- 1Instituto de Investigación en Recursos Cinegéticos (IREC), CSIC-UCLM-JCCM, Ciudad Real, Spain
- 2Estación Biológica de Doñana-CSIC, Sevilla, Spain
- 3Departamento de Zoología, Facultad de Ciencias, Universidad de Granada, Granada, Spain
Telomere length is a marker of cellular senescence that relates to different components of individual fitness. Oxidative stress is often claimed as a main proximate factor contributing to telomere attrition, although the importance of this factor in vivo has recently been challenged. Early development represents an ideal scenario to address this hypothesis because it is characterized by the highest rates of telomere attrition of the life and by an arguably high susceptibility to oxidative stress. We tested the effect of oxidative stress on telomere dynamics during early development by exposing pied flycatcher nestlings (Ficedula hypoleuca) to either an oxidative challenge (diquat injections), an antioxidant (vitamin E) or control treatments (PBS injections and supplementation with vehicle substance). We found no effects of treatments on average telomere change during the nestling period. However, vitamin E supplementation, which increased growth, removed the association between initial telomere length and telomere attrition. Diquat-treated nestlings, by contrast, showed no differences in growth or telomere dynamics with respect to controls. These results do not support the hypothesis that oxidative stress is the main direct mechanism explaining telomere attrition in vivo, and highlight the importance of micronutrient intake during early development on telomere dynamics. Studies addressing alternative action pathways of vitamins on growth and telomere dynamics, perhaps via restoration mechanisms, would provide important insights on the proximate factors affecting telomere attrition during this critical phase of life.
Introduction
Telomeres are repetitive non-coding DNA sequences that protect the ends of eukaryotic chromosomes during cell replication (Blackburn, 1991). Telomeres typically shorten with age, and telomere length and attrition rate often relate to key components of individual fitness, like survival or lifespan in different taxa (e.g., Bize et al., 2009; Heidinger et al., 2012; Boonekamp et al., 2014; Eastwood et al., 2018). In addition to age effects, telomere length is particularly sensitive to different internal and external stressors that may accelerate telomere attrition and hence cellular aging (Boonekamp et al., 2014; Nettle et al., 2015). Telomere shortening rates are particularly high during early phases of development (e.g., Salomons et al., 2009; Heidinger et al., 2012), precisely in a period of life when exposure to harsh conditions can have long-lasting consequences on adult phenotype and fitness (Lindstrom, 1999). For this reason, telomere dynamics during this critical phase of life constitutes a key link between development, capacity to cope with stressful conditions, and lifetime fitness.
Oxidative stress is often claimed as a main proximate factor contributing to telomere attrition (von Zglinicki, 2002). Oxidative stress results from the imbalance between the production rate of pro-oxidant molecules (collectively termed “reactive oxygen species”) and the antioxidant defense system (Halliwell and Gutteridge, 2007). Most reactive oxygen species in the organism are by-products generated in the mitochondria during aerobic metabolism, although immune responses or exposure to pollutants can also contribute to increase their levels (Halliwell and Gutteridge, 2007). Telomere sequences are rich in guanine, a base that is particularly sensitive to oxidative modifications that ultimately result in double and single-stranded breaks to DNA (Kawanishi and Oikawa, 2004). Such DNA damage impairs replication during cell division, leading to enhanced telomere attrition when oxidative stress levels increase.
Most in vitro studies have provided empirical support for the hypothesis that oxidative stress accelerates telomere shortening (von Zglinicki, 2002). However, whether this causal link can be extrapolated to a whole living organism is controversial (Boonekamp et al., 2017a; Reichert and Stier, 2017; Monaghan and Ozanne, 2018). The relevance of oxidative stress as a main determinant of telomere dynamics in vivo has been recently challenged (Boonekamp et al., 2017a), and a recent review showed that very few studies have addressed this hypothesis experimentally, particularly in the wild (Reichert and Stier, 2017).
Early development represents an ideal scenario to test adaptive trade-offs mediated by oxidative stress. Developing individuals are probably very vulnerable to oxidative stress because they experience a period of fast growth associated to increased metabolism and high production of reactive oxygen species (Metcalfe and Alonso-Alvarez, 2010). This is particularly exacerbated in nestlings of altricial bird species, which experience the highest growth rates amongst vertebrates. Indeed, a recent meta-analysis has shown that oxidative stress can act both as a constraint on and as a cost of growth, providing evidence for a growth–self-maintenance trade-off during development (Smith et al., 2016). This trade-off is concomitant with the highest rates of telomere attrition of the life (Salomons et al., 2009; Heidinger et al., 2012), making this period particularly suitable to assess the causal link between telomere dynamics and oxidative stress.
In this study we manipulated the oxidative status of developing nestlings of an altricial passerine, the pied flycatcher (Ficedula hypoleuca), and assessed the effects on growth and telomere dynamics. In order to alter the oxidative status of individuals, nestlings of the same brood were exposed to two different compounds: a free radical generator (diquat) or an antioxidant supplement (vitamin E). Diquat (1,1′-ethylene-2,2′-bipyridylium) is commercially used as an herbicide whose action mechanism relies on its capacity to generate free radicals. When entering the organism, diquat molecules undergo a chemical reduction, forming radical cations that react with molecular oxygen, triggering a chain reaction that generates superoxide anion, H2O2 and, in the presence of transition metals, highly toxic hydroxyl radicals (Koch et al., 2017). At low concentrations, diquat and similar bipyridines have been repeatedly used to induce oxidative challenges in birds (e.g., Isaksson and Andersson, 2008; Alonso-Alvarez and Galván, 2011; García-de Blas et al., 2016). Vitamin E is considered one of the most important antioxidants in cell membranes, where it prevents oxidative damage by scavenging reactive oxygen species—alone or in concert with other antioxidant molecules (Surai, 2002). Vitamin E can only be obtained from diet, which may limit the capacity of the organism to satisfy its requirements under highly demanding periods like early development.
