with both dual and sprinkler irrigation leading to greater abundance overall compared to drip irrigation, though the strength of this effect depended on sampling period (Figure 4).
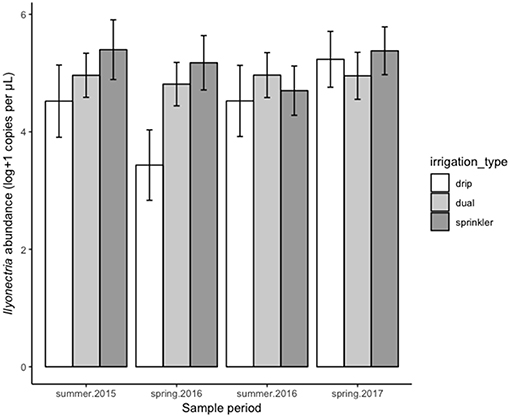
Figure 4. Effect of irrigation type (shades) on Ilyonectria spp. abundance across all sites at four sampling periods. Error bars indicate 95% confidence limits based on likelihood of these estimates within the final model, i.e., accounting for all other factors included in the model.
Discussion
Groundcover vegetation was related to the abundance of both soil fungal guilds studied. As expected, native plants were associated with greater amounts of EP fungi, suggesting that coadaptation may promote positive plant soil feedback through improved herbivore control and plant protection. The positive effect of legumes on EP fungi also matched our predictions, though there were no consistent effects of legumes on Ilyonectria spp. Instead, Ilyonectria spp. were mostly deterred by the presence of grasses and native species, further suggesting that native species could play a role in promoting positive plant-soil feedbacks in these vineyards by limiting this soil borne pathogen in this semi-arid region.
Beauveria bassiana
The increase in B. bassiana with locally adapted plant communities as seen in this study may have a basis in co-adaptation, as B. bassiana is now known to have diverged from fungi with purely endophytic lifestyles (Moonjely et al., 2016). This is, to our knowledge, the first report of a preferential association between EP fungi and native plant communities within an agricultural field. The inclusion of native species may encourage positive plant-soil feedback for crop plants such as grapevines if the larger EP fungal populations help control soil-dwelling herbivores such as climbing cutworm, mealybug, or phylloxera. To date there are no studies that specifically include EP fungi as an important player in plant-soil feedbacks, but regulation of herbivory as well as pathogen protection offered by these fungi (Ownley et al., 2010) warrant further investigation, e.g., in the context of conservation biological control (Pell et al., 2010).
The positive effect of annual species on B. bassiana could be because this EP fungus can persist in annually cropped fields, unlike some other EP fungi that prefer undisturbed habitats (Meyling and Eilenberg, 2006; Meyling et al., 2009; Medo and Cagan, 2011). Randhawa et al. (2018) recently showed that another common EP fungus, Metarhizium robertsii, occurs in higher numbers soon after disturbance and then declines with time since disturbance. Perhaps common EP fungi such as B. bassiana and Metarhizium spp. are most dominant in annual agricultural fields due to some unknown adaptation to physical disturbance, annual and weedy plant species, or the insect communities that occur in these disturbed habitats (e.g., ground-dwelling decomposers).
The increase in B. bassiana with legumes as seen here is consistent with a previous finding that B. bassiana persists well in legume cover crops in orchards (Shapiro-Ilan et al., 2012), although that study only compared legumes to the absence of a cover crop. Because legumes had a positive influence on B. bassiana in our large dataset looking at many different plant traits, this hints at some functional attribute of legumes that is especially beneficial for the success of B. bassiana. Most EP fungi including B. bassiana are poor competitors as saprotrophs (Meyling and Eilenberg, 2007), ruling out the effect of high litter quality of legumes. Most likely the benefits of a legume for EP fungi lie either in the attractiveness of the legume roots to soil herbivores (Schallhart et al., 2012), their suitability for endophytic colonization by EP fungi (Behie et al., 2015), protection from environmental stresses (Shapiro-Ilan et al., 2012) or some combination of these. The higher litter N quality of legumes may also attract more soil-dwelling insects (House and Alzugaray, 1989), thus indirectly increasing EP fungi.
