- 1Faculty of Engineering and Science, Western Norway University of Applied Sciences, Sogndalsfjøra, Norway
- 2Faculty of Environmental Sciences and Natural Resource Management, Norwegian University of Life Sciences, Ås, Norway
- 3Department of Entomology, Philip E. Marucci Center for Blueberry and Cranberry Research, Rutgers – The State University of New Jersey, Chatsworth, NJ, United States
The activation of plant defense systems in response to herbivory or experimentally applied methyl jasmonate (MeJA) involves the production of chemical defense substances functioning as warning signals to repel herbivores and protect against pathogens. They also serve as signals detectable by undamaged neighboring plants, a phenomenon called plant–plant communication. We studied how altitudinal variation in temperature and timing of snowmelt affected herbivore resistance, growth and reproduction of untreated bilberry (Vaccinium myrtillus L.) 20–500 cm from MeJA-treated ramets. Across 2 years, responses of MeJA-treated and untreated bilberry ramets were recorded twice per season along an elevational gradient in a boreal system. At low and medium altitudes, untreated bilberry showed increased herbivore resistance and reduced growth and reproduction up to 500 cm from MeJA-treated ramets. In the warmer sites at these altitudes, the effects persisted for 2 years for ramets up to 100 cm from the treated ramets. At high altitudes, however, only untreated ramets growing 20–100 cm from the treated ramets showed increased resistance to insect herbivores and reduced reproduction, but these effects did not persist into the second year. Altitudinal variation in climate affected trade-offs between plant defense, growth, and reproduction. Our findings indicate that plant–plant communication is also influenced by the combination of changes in climate and time after induction. Adaptations of plants growing under increasing temperature in high-latitude environments can profoundly impact ecosystem functioning, especially where bilberry, the key plant species in the boreal system, interacts with its herbivores.
Introduction
Global warming is currently occurring at an unprecedented rate (Brooker, 2006). Mean global temperature is projected to increase by 1.5–2.0°C in coming decades, causing longer drought periods in some areas and more precipitation in others (IPCC, 2018). Climate change is likely to be a major threat to biodiversity in many regions worldwide (Sala et al., 2000; Thomas et al., 2004). High latitude tundra and boreal areas are particularly vulnerable to climate change-induced degradation and loss, with woody shrubs encroaching onto the tundra (IPCC, 2018). At northern latitudes, milder winters and warmer summers will prolong the growing season, alter plant growth patterns, and eventually cause a mismatch between, for example, herbivore larvae emergence and bud burst, and leafing in host plants (Bale et al., 2002; Stenseth and Mysterud, 2002; Visser and Both, 2005). Climate change is also expected to influence plant phenology (differences in growth and maturation), physiology (e.g., stomatal functioning in plants and the physicochemical properties of volatiles compounds), and resource allocation from growth or reproduction, or both, to defense when under herbivore attack. These can have direct and indirect implications for natural ecosystem functioning (Post et al., 2009; Penuelas and Staudt, 2010).
Plant coexistence can influence interactions with organisms such as herbivores, predators, pollinators, and microorganisms. Indeed, a plant’s association with its neighbors can take several forms, with competitive and facilitative interactions playing fundamental roles in shaping natural communities. Plant–plant competition has long been a key component of many classic ecological theories (Keddy, 1989). In contrast, the importance of positive plant–plant interactions, such as facilitation, has only more recently been recognized as being equally important in regulating community composition and ecosystem functioning (Brooker, 2006; Callaway, 2007; Brooker et al., 2008). Neighboring plants can facilitate each other by promoting their growth (Plath et al., 2011), for example, through fixing nitrogen (Joëlle et al., 2010), or helping each other in defense against herbivores either directly, through reducing damage by diverting herbivores from their host plant (Morley et al., 2005), or indirectly, by attracting the herbivores’ natural enemies (Landis et al., 2000). These negative and positive plant–plant interactions can be mediated by biotic (i.e., herbivore attack) and abiotic factors (i.e., environmental drivers) (Ameye et al., 2018; Catola et al., 2018). However, little is known about the effects of climate in modulating these interactions.
Plants under attack by herbivores and pathogens activate inducible defense mechanisms such as the emission of volatile organic compounds (VOCs) from aboveground and belowground tissues (Knudsen et al., 1993; Dudareva et al., 2004; Steeghs et al., 2004). Once VOCs are emitted, however, they convey ‘public’ information that can be perceived by surrounding plants and organisms. For instance, herbivore-induced VOCs can attract natural enemies of herbivores (Sabelis, 1999), provide protection against pathogens (Shiojiri et al., 2006), and repel herbivores (Heil, 2004), potentially defending plants against their enemies (Kessler and Baldwin, 2001). Moreover, undamaged neighboring plants can recognize VOCs emitted from attacked plants and use them as a cue to mobilize their own defense systems (Kobayashi and Yamamura, 2003; Karban, 2008; Karban et al., 2014). Plant communication can be influenced by climate because VOC production, volatility and, consequently, VOC movement at the leaf surface, are controlled by temperature (Niinemets et al., 2004). Recent studies highlight possible effects of climate change on the biosynthesis and abundance of VOCs (Rosenstiel et al., 2003; Calfapietra et al., 2007), which could mitigate their ecological functions (Pinto et al., 2007). Higher VOC production and volatility under elevated temperatures (Helmig et al., 2007) are expected to change plant–herbivore interactions by altering the ability of specialist herbivores to locate their hosts, thereby increasing antiherbivore defense responses and modifying plant–plant interactions (Yuan et al., 2009). However, we still do not fully understand how inter-plant communication mechanisms vary under different climate regimes, how these could be affected by changing environmental conditions, and what the consequences could be for ecological interactions and ecosystem functioning.
Plants incur costs if limiting resources, such as nitrogen and carbon, are invested in defense rather than biomass and fruit production (Redman et al., 2001). Studies have shown that the activation of plant defense systems are energetically costly and reduce plant growth and reproduction (Accamando and Cronin, 2012; Nabity et al., 2013; Seldal et al., 2017; Benevenuto et al., 2018). As plant defense responses induced by the phytohormone methyl jasmonate (MeJA) are generally similar to those induced by insect herbivory (Yang et al., 2013; Moreira et al., 2018a), the application of exogenous MeJA is a useful tool to stimulate plant resistance in studies of plant–herbivore interactions. MeJA can trigger the re-allocation of energy from plant primary metabolism to the activation of plant defensive genes (Howe and Jander, 2008). Plant defense responses to MeJA include the production of various secondary metabolites and anti-digestive proteins such as proteinase inhibitors, which can harm both specialist and generalist herbivores (Baldwin, 1999; Kessler and Baldwin, 2001; Thaler et al., 2001; Bruinsma et al., 2008). MeJA has been also shown to induce the emission of plant volatiles that affect the plants’ interactions with individuals of the same trophic level (i.e., neighboring plants) as well as those from higher trophic levels such as the attraction of natural enemies of herbivores (Dicke et al., 1999; Gols et al., 2003; Rodriguez-Saona et al., 2013). For example, in bilberry (Vaccinium myrtillus L.), a key food source for many herbivore species in boreal forests, treatment with MeJA increases resistance to herbivores and reduces plant growth and reproduction for several years (i.e., prolonged multiannual defense) (Benevenuto et al., 2018). These trade-offs between herbivore resistance and both growth and reproduction have previously been observed on MeJA-treated bilberry plants as well as on untreated neighbors (Benevenuto et al., 2018). Given that ecosystem composition and functioning can affect the ecosystem services on which human society depends (Peterson et al., 1998; Hooper et al., 2005), there is a strong practical need to understand those processes that regulate biodiversity, particularly in the light of potential impacts of climate change. It is therefore timely to consider how variations in climate might modulate plant–plant interactions and their effects, especially in high-latitude ecosystems.
In this study, we investigate how altitudinal variation in temperature and the time of snowmelt affect growth, reproduction and the induction of defenses in MeJA-treated and untreated neighboring bilberry ramets. Because VOCs can induce defenses in neighboring plants some distance away (Heil and Karban, 2010; Hare, 2011; Benevenuto et al., 2018), we predict similar patterns of defense in MeJA-induced and untreated neighboring bilberry ramets at different distances from the induced plant, for example, reduced growth, reproduction, and herbivory. Such a distance effect would indicate plant–plant communication. Furthermore, because plant-induced defenses appear to decline with increasing elevation (Moreira et al., 2018b) and elevated temperature is known to increase biogenic VOC emissions (Guenther et al., 1995; Helmig et al., 2007), we expect to find stronger plant–plant communication effects in the warmer, lower altitude environments.
Materials and Methods
Study System
We conducted this study along an elevational gradient in the boreal pine-bilberry forest ecosystems of Kaupanger, the inner Sognefjord, western Norway (61.2°N, 007.2°E) in 2016 and 2017. Annual precipitation there is 700–900 mm with a mean summer temperature range of 12–16°C (Moen et al., 1999). The most abundant tree is pine (Pinus sylvestris L.), with dwarf shrubs, such as bilberry, lingonberry (Vaccinium vitis-idaea L.), and crowberry (Empetrum nigrum L.) dominating the field layer. Bilberry is a long-lived deciduous clonal dwarf shrub with evergreen stems usually 10–60 cm high (Ritchie, 1956; Flower-Ellis, 1971). Although we do not have specific information on clone size and distribution in the study area, we have based our study on the assumptions that rhizomes can reach approximately 200 cm in length, depending on age, and that there is considerable genetic variation within the population (Flower-Ellis, 1971; Albert et al., 2003, 2004). Bilberry is considered a key plant in boreal forests because it is a food source for many vertebrate and invertebrate herbivores (Hegland et al., 2010). The main mammalian herbivores feeding on bilberry in this area are red deer (Cervus elaphus L.) and various rodent species, whereas the commonest insect herbivores are Geometridae larvae (S. J. Hegland, personal observation), and the main visitors to bilberry flowers are bumblebees, solitary bees, as well as ants (S. J. Hegland, unpublished data).
Gradient Design
Inner Sognefjord is topographically diverse with marked altitudinal gradients occurring within a relatively small area. These are ideal for climate research, making it practical and convenient to carrying out ecological experiments over short geographical distances. We used a natural altitudinal gradient ranging from just above sea level to the subalpine zone to investigate the effects of variation in climate, mainly temperature and the timing of snowmelt, on plant–herbivore interactions and plant–plant communication. Anorthosite bedrock and moraine material dominate the whole gradient (NGU, 2019) and there are no latitudinal or longitudinal influences. As such this gradient design reduces the possibility of confounding effects that can often be a challenge in climate-gradient studies.
We conducted an experiment at three different elevations representing an altitudinal gradient along which temperature, timing of snowmelt and length of the growth season varied (Figure 1): ‘Low’ elevation, ca. 100 m a.s.l. (submontane zone); ‘Medium’ elevation, ca. 500 m a.s.l. (mid-montane zone); and ‘High’ elevation, ca. 900 m a.s.l. (subalpine zone). We placed four data loggers (TRIX 8 LogTag, Auckland, New Zealand) at each site to record data on average temperature and relative humidity throughout the study season in both years. We recorded the timing of snowmelt as the Julian day on which each site became largely snow free. All experimental sites faced south west, thereby reducing incidental environmental variation, such as light conditions. Low and Medium sites consisted of more than 10-year-old clear-cuts with small pines, birches and alders that produced negligible shadow. The High site was a naturally open subalpine area just below the tree line. Unpublished data from 2016 showed that there was relatively little variation in pH (mean, 4.19) and slope (16.4 degrees) among sites, although soil organic matter (mean, 60.4%) was slightly lower at the low altitude (Knut Rydgren, personal communication 2016). Vegetation structure was similar among the sites, with a field layer and scattered tree layer up to ca. 5 m. In total, the sites represent similar habitat characteristics in regard to factors that are not under direct control of climate – such as shading, slope, and soil parameters.
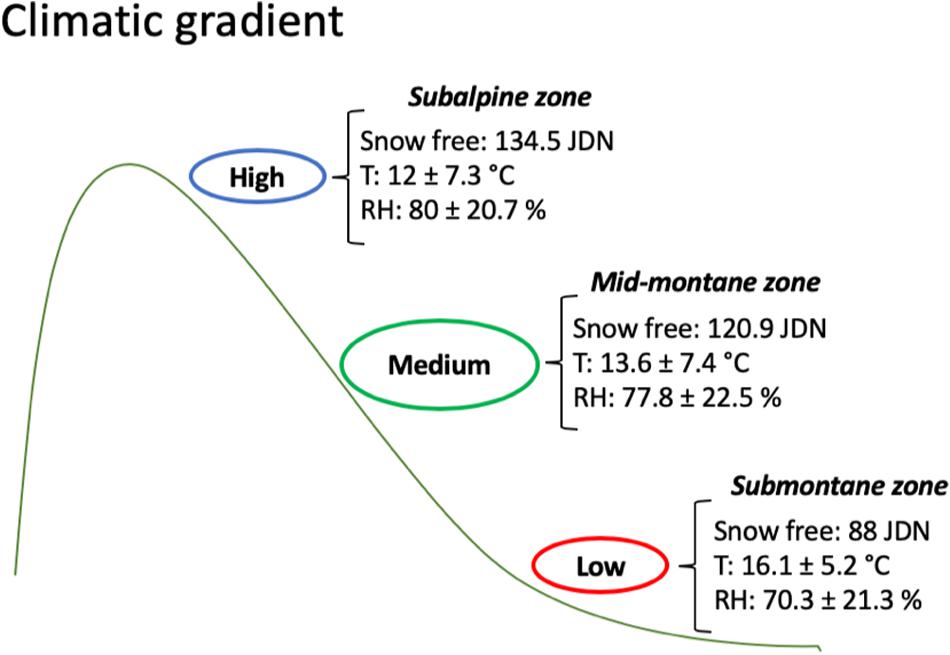
Figure 1. Average timing of snowmelt, temperature, and relative humidity across the elevational gradient. Snow free = Julian Day Number (JDN) for complete snowmelt; T = air temperature; RH = relative air humidity.
Experimental Design and Data Collection
In May and June 2016, at each site (Low, Medium, and High), we established 20 150 m2 (10 m × 15 m) blocks, leaving a minimum of 10 m between each to avoid interference from neighboring blocks. Within each block, two transects were established at least 10 m apart with five individually marked bilberry ramets in each, ranging in height from 10 to 25 cm. We located the five ramets at 10–30 cm (called dist. 20 hereafter), 40–60 cm (dist. 50), 80–120 cm (dist. 100), and 400–530 cm (dist. 500) from the first ramet (dist. 0) in each transect (Figure 2). Treated and control transects were randomly assigned within each block. The first ramets (dist. 0) were subjected to one of two treatments: 10 mM MeJA application, termed the MeJA-treated or focal ramet (treated transect), or a water: ethanol application (control transect). To achieve the desired concentration of MeJA, 4.1M MeJA stock solution (Bedoukian Research, Danbury, CT, United States) was diluted 1:10 with 95% (v/v) ethanol and diluted further with water to get a final concentration of 10 mM MeJA (Seldal et al., 2017). Ethanol was added to water at the same final concentration as that in the 10 mM MeJA solution (41:1) for the control. To avoid rapid evaporation of MeJA, a cotton wad was attached to the stem at the ground and saturated with 10 mM MeJA (treatment) or water-ethanol (control) to the point of run-off. This was repeated three times at 1-week intervals after the initial application to simulate attack by herbivores. This MeJA concentration (10 mM) and application method and timing were shown in our earlier studies to activate defenses in the treated bilberry plants as well as in conspecific neighboring plants under similar natural settings (Seldal et al., 2017; Benevenuto et al., 2018, 2019, 2020). The ramets were not exposed to further treatments in 2017, to reveal any possible multiannual effects. To evaluate possible plant–plant communication between MeJA-treated and untreated neighboring ramets, only the first ramet (dist. 0) in the transect was treated (Benevenuto et al., 2018). The other ramets along the transects served as test subjects.
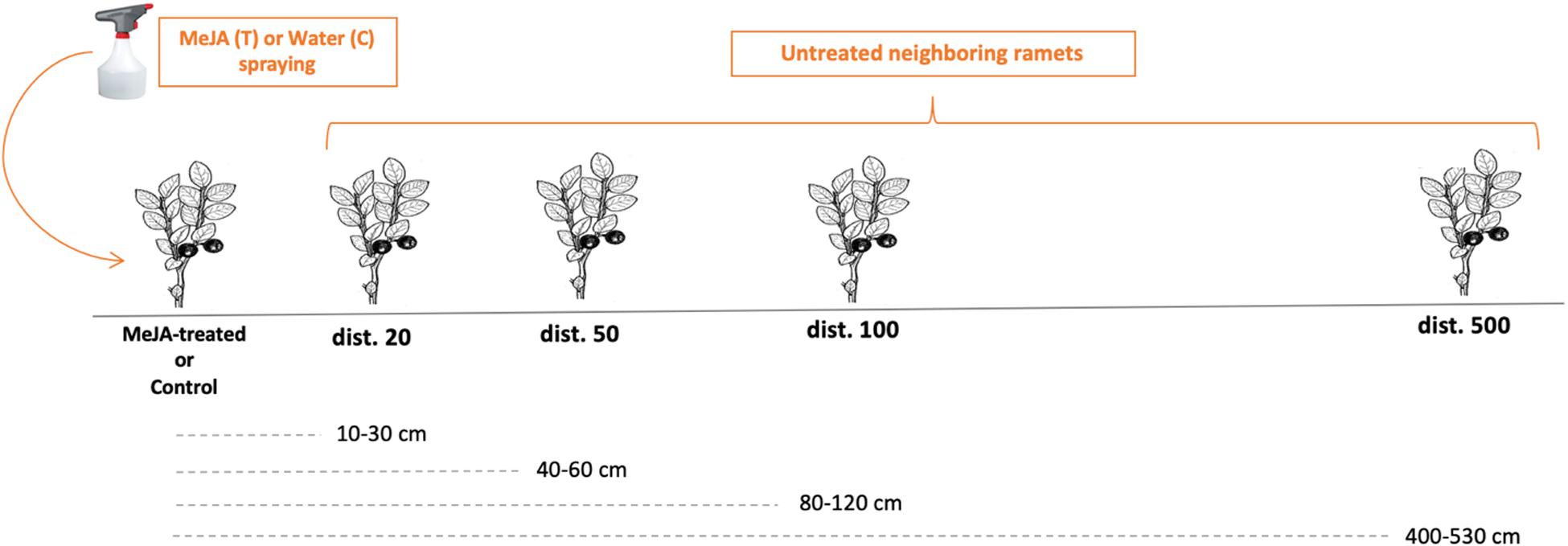
Figure 2. Transect design showing the distances between MeJA-treated or Control bilberry ramets and their untreated neighbors. C = control transect; T = treated transect; MeJA = methyl jasmonate.
We used a similar sampling procedure to that adopted in a previous study (Benevenuto et al., 2018). In May/June 2016 (sampling time 1 [ST1]), before the start of treatment at all sites, we measured ramet height from the ground to crown with a ruler, and stem diameter at ground level with a digital caliper, for all the marked plants on the transects. We also counted the number of annual shoots, flowers, leaves, browsed shoots and insect-chewed leaves. We measured the same variables again at the end of the season (sampling time 2 [ST2]), 6 weeks (42 days) after the start of treatment. On this latter occasion, we also counted the number of berries. The same procedure was repeated in 2017 except that the focal plants and controls were not treated then. Plant height (H), stem diameter (DS), and the number of shoots (AS) were used to calculate the dry mass (DM) of each ramet, applying the formula proposed by Hegland et al. (2010): log2(DM) = 1.41700 × log2(DS) + 0.97104 × log2(H) + 0.44153 × log2(AS + 1) - 7.52070. This provided a non-destructive estimate of plant size.
Data Analyses
We assessed if MeJA induced anti-herbivore defenses in wild bilberry ramets by analyzing the patterns of herbivory (proportion of chewed leaves by insect herbivores and proportion of mammalian-browsed shoots), growth (changes in biomass, through dry mass calculation), and fruit set (proportion of berries to flowers) of MeJA-treated and untreated neighboring ramets at different distances, across an elevational gradient in two consecutive seasons. The analysis involved comparing control ramets with the corresponding ramets in the experimental MeJA-treated transects (dist. 0), and for untreated neighboring ramets at increasing distance from the control and experimental ramets (dist. 20–500), at each site along the elevational gradient in 2016 and 2017. The response variables were the changes in the proportion of chewed leaves, proportion of browsed shoots, and biomass, calculated by subtracting the values at ST1 (beginning of the season) from those at ST2 (end of the season). These provided measures of seasonal change for each year. Effects on reproduction were analyzed yearly, based on measurements of the number of flowers present in May–June and the number of berries in August–September in each year, from which fruit set was calculated as the proportion of berries to flowers.
For each response variable, we used the lme4 (Bates et al., 2014) and mixlm (Liland and Saebø, 2014) libraries in R Development Core Team (2012) to fit generalized linear mixed effect models (GLMM) with Gaussian error distribution with identity link and performed posterior analysis of variance (ANOVA). For all models, in each year (2016 and 2017), we entered treatment (control and MeJA dist. 0–500) and site (Low, Medium, and High), with interaction terms as fixed effects. Blocks within each elevational site were fitted as random effects. To account for variation in plant size, the following covariates were included: total number of leaves at ST1 (for insect herbivory model), total number of shoots at ST1 (for mammalian herbivory model), total biomass at ST1 (for growth model), and total number of flowers at ST2 (for the fruit-set model). The treatment effects on reproduction were analyzed yearly, based on estimates of fruit set as defined above. Visual inspection of residual plots did not reveal any obvious deviation from homoscedasticity or normality for any model. To determine the presence of an interaction effect, we performed likelihood ratio tests of the full model against the model without the effect or interaction in question. We assessed the statistical significance (P-values) of single factors and their respective interactions by performing ANOVA for each model. Differences and mean separations between control and MeJA (dist. 0–500) ramets, within each elevational site, were analyzed by Tukey post hoc comparisons (α = 0.05) (Supplementary Tables S1, S2).
Results
Insect herbivory, measured as the change in the proportion of chewed leaves, was significantly reduced in MeJA-treated and untreated neighboring bilberry ramets at all distances (dist. 20–500) at the Low and Medium sites, and up to dist. 100 at the High site in 2016, compared with that of control ramets. This reduction in insect herbivory was maintained in 2017, but only in MeJA-treated ramets and in untreated neighbors up to dist. 50 at the Low and Medium sites, and not at all at the High site (Figure 3 and Supplementary Table S1).
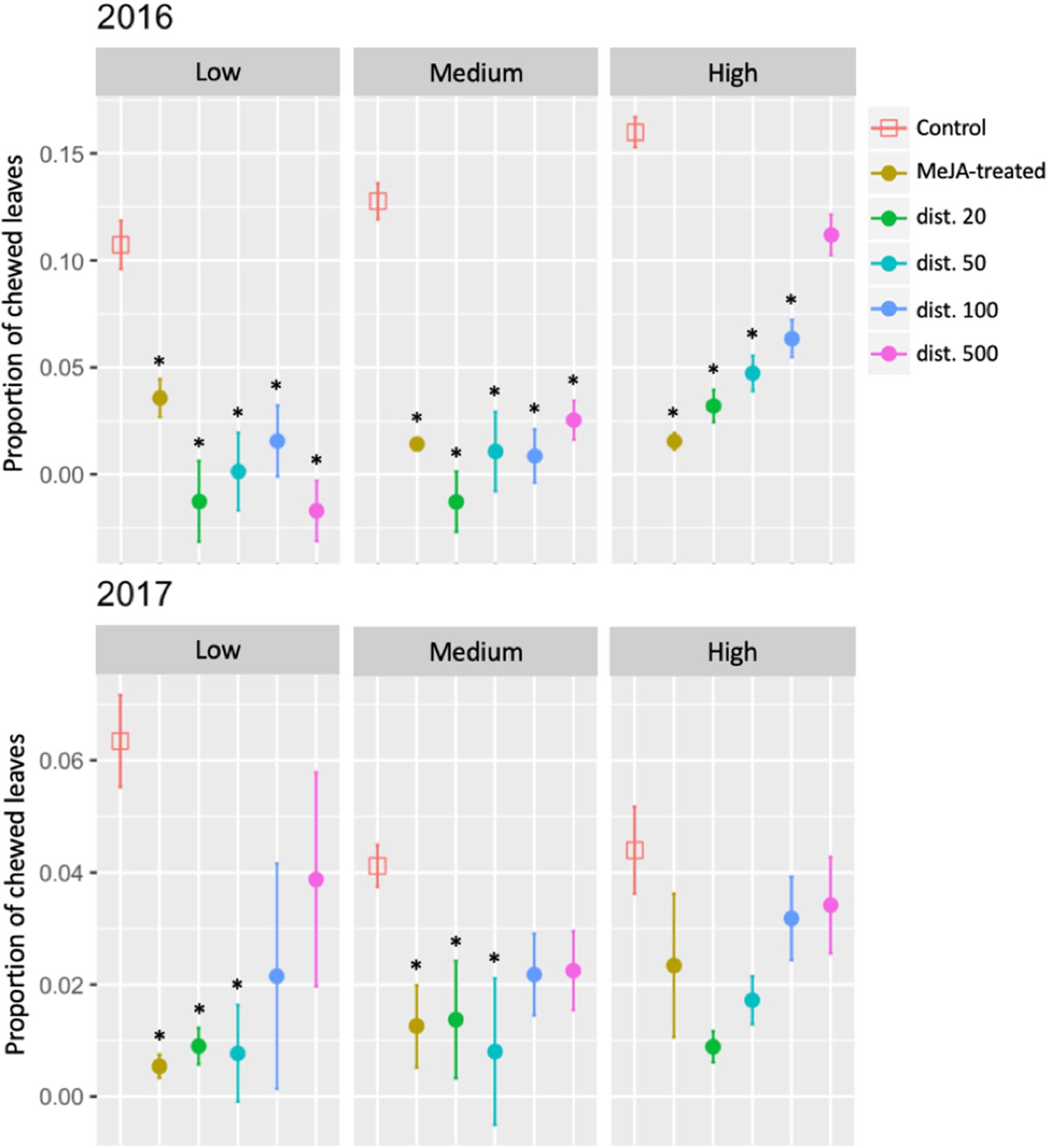
Figure 3. Seasonal changes in insect herbivory (proportion of chewed leaves) of MeJA-treated and untreated neighboring (dist. 20–500) bilberry plants (Vaccinium myrtillus L.) across an elevational gradient (Low, Medium, and High) for two consecutive years (2016 and 2017). The seasonal change in the proportion of insect chewed leaves (no. chewed leaves/total number of leaves) was calculated as the difference each year between the value at the end of the growing season (August/September) and that at the beginning (May/June). Error bars represent standard error (SE) of the mean; ∗ represents significant differences between MeJA-treated and untreated neighbors (dist. 20–500) vs. Control ramets (Tukey, P < 0.05).
Mammalian herbivory, measured as the change in the proportion of browsed shoots, was similarly significantly reduced in both years in MeJA-treated and neighboring ramets at all distances at both Low and Medium sites, compared with that in control plants. At the High site, however, there was no statistically significant reduction in browsing pressure other than in MeJA-treated ramets (dist. 0), and then only in 2016 (Figure 4 and Supplementary Table S1).
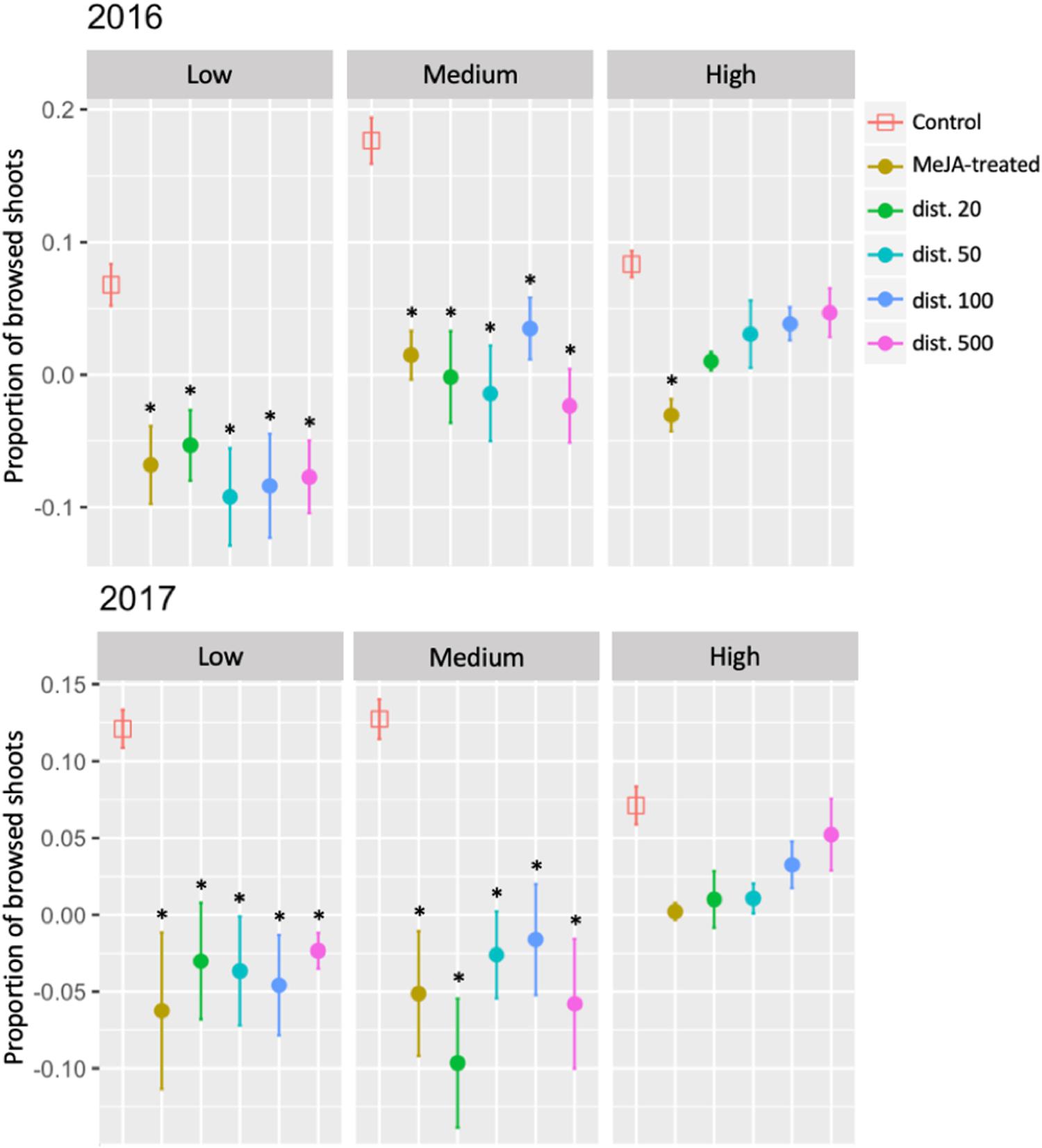
Figure 4. Seasonal changes in mammalian herbivory (proportion of browsed shoots) of MeJA-treated and untreated neighboring (dist. 20–500) bilberry plants (Vaccinium myrtillus L.) across an elevational gradient (Low, Medium, and High) for two consecutive years (2016 and 2017). The seasonal change in the proportion of deer-browsed shoots (no. browsed shoots/total number of shoots) was calculated as difference each year between the value at the end of the growing season (August/September) and that at the beginning (May/June). Error bars represent standard error (SE) of the mean; ∗ represents significant differences between MeJA-treated and untreated neighbors (dist. 20–500) vs. Control ramets (Tukey, P < 0.05).
In 2016, seasonal growth (change in biomass) was reduced in MeJA-treated and nearby neighboring ramets (dist. 20–50) at both Low and Medium sites compared with the control plants, but no significant difference was observed at the High site. A year after treatment (2017), biomass production continued to be significantly depressed relative to control plants at the Low site at distances up to ca. 50 cm, and at all distances at the Medium site. At the High site, however, which had displayed no significant response in 2016, significant reductions in growth were recorded in 2017 in the 2016 MeJA-treated experimental ramets and their closest neighbors (dist. 20), relative to the control (Figure 5 and Supplementary Table S2).
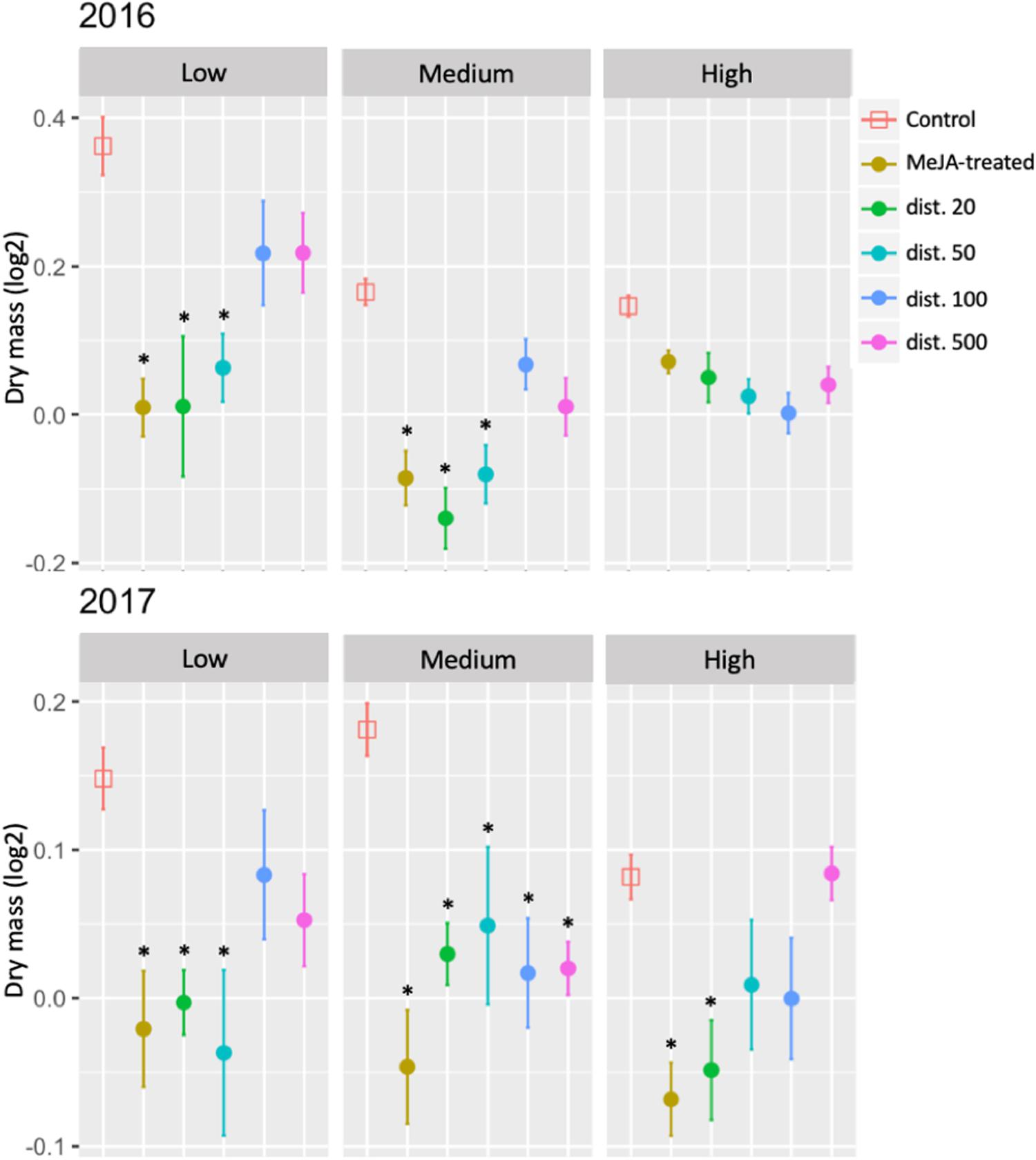
Figure 5. Seasonal changes in growth (biomass) of MeJA-treated and untreated neighboring (dist. 20–500) bilberry plants (Vaccinium myrtillus L.) across an elevational gradient (Low, Medium, and High) for two consecutive years (2016 and 2017). The seasonal change in growth (biomass) was calculated as the difference each year between the value at the end of the growing season (August/September) and that at the beginning (May/June). Error bars represent standard error (SE) of the mean; ∗ represents significant differences between MeJA-treated and untreated neighbors (dist. 20–500) vs. Control ramets (Tukey, P < 0.05).
Fruit set, measured as the proportion of berries to flowers, was significantly reduced in MeJA-treated and untreated neighboring ramets at distances from ca. 20 to 100 cm at both Low and High sites in 2016. The effect of MeJA-application was more marked at the Medium site where reduced fruit set was recorded in untreated neighbors up to ca. 500 cm away (Figure 6 and Supplementary Table S2). Reduced fruit set was recorded for MeJA-treated and untreated neighboring ramets in 2017 at the Low and Medium sites at distances up to ca. 100 cm, but not in any of the plants at the High site at any distance (Figure 6 and Supplementary Table S2).
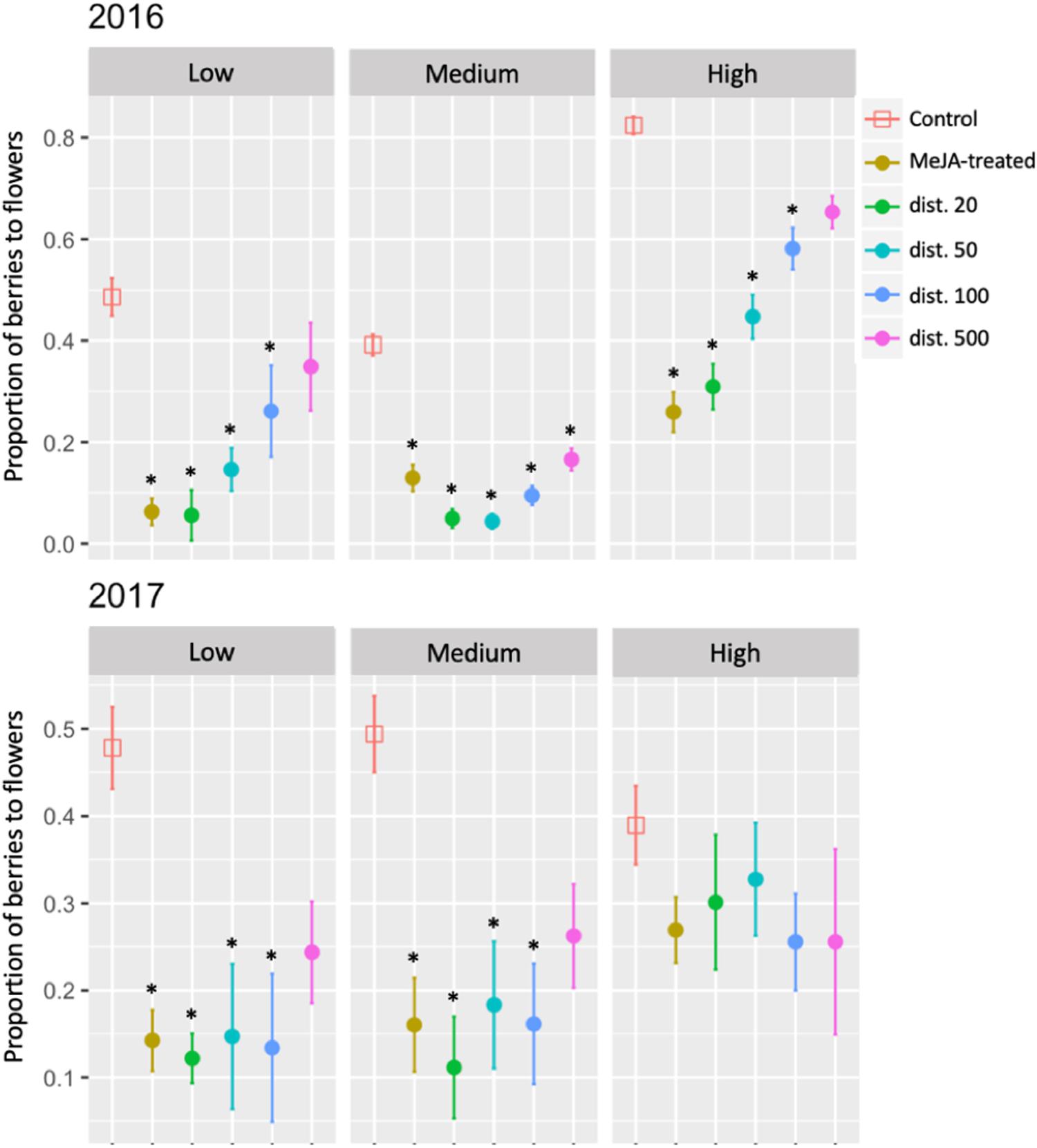
Figure 6. Fruit set (proportion of berries to flowers) of MeJA-treated and untreated neighboring (dist. 20–500) bilberry plants (Vaccinium myrtillus L.) across an elevational gradient (Low, Medium, and High) for two consecutive years (2016 and 2017). Fruit set is the ratio between the total number of berries divided by the total number of flowers in each year. Error bars represent standard error (SE) of the mean; ∗ represents significant differences between MeJA-treated and untreated neighbors (dist. 20–500) vs. Control ramets (Tukey, P < 0.05).
Discussion
Our results show that treated plants and untreated neighbors up to 500 cm away appear to have activated their defense systems in response to treatment with MeJA, as shown by reductions in both insect and mammalian herbivory, relative to control plants in the same area. This in turn resulted in reduced growth and reproduction in these MeJA-treated plants and their untreated neighbors, presumably due to the reallocation of resources from growth and reproduction to defense. This effect was observed at all elevations, but was strongest at Low and Medium altitude sites, where the effect persisted into a second year, in line with our predictions. At these sites the effects were more strongly induced and persistent also among untreated conspecific ramets at intermediate to long distances (ca. 100–500 cm). Conversely, at the High site, where temperatures are lower and the growing season is shorter, only MeJA-treated and untreated neighboring ramets at short to intermediate distances (ca. 20–100 cm) showed reduced herbivory and fruit set, and this effect did not persist into the next year. The single instance of reduced growth recorded in 2017 at the High site in the 2016 MeJA-treated experimental plants and close neighbors (dist. 20) may have been coincidental and could have arisen if the plants were responding to an instance of natural herbivory and the release of volatile defense compounds.
We found clear neighborhood/distance effects of MeJA-treatment on bilberry growth, reproduction, and herbivory resistance across all elevations in both MeJA-treated and untreated neighboring ramets. These effects were largely as predicted across elevation and time, and support the idea of plant–plant communication, with both MeJA-treated and untreated neighboring ramets up to 5 m away evidently activating their defense systems, at least in 2016 when the plants were treated. Our results are consistent with other studies showing that above- (Tamogami et al., 2008; Rodriguez-Saona et al., 2009; Moreira et al., 2018b; Kalske et al., 2019) and belowground (Jassbi et al., 2010; Falik et al., 2011; Rasmann et al., 2012; Elhakeem et al., 2018) signals emitted by induced plants can activate the defense system of uninduced neighbors (Dicke and Bruin, 2001; Baldwin et al., 2002; Pickett et al., 2003; Karban et al., 2014; Delory et al., 2016). Moreover, these effects were more pronounced and consistent in the sub-montane and mid-montane climatic zones (Low and Medium sites) than in the subalpine zone (High sites), suggesting that plant–plant communication in bilberry, either through airborne or belowground signaling from MeJA-treated ramets, is apparently modulated by climatic conditions.
The reduction in growth, fruit set, and levels of insect and mammalian herbivory, in untreated bilberry ramets growing within about 5 m of MeJA-treated ramets suggests that there is a trade-off between growth and defense. This trade-off apparently persisted, albeit less markedly, for at least the next year in untreated neighboring plants up to ca. 100 cm from the treated ramets at the Low and Medium sites, suggesting that defense activation of induced plants and their neighbors growing in sub-montane to mid-montane zones in the boreal forest is multiannual. In a previous study, in the same ecosystem and area, at ca. 350 m a.s.l., we also found evidence of multiannual plant–plant communication effects between MeJA-treated and untreated neighboring ramets (Benevenuto et al., 2018).
Although MeJA-treated and untreated bilberry ramets up to ca. 100 cm away showed reduced insect herbivory and fruit set initially at the High site, neither growth nor mammalian herbivory, other than on the treated ramets, were significantly reduced. Furthermore, in contrast to plants growing at lower elevations, these effects did not persist through to the next growing season, further evidence that climate impinges the effectiveness of plant–plant communication in an induced defense system. The elevational gradient used in our study parallels a natural climatic gradient and, as such, average temperatures together with relative humidity and consequent timing of snow melt at the Low and Medium sites are higher than at the High site, in what is effectively the subalpine zone. Plants growing at this latter zone experience a more stressful environment with lower average temperatures, later snowmelt, and, consequently, a shorter growing season than plants at lower altitudes in this boreal ecosystem. Overall, climate can be an important abiotic factor in determining the intensity and efficacy of induced plant defenses, for example, by affecting the release of plant volatiles (Gouinguené and Turlings, 2002; Ameye et al., 2018). Such effects can alter plant–herbivore and plant–plant interactions and can result in important changes in natural ecosystem functioning, such as cyclic fluctuations in herbivore populations (Haukioja and Hanhimäki, 1985).
The relatively warmer environments at the Low and Medium elevation sites, together with their longer growth seasons, boosted the performance of the induced defense system in bilberry and produced more effective plant–plant communication. Although we have not determined the type of signaling, it is probable that both aboveground (airborne VOCs) and belowground (VOCs or ramet contact) processes were involved in activating the defense systems of untreated neighboring ramets. Internal and external factors can effect VOC emissions qualitatively and quantitatively (Kesselmeier and Staudt, 1999; Penuelas and Llusia, 2001, 2003; Niinemets et al., 2004; Funk et al., 2005; Duhl et al., 2008; Pellegrini et al., 2012; Kegge et al., 2013; Pezzola et al., 2017). For instance, elevated temperature increases biogenic VOC emission by enhancing enzyme activity during synthesis, raising vapor pressure, and decreasing the resistance to diffusion (Tingey et al., 1991; Guenther et al., 1993; Gouinguené and Turlings, 2002). Altered VOC emissions in a warmer environment can affect signaling efficacy directly in various ecological interactions in natural ecosystems such as plant–herbivore, plant–pollinator, and multi-trophic interactions (Helmig et al., 2007; Valkama et al., 2007; Yuan et al., 2009), which can enhance the defense role of induced VOCs in warmer climates. Conversely, moderately elevated temperatures enhance feeding by certain herbivores (Bale et al., 2002; Valkama et al., 2007), thereby increasing inducible VOC emissions (Gouinguené and Turlings, 2002; Himanen et al., 2009). Besides temperature and other main environmental factors of climate change, VOC emissions from plants could also be altered by other abiotic factors, such as water, nutrient and resource availability, interacting with these main ones (Himanen et al., 2009; Copolovici et al., 2014; Catola et al., 2018), as well as altering phenology such as plant seasonality and leaf duration (Constable et al., 1999). Our high-altitude site, located in the subalpine zone of the boreal forest biome, experienced cooler average temperatures, limited resource availability, and had a shorter growing season. This could result in lower VOCs emissions from MeJA-treated plants or lower response of non-induced plants to these VOCs in plants at higher altitudes, perhaps explaining their reduced plant–plant communication capacity compared with that of bilberry ramets growing at lower altitudes. Future studies are needed to confirm the involvement of VOCs (above and belowground) in plant–plant communication in bilberry and the changes in emissions with increasing elevation.
The more pronounced plant–plant communication found in bilberry ramets growing in the submontane (Low site) and mid-montane (Medium site) zones reflects the antiherbivore defense strategy of plants growing under milder environmental conditions. In a previous study using the same elevational gradient, our molecular and ecological data showed that high-altitude bilberry in the boreal forest invest more in constitutive defenses, whereas low-altitude plants rely more on induced defenses (Benevenuto et al., 2020). In that study by Benevenuto et al. (2020) we also showed higher herbivory at lower altitudes compared to the subalpine site. Bilberry plants growing at lower altitudes, where herbivore pressure and seasonal temperatures are higher, appeared more responsive to simulated herbivore attack by diverting resources from growth and reproduction to induced antiherbivore defenses in both treated and untreated neighboring ramets. This suggests that bilberry plants, growing under warmer conditions and higher levels of herbivory, are adapted to be more flexible in their responses compared with conspecifics growing in subalpine zones. This flexibility has consequences both for natural ecosystem functioning, by modulating plant–herbivore interactions (e.g., outbreaks of herbivore populations), and for enhanced plant–plant interactions (e.g., detection and response to VOC emissions by adjacent plants).
In summary, our findings provide evidence for climate modulation of plant–plant communication as part of the induced plant defense system in bilberry. Low (submontane zone) to Medium altitude (mid-montane zone) environments in the boreal system, with higher average temperatures and longer growth seasons, favored induced defenses in the ramets of neighboring conspecifics, at least within ca. 5 m of a source plant. The greater prominence of this inducible plant defense system at these lower altitudes may presage the kinds of changes expected in plant–plant communication systems in a warmer world, which will likely feedback to further influence ecological functioning. Although challenging to perform, simulations of climate-change effects on plant–herbivore and plant–plant interactions in natural systems could help us predict the possible ecological consequences of a changing climate on vulnerable ecosystems, especially high-latitude systems in which there are marked dependences among species, such as bilberry and most insect and mammalian herbivores in the boreal forest.
Data Availability Statement
All datasets generated for this study are included in the article/Supplementary Material.
Author Contributions
TS, SH, SM, and CR-S conceived the initial idea and designed field work. RB carried out the field experiments, collected, analyzed and interpreted the data, and wrote the manuscript. All authors contributed to critically reading and editing the manuscript, and they approved its final form.
Funding
This research was financially supported by the Norwegian Research Council (project no. 204403/E40), the Norwegian Environment Agency.
Conflict of Interest
The authors declare that the research was conducted in the absence of any commercial or financial relationships that could be construed as a potential conflict of interest.
Acknowledgments
We thank Torbjørn Stokke, Sigurd Daniel Nerhus, and Mark Andrew Gillespie for their help with establishment and execution of the field experiment.
Supplementary Material
The Supplementary Material for this article can be found online at: https://www.frontiersin.org/articles/10.3389/fevo.2020.00117/full#supplementary-material
References
Accamando, A. K., and Cronin, J. T. (2012). Costs and benefits of jasmonic acid induced responses in soybean. Environ. Entomol. 41, 551–561. doi: 10.1603/EN11277
Albert, T., Raspé, O., and Jacquemart, A.-L. (2003). Clonal structure in Vaccinium myrtillus L. revealed by RAPD and AFLP markers. Int. J. Plant Sci. 164, 649–655.
Albert, T., Raspé, O., and Jacquemart, A.-L. (2004). Clonal diversity and genetic structure in Vaccinium myrtillus populations from different habitats. Belgian J. Bot. 137, 155–162.
Ameye, M., Allmann, S., Verwaeren, J., Smagghe, G., Haesaert, G., Schuurink, R. C., et al. (2018). Green leaf volatile production by plants: a meta-analysis. New Phytol. 220, 666–683. doi: 10.1111/nph.14671
Baldwin, I. T. (1999). Inducible nicotine production in native Nicotiana as an example of adaptive phenotypic plasticity. J. Chem. Ecol. 25, 3–30. doi: 10.1023/A:1020880931488
Baldwin, I. T., Kessler, A., and Halitschke, R. (2002). Volatile signaling in plant–plant–herbivore interactions: what is real? Curr. Opin. Plant Biol. 5, 351–354. doi: 10.1016/s1369-5266(02)00263-7
Bale, J. S., Masters, G. J., Hodkinson, I. D., Awmack, C., Bezemer, T. M., Brown, V. K., et al. (2002). Herbivory in global climate change research: direct effects of rising temperature on insect herbivores. Glob. Change Biol. 8, 1–16. doi: 10.1046/j.1365-2486.2002.00451.x
Bates, D., Maechler, M., Bolker, B., and Walker, S. (2014). lme4: linear mixed-effects models using Eigen and S4. J. Stat. Softw. 1, 1–23.
Benevenuto, R. F., Hegland, S. J., Töpper, J. P., Rydgren, K., Moe, S. R., Rodriguez-Saona, C., et al. (2018). Multiannual effects of induced plant defenses: are defended plants good or bad neighbors? Ecol. Evol. 8, 8940–8950. doi: 10.1002/ece3.4365
Benevenuto, R. F., Seldal, T., Hegland, S. J., Rodriguez-Saona, C., Kawash, J., and Polashock, J. (2019). Transcriptional profiling of methyl jasmonate-induced defense responses in bilberry (Vaccinium myrtillus L.). BMC Plant Biol. 19:70. doi: 10.1186/s12870-019-1650-0
Benevenuto, R. F., Seldal, T., Polashock, J., Moe, S. R., Rodriguez-Saona, C., Gillespie, M. A., et al. (2020). Molecular and ecological plant defense responses along an elevational gradient in a boreal ecosystem. Ecol. Evol. 10, 2478–2491. doi: 10.1002/ece3.6074
Brooker, R. W., Maestre, F. T., Callaway, R. M., Lortie, C. L., Cavieres, L. A., Kunstler, G., et al. (2008). Facilitation in plant communities: the past, the present, and the future. J. Ecol. 96, 18–34. doi: 10.1111/j.1365-2745.2007.01295.x
Bruinsma, M., IJdema, H., van Loon, J. J. A., and Dicke, M. (2008). Differential effects of jasmonic acid treatment of Brassica nigra on the attraction of pollinators, parasitoids, and butterflies. Entomol. Exp. Applicata 128, 109–116.
Calfapietra, C., Wiberley, A. E., Falbel, T. G., Linskey, A. R., Mugnozza, G. S., Karnosky, D. F., et al. (2007). Isoprene synthase expression and protein levels are reduced under elevated O3 but not under elevated CO2 (FACE) in field-grown aspen trees. Plant Cell Environ. 30, 654–661. doi: 10.1111/j.1365-3040.2007.01646.x
Callaway, R. M. (2007). Positive Interactions and Interdependence in Plant Communities. Berlin: Springer.
Catola, S., Centritto, M., Cascone, P., Ranieri, A., Loreto, F., Calamai, L., et al. (2018). Effects of single or combined water deficit and aphid attack on tomato volatile organic compound (VOC) emission and plant-plant communication. Environ. Exp. Bot. 153, 54–62.
Constable, J. V. H., Litvak, M. E., Greenberg, J. P., and Monson, R. K. (1999). Monoterpene emission from coniferous trees in response to elevated CO2 concentration and climate warming. Glob. Change Biol. 5, 255–267.
Copolovici, L., Kännaste, A., Remmel, T., and Niinemets, Ü. (2014). Volatile organic compound emissions from Alnus glutinosa under interacting drought and herbivory stresses. Environ. Exp. Bot. 100, 55–63. doi: 10.1016/j.envexpbot.2013.12.011
Delory, B. M., Delaplace, P., Fauconnier, M.-L., and Du Jardin, P. (2016). Root-emitted volatile organic compounds: can they mediate belowground plant-plant interactions? Plant Soil 402, 1–26.
Dicke, M., and Bruin, J. (2001). Chemical information transfer between plants: back to the future. Biochem. Syst. Ecol. 29, 981–994.
Dicke, M., Gols, R., Ludeking, D., and Posthumus, M. A. (1999). Jasmonic acid and herbivory differentially induce carnivore-attracting plant volatiles in lima bean plants. J. Chem. Ecol. 25, 1907–1922.
Dudareva, N., Pichersky, E., and Gershenzon, J. (2004). Biochemistry of plant volatiles. Plant Physiol. 135, 1893–1902. doi: 10.1104/pp.104.049981
Duhl, T., Helmig, D., and Guenther, A. (2008). Sesquiterpene emissions from vegetation: a review. Biogeosciences 5, 761–777.
Elhakeem, A., Markovic, D., Broberg, A., Anten, N. P., and Ninkovic, V. (2018). Aboveground mechanical stimuli affect belowground plant-plant communication. PLoS One 13:e0195646. doi: 10.1371/journal.pone.0195646
Falik, O., Mordoch, Y., Quansah, L., Fait, A., and Novoplansky, A. (2011). Rumor has it.: relay communication of stress cues in plants. PLoS One 6:e23625. doi: 10.1371/journal.pone.0023625
Flower-Ellis, J. (1971). Age, Structure and Dynamics in Stands of Bilberry (Vaccinium myrtillus L.). Ph.D dissertation, Royal College, Stockholm.
Funk, J. L., Jones, C. G., Gray, D. W., Throop, H. L., Hyatt, L. A., and Lerdau, M. T. (2005). Variation in isoprene emission from Quercus rubra: sources, causes, and consequences for estimating fluxes. J. Geophys. Res. 110:D04301.
Gols, R., Roosjen, M., Dijkman, H., and Dicke, M. (2003). Induction of direct and indirect plant responses by jasmonic acid, low spider mite densities, or a combination of jasmonic acid treatment and spider mite infestation. J. Chem. Ecol. 29, 2651–2666. doi: 10.1023/b:joec.0000008010.40606.b0
Gouinguené, S. P., and Turlings, T. C. (2002). The effects of abiotic factors on induced volatile emissions in corn plants. Plant Physiol. 129, 1296–1307. doi: 10.1104/pp.001941
Guenther, A., Hewitt, C. N., Erickson, D., Fall, R., Geron, C., Graedel, T., et al. (1995). A global-model of natural volatile organic-compound emissions. J. Geophys. Res. Atmos. 100, 8873–8892. doi: 10.1029/94jd02950
Guenther, A. B., Zimmerman, P. R., Harley, P. C., Monson, R. K., and Fall, R. (1993). Isoprene and monoterpene emission rate variability - model evaluations and sensitivity analyses. J. Geophys. Res. Atmos. 98, 12609–12617. doi: 10.1029/93jd00527
Hare, J. D. (2011). Ecological role of volatiles produced by plants in response to damage by herbivorous insects. Annu. Rev. Entomol. 56, 161–180. doi: 10.1146/annurev-ento-120709-144753
Haukioja, E., and Hanhimäki, S. (1985). Rapid wound-induced resistance in white birch (Betula pubescens) foliage to the geometrid Epirrita autumnata: a comparison of trees and moths within and outside the outbreak range of the moth. Oecologia 65, 223–228. doi: 10.1007/BF00379221
Hegland, S. J., Jongejans, E., and Rydgren, K. (2010). Investigating the interaction between ungulate grazing and resource effects on Vaccinium myrtillus populations with integral projection models. Oecologia 163, 695–706. doi: 10.1007/s00442-010-1616-2
Heil, M. (2004). Direct defense or ecological costs: responses of herbivorous beetles to volatiles released by wild Lima bean (Phaseolus lunatus). J. Chem. Ecol. 30, 1289–1295. doi: 10.1023/b:joec.0000030299.59863.69
Heil, M., and Karban, R. (2010). Explaining evolution of plant communication by airborne signals. Trends Ecol. Evol. 25, 137–144. doi: 10.1016/j.tree.2009.09.010
Helmig, D., Ortega, J., Duhl, T., Tanner, D., Guenther, A., Harley, P., et al. (2007). Sesquiterpene emissions from pine trees–identifications, emission rates and flux estimates for the contiguous United States. Environ. Sci. Technol. 41, 1545–1553. doi: 10.1021/es0618907
Himanen, S. J., Nerg, A. M., Nissinen, A., Pinto, D. M., Stewart, C. N. Jr., Poppy, G. M., et al. (2009). Effects of elevated carbon dioxide and ozone on volatile terpenoid emissions and multitrophic communication of transgenic insecticidal oilseed rape (Brassica napus). New Phytol. 181, 174–186. doi: 10.1111/j.1469-8137.2008.02646.x
Hooper, D. U., Chapin, F. S., Ewel, J. J., Hector, A., Inchausti, P., Lavorel, S., et al. (2005). Effects of biodiversity on ecosystem functioning: a consensus of current knowledge. Ecol. Monographs 75, 3–35. doi: 10.1890/04-0922
Howe, G. A., and Jander, G. (2008). Plant immunity to insect herbivores. Annu. Rev. Plant Biol. 59, 41–66. doi: 10.1146/annurev.arplant.59.032607.092825
IPCC, (2018). IPCC report 2018: Global Warming of 1.5°C: An IPCC Special Report on the Impacts of Global Warming of 1.5°C Above Pre-industrial Levels and Related Global Greenhouse Gas Emission Pathways, in the Context of Strengthening the Global Response to the Threat of Climate Change, Sustainable Development, and Efforts to Eradicate Poverty. Geneva: IPCC.
Jassbi, A. R., Zamanizadehnajari, S., and Baldwin, I. T. (2010). Phytotoxic volatiles in the roots and shoots of Artemisia tridentata as detected by headspace solid-phase microextraction and gas chromatographic-mass spectrometry analysis. J. Chem. Ecol. 36, 1398–1407. doi: 10.1007/s10886-010-9885-0
Joëlle, F., Fabien, L., Stéphanie, M., and Jean-Bernard, C. (2010). Nitrogen rhizodeposition of legumes. A review. Agron. Sustain. Dev. 30, 57–66.
Kalske, A., Shiojiri, K., Uesugi, A., Sakata, Y., Morrell, K., and Kessler, A. (2019). Insect herbivory selects for volatile-mediated plant-plant communication. Curr. Biol. 29, 3128–3133. doi: 10.1016/j.cub.2019.08.011
Karban, R., Yang, L. H., and Edwards, K. F. (2014). Volatile communication between plants that affects herbivory: a meta-analysis. Ecol. Lett. 17, 44–52. doi: 10.1111/ele.12205
Keddy, P. A. (1989). Effects of competition from shrubs on herbaceous wetland plants - a 4-year field experiment. Can. J. Bot. Revue Can. Bot. 67, 708–716. doi: 10.1139/b89-094
Kegge, W., Weldegergis, B. T., Soler, R., Eijk, M. V. V., Dicke, M., Voesenek, L. A., et al. (2013). Canopy light cues affect emission of constitutive and methyl jasmonate-induced volatile organic compounds in A rabidopsis thaliana. New Phytol. 200, 861–874. doi: 10.1111/nph.12407
Kesselmeier, J., and Staudt, M. (1999). Biogenic volatile organic compounds (VOC): an overview on emission, physiology and ecology. J. Atmos. Chem. 33, 23–88. doi: 10.1023/A:1006127516791
Kessler, A., and Baldwin, I. T. (2001). Defensive function of herbivore-induced plant volatile emissions in nature. Science 291, 2141–2144. doi: 10.1126/science.291.5511.2141
Knudsen, J. T., Tollsten, L., and Bergström, L. G. J. P. (1993). Floral scents—a checklist of volatile compounds isolated by head-space techniques. Phytochemistry 33, 253–280.
Kobayashi, Y., and Yamamura, N. (2003). Evolution of signal emission by non-infested plants growing near infested plants to avoid future risk. J. Theor. Biol. 223, 489–503. doi: 10.1016/s0022-5193(03)00124-3
Landis, D. A., Wratten, S. D., and Gurr, G. M. (2000). Habitat management to conserve natural enemies of arthropod pests in agriculture. Annu. Rev. Entomol. 45, 175–201. doi: 10.1146/annurev.ento.45.1.175
Liland, K. H., and Saebø, S. (2014). mixlm: Mixed Model ANOVA and Statistics for Education. R package version.
Moen, A., Lillethun, A., and Odland, A. (1999). Vegetation. National atlas of Norway. Hønefoss: Norwegian Mapping Authority.
Moreira, X., Nell, C. S., Katsanis, A., Rasmann, S., and Mooney, K. A. (2018a). Herbivore specificity and the chemical basis of plant–plant communication in B accharis salicifolia (A steraceae). New Phytol. 220, 703–713. doi: 10.1111/nph.14164
Moreira, X., Petry, W. K., Mooney, K. A., Rasmann, S., and Abdala-Roberts, L. (2018b). Elevational gradients in plant defences and insect herbivory: recent advances in the field and prospects for future research. Ecography 41, 1485–1496.
Morley, K., Finch, S., and Collier, R. H. (2005). Companion planting–behaviour of the cabbage root fly on host plants and non-host plants. Entomol. Exp. Appl. 117, 15–25.
Nabity, P. D., Zavala, J. A., and DeLucia, E. H. (2013). Herbivore induction of jasmonic acid and chemical defences reduce photosynthesis in Nicotiana attenuata. J. Exp. Bot. 64, 685–694. doi: 10.1093/jxb/ers364
NGU, (2019). Norges Geologiske Undersøkelse. Sogndal, SOGN OG FJORDANE. Available at: http://geo.ngu.no/kart/minkommune/?kommunenr=1420 (accessed February 21, 2020).
Niinemets, U., Loreto, F., and Reichstein, M. (2004). Physiological and physicochemical controls on foliar volatile organic compound emissions. Trends Plant Sci. 9, 180–186. doi: 10.1016/j.tplants.2004.02.006
Pellegrini, E., Cioni, P. L., Francini, A., Lorenzini, G., Nali, C., and Flamini, G. (2012). Volatiles emission patterns in poplar clones varying in response to ozone. J. Chem. Ecol. 38, 924–932. doi: 10.1007/s10886-012-0162-2
Penuelas, J., and Llusia, J. (2001). The complexity of factors driving volatile organic compound emissions by plants. Biol. Plant. 44, 481–487. doi: 10.1023/A:1013797129428
Penuelas, J., and Llusia, J. (2003). BVOCs: plant defense against climate warming? Trends Plant Sci. 8, 105–109. doi: 10.1016/S1360-1385(03)00008-6
Penuelas, J., and Staudt, M. (2010). BVOCs and global change. Trends Plant Sci. 15, 133–144. doi: 10.1016/j.tplants.2009.12.005
Peterson, G., Allen, C. R., and Holling, C. S. (1998). Ecological resilience, biodiversity, and scale. Ecosystems 1, 6–18. doi: 10.1007/s100219900002
Pezzola, E., Mancuso, S., and Karban, R. (2017). Precipitation affects plant communication and defense. Ecology 98, 1693–1699. doi: 10.1002/ecy.1846
Pickett, J., Rasmussen, H., Woodcock, C., Matthes, M., and Napier, J. (2003). Plant Stress Signalling: Understanding and Exploiting Plant–Plant Interactions. London: Portland Press Limited.
Pinto, D. M., Blande, J. D., Nykänen, R., Dong, W.-X., Nerg, A.-M., and Holopainen, J. K. (2007). Ozone degrades common herbivore-induced plant volatiles: does this affect herbivore prey location by predators and parasitoids? J. Chem. Ecol. 33, 683–694. doi: 10.1007/s10886-007-9255-8
Plath, M., Mody, K., Potvin, C., and Dorn, S. J. A. S. (2011). Do multipurpose companion trees affect high value timber trees in a silvopastoral plantation system? Agroforestry Syst. 81, 79–92.
Post, E., Forchhammer, M. C., Bret-Harte, M. S., Callaghan, T. V., Christensen, T. R., Elberling, B., et al. (2009). Ecological dynamics across the Arctic associated with recent climate change. Science 325, 1355–1358. doi: 10.1126/science.1173113
R Development Core Team, (2012). TEAM 2009: R: A Language and Environment for Statistical Computing. Vienna: R Foundation for Statistical Computing.
Rasmann, S., Hiltpold, I., and Ali, J. (2012). “The role of root-produced volatile secondary metabolites in mediating soil interactions,” in Advances in Selected Plant Physiology Aspects, eds G. Montanaro, and D. Bartolomeo, (Rijeka: InTech).
Redman, A. M., Cipollini, D. F. Jr., and Schultz, J. C. (2001). Fitness costs of jasmonic acid-induced defense in tomato, Lycopersicon esculentum. Oecologia 126, 380–385. doi: 10.1007/s004420000522
Rodriguez-Saona, C., Polashock, J., and Malo, E. (2013). Jasmonate-mediated induced volatiles in the American cranberry, Vaccinium macrocarpon: from gene expression to organismal interactions. Front. Plant Sci. 4:115. doi: 10.3389/fpls.2013.00115
Rodriguez-Saona, C. R., Rodriguez-Saona, L. E., and Frost, C. J. (2009). Herbivore-induced volatiles in the perennial shrub, Vaccinium corymbosum, and their role in inter-branch signaling. J. Chem. Ecol. 35, 163–175. doi: 10.1007/s10886-008-9579-z
Rosenstiel, T. N., Potosnak, M. J., Griffin, K. L., Fall, R., and Monson, R. K. (2003). Increased CO 2 uncouples growth from isoprene emission in an agriforest ecosystem. Nature 421:256. doi: 10.1038/nature01312
Sabelis, M. (1999). “Behavioral responses of predatory and herbivorous arthropods to induced plant volatiles: from evolutionary ecology to agricultural applications” in Induced Plant Defenses Against Pathogens Herbivores, eds A. A. Agrawal, S. Tuzun, and E. Bent, (St. Paul, MN: APS Press) 269–296.
Sala, O. E., Chapin, F. S. III, Armesto, J. J., Berlow, E., Bloomfield, J., Dirzo, R., et al. (2000). Global biodiversity scenarios for the year 2100. Science 287, 1770–1774. doi: 10.1126/science.287.5459.1770
Seldal, T., Hegland, S. J., Rydgren, K., Rodriguez-Saona, C., and Töpper, J. P. (2017). How to induce defense responses in wild plant populations? Using bilberry (Vaccinium myrtillus) as example. Ecol. Evol. 7, 1762–1769. doi: 10.1002/ece3.2687
Shiojiri, K., Kishimoto, K., Ozawa, R., Kugimiya, S., Urashimo, S., Arimura, G., et al. (2006). Changing green leaf volatile biosynthesis in plants: an approach for improving plant resistance against both herbivores and pathogens. Proc. Natl. Acad. Sci. U.S.A. 103, 16672–16676. doi: 10.1073/pnas.0607780103
Steeghs, M., Bais, H. P., de Gouw, J., Goldan, P., Kuster, W., Northway, M., et al. (2004). Proton-transfer-reaction mass spectrometry as a new tool for real time analysis of root-secreted volatile organic compounds in arabidopsis. Plant Physiol. 135, 47–58. doi: 10.1104/pp.104.038703
Stenseth, N. C., and Mysterud, A. (2002). Climate, changing phenology, and other life history traits: nonlinearity and match–mismatch to the environment. Proc. Natl. Acad. Sci. U.S.A. 99, 13379–13381. doi: 10.1073/pnas.212519399
Tamogami, S., Rakwal, R., and Agrawal, G. K. (2008). Interplant communication: airborne methyl jasmonate is essentially converted into JA and JA-Ile activating jasmonate signaling pathway and VOCs emission. Biochem. Biophys. Res. Commun. 376, 723–727. doi: 10.1016/j.bbrc.2008.09.069
Thaler, J. S., Stout, M. J., Karban, R., and Duffey, S. S. (2001). Jasmonate-mediated induced plant resistance affects a community of herbivores. Ecol. Entomol. 26, 312–324. doi: 10.1023/a:1016225515936
Thomas, C. D., Cameron, A., Green, R. E., Bakkenes, M., Beaumont, L. J., Collingham, Y. C., et al. (2004). Extinction risk from climate change. Nature 427, 145–148. doi: 10.1038/nature02121
Tingey, D., Turner, D., and Weber, J. (1991). “Factors controlling the emissions of monoterpenes and other volatile organic com- pounds,” in Trace Gas Emissions by Plants. eds T. Sharkey, E. Holland and H. Mooney (New York: Academic Press), 93–120.
Valkama, E., Koricheva, J., and Oksanen, E. (2007). Effects of elevated O3, alone and in combination with elevated CO2, on tree leaf chemistry and insect herbivore performance: a meta-analysis. Glob. Change Biol. 13, 184–201.
Visser, M. E., and Both, C. (2005). Shifts in phenology due to global climate change: the need for a yardstick. Proc. R. Soc. B Biol. Sci. 272, 2561–2569. doi: 10.1098/rspb.2005.3356
Yang, S., Wu, H., Xie, J., and Rantala, M. J. (2013). Depressed performance and detoxification enzyme activities of Helicoverpa armigera fed with conventional cotton foliage subjected to methyl jasmonate exposure. Entomol. Exp. Applicata 147, 186–195.
Keywords: induced defenses, methyl jasmonate, neighborhood effects, plant–plant communication, Vaccinium myrtillus
Citation: Benevenuto RF, Seldal T, Moe SR, Rodriguez-Saona C and Hegland SJ (2020) Neighborhood Effects of Herbivore-Induced Plant Resistance Vary Along an Elevational Gradient. Front. Ecol. Evol. 8:117. doi: 10.3389/fevo.2020.00117
Received: 27 January 2020; Accepted: 14 April 2020;
Published: 08 May 2020.
Edited by:
Maria L. Pappas, Democritus University of Thrace, GreeceReviewed by:
Paula Baptista, Polytechnic Institute of Bragança, PortugalRocio Escobar Bravo, University of Bern, Switzerland
Copyright © 2020 Benevenuto, Seldal, Moe, Rodriguez-Saona and Hegland. This is an open-access article distributed under the terms of the Creative Commons Attribution License (CC BY). The use, distribution or reproduction in other forums is permitted, provided the original author(s) and the copyright owner(s) are credited and that the original publication in this journal is cited, in accordance with accepted academic practice. No use, distribution or reproduction is permitted which does not comply with these terms.
*Correspondence: Rafael Fonseca Benevenuto, cmZiZW5ldmVudXRvQGdtYWlsLmNvbQ==