We predicted that diquat treatment would increase oxidative stress, which would reduce nestling growth and enhance telomere attrition relative to control nestlings of the same brood. By contrast, vitamin E supplementation would reduce oxidative stress, allowing an enhancement of growth and a reduction in telomere attrition.
Materials and Methods
Experimental Design
The study was carried out in 2015 in a pied flycatcher population breeding in nest-boxes in an old oak (Quercus pyrenaica) forest near La Hiruela (central Spain; 41°04N 3°27W). Nestboxes were inspected regularly to determine the exact hatching date. The experiment was performed with 216 chicks from 41 nests (sample size was gradually reduced during the experiment due to nest failure or predation; final sample sizes for each response variable are reported in Table S1). When nestlings were 5 days old, they were individually marked with non-toxic pens to allow individual recognition and we recorded their mass (±0.01 g). At this time, nestlings were ranked according to their mass and assigned to one of the three different treatments, changing the order between successive nests to assure an unbiased sample. Treatments consisted of vitamin E supplementation (V), oxidative challenge via diquat injection (D), or control (C). V nestlings were fed a dipteran larva (Calliphora sp.) impregnated in a solution of vitamin E (DL-alpha-tocopherol acetate; Sigma-Aldrich ref. T3376) in organic coconut oil, which was selected as solvent due to its low vit. E content (Herting and Drury, 1963). The concentration of this vit. E solution was adjusted across nestling development in order to supplement nestlings throughout the experiment with a constant dose of 1.2 mg of vit. E per kg. This dosage was equivalent to 1 s.d. of the mean estimated vitamin E daily intake of barn swallow (Hirundo rustica) nestlings (Ayala et al., 2006). We chose this value as a reference because there are no available data of the vitamin E content of the diet of pied flycatcher nestlings. However, it should be noted that caterpillars –one of the main components of flycatcher nestlings' diet (Cholewa and Wesolowski, 2011)—contain about 10- to 30-fold more vitamin E than aerial insects fed by barn swallow parents to their offspring (Ayala et al., 2006; Arnold et al., 2010); see Matrková and Remeš (2014) for a detailed discussion of this issue. Therefore, the vitamin E dosage used in this study can be considered low-moderate, which makes our results conservative.
Apart from the vitamin E supplement, V nestlings received an intraperitoneal injection of 2 μl of sterile PBS per gram of body mass. D nestlings were intraperitoneally injected with the same volume of a solution of diquat (Syngenta, Madrid) in sterile PBS (0.6 mg/ml), corresponding to a dose of 1.2 mg/kg. We selected this dosage based on a pilot study that allowed us to identify a diquat concentration that imposed a significant oxidative challenge without falling into pharmacological levels (see Supplementary Material). Also, D nestlings were fed a larva impregnated in coconut oil. Finally, C nestlings received an intraperitoneal injection of sterile PBS and a larva impregnated in coconut oil. We repeated these experimental manipulations on days 7, 10, and 12 post-hatching, recording nestling mass at every visit. On day 13, we also recorded body mass and tarsus length (±0.01 mm) of all chicks.
Telomeres
To test the effect of our treatments on telomere dynamics, we selected a random subsample of 32 nests (126 nestlings) of those exposed to our experimental manipulation and collected ca. 40 μl of blood using heparinized capillaries on days 5 and 13 after hatching. Samples were stored at −80°C until analysis. Samples were extracted using a standard ammonium acetate protocol and telomere length was analyzed by q-PCR following Criscuolo et al. (2009) and expressed as the quantity of telomere sequences in the q-PCR reaction of the sample relative to that of a single copy of the gene that encodes for glyceraldehyde-3-phosphate dehydrogenase (GAPDH), i.e., T/S ratio. The final PCR volume was 20 μl containing 10 μl of Light Cycler 480 SYBR Green I Master (Roche, Diagnostics GmbH, Mannheim, Germany) and 20 ng/μl DNA. PCR conditions for telomere analyses were 10 min at 95°C followed by 30 cycles of 1 min at 56°C and finally 1 min at 95°C. PCR conditions for GAPDH were 10 min at 95°C followed by 40 cycles of 1 min at 60°C and finally 1 min at 95°C. Further details of the protocol have been detailed elsewhere (Badas et al., 2015). Samples were assayed in duplicate, and nestlings from the same brood were always included in the same plate. A five-point calibration curve was included in triplicate in every assay plate. Repeatability for T/S ratio measurements, assayed in a set of 10 samples extracted and analyzed twice, was high (r = 0.77, p < 0.001). For the analyses, we considered the measure of telomere lengths (T/S) at day 5 and 13 –transformed to a standard normal distribution; see Statistics section-, and the change T/S ratios in that period after correcting for regression to the mean according to Verhulst et al. (2013). In this way, a more negative value indicates greater telomere loss. Results are qualitatively the same if absolute changes are considered instead.
Oxidative Stress
Due to a problem with sample storage conditions, the impact of our treatments on oxidative stress biomarkers could not be assessed in the samples collected in 2015. For this reason, in 2016 we confirmed the effectiveness of our treatments in altering nestlings' oxidative status by applying the same experimental setup described above to a set of 46 nestlings belonging to 10 nests of the same study area. These nests were the same (n = 3) or adjacent to those used for the 2015 experiment. Fledging success, average tarsus lengths and body masses per nest did not differ between 2015 and 2016 in our study site (all p > 0.4). This suggests that breeding conditions were similar in both years, making the results reasonably comparable. After using the experimental protocol described before, at day 13, a 40 μl blood sample was collected from the jugular vein of every nestling using a heparinised syringe. As in the pilot study, samples were transported immediately to the laboratory in cooled containers and centrifuged at 5,000 g. Plasma was separated from the cellular fraction and both fractions were stored at −80°C until analysis.
Oxidative stress is a complex phenomenon that can only be described by targeting different components of the system with several biomarkers (Halliwell and Gutteridge, 2007; Monaghan et al., 2009; Pérez-Rodríguez, 2009). Thus, to assess the oxidative status of individuals, we analyzed one marker of oxidative damage in lipids (malondialdehyde, MDA) and one marker of non-enzymatic antioxidant capacity (TEAC) in plasma, and the activities of four enzymes involved in antioxidant defense in red blood cells: catalase, superoxide dismutase, glutathione reductase, and glutathione peroxidase. According to our predictions, we would expect an increase in MDA levels in D nestlings as compared to controls. Conversely, if vitamin E availability limits nestling capacity to maintain redox status, we would expect lower MDA levels in V relative to C nestlings. However, given the interconnection among different components of the antioxidant system and the dynamic nature of antioxidant responses, it is difficult to make unique and accurate predictions on the responses of markers of antioxidant levels (Costantini and Verhulst, 2009; Meitern et al., 2013).
MDA is the most widely used marker of lipid peroxidation in biological and medical sciences (Halliwell and Gutteridge, 2007; Mateos and Bravo, 2007). For the quantification of MDA in plasma we used a well-established method based on the reaction of MDA with thiobarbituric acid (TBA) at high temperature followed by a detection of MDA-TBA adducts by fluorescence via high performance liquid chromatography; a detailed protocol has been described in Pérez-Rodríguez et al. (2015). Results are reported as mM MDA per liter of plasma. TEAC quantifies the capacity of a biological sample—plasma, in this case- to inhibit the oxidation of a chromogen (2,2′-azino-bis-[3-ethylbenzothiazoline-6-sulphonic acid], ABTS) induced by the action a hydrogen peroxide, using TROLOX (a water-soluble vitamin-E derivative) as a standard. We assessed TEAC levels in plasma following Cohen et al. (2007). Concentrations of all reactives and details of the analytical procedure can be found in López-Arrabé et al. (2015). Values obtained using this technique are particularly sensitive to the concentration of uric acid in the sample (Cohen et al., 2007). For this reason, we quantified the levels of this metabolite using a commercial kit based on the uric acid oxidase/peroxidase method (Biosystems, Barcelona). For the analysis of antioxidant enzymes, red blood cells were homogenized in a buffered solution (100 mM Tris-HCL,0.1 mM EDTA, 0.1% triton X-100, 0.1 mM PMSF; pH 7.8) and analyzed following the protocols described in Burraco and Gomez-Mestre (2016), using a Victor 3 Perkin Elmer microplate reader. Finally, protein concentration in red blood cell homogenates was analyzed following the standard Bradford's method. All laboratory analyses were run blind with respect to treatment and nest.
Statistics
We used R 3.5.0 for all the analyses. Brood identity was entered in the models as a random effect using the lme4 statistical package (Bates et al., 2015). When considering repeated measures of the same individual, nestling identity was nested within brood identity. For the analysis of TEAC, uric acid concentration was included as a covariate. Given that protein levels in erythrocytes showed differences among experimental treatments (see section Results), the activity of the enzyme (in UI per ml of homogenization buffer) was used as the response variable in the analysis of antioxidant enzymes, whereas protein levels were included as a covariate. T/S ratios were transformed for day 5 and 13 data pooled to a standard normal distribution (i.e., z-scored) prior to analysis. Total protein levels in the pellet were Box Cox-transformed, whereas MDA, glutathione peroxidase levels and z-scored telomere lengths at day 5 and 13 were log-transformed when entered as response variables in the models. Due to the limited amount of blood collected, not all oxidative stress biomarkers could be assayed for all individuals, leading to small differences in sample size among models. A list of the sample sizes for each biomarker is given in Table S1. Brood size, hatching date, and nestling mass at 5 days were tested, but finally not included as covariates—except in the case of the model for final body mass—because of their low explanatory power. Given the large number of oxidative stress biomarkers used, p-values of the models for these variables were adjusted for multiple testing (Krzywinski and Altman, 2014) by using the Hochberg correction (Hochberg, 1988) as implemented in R p.adjust() function (R-Core-Team, 2017). The effect size of treatment for adjusted statistically significant biomarkers was measured as the standardized regression coefficient (non-transformed data), and its confidence interval computed by using the R ci.src() function in package MBESS (Kelley, 2018). In all cases, we verified that model residuals fulfilled the requirements of normality and homoscedasticity. We summarize in the Results section the main effects found in the analyses. Full models and final sample sizes for all response variables can be found in the Supplementary Material. The raw data supporting the conclusions of this work are available at http://dx.doi.org/10.20350/digitalCSIC/8627.
Results
Oxidative Stress
We found clear effects on lipid peroxidation levels, as revealed by MDA analysis [F(2, 32.7) = 7.9, p-adjusted < 0.01]. D nestlings tended to show higher MDA levels than C nestlings [t(33.1) = 2.68, p-adjusted = 0.06]. Indeed, D nestlings showed average MDA levels 25% higher than C nestlings (mean±s.e. in mmol/l: 9.89 ± 2.79 vs. 7.88 ± 2.27, respectively), which represents an effect size of 5.9 (1.58–10.21, 95% CI) standard deviations above control values. The difference between C and V nestlings was however not statistically clear [t(32.9) = 0.74, p-adjusted = 0.80], as V nestlings showed average MDA levels only 9% lower than C nestlings (7.12 ± 1.37 mmol/l), which represents an effect size of 1.6 (−5.22 to 3.23, 95% CI) standard deviations below control values. We also found an effect on total protein levels in erythrocytes [F(2, 43) = 6.1, p < 0.01]: V nestlings showed lower protein levels than C [t(43) = 3.44, p = 0.01], whereas no difference was detected between D and C nestlings [t(43) = 1.52, p = 0.13]. We found no difference among treatments for catalase, superoxide dismutase, glutathione reductase, glutathione peroxidase or TEAC levels (all p-adjusted > 0.70).
Growth and Telomeres
We found no differences in body mass or telomere length among experimental groups at the beginning of the experiment in 2015 [F(2, 185.5) = 0.003, p = 0.99 and F(2, 99.1) = 0.06, p = 0.94]. Our experimental manipulations affected body mass changes during the nestling period (age × treatment: F(8, 820.1) = 2.08, p = 0.03]: on average, V nestlings ended up with the highest body mass and D nestlings with the lowest, C nestlings being intermediate (Figure 1). As a result there were differences in mass at the end of development—after controlling for initial body mass- among treatments [F(2, 158.6) = 5.73, p < 0.01]: V nestlings were heavier than C [t(157.8) = 2.12, p = 0.03], whereas no difference was detected between C and D nestlings [t(158.7) = 1.25, p = 0.21]. Despite these effects on body mass, our treatments did not affect structural size, as revealed by the lack of differences in tarsus length among groups [F(2, 158.6) = 0.46, p = 0.63].
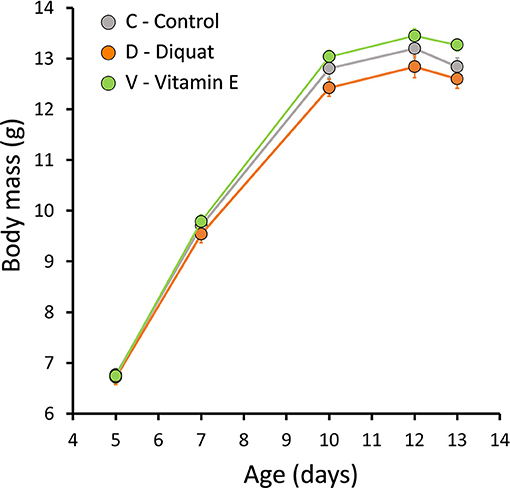
Figure 1. Effect of the experimental treatments (diquat injections, vitamin E supplementation or control) on body mass (mean ± s.e.).
Overall, average telomere length decreased from day 5 to 13 of life [z-scored means ± s.e.: d5 = 0.40 ± 0.089; d13 = −0.40 ± 0.073; F(1, 125) = 152.4, p < 0.001], although both measurements were positively correlated (Figure 2A). Within individual repeatability relative telomere length from day 5 to 13 was 0.40 [F(1, 125) = 2.32, p < 0.001]. Experimental treatments did not result in differences among groups in telomere lengths at day 13 [F(2, 102.3) = 0.500, p = 0.61] (Figure 2B). Similarly changes in telomere length during the experiment (after correction for regression to the mean Verhulst et al., 2013 did not differ among experimental groups either [F(2, 105.4) = 0.124, p = 0.88]. Nevertheless, when the effect of telomere length at day 5 was considered, as well as its interaction with treatment, an interesting pattern emerged: changes in telomere length during the experiment were related to telomere length at day 5, with birds with longer initial telomeres showing greater attrition [estimate±s.e. = −0.29 ± 0.27; t(121.6) = −2.34, p = 0.02]. However, this relationship was affected by our experimental treatment [telomere at day 5 × treatment: F(2, 118.5) = 4.58, p = 0.01] (Figure 2C). This negative association between telomere change and initial telomere length did not differ between C and D nestlings [t(118.1) = 0.06, p = 0.96] and was statistically relevant [estimate±s.e. = −0.10 ± 0.03, t(67.1) = −3.47, p < 0.001 for both groups combined]. By contrast, this relationship disappeared among V nestlings [estimate±s.e. = 0.02 ± 0.03; t(39.5) = 0.55, p = 0.58], which statistically differed from C nestlings [t(118.3) = 2.62, p = 0.01] (Figure 2C).
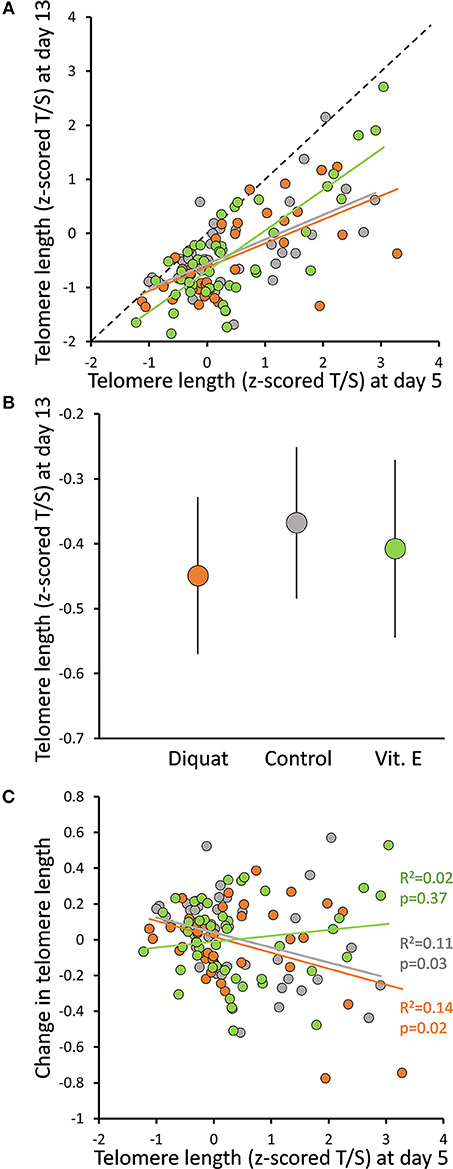
Figure 2. Effect of experimental treatments (diquat injections, vitamin E supplementation or control) on (A) the relationship between telomere length at day 5 and 13, (B) telomere length at day 13 (mean ± s.e.), and (C) the relationship between change in telomere length from day 5 to 13 of age (after correcting for regression to the mean; Verhulst et al., 2013) and telomere length at day 5. When telomere lengths are shown, we report T/S ratios transformed for day 5 and 13 data pooled to a standard normal distribution (i.e., z-scored T/S). The dashed line in (A) describes equal values of x and y, thus the vertical distance indicates the amount of telomere shortening. R2 and p-values in (C) correspond to simple correlations within groups; see main text for estimates derived from full statistical models. Color codes for treatments are: orange for diquat, gray for control and green for vitamin E-treated nestlings.
Discussion
In this study we manipulated the antioxidant (i.e., vitamin E) intake and the exposure to reactive oxygen species (via diquat injections) during post-hatching development in pied flycatcher nestlings. We found a moderate effect of our treatments on nestling growth, characterized by an increase in body mass gain in V relative to C nestlings. Regarding cellular aging, our initial hypothesis that oxidative stress would enhance telomere attrition during early development was not supported: neither D nor V nestlings differed from C chicks in telomere change from hatching to fledging. However, even though our measures of telomere attrition were controlled for regression to the mean (Verhulst et al., 2013), we found that telomere attrition was more intense in hatchlings with relatively longer telomeres, and vitamin E supplementation removed this association.
The protective effect of vitamin E on telomeres has been tested previously in a few studies on birds. Vitamin E supplementation in yellow legged gull chicks (Larus michahellis) shortly after hatching showed no overall effects on telomere length (Kim and Velando, 2015). However, a post-hoc analysis revealed an interaction between nestling personality and vitamin supplementation, showing that vitamin E tended to reduce or increase telomere attrition among bold and shy nestlings, respectively (Kim and Velando, 2015). Another study in the same species showed that in ovo injections of vitamin E in clutches also failed to affect telomere length at hatching despite slightly improving the redox status of hatchlings (Parolini et al., 2017). Among altricial species, micronutrient supplementation (involving vitamin E, but also other vitamins and essential minerals) reduced telomere loss during sexual maturation in male zebra finches (Taeniopygia guttata), but did not affect telomere dynamics in either sex during the nestling period (Noguera et al., 2015). Finally, a single dose of vitamin E (administered in combination with methionine) reduced telomere attrition among adult blue tits (Cyanistes caeruleus) 1 year after treatment (Badas et al., 2015). Taking together these results and ours, it seems that vitamin E can influence telomere dynamics, but this is strongly influenced by the interplay of other internal and external factors that modulate the magnitude of the effect. In our case, no overall effect on telomere attrition or relative telomere length at the end of the nestling period was found, but a size-dependent effect was detected. We have no clear explanation for this pattern. It may suggest an action mechanism of vitamin E that is either non-linear or exerted only above threshold telomere size. Or, alternatively, it may simply result from a linear protective effect on an attrition process that is, by nature, size-dependent (op den Buijs et al., 2004). In any case, this pattern was not explicitly predicted by our initial hypothesis but, if it is further confirmed, it may have important implications for how avian longevity is affected by early nutritional conditions. Studies in birds have shown that telomere length or attrition during early development are particularly good predictors of individual survival probabilities (Heidinger et al., 2012; Boonekamp et al., 2014). Thus, by influencing telomere dynamics during early development, vitamin E could be exerting a significant effect on individual viability.
The starting hypothesis of our study was that oxidative stress is the main causal mechanism of telomere attrition. Although we found an effect of vitamin E supplementation on telomere dynamics, this hypothesis was mostly unsupported. Indeed vitamin E supplementation, which was the only treatment that impacted telomere dynamics, showed no statistically clear effects on oxidative stress biomarkers. This does not necessarily imply that vitamin E did not affect the redox status of individuals, as supplementation might have resulted in tissue specific effects, or lead to a reorganization of antioxidant defenses (Costantini et al., 2013). In addition, a lack of effect on a series of oxidative stress biomarkers does not prove that oxidative status has not been challenged (Meitern et al., 2013). In contrast to vitamin E supplementation, diquat treatment increased oxidative damage. Diquat is a powerful pro-oxidant (Koch et al., 2017) and the dose selected for this study was approximately half of the dose that cause 20% of mortality after a single injection. Accordingly, dosed nestlings in 2016 showed lipid peroxidation levels 25% higher than controls. Despite such effect on oxidative damage, no effect on telomere dynamics was found, which argues against a direct causal implication of oxidative stress on telomere dynamics. It should be noted, however, that our verification of the impact of our treatments on oxidative stress biomarkers was done in the following breeding season. Although both tests were made in the same population and under reasonably similar ecological conditions, extrapolation of results from among both sets of individuals should be done with caution. One possible alternative explanation for the lack of effect of diquat on telomere dynamics is that challenged birds allocated resources to channel oxidative stress effects to traits that were less important to fitness than telomere length (Boonekamp et al., 2017b). What mechanism—not necessarily linked to oxidative stress—may explain the effect of vitamin E on telomere dynamics then? Apart from acting as a significant antioxidant, either alone or in concert with other antioxidant compounds (Halliwell and Gutteridge, 2007), vitamin E also plays a major role in mediating cellular signaling and gene expression (Brigelius-Flohe, 2009). Thus, an alternative—but not necessarily mutually exclusive—mechanism for the observed effect of vitamin E on telomere dynamics would imply a positive effect of vitamin E on telomerase activity, as found in cell cultures in vitro (Tanaka et al., 2007; Makpol et al., 2010). This mechanism is plausible as this enzyme is still active during the nestling period in birds (Haussmann et al., 2007).
There is compelling evidence that growth compromises telomere integrity (Vedder et al., 2017; Monaghan and Ozanne, 2018). The proximate mechanisms for such a negative effect are not clear, probably resulting from resource allocation trade-offs or constraints between cell proliferation and telomere protection (Monaghan and Ozanne, 2018). Although oxidative stress is often claimed as a potential mediator of these trade-offs (Smith et al., 2016), we found little support for this, as diquat-treated nestlings showed higher oxidative damage than controls with no significant impairment of growth. Vitamin E supplemented nestlings, in turn, showed a marginal increase in growth that was not accompanied by remarkable changes in their oxidative status. However, we are dealing with phenomena involving complex physiological trade-offs, so we cannot fully discard an underlying or indirect effect of oxidative stress. For instance, vitamin E treatment might have driven supplemented nestlings to a new optimum level in the trade-off, characterized by a simultaneous—marginal—increase in growth and improved telomere dynamics with no apparent costs in terms of oxidative damage. Irrespective of the pathway, it seems that vitamin E supplementation buffered this pattern, allowing higher growth rates with no negative effects on telomere length. A positive effect of vitamin E supplementation on growth has been reported for different taxa, including birds (reviewed in Smith et al., 2016). It is often assumed that this effect is due to the direct effect of this compound on the neutralization of reactive species, but future studies might consider alternative mechanisms for the role of this compound in growth vs. self-maintenance trade-offs. Those involving effects on modulation of restoration mechanisms (e.g., telomerase activity), signaling pathways or micronutrient reallocation (Paul, 2011) are avenues worth exploring.
In conclusion, our results do not support the hypothesis that oxidative stress is a major mechanism underlying telomere attrition during early development (Boonekamp et al., 2017a; Reichert and Stier, 2017). Instead, vitamin E supplementation affected telomere dynamics, but in a process that does not necessarily involve oxidative stress as a main actor. These results highlight the relevance of nutrition—particularly micronutrient intake—during early life in cellular senescence.
Ethics Statement
The experimental procedure was approved by the CSIC Ethical Committee (ref. 266/2015) and the Madrid Competent Authority (ref. PROEX 117/15) to comply with Spanish and European laws.
Author Contributions
LP-R and TR designed the study and analyzed the data. LP-R wrote the first draft and all co-authors contributed to the final version of the manuscript. All authors contributed to fieldwork.
Funding
LP-R was supported by Severo Ochoa (SEV-2012-0262) and SECTI (UCLM) postdoctoral contracts during data collection manuscript and writing, respectively. Funding was provided by Spanish Ministry of Economy and Competitiveness (SMEC); grant number CGL2014-55969-P. Additional funding came from the project “A test of the oxidative cost of a reliable signal,” granted to TR by the internal EBD proposal call Microproyectos and financed by the SMEC, through the Severo Ochoa Program for Centers of Excellence in R+D+I (SEV-2012-0262).
Conflict of Interest Statement
The authors declare that the research was conducted in the absence of any commercial or financial relationships that could be construed as a potential conflict of interest.
Acknowledgments
We thank F. Miranda and Laboratory of Ecophysiology-EBD (ISO9001:2015 and ISO14001:2015) for assistance with lab analyses. E. Roldán, at the National Museum of Natural History-CSIC, helped with sample conservation and transport.
Supplementary Material
The Supplementary Material for this article can be found online at: https://www.frontiersin.org/articles/10.3389/fevo.2019.00173/full#supplementary-material
References
Alonso-Alvarez, C., and Galván, I. (2011). Free radical exposure creates paler carotenoid-based ornaments: a possible interaction in the expression of black and red traits. PLoS ONE 6:e19403. doi: 10.1371/journal.pone.0019403
Arnold, K. E., Ramsay, S. L., Henderson, L., and Larcombe, S. D. (2010). Seasonal variation in diet quality: antioxidants, invertebrates and blue tits Cyanistes caeruleus. Biol. J. Linn. Soc. 99, 708–717. doi: 10.1111/j.1095-8312.2010.01377.x
Ayala, R. M., Martinelli, R., and Saino, N. (2006). Vitamin E supplementation enhances growth and condition of nestling barn swallows (Hirundo rustica). Behav. Ecol. Sociobiol. 60, 619–630. doi: 10.1007/s00265-006-0206-0
Badas, E. P., Martinez, J., Rivero de Aguilar Cachafeiro, J., Miranda, F., Figuerola, J., and Merino, S. (2015). Ageing and reproduction: antioxidant supplementation alleviates telomere loss in wild birds. J. Evol. Biol. 28, 896–905. doi: 10.1111/jeb.12615
Bates, D., Machler, M., Bolker, B. M., and Walker, S. C. (2015). Fitting linear mixed-effects models using lme4. J. Stat. Softw. 67, 1–48. doi: 10.18637/jss.v067.i01
Bize, P., Criscuolo, F., Metcalfe, N. B., Nasir, L., and Monaghan, P. (2009). Telomere dynamics rather than age predict life expectancy in the wild. Proc. Biol. Sci. 276, 1679–1683. doi: 10.1098/rspb.2008.1817
Blackburn, E. H. (1991). Structure and function of telomeres. Nature 350, 569–573. doi: 10.1038/350569a0
Boonekamp, J. J., Bauch, C., Mulder, E., and Verhulst, S. (2017a). Does oxidative stress shorten telomeres? Biol. Lett. 13:20170164. doi: 10.1098/rsbl.2017.0164
Boonekamp, J. J., Dijkstra, R., Dijkstra, C., Verhulst, S., and Blanckenhorn, W. (2017b). Canalization of development reduces the utility of traits as fitness biomarkers: feather fault bars in nestling birds. Funct. Ecol. 31, 719–727. doi: 10.1111/1365-2435.12765
Boonekamp, J. J., Mulder, G. A., Salomons, H. M., Dijkstra, C., and Verhulst, S. (2014). Nestling telomere shortening, but not telomere length, reflects developmental stress and predicts survival in wild birds. Proc. Biol. Sci. 281:20133287. doi: 10.1098/rspb.2013.3287
Brigelius-Flohe, R. (2009). Vitamin E: the shrew waiting to be tamed. Free Radic. Biol. Med. 46, 543–554. doi: 10.1016/j.freeradbiomed.2008.12.007
Burraco, P., and Gomez-Mestre, I. (2016). Physiological stress responses in amphibian larvae to multiple stressors reveal marked anthropogenic effects even below lethal levels. Physiol. Biochem. Zool. 89, 462–472. doi: 10.1086/688737
Cholewa, M., and Wesolowski, T. (2011). Nestling food of European hole-nesting passerines: do we know enough to test the adaptive hypotheses on breeding seasons? Acta Ornithol. 46, 105–116. doi: 10.3161/000164511X625874
Cohen, A., Klasing, K., and Ricklefs, R. (2007). Measuring circulating antioxidants in wild birds. Comp. Biochem. Physiol. B 147, 110–121. doi: 10.1016/j.cbpb.2006.12.015
Costantini, D., Monaghan, P., and Metcalfe, N. B. (2013). Loss of integration is associated with reduced resistance to oxidative stress. J. Exp. Biol. 216, 2213–2220. doi: 10.1242/jeb.083154
Costantini, D., and Verhulst, S. (2009). Does high antioxidant capacity indicate low oxidative stress? Funct. Ecol. 23, 506–509. doi: 10.1111/j.1365-2435.2009.01546.x
Criscuolo, F., Bize, P., Nasir, L., Metcalfe, N. B., Foote, C. G., Griffiths, K., et al. (2009). Real-time quantitative PCR assay for measurement of avian telomeres. J. Avian Biol. 40, 342–347. doi: 10.1111/j.1600-048X.2008.04623.x
Eastwood, J. R., Hall, M. L., Teunissen, N., Kingma, S. A., Hidalgo Aranzamendi, N., Fan, M., et al. (2018). Early-life telomere length predicts lifespan and lifetime reproductive success in a wild bird. Mol. Ecol. 28, 1127–1137. doi: 10.1111/mec.15002
García-de Blas, E., Mateo, R., and Alonso-Alvarez, C. (2016). Specific carotenoid pigments in the diet and a bit of oxidative stress in the recipe for producing red carotenoid-based signals. PeerJ 4:e2237. doi: 10.7717/peerj.2237
Halliwell, B., and Gutteridge, J. M. C. (2007). Free Radicals in Biology and Medicine. Oxford: Oxford University Press.
Haussmann, M. F., Winkler, D. W., Huntington, C. E., Nisbet, I. C., and Vleck, C. M. (2007). Telomerase activity is maintained throughout the lifespan of long-lived birds. Exp. Gerontol. 42, 610–618. doi: 10.1016/j.exger.2007.03.004
Heidinger, B. J., Blount, J. D., Boner, W., Griffiths, K., Metcalfe, N. B., and Monaghan, P. (2012). Telomere length in early life predicts lifespan. Proc. Natl. Acad. Sci. USA. 109, 1743–1748. doi: 10.1073/pnas.1113306109
Herting, D. C., and Drury, E. J. E. (1963). Vitamin E content of vegetable oils and fats. J. Nutr. 81, 335–342. doi: 10.1093/jn/81.4.335
Hochberg, Y. (1988). A sharper Bonferroni procedure for multiple tests of significance. Biometrika 75, 800–803. doi: 10.1093/biomet/75.4.800
Isaksson, C., and Andersson, S. (2008). Oxidative stress does not influence carotenoid mobilization and plumage pigmentation. Proc. Biol. Sci. 275, 309–314. doi: 10.1098/rspb.2007.1474
Kawanishi, S., and Oikawa, S. (2004). “Mechanism of telomere shortening by oxidative stress,” in Strategies for Engineered Negligible Senescence: Why Genuine Control of Aging May Be Foreseeable, ed. A. D. N. DeGrey (New York, NY: New York Academy of Sciences), 278–284.
Kelley, K. (2018). MBESS: The MBESS R Package. R package version 4.4.3. Available online at: https://CRAN.R-project.org/package=MBESS
Kim, S. Y., and Velando, A. (2015). Antioxidants safeguard telomeres in bold chicks. Biol. Lett. 11:20150211. doi: 10.1098/rsbl.2015.0211
Koch, R. E., Hill, G. E., and Costantini, D. (2017). An assessment of techniques to manipulate oxidative stress in animals. Funct. Ecol. 31, 9–21. doi: 10.1111/1365-2435.12664
Krzywinski, M., and Altman, N. (2014). Comparing samples—part II. Nat. Methods 11, 355–356. doi: 10.1038/nmeth.2900
Lindstrom, J. (1999). Early development and fitness in birds and mammals. Trends Ecol. Evol. 14, 343–348. doi: 10.1016/S0169-5347(99)01639-0
López-Arrabé, J., Cantarero, A., Pérez-Rodríguez, L., Palma, A., Alonso-Alvarez, C., Gonzalez-Braojos, S., et al. (2015). Nest-dwelling ectoparasites reduce antioxidant defences in females and nestlings of a passerine: a field experiment. Oecologia 179, 29–41. doi: 10.1007/s00442-015-3321-7
Makpol, S., Zainuddin, A., Rahim, N. A., Yusof, Y. A. M., and Ngah, W. Z. (2010). Alpha-tocopherol modulates hydrogen peroxide-induced DNA damage and telomere shortening of human skin fibroblasts derived from differently aged individuals. Planta Med. 76, 869–875. doi: 10.1055/s-0029-1240812
Mateos, R., and Bravo, L. (2007). Chromatographic and electrophoretic methods for the analysis of biomarkers of oxidative damage to macromolecules (DNA, lipids, and proteins). J. Sep. Sci. 30, 175–191. doi: 10.1002/jssc.200600314
Matrková, J., and Remeš, V. (2014). Vitamin E improves growth of collared fl ycatcher Ficedula albicollis young: a supplementation experiment. J. Avian Biol. 45, 475–483. doi: 10.1111/jav.00368
Meitern, R., Sild, E., Kilk, K., Porosk, R., and Horak, P. (2013). On the methodological limitations of detecting oxidative stress: effects of paraquat on measures of oxidative status in greenfinches. J. Exp. Biol. 216, 2713–2721. doi: 10.1242/jeb.087528
Metcalfe, N. B., and Alonso-Alvarez, C. (2010). Oxidative stress as a life-history constraint: the role of reactive oxygen species in shaping phenotypes from conception to death. Funct. Ecol. 24, 984–996. doi: 10.1111/j.1365-2435.2010.01750.x
Monaghan, P., Metcalfe, N. B., and Torres, R. (2009). Oxidative stress as a mediator of life history trade-offs: mechanisms, measurements and interpretation. Ecol. Lett. 12, 75–92. doi: 10.1111/j.1461-0248.2008.01258.x
Monaghan, P., and Ozanne, S. E. (2018). Somatic growth and telomere dynamics in vertebrates: relationships, mechanisms and consequences. Philos. Trans. R. Soc. B 373:20160446. doi: 10.1098/rstb.2016.0446
Nettle, D., Monaghan, P., Gillespie, R., Brilot, B., Bedford, T., and Bateson, M. (2015). An experimental demonstration that early-life competitive disadvantage accelerates telomere loss. Proc. Biol. Sci. 282:20141610. doi: 10.1098/rspb.2014.1610
Noguera, J. C., Metcalfe, N. B., Boner, W., and Monaghan, P. (2015). Sex-dependent effects of nutrition on telomere dynamics in zebra finches (Taeniopygia guttata). Biol. Lett. 11:20140938. doi: 10.1098/rsbl.2014.0938
op den Buijs, J., van den Bosch, P. P., Musters, M. W., and van Riel, N. A. (2004). Mathematical modeling confirms the length-dependency of telomere shortening. Mech. Ageing Dev. 125, 437–444. doi: 10.1016/j.mad.2004.03.007
Parolini, M., Khoriauli, L., Possenti, C. D., Colombo, G., Caprioli, M., Santagostino, M., et al. (2017). Yolk vitamin E prevents oxidative damage in gull hatchlings. Royal Soc. Open Sci. 4:170098. doi: 10.1098/rsos.170098
Paul, L. (2011). Diet, nutrition and telomere length. J. Nutr. Biochem. 22, 895–901. doi: 10.1016/j.jnutbio.2010.12.001
Pérez-Rodríguez, L. (2009). Carotenoids in evolutionary ecology: re-evaluating the antioxidant role. Bioessays 31, 1116–1126. doi: 10.1002/bies.200900070
Pérez-Rodríguez, L., Romero-Haro, A. A., Sternalski, A., Muriel, J., Mougeot, F., Gil, D., et al. (2015). Measuring oxidative stress: the confounding effect of lipid concentration in measures of lipid peroxidation. Physiol. Biochem. Zool. 88, 345–351. doi: 10.1086/680688
R-Core-Team (2017). R: A Language and Environment for Statistical Computing. Vienna: R Foundation for Statistical Computing. Available online at: https://www.R-project.org/
Reichert, S., and Stier, A. (2017). Does oxidative stress shorten telomeres in vivo? A review. Biol. Lett. 13:20170463. doi: 10.1098/rsbl.2017.0463
Salomons, H. M., Mulder, G. A., van de Zande, L., Haussmann, M. F., Linskens, M. H., and Verhulst, S. (2009). Telomere shortening and survival in free-living corvids. Proc. Biol. Sci. 276, 3157–3165. doi: 10.1098/rspb.2009.0517
Smith, S. M., Nager, R. G., and Costantini, D. (2016). Meta-analysis indicates that oxidative stress is both a constraint on and a cost of growth. Ecol. Evol. 6, 2833–2842. doi: 10.1002/ece3.2080
Surai, P. F. (2002). Natural Antioxidants in Avian Nutrition and Reproduction. Nottingham: Nottingham University Press.
Tanaka, Y., Moritoh, Y., and Miwa, N. (2007). Age-dependent telomere-shortening is repressed by phosphorylated alpha-tocopherol together with cellular longevity and intracellular oxidative-stress reduction in human brain microvascular endotheliocytes. J. Cell Biochem. 102, 689–703. doi: 10.1002/jcb.21322
Vedder, O., Verhulst, S., Bauch, C., and Bouwhuis, S. (2017). Telomere attrition and growth: a life-history framework and case study in common terns. J. Evol. Biol. 30, 1409–1419. doi: 10.1111/jeb.13119
Verhulst, S., Aviv, A., Benetos, A., Berenson, G. S., and Kark, J. D. (2013). Do leukocyte telomere length dynamics depend on baseline telomere length? An analysis that corrects for 'regression to the mean'. Eur. J. Epidemiol. 28, 859–866. doi: 10.1007/s10654-013-9845-4
Keywords: antioxidant, development, diquat, growth, micronutrient, nestling, telomere attrition, tocopherol
Citation: Pérez-Rodríguez L, Redondo T, Ruiz-Mata R, Camacho C, Moreno-Rueda G and Potti J (2019) Vitamin E Supplementation—But Not Induced Oxidative Stress—Influences Telomere Dynamics During Early Development in Wild Passerines. Front. Ecol. Evol. 7:173. doi: 10.3389/fevo.2019.00173
Received: 16 December 2018; Accepted: 30 April 2019;
Published: 21 May 2019.
Edited by:
Geoffrey E. Hill, Auburn University, United StatesReviewed by:
Clotilde Biard, Université Pierre et Marie Curie, FranceSimon Verhulst, University of Groningen, Netherlands
Rebecca E. Adrian, Monash University, Australia
Copyright © 2019 Pérez-Rodríguez, Redondo, Ruiz-Mata, Camacho, Moreno-Rueda and Potti. This is an open-access article distributed under the terms of the Creative Commons Attribution License (CC BY). The use, distribution or reproduction in other forums is permitted, provided the original author(s) and the copyright owner(s) are credited and that the original publication in this journal is cited, in accordance with accepted academic practice. No use, distribution or reproduction is permitted which does not comply with these terms.
*Correspondence: Lorenzo Pérez-Rodríguez, bG9yZW56b3BlcmV6cm9kcmlndWV6QGdtYWlsLmNvbQ==