Organic matter was positively associated with B. bassiana abundance at most sampling periods. A positive correlation between organic matter and the organisms that contribute to its formation can be expected (Kallenbach et al., 2016). There are several studies that show greater EP fungal isolation associated with higher organic matter soils (Ali-Shtayeh et al., 2003; Medo and Cagan, 2011) and organic fertilization (Clifton et al., 2015). Because Beauveria are generally poor competitors as saprotrophs, it is unlikely that there is a direct effect of organic matter on their populations. Instead, higher organic matter is likely associated with greater biological activity in general, including more plant roots and insects, both of which are hosts for these fungi.
Ilyonectria spp.
The decrease in Ilyonectria spp. with grass cover is consistent with the small amount of peripheral work on the effect of non-crop vegetation on these pathogens. For example, although a survey of vineyard weeds by Agustí-Brisach et al. (2011) found Ilyonectria spp. in many common weeds, only six species of grass were included in that study, of which only two hosted Ilyonectria spp. In studies of replant disease of apple, Mazzola et al. (2004) were able to reduce damage caused by pathogens such as Ilyonectria spp. through stimulation of an antagonistic rhizobacteria population using a wheat cover crop. Other grasses, such as Lolium perenne, have also been implicated in promoting bacteria with fungistatic genes (Latz et al., 2015), suggesting that perhaps some grasses deter these generalist pathogens by culturing an antagonistic rhizosphere community and thus are not good hosts. Although these potential mechanisms are speculative, the consistency of our results suggest that grasses are somehow poorer hosts for Ilyonectria spp. in these vineyards than broadleaves and might then promote positive plant-soil feedback by deterring generalist pathogens.
Exotic plants tended to increase Ilyonectria spp. abundance overall, but this was due to the strong effects seen only during spring sampling periods. It could be that this pathogen thrives in cultivated plants and associated weedy species, which are mostly exotic species in the studied region. Work on invasive plant species has shown that generalist pathogens can build up on exotic species with negligible effects on those plants (Mangla and Callaway, 2008). If Ilyonectria spp. accumulates on exotic plants, this may lead to negative feedback on vines sharing the same soil through a spillover effect. A possible explanation for the springtime effects of exotic plants on Ilyonectria spp. abundance could be that many of the exotic weedy species may be more active in the spring.
As with B. bassiana, Ilyonectria spp. was positively related to soil organic matter, perhaps relating to the general microbial contribution to stable organic matter (Kallenbach et al., 2016). This increase in Ilyonectria spp. with greater amounts of organic matter is not necessarily an indication of increased disease pressure for crop plants occupying this soil because increased microbial competition and antagonism also occurs with the use of organic amendments(Bonanomi et al., 2007; Watson et al., 2017).
The increase in Ilyonectria spp. with supplemental irrigation could be expected given that Ilyonectria spp.-related diseases such as black foot disease of grape tend to be more problematic with prolonged periods of excessive soil moisture (Halleen et al., 2006). It is also likely that the use of sprinkler irrigation leads to broader distribution of grapevine roots throughout the vineyard floor. As grapevines are good hosts for these fungi, the proximity of vine roots to the drive row sample plots could have also contributed to this effect in addition to the increased frequency of wetting.
Conclusion
This study is the first to investigate the relationships between groundcover plant community characteristics as well as soil and irrigation factors on the abundance of the entomopathogenic B. bassiana, and the plant pathogenic Ilyonectria spp. We conclude that native species may play an important role in managing plant-soil feedbacks in perennial agroecosystems as they promoted the plant-beneficial fungi B. bassiana but deterred the plant-pathogenic Ilyonectria spp. Practical application of this work will require further studies linking these plant-induced changes to soil fungi with measurable plant-soil feedbacks along with continued field trials to find locally adapted species best suited for use in perennial agricultural groundcovers.
Author Contributions
EV performed all data collection, laboratory analysis, and manuscript preparation. DL maintained experimental field plots, identified potential sample sites, and gave feedback on the manuscript. JB performed statistical analysis. MH provided laboratory resources, extra student help, and feedback on the manuscript.
Funding
EV was supported by grants from the British Columbia Winegrape Council and Agriculture and Agri-food Canada's Growing Forward 2 program. MH was supported by a grant from Agriculture and Agri-food Canada's Organic Science Cluster.
Conflict of Interest Statement
The authors declare that the research was conducted in the absence of any commercial or financial relationships that could be construed as a potential conflict of interest.
Acknowledgments
The authors wish to thank those who helped with data collection during this project: summer co-op students at AAFC-Summerland and lab research assistants Michelle MacDonald and Hayley Bessette at UBC-Okanagan. Dr. Pat Bown and Dr. José Ramon Úrbez Torres at AAFC-Summerland generously provided consultation and equipment during this project. We also thank local vineyard managers for their cooperation and consultation during this project.
Supplementary Material
The Supplementary Material for this article can be found online at: https://www.frontiersin.org/articles/10.3389/fevo.2019.00118/full#supplementary-material
References
Agustí-Brisach, C., Gramaje, D., Leon, M., Garcia-Jimenez, J., and Armengol, J. (2011). Evaluation of vineyard weeds as potential hosts of black-foot and petri disease pathogens. Plant Dis. 95, 803–810. doi: 10.1094/PDIS-12-10-0888
Agusti-Brisach, C., Mostert, L., and Armengol, J. (2014). Detection and quantification of Ilyonectria spp. spp. associated with black- foot disease of grapevine in nursery soils using multiplex nested PCR and quantitative PCR. Plant Pathol. 63, 316–322. doi: 10.1111/ppa.12093
Ali-Shtayeh, M. S., Mara'i, A., and Jamous, R. M. (2003). Distribution, occurrence and characterization of entomopathogenic fungi in agricultural soil in the Palestinian area. Mycopathologia 156, 235–244. doi: 10.1023/A:1023339103522
Badri, D. V., and Vivanco, J. M. (2009). Regulation and function of root exudates. Plant Cell Environ. 32, 666–681. doi: 10.1111/j.1365-3040.2009.01926.x
Bardgett, R. D., and van der Putten, W. H. (2014). Belowground biodiversity and ecosystem functioning. Nature 515, 505–511. doi: 10.1038/nature13855
Barton, K. (2017). MuMIn: Multi-Model Inference. R Package Version 1.40.0. Vienna: R Foundation for Statistical Computing.
Bates, D. M., Mächler, B., Bolker, S., and Walker (2015). Fitting linear mixed-effects models using lme4. J. Stat. Soft. 67, 1–48. doi: 10.18637/jss.v067.i01
Behie, S. W., Jones, S. J., and Bidochka, M. J. (2015). Plant tissue localization of the endophytic insect pathogenic fungi Metarhizium and Beauveria. Fungal Ecol. 13, 112–119. doi: 10.1016/j.funeco.2014.08.001
Behie, S. W., Moreira, C. C., Sementchoukova, I., Barelli, L., Zelisko, P. M., and Bidochka, M. J. (2017). Carbon translocation from a plant to an insect-pathogenic endophytic fungus. Nat. Commun. 8:14245. doi: 10.1038/ncomms14245
Behie, S. W., Zelisko, P. M., and Bidochka, M. J. (2012). Endophytic insect-parasitic fungi translocate nitrogen directly from insects to plants. Science 336, 1576–1577. doi: 10.1126/science.1222289
Benitez, M. S., Taheri, W. I., and Lehman, R. M. (2016). Selection of fungi by candidate cover crops. Appl. Soil Ecol. 103, 72–82. doi: 10.1016/j.apsoil.2016.03.016
Bonanomi, G., Antignani, V., Pane, C., and Scala, E. (2007). Suppression of soilborne fungal diseases with organic amendments. J. Plant Pathol. 89, 311–324. Available online at: https://www.jstor.org/stable/41998410
Broeckling, C. D., Broz, A. K., Bergelson, J., Manter, D. K., and Vivanco, J. M. (2008). Root exudates regulate soil fungal community composition and diversty. Appl. Environ. Microbiol. 74, 738–744. doi: 10.1128/AEM.02188-07
Bullock, D. G. (1992). Crop-rotation. Crit. Rev. Plant Sci. 11, 309–326. doi: 10.1080/07352689209382349
Burnham, K. P., and Anderson, D. R. (2002). Model Selection and Multi-Model Inference: A Practical Information-Theoretic Approach, 2nd Edn. Secaucus, NJ: Springer.
Clifton, E. H., Jaronski, S. T., Hodgson, E. W., and Gassmann, A. J. (2015). Abundance of soil-borne entomopathogenic fungi in organic and conventional fields in the Midwestern USA with an emphasis on the effect of herbicides and fungicides on fungal persistence. PLoS ONE 10:0133613. doi: 10.1371/journal.pone.0133613
Corneo, P. E., Pellegrini, A., Cappellin, L., Gessler, C., and Pertot, I. (2013). Weeds influence soil bacterial and fungal communities. Plant Soil 373, 107–123. doi: 10.1007/s11104-013-1754-5
Davies, B. E. (1974). Loss-on-ignition as an estimate of soil organic-matter. Soil Sci. Soc. Am. J. 38, 150–151. doi: 10.2136/sssaj1974.03615995003800010046x
De Bellis, T., Kernaghan, G., and Widden, P. (2007). Plant community influences on soil microfungal assemblages in boreal mixed-wood forests. Mycologia 99, 356–367. doi: 10.1080/15572536.2007.11832560
De Deyn, G. B., Quirk, H., and Bardgett, R. D. (2011). Plant species richness, identity and productivity differentially influence key groups of microbes in grassland soils of contrasting fertility. Biol. Lett. 7, 75–78. doi: 10.1098/rsbl.2010.0575
Detheridge, A. P., Brand, G., Fychan, R., Crotty, F. V., Sanderson, R., Griffith, G. W., et al. (2016). The legacy effect of cover crops on soil fungal populations in a cereal rotation. Agric. Ecosyst. Environ. 228, 49–61. doi: 10.1016/j.agee.2016.04.022
Dubrovsky, S., and Fabritius, A. L. (2007). Occurrence of Ilyonectria spp.Indrocarpon spp. in nursery grapevines in California. Phytopathol. Mediterr. 46, 84–86. doi: 10.14601/Phytopathol_Mediterr-1859
Fanin, N., Haettenschwiler, S., and Fromin, N. (2014). Litter fingerprint on microbial biomass, activity, and community structure in the underlying soil. Plant Soil 379, 79–91. doi: 10.1007/s11104-014-2051-7
Halleen, F., Fourie, P. H., and Crous, P. W. (2006). A review of black foot disease of grapevine. Phytopathol. Mediterr. 45, S55–S67. doi: 10.14601/Phytopathol_Mediterr-1845
House, G. J., and Alzugaray, M. D. (1989). Influence of cover cropping and no-tillage practices on community composition of soil arthropods in a North-Carolina agroecosystem. Environ. Entomol. 18:302–307. doi: 10.1093/ee/18.2.302
Hu, G., and St. Leger, J. (2002). Field studies using a recombinant mycoinsecticide (Metarhizium anisopliae) reveal that it is rhizosphere competent. Appl. Environ. Microbiol. 68, 6383–6387. doi: 10.1128/AEM.68.12.6383-6387.2002
Ingels, C. A., Scow, K. M., Whisson, D. A., and Drenovsky, R. E. (2005). Effects of cover crops on grapevines, yield, juice composition, soil microbial ecology, and gopher activity. Am. J. Enol. Viticul. 56, 19–29.
Kallenbach, C. M., Frey, S. D., and Grandy, A. S. (2016). Direct evidence for microbial-derived soil organic matter formation and its ecophysiological controls. Nat. Commun. 7:13630. doi: 10.1038/ncomms13630
Kjeldahl, J. (1883). Neue methode zur bestimmung des stickstoffs in organischen körpern (New method for the determination of nitrogen in organic substances). Z. Analyt. Chem. 22, 366–383. doi: 10.1007/BF01338151
Laliberté, E., and Legendre, P. (2010). A distance-based framework for measuring functional diversity from multiple traits. Ecology 91, 299–305. doi: 10.1890/08-2244.1
Landa, B. B., López-Díaz, C., Jiménez-Fernández, D., Montes-Borrego, M., Muñoz-Ledesma, F. J., Ortiz-Urquiza, A., et al. (2013). In-planta detection and monitorization of endophytic colonization by a Beauveria bassiana strain using a new-developed nested and quantitative PCR-based assay and confocal laser scanning microscopy. J. Invert. Pathol. 114, 128–138. doi: 10.1016/j.jip.2013.06.007
Lankau, E. W., and Lankau, R. A. (2014). Plant species capacity to drive soil fungal communities contributes to differential impacts of plant-soil legacies. Ecology 95, 3221–3228. doi: 10.1890/13-2342.1
Latz, E., Eisenhauer, N., Scheu, S., and Jousset, A. (2015). Plant identity drives the expression of biocontrol factors in a rhizosphere bacterium across a plant diversity gradient. Funct. Ecol. 29, 1225–1234. doi: 10.1111/1365-2435.12417
Lenth, R. (2018). emmeans: Estimated Marginal Means, aka Least-Squares Means. R Package Version 1.1.3. Available online at: https://CRAN.R-project.org/package=emmeans (accessed December 6, 2018).
Mangla, S., and Callaway, R. M. (2008). Exotic invasive plant accumulates native soil pathogens which inhibit native plants. J. Ecol. 96, 58–67. doi: 10.1111/j.1365-2745.2007.01312.x
Manici, L., Kelderer, M., Caputo, F., and Mazzola, M. (2015). Auxin-mediated relationships between apple plants and root inhabiting fungi: impact on root pathogens and potentialities of growth-promoting populations. Plant Pathol. 64, 843–851. doi: 10.1111/ppa.12315
Mazzola, M., Funnell, D. L., and Raaijmakers, J. M. (2004). Wheat cultivar-specific selection of 2,4-diacetylphloroglucinol-producing fluorescent Pseudomonas species from resident soil populations. Microb. Ecol. 48, 338–348. doi: 10.1007/s00248-003-1067-y
Medo, J., and Cagan, L. (2011). Factors affecting the occurrence of entomopathogenic fungi in soils of Slovakia as revealed using two methods. Biol. Control 59, 200–208. doi: 10.1016/j.biocontrol.2011.07.020
Mehlich, A. (1984). Mehlich 3 soil test extractant: a modification of the Mehlich 2 extractant. Commun Soil Sci. Plant Anal. 15:1409–1416 doi: 10.1080/00103628409367568
Meyling, N. V., and Eilenberg, J. (2006). Occurrence and distribution of soil borne entomopathogenic fungi within a single organic agroecosystem. Agric. Ecosyst. Environ. 113, 336–341. doi: 10.1016/j.agee.2005.10.011
Meyling, N. V., and Eilenberg, J. (2007). Ecology of the entomopathogenic fungi Beauveria bassiana and Metarhizium anisopliae in temperate agroecosystems: potential for conservation biological control. Biol. Control 43, 145–155. doi: 10.1016/j.biocontrol.2007.07.007
Meyling, N. V., Lübeck, M., Buckley, E. P., Eilenberg, J., and Rehner, S. A. (2009). Community composition, host range and genetic structure of the fungal entomopathogen Beauveria in adjoining agricultural and seminatural habitats. Mol. Ecol. 18, 1282–1293. doi: 10.1111/j.1365-294X.2009.04095.x
Moonjely, S., Barelli, L., and Bidochka, M. J. (2016). Insect pathogenic fungi as endophytes. Genet. Mol. Biol. Entomopathog. Fungi 94, 107–135. doi: 10.1016/bs.adgen.2015.12.004
Nakagawa, S., and Schielzeth, H. (2013). A general and simple method for obtaining R2 from generalized linear mixed-effects models. Methods Ecol. Evol. 4, 133–142. doi: 10.1111/j.2041-210x.2012.00261.x
Ownley, B., Gwinn, K., and Vega, F. (2010). Endophytic fungal entomopathogens with activity against plant pathogens: ecology and evolution. Biocontrol 55, 113–128. doi: 10.1007/s10526-009-9241-x
Pell, J. K., Hannam, J. J., and Steinkraus, D. C. (2010). Conservation biological control using fungal entomopathogens. Biocontrol 55, 187–198. doi: 10.1007/s10526-009-9245-6
Randhawa, P. K., Mullen, C., and Barbercheck, M. (2018). Plant identity, but not diversity, and agroecosystem characteristics affect the occurrence of M. robertsii in an organic cropping system. Biol. Control 124, 18–29. doi: 10.1016/j.biocontrol.2018.06.001
Schallhart, N., Tusch, M. J., Wallinger, C., Staudacher, K., and Traugott, M. (2012). Effects of plant identity and diversity on the dietary choice of a soil-living insect herbivore. Ecology 93, 2650–2657. doi: 10.1890/11-2067.1
Shah, P. A., and Pell, J. K. (2003). Entomopathogenic fungi as biological control agents. Appl. Microbiol. Biotechnol. 61, 413–423. doi: 10.1007/s00253-003-1240-8
Shapiro-Ilan, D. I., Gardner, W. A., Wells, L., and Wood, B. W. (2012). Cumulative impact of a clover cover crop on the persistence and efficacy of Beauveria bassiana in suppressing the pecan Weevil (Coleoptera1: Curculionidae). Environ. Entomol. 41, 298–307. doi: 10.1603/EN11229
Steenwerth, K., and Belina, K. M. (2008). Cover crops and cultivation: impacts on soil N dynamics and microbiological function in a Mediterranean vineyard agroecosystem. Appl. Soil Ecol. 40, 370–380. doi: 10.1016/j.apsoil.2008.06.004
Tewoldemedhin, Y. T., Mazzola, M., Mostert, L., and McLeod, A. (2011). Ilyonectria spp.indrocarpon species associated with apple tree roots in South Africa and their quantification using real-time PCR. Eur. J. Plant Pathol. 129, 637–651. doi: 10.1007/s10658-010-9728-4
Trypsteen, W., Vynck, M., De Neve, J., Bonczkowski, P., Kiselinova, M., Malatinkova, E., et al. (2015). ddpcRquant: threshold determination for single channel droplet digital PCR experiments. Analyt. Bioanalyt. Chem. 407, 5827–5834. doi: 10.1007/s00216-015-8773-4
Úrbez-Torres, J. R., Haag, P., Bowen, P., Lowery, T., and O'Gorman, D. T. (2015). Development of a DNA macroarray for the detection and identification of fungal pathogens causing decline of young grapevines. Phytopathology 105:1373–1388. doi: 10.1094/PHYTO-03-15-0069-R
Vukicevich, E., Lowery, D. T., Urbez-Torres, J. R., Bowen, P., and Hart, M. (2018). Groundcover management changes grapevine root fungal communities and plant-soil feedback. Plant Soil 424, 419–433. doi: 10.1007/s11104-017-3532-2
Vukicevich, E., Lowery, T., Bowen, P., Urbez-Torres, J. R., and Hart, M. (2016). Cover crops to increase soil microbial diversity and mitigate decline in perennial agriculture. A review. Agron. Sustain. Dev. 36:48. doi: 10.1007/s13593-016-0385-7
Watson, T. T., Nelson, L. M., Neilsen, D., Neilsen, G. H., and Forge, T. A. (2017). Soil amendments influence Pratylenchus penetrans populations, beneficial rhizosphere microorganisms, and growth of newly planted sweet cherry. Appl. Soil Ecol. 117, 212–220. doi: 10.1016/j.apsoil.2017.04.014
Whitelaw-Weckert, M. A., Rahman, L., Hutton, R. J., and Coombes, N. (2007). Permanent swards increase soil microbial counts in two Australian vineyards. Appl. Soil Ecol. 36, 224–232. doi: 10.1016/j.apsoil.2007.03.003
Keywords: cover crops, entomopathogenic fungi, black foot disease, conservation biocontrol, vineyards
Citation: Vukicevich E, Lowery DT, Bennett JA and Hart M (2019) Influence of Groundcover Vegetation, Soil Physicochemical Properties, and Irrigation Practices on Soil Fungi in Semi-arid Vineyards. Front. Ecol. Evol. 7:118. doi: 10.3389/fevo.2019.00118
Received: 19 November 2018; Accepted: 22 March 2019;
Published: 10 April 2019.
Edited by:
Martijn Bezemer, Netherlands Institute of Ecology (NIOO-KNAW), NetherlandsReviewed by:
Annette Reineke, Hochschule Geisenheim University, GermanyChristina Hazard, Ecole Centrale of Lyon, France
Copyright © 2019 Vukicevich, Lowery, Bennett and Hart. This is an open-access article distributed under the terms of the Creative Commons Attribution License (CC BY). The use, distribution or reproduction in other forums is permitted, provided the original author(s) and the copyright owner(s) are credited and that the original publication in this journal is cited, in accordance with accepted academic practice. No use, distribution or reproduction is permitted which does not comply with these terms.
*Correspondence: Eric Vukicevich, ZXJpY3Z1a2VAZ21haWwuY29t