- 1School of BioSciences, The University of Melbourne, Melbourne, VIC, Australia
- 2School of Life Sciences, La Trobe University, Melbourne, VIC, Australia
- 3Department of Behavioral Neurobiology, Max Planck Institute for Ornithology, Seewiesen, Germany
- 4Institute of Neuroinformatics, University of Zurich and ETH Zurich, Zurich, Switzerland
Artificial light at night could have widespread and detrimental impacts on sleep. To reduce disruptive effects of artificial light on sleep in humans, most smartphones and computers now have software that reduces blue light emissions at night. Little is known about whether reducing blue light emissions from city lights could also benefit urban wildlife. We investigated the effects of blue-rich (white) and blue-reduced (amber) LED streetlights on accelerometry-defined rest, electrophysiologically-identified sleep, and plasma melatonin in a diurnal bird, the black swan (Cygnus atratus). Urban swans were exposed to 20 full nights of each lighting type in an outdoor, naturalistic environment. Contrary to our predictions, we found that night-time rest was similar during exposure to amber and white lights but decreased under amber lights compared with dark conditions. By recording brain activity in a subset of swans, we also demonstrated that resting birds were almost always asleep, so amber light also reduced sleep at night. We found no effect of light treatment on total (24 h) daily rest or plasma melatonin. Our study provides the first electrophysiologically-verified evidence for effects of streetlights on sleep in an urban animal, and furthermore suggests that reducing blue wavelengths of light might not mitigate these effects.
Introduction
Over the past 150 years, the proliferation of artificial light at night has become a growing global issue (Hölker et al., 2010; Bennie et al., 2015; Falchi et al., 2016). By disrupting natural light cycles, artificial light can disrupt daily rhythms of behavior and physiology in humans and wildlife (Stevens and Zhu, 2015; Dominoni et al., 2016). Effects on behavior are particularly well-documented for diurnal birds, which often show earlier onset of daily activity (Kempenaers et al., 2010; Dominoni et al., 2013b; Da Silva et al., 2014; de Jong et al., 2017; Spoelstra et al., 2018; Ulgezen et al., 2019) and increased night-time activity (Dominoni et al., 2013a; de Jong et al., 2016, 2017; Ouyang et al., 2017; Alaasam et al., 2018; Ulgezen et al., 2019) when exposed to light at night. These behavioral changes may arise as a direct result of artificial light enhancing the visual environment, leading diurnal animals to forage more at night (Russ et al., 2014) or become more vigilant (Yorzinski et al., 2015). Alternatively, increased night-time activity could be driven by physiological impacts of light at night, including the suppression of melatonin – a hormone that drives endogenous day-night (circadian) rhythms (Dominoni et al., 2013a, b) and promotes sleep in diurnal animals (Reiter et al., 2010; Gandhi et al., 2015). While increased nocturnal activity under artificial light has been linked with indicators of poorer health (Ouyang et al., 2017; Alaasam et al., 2018), the extent of associated costs remains largely unknown. However, if increased night-time activity reflects decreased sleep (Raap et al., 2015; Yorzinski et al., 2015; Ouyang et al., 2017), then there could be negative consequences for development, memory, learning, health, and fitness (Aulsebrook et al., 2016; Aulsebrook et al., 2018).
One approach to mitigate any negative effects of artificial light is to alter the color of lighting. Amber lights with lower emissions of short, blue wavelengths are broadly predicted to have less impact on wildlife than blue-rich, white lights (Longcore et al., 2018). A putative mechanism underpinning this rationale is that blue wavelengths of light (around 460 to 480 nm) have the most suppressive effect on the production of melatonin (Reiter et al., 2010; Dominoni, 2015). This effect is highly conserved across taxa (Pandi-Perumal et al., 2006; Jones et al., 2015) and may explain many of the disruptive impacts of artificial light on sleep (Aulsebrook et al., 2018). Accordingly, there is evidence that reducing exposure to blue wavelengths in the evening can improve sleep duration and quality in humans (Santhi et al., 2012; Ayaki et al., 2016). By implication, this strategy could also reduce impacts on night-time rest and sleep in diurnal wildlife.
To test this, we compared the effects of two types of streetlights on the behavior and physiology of an urban bird, the black swan (Cygnus atratus). The urban population of swans at Albert Park Lake (37°50′S, 144°58′E; Melbourne, VIC, Australia) has been well-studied for the past decade (Guay and Mulder, 2009). Swans in this population are exposed to light at night from streetlights, vehicles, buildings, sporting events, and sky glow. Swans are large and are re-captured relatively easily, making them ideal subjects for research involving repeated deployment of data loggers to record behavior, as well as repeated blood sampling. Although swans are mostly diurnal, they exhibit some flexibility in the timing of their behavior. For example, swans will fly long distances between water bodies at night (Frith, 1982). During breeding, males and females also show marked role division, with males typically incubating eggs during the day (while females presumably forage) and switching roles at night (unpublished data). Such flexibility in the timing of activity could allow swans to shift the timing of their sleep in response to light at night, rather than losing sleep overall. Furthermore, if artificial light suppresses sleep in birds with such flexible activity patterns, we would predict that other, less flexible, urban birds are similarly, if not more, affected.
We experimentally tested whether unfiltered white (blue-rich) and filtered amber (blue-reduced) light emitting diode (LED) streetlights would reduce rest, sleep, and circulating melatonin concentrations in black swans. The effects of ecologically-realistic artificial lighting on sleep are seldom tested; most studies have been on captive or laboratory animals, with relatively few exceptions (Aulsebrook et al., 2018). Although animals exposed to lights inside a cage or nest box show reduced sleep behavior and night-time rest (Raap et al., 2015; Yorzinski et al., 2015; de Jong et al., 2016; Raap et al., 2016; de Jong et al., 2017; Alaasam et al., 2018), it is unclear whether, in real-world, heterogeneously-lit environments, animals would inhabit such intensely-lit spaces in the first place (Yorzinski et al., 2015; Aulsebrook et al., 2018; Raap et al., 2018; Caorsi et al., 2019). To address these gaps in the literature, we conducted our study in a large, naturalistic, heterogeneously-lit outdoor environment, where birds could limit their exposure to artificial lights by moving to less-lit areas at night.
An additional challenge for previous studies has been measuring sleep in naturalistic contexts (Aulsebrook et al., 2016). Sleep is best quantified by measuring brain activity with the electroencephalogram (EEG). Analysis of the EEG reveals two sleep states in birds and mammals called non-rapid eye movement (non-REM) sleep and REM sleep (Lesku and Rattenborg, 2014). Although advances in technology have facilitated EEG recordings in the wild (Aulsebrook et al., 2016; Rattenborg et al., 2017), studies outside the laboratory remain challenging. We therefore examined whether resting birds were indeed sleeping, or simply sitting quietly awake, by recording the EEG in a subset of swans (see also Lesku et al., 2012).
Materials and Methods
Animals and Housing
We captured wild adult black swans (six males, five females) and transported them to Serendip Sanctuary, Lara, Australia (38°0′S, 144°24′E; Figure 1A). Swan sex was determined from standard genetic techniques and/or known morphological differences (see Supplementary Methods). Nine swans (two pairs, three single males, and two single females) were caught at Albert Park Lake (37°50′S, 144°58′E), and two swans (one pair) were from Altona Beach, Victoria (37°52′S, 144°59′E). Both sites are exposed to light pollution from streetlights, buildings, vehicles, and skyglow. All swans were individually identifiable from metal leg bands (Australian Bird and Bat Banding Scheme) and plastic neck collars as part of an ongoing research project (Guay and Mulder, 2009; Mulder et al., 2010). Breeding and pairing data for most swans were available from monthly censuses conducted at Albert Park Lake. At capture, no swan had a nest, cygnets, or dependent offspring.
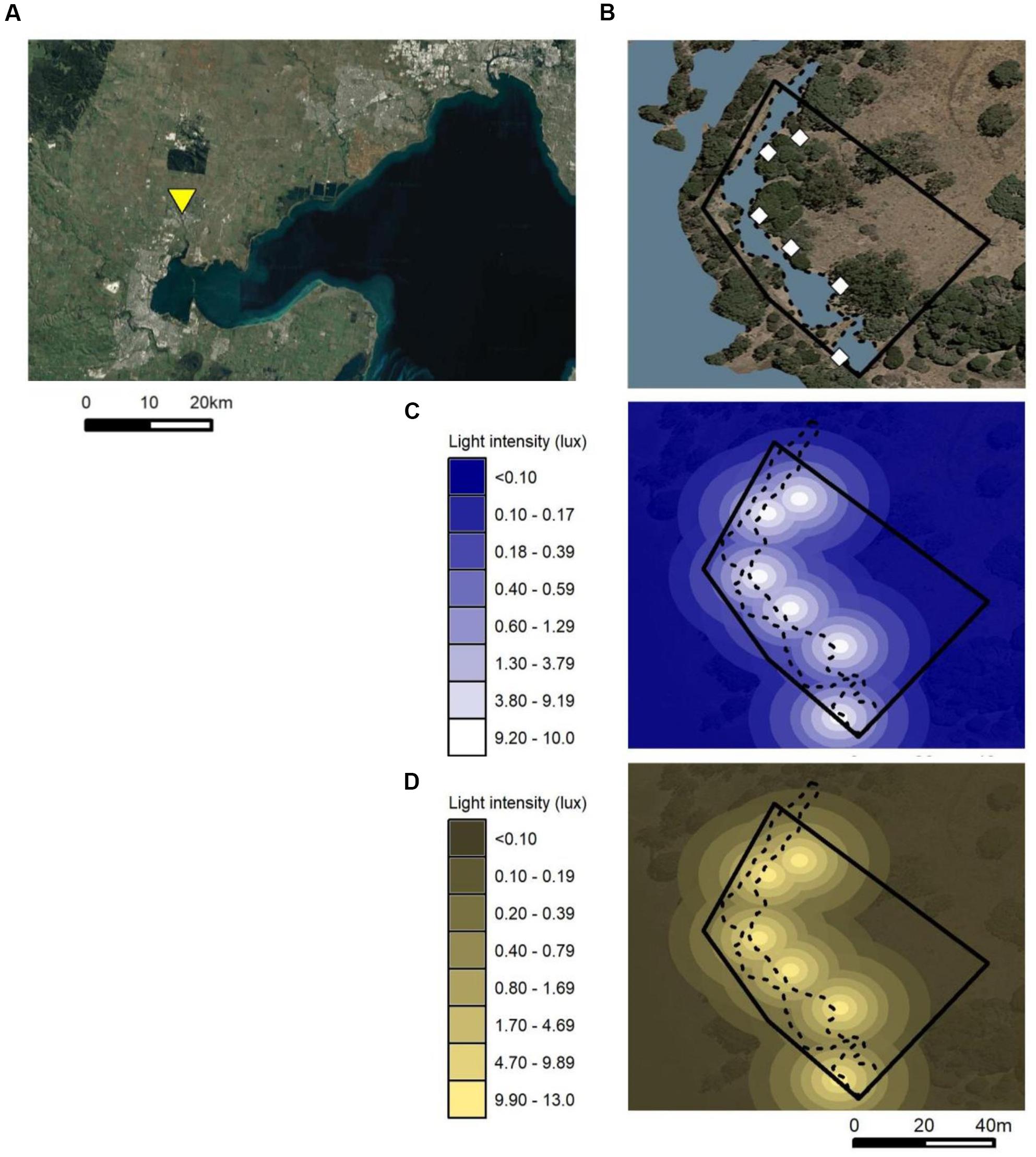
Figure 1. Maps of swan enclosure. (A) Map showing location of swan enclosure at Serendip Sanctuary, Lara (38°0′S, 144°24′E; yellow triangle) to the south-west of Melbourne (at the northern end of Port Phillip Bay); (B) satellite image showing vegetation, ponds (blue), outer fence (solid black line), and positions of streetlights (white diamonds); (C) light intensity with distance from light poles during the white light treatment, and (D) during the amber light treatment. Light intensity values do not account for shielding provided by vegetation. Light intensity was measured at ground level facing the light pole using an SKL 30046473 lux meter with sensor SKL 310L 46472 (Skye Instruments, Llandrindod Wells, United Kingdom) on a single night. When facing away from the light, light intensity was similar to moonlight (<0.05 lux) 11 m away from the light pole. Maps were generated using Google Earth and ggmap (Kahle and Wickham, 2013).
At Serendip Sanctuary, all swans were housed together in a large (4,600 m2) naturalistic outdoor enclosure containing native terrestrial vegetation and a 1,470 m2 pond, surrounded by a fox-proof fence (Figure 1B). Swans were fed poultry crumble (Barastoc Pullet Grower and Chick Starter, Ridley Corporation, Melbourne, Australia) and fresh lettuce daily to supplement foods (grasses) naturally available within the enclosure. To prevent escape, we trimmed the first seven primary feathers of each swan’s wings; this allowed for take-off and gliding, but only at low heights. We captured swans fortnightly to ensure their weight was within a healthy range, check for any regrowth of feathers, and trim feathers when necessary. For all experimental procedures, swans were recaptured by herding them into a run that led to a small pen (~6 × 5 m), where they could be caught by hand.
All data were collected between November 2017 and March 2018 (spring and summer). Swans were given at least six weeks to acclimate to the enclosure before collecting data or implanting electrodes (see sleep recordings). During the acclimation period, swans experienced only minimal light at night (<0.01 lux during new moon; see “Environmental measurements”). After the study concluded, the swans were returned to Albert Park Lake.
Environmental Measurements
Local times for sunrise, sunset, astronomical dawn, astronomical dusk, and lunar phase were obtained from Geoscience Australia1. Wet bulb temperature data (recorded as an average per minute) were obtained from the Avalon Airport weather station (station reference: 087113; 38°1′S, 144°28′E; Bureau of Meteorology, Australia), ∼8 km from the swan enclosure. Light intensity within the swan enclosure was recorded each minute throughout the study using custom-made light loggers (University of Konstanz, Konstanz, Germany; Dominoni et al., 2014; Table B1), each calibrated against a lux meter (SKL 30046473 with sensor SKL 310L 46472, Skye Instruments, Llandrindod Wells, United Kingdom) during evening twilight. We chose to measure light in lux, despite this measure being based on human vision, since human perspectives regarding illumination are commonplace for lighting design and urban planning (Gaston et al., 2013). However, it is important to recognize that these units do not necessarily represent what would be perceived by the birds.
Light Treatments
Artificial light at night was manipulated by installing six LED streetlights (IP66 LEDway® SLMTM Streetlight 4,000 K, Ruud Lighting, Racine, WI, United States) mounted on steel poles (3 × 3 × 196 cm; see Supplementary Methods). We investigated the effects of two types of streetlighting: white (3,700 K) and amber (2,100 K). The amber lighting was similar in intensity to the white lighting (Supplementary Table S1) but was filtered to shift its spectral output toward longer, red wavelengths, almost eliminating emission of blue wavelengths (Supplementary Figure S1). Lights were positioned to mimic lighting conditions at Albert Park Lake and produced environmentally-realistic light intensities (0.1 – 10 lux around the pond, 10 lux directly underneath the light; Figures 1C,D). Light intensities within this range, and even lower, have previously been shown to have a detectable effect on bird behavior and physiology (e.g., Dominoni et al., 2013a; Alaasam et al., 2018; also see van Hasselt et al., 2020).
To investigate short-term effects of streetlights on sleep, we exposed swans to white light for one night in December 2017. To describe longer-term effects on time spent resting, circulating melatonin, and subsequent daytime behavior, we exposed the swans to at least 20 consecutive nights of white light and 20 consecutive nights of amber light. As a control, lights were switched off for 18 nights before (“first dark control”) and nine nights between (“second dark control”) the artificial light treatments. The durations of the dark controls were determined by lunar phase, so that blood samples for melatonin analysis could be collected at similar lunar illumination during light treatments (see melatonin analysis). Light intensity was higher during the first dark control than during the second owing to differences in lunar phase (Supplementary Table S1). Due to a technical malfunction, lights were switched off for part of Night 20 of the white light treatment. As the final blood samples for this light treatment had not yet been collected, the white light treatment was extended a further 13 nights (total duration: 33 nights). For consistency we only used data from the first 20 nights of each light treatment for our analysis of sleep and rest.
To observe directly how swans responded to the streetlights, one observer (A.E.A.) watched the swans from outside the enclosure when the lights first switched on. The birds were not visibly disturbed by either the white or amber lighting. The same observer also watched the swans from a bird hide during a night of white light exposure, after all experimental data had been collected. The birds did not appear to avoid the light and were mostly observed along the banks of the pond, <6 m from the nearest streetlight, which is where they were also frequently observed during the day. Some swans were even observed foraging, preening and resting almost directly beneath streetlights. We have seen a similar lack of overt responses to streetlights in wild swans. Many swans rested close to each other, although some did move during the night, and swans also rested in close proximity (<1 m) to swans that were simultaneously active (e.g., preening).
Recording and Analyzing Resting Behavior
Activity from all swans was recorded near-continuously throughout the study using tri-axial accelerometer data loggers (Axivity AX3, Open Movement, Newcastle, United Kingdom). Using the raw accelerometry data, we calculated average overall dynamic body acceleration (ODBA; Wilson et al., 2006) for 4 s time bins. ODBA is a common measure of animal activity and allowed us to classify animals as resting or active (see Supplementary Methods). To define resting and active behaviors, we also observed swans and recorded time spent on various behaviors, including preening and foraging; these data were used to test whether light at night affected subsequent daytime behavior.
Recording and Analyzing Sleep
To record the electroencephalogram (EEG) and electromyogram (EMG), a subset of six swans (three females, three males) were surgically implanted with electrodes to measure brain waves and neck muscle tone, using standard stereotaxic techniques (Lesku et al., 2011a, b; see Supplementary Methods). Swans were given at least 2 weeks of post-operative recovery before commencing data collection. Data were recorded using a miniature data logger (Neurologger 2A, www.vyssotski.ch/neurologger2; Vyssotski et al., 2009; Anisimov et al., 2014), which had an inbuilt tri-axial accelerometer for capturing head movements.
Ultimately, due to logistic challenges, we obtained sleep data from two swans. Many recordings failed owing to water damage, or detachment of the logger or implant. Electrophysiological and accelerometry signals were imported into RemLogic v. 3.4.4 (Embla Systems, Pleasanton, CA, United States) for scoring and analysis. Data were manually scored by a single scorer (A.E.A.) from 600 h the day after the data logger was deployed (≥20 h post-handling, near sunrise) until 48 h later. Data were visually classified in 4 s epochs (time bins) as wakefulness, non-REM, or REM sleep following Martinez-Gonzalez et al.(2008; see Supplementary Methods).
Blood Collection and Melatonin Analysis
To assess daily variation in circulating melatonin under dark control conditions, we collected blood samples from each swan around sunset (–11 to 39 min relative to sunset), sunrise (15 to 56 min before sunrise), midday (5.2 to 6.1 h after sunrise), and night (6.2 to 7.3 h after sunset; 3.4 to 4.5 h before sunrise). These blood samples were collected after at least three nights without artificial light, and 4–8 nights from a new moon. We collected blood samples at least 24 h apart to minimize potential stress associated with repeated handling. All activity and sleep data collected <20 h after handling the birds were excluded from analyses.
To quantify any effects of light at night on plasma melatonin concentrations, we collected blood samples from all swans on six nights: two nights before each light treatment (during the first and second dark controls), on Night 2 of each light treatment (white light and amber night), and on the final night of each light treatment (Night 33 of white light, Night 20 of amber light). Blood samples were collected around 6 h after sunset (mean ± SE; first blood sample: 6.0 ± 0.1 h after sunset; final sample 7.0 ± 0.1 h after sunset), which was 4 h before sunrise (first sample: 4.4 ± 0.2 h before sunrise; final sample: 3.3 ± 0.3 h before sunrise), when circulating melatonin concentrations were expected to be highest (Zawilska et al., 2002, 2003). All blood samples were collected 4–9 nights from a new moon. Capture, handling, and bleeding of birds, as well as blood processing, was conducted under dim red light from head torches (see Supplementary Methods). Time between herding swans into the pen and collecting a blood sample from each swan ranged from 5 to 82 min.
Plasma concentrations of melatonin were determined by direct radioimmunoassay after extraction (Goymann et al., 2008). A four-parameter logistic curve was derived using Immunofit 3.0 (Beckman, Inc., Fullerton, CA, United States) to calculate sample concentration. Samples were run in two separate assays, and the lower detection limit was determined as the first value outside the 95% confidence intervals for the zero standard (Bmax): 0.80 and 0.86 pg/mL, respectively. The intra-assay co-efficients of variation (CV) of melatonin standards were 5.94 and 3.60%. The intra-extraction CVs of plasma pool samples ran in both assays were 5.07 and 0.44%. The inter-assay (standard melatonin) and inter-extraction CVs were 1.5 and 10.1%, respectively. Melatonin concentrations were adjusted for individual extraction recoveries (mean ± SD, 91.7 ± 5.02%).
Statistical Analysis
We performed statistical analyses using R version 3.5.1 (R Development Core Team, 2018). We used linear mixed effects models (LMM) to investigate effects of light at night on the duration of rest (based on accelerometry), circulating melatonin concentrations, and daytime behavior (based on behavioral observations) in swans. To determine the relationship between sleep and rest, we used a linear model that accounted for the temporal structure of the data. These analyses are described in further detail below.
LMM were fitted using the package lme4 and p-values were calculated using the package lmerTest (Bates et al., 2015; Kuznetsova et al., 2017). We performed visual inspection of model residuals to confirm that linear model assumptions were met. The outcome of each model was also compared to a robust variant of the same model using the package robustlmm (Koller, 2016; Field and Wilcox, 2017). In all cases, outcomes from conventional and robust models were very similar; for ease of interpretation, we have chosen to report results from conventional models. Where a variable of interest had a significant effect (p < 0.05), post hoc tests were run using the function emmeans with a Tukey adjustment for multiple tests (Lenth, 2018). For analyses of rest, “night-time rest” was defined as the time spent resting between astronomical dusk and astronomical dawn (astronomical night). To account for changes in daylength (the duration of astronomical nights ranged from 5.3 to 8.1 h; Supplementary Table S2), the latest astronomical dusk and earliest astronomical dawn times were used for analyses involving multiple nights.
For LMM investigating effects on rest, dependent variables were individual data from swans on each night/date. Swan identity was included as a random factor to account for the repeated measures design. Date was also included as a random factor, since data collected from the same day cannot be treated as independent. A preliminary analysis indicated no difference in night-time rest duration between the first and second dark controls (mean ± SE; first dark control: 3.35 ± 0.10 h, second dark control: 3.52 ± 0.09 h; LMM: t18 = 0.48, p = 0.64); we therefore pooled these data for analysis. We first tested for effects of lunar phase and mean night-time temperature on night-time rest during the dark control (Table 1). Lunar phase was defined as the absolute number of days from full moon (included in the model as a fixed numeric variable). We also included sex as a categorical variable in this model and subsequent models, to assess whether rest differed between males and females. We then investigated the effects of artificial light at night on night-time rest and total rest each 24 h day (with the 24 h day commencing at 1,900, around the time the lights were switched on; Table 1; also see Supplementary Methods). Initial models included an interaction term for sex and light treatment that was excluded if non-significant. Based on preliminary analyses that found an effect of light treatment on night-time, but not total daily rest, we made the post hoc decision to explore effects of light at night on dawn rest (rest during the hour preceding sunrise) and dusk rest (rest during the hour after sunset; Table 1). This decision was based on visual inspection of the data, which suggested that these were the only times of day when rest might have increased during light treatments. We also explored whether swans habituated to artificial light by examining whether night-time rest changed over time within light treatments, with mean night-time temperature (range: 9.6 – 21.5°C) included as a covariate (see Supplementary Methods).
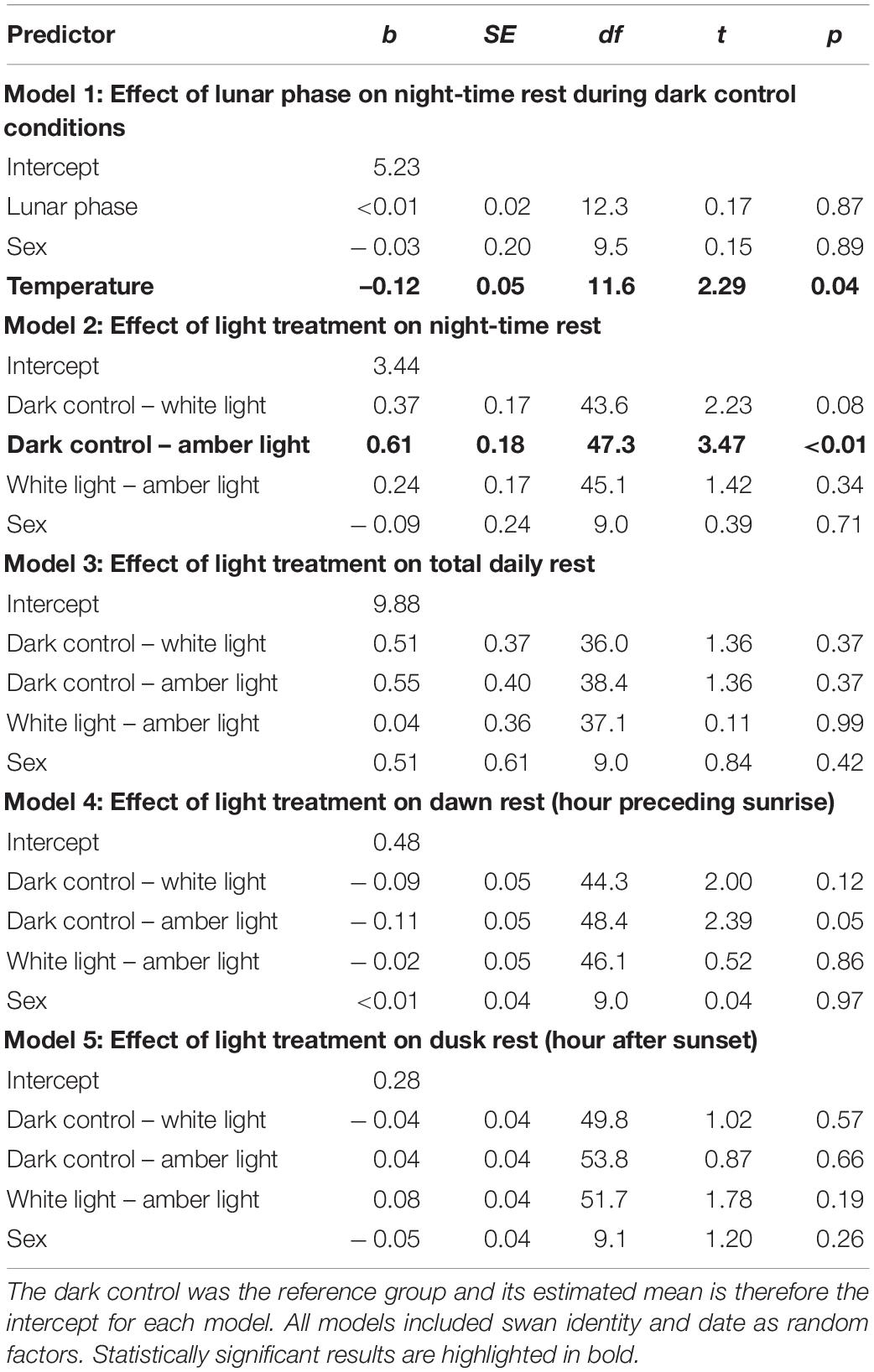
Table 1. Results from linear mixed-effects models for impacts of light at night on time spent resting in black swans (b,unstandardized regression co-efficient; SE, standard error of regression co-efficient; df, degrees of freedom; t, t-ratio; p, p-value).
For analysis of melatonin data, we tested whether circulating melatonin varied with time of day or light treatment in two separate models, which included time between capture (defined as when the swan was herded into the smaller pen) and bleeding as a fixed covariate. These models also included sex as a fixed categorical variable and swan identity as a random factor. We excluded two samples with much smaller volumes (<45 μL) from the light treatment model (one from the amber light treatment, another from dark control) and one from the time of day model (collected at midday).
To test for effects of light treatment on subsequent daytime behavior, dependent variables were the proportion of time spent resting, preening, and foraging, with observation duration (fixed covariate), sex (fixed categorical variable), and swan identity (random factor) included in each model (also see Supplementary Methods).
To investigate whether time spent resting is a reliable proxy for time spent sleeping, we modeled the relationship between these two variables with a linear regression model (LM) that accounted for the temporal structure of the data, using the function gls in the package nlme. This model incorporated a first-order autoregressive process, with time spent resting (per hour) as the dependent variable and time spent sleeping (per hour) as the independent variable. For ease of interpretation, we have also presented correlation co-efficients obtained using Pearson’s product-moment correlation.
Results
Rest and Behavior
Night-Time Rest
We found a strong effect of light treatment on night-time rest [F(2,45) = 6.14, p < 0.01; Figure 2 and Table 1]. Birds rested 40 min (19.5%) less during amber nights compared with dark control nights (mean night-time rest during amber light ± SE: 2.76 ± 0.07 h; dark control: 3.43 ± 0.07 h; t47 = 3.47, p < 0.01) and 25 min (12.0%) less during white nights than dark control nights (white light: 3.02 ± 0.06 h; t43 = 2.23, p = 0.08). Night-time rest did not differ between the two artificial light treatments (t44 = 1.42, p = 0.34). During dark control conditions, the amount of night-time rest was not related to lunar phase (t12 = 0.17, p = 0.87). Although warmer nights during dark control were associated with less night-time rest (t12 = 2.29, p = 0.04), this effect did not persist during white (t172 = 0.20, p = 0.84) or amber (t99 = 0.78, p = 0.44) light treatments. Night-time rest was comparable for males and females across all light treatments (Table 1). In our model comparing night-time rest across light treatments, we found substantial variance in night-time rest within individual swans, with 49.7% of total variance attributed to differences within individuals. There was less variance in night-time rest between swans, with 22.0% of total variance attributed to differences between individuals (with the remaining 28.4% variance attributed to differences between dates). We found no evidence to suggest that swans habituated to the presence of light at night during our study timeframe (Supplementary Figure S2).
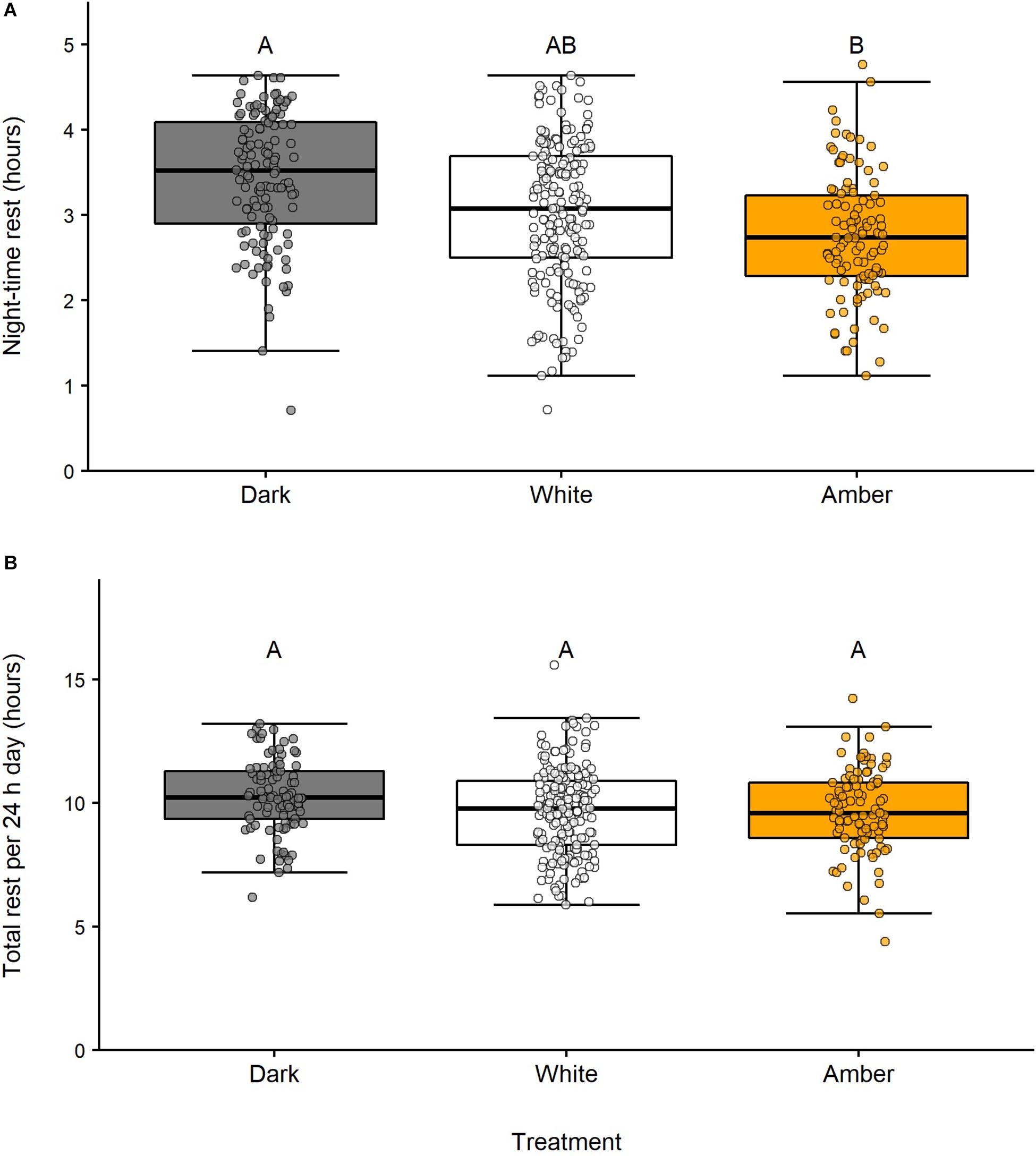
Figure 2. Effects of streetlighting at night on (A) time swans spent resting during astronomical night and (B) total rest over 24 h days. Different letters denote significant differences between treatments; treatments with no letters in common were significantly different to each other (p < 0.05). Boxplots are Tukey boxplots showing median values (horizontal lines), interquartile ranges (IQR; boxes), and ranges excluding outliers (whiskers; outliers defined as values more than 1.5 × IQR beyond box edges). Individual points represent data for each swan (n = 11) on each day/night, including outliers (total observations: dark a = 123, dark b = 93, white a = 185, white b = 185, amber a = 109, amber b = 96). Differences in the numbers of observations were due to exclusion of data after handling swans, loss of data loggers, and temporary removal of data loggers from individuals due to abrasion of feathers.
Daily Rest
Despite disrupted night-time rest, we found no effect of light treatment on total rest across the 24 h day [F(2,37) = 1.18, p = 0.32; Figure 2 and Table 1]. The amount of daily rest was highly variable (range: 4.4 to 15.6 h) but averaged ~10 h per 24 h day (dark control: 10.20 ± 0.15 h; white light: 9.66 ± 0.13 h; amber light: 9.66 ± 0.17 h). Our model indicated that 32.9% of total variance could be attributed to differences between birds and 41.0% to differences within individuals (the remaining 26.1% variance was attributed to differences between dates). The only time of day when the amount of rest appeared greater during amber or white light, compared with the dark control, was in the hour preceding sunrise (dawn rest; Figure 3). This difference was small (<10 min), with swans tending to rest slightly more at dawn under amber light (0.60 ± 0.02 h; t50 = 2.39, p = 0.05), but not white light (0.57 ± 0.02 h; t46 = 2.00, p = 0.12) compared to the dark control (0.47 ± 0.02 h). There was no difference in dawn rest between the amber and white light treatments (t47 = 0.52, p = 0.86), and no effect of light treatment on rest during the hour after sunset [dusk rest: F(2,52) = 1.61, p = 0.21].
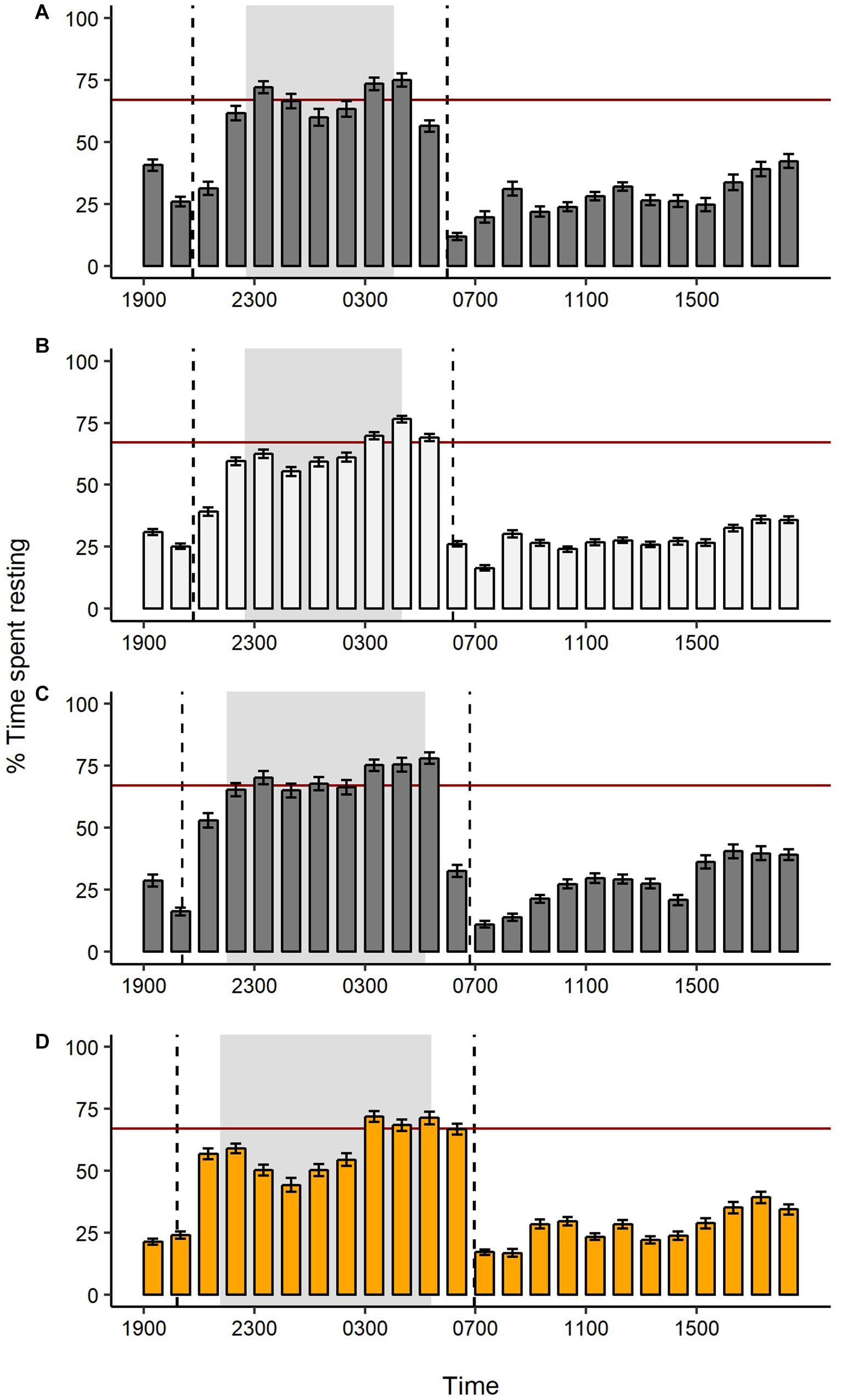
Figure 3. Percentage of time swans spent resting each hour (mean ± SE) during four consecutive light treatments: (A) first dark control, (B) white light at night, (C) second dark control, and (D) amber light at night. Daylength decreased during the study, such that the earliest sunrise and latest sunset times for each treatment are marked by dashed vertical lines; astronomical night is shaded in gray. Mean night-time rest during the first dark control is marked for visual reference (red horizontal line at 67%).
Daytime Behavior
Light at night did not strongly influence the proportion of time spent resting, preening, or foraging during the subsequent day (Supplementary Figure S3).
Sleep
Sleep electrophysiology (EEG-based sleep) was successfully recorded from one female and one male swan, at separate times (Figure 4). These data are thus preliminary but are also the first recordings of sleep in this species. We analyzed 48 h of sleep data for each swan, commencing from sunrise ≥ 20 h after capture. The female swan experienced the dark control condition on both nights, whereas the male swan experienced dark control conditions on Night 1 and was exposed to white light throughout Night 2. The male swan’s data logger was damaged the morning after Night 2, so that we were unable to investigate potential recovery of sleep after the light exposure.
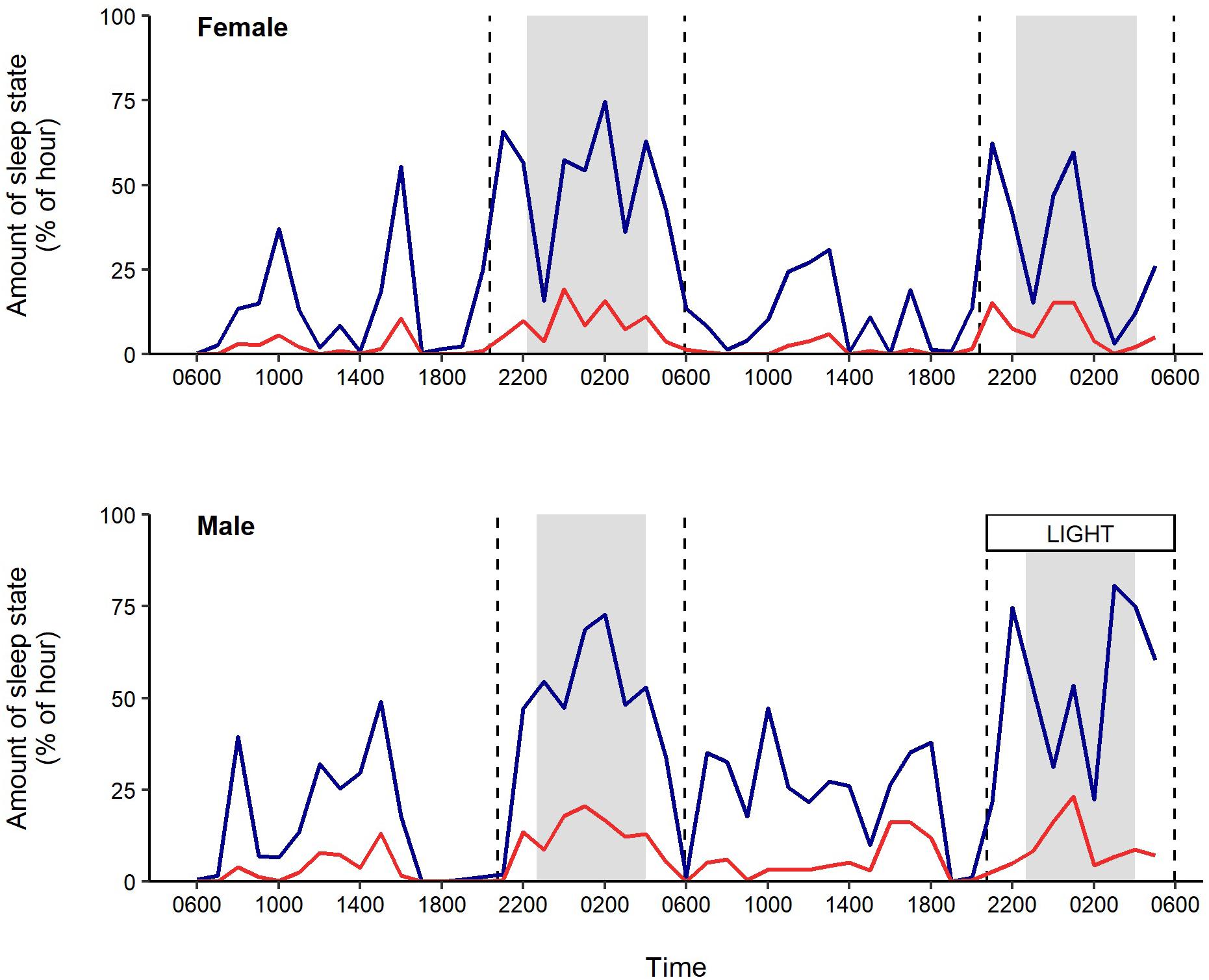
Figure 4. Percentage of time spent in non-REM (blue) and REM sleep (red) per hour in an adult female and male black swan. The male swan was exposed to light from white streetlights throughout the second night. Sunrise and sunset times are marked by dashed vertical lines; astronomical night is shaded in gray.
Dark Control Conditions
The female swan slept a total of 5.40 h on day one and 7.72 h on day two; the male swan slept for 8.02 h. Both swans slept mostly between sunset and sunrise (66.8 – 74.3% of total sleep time); 41.2 – 48.9% of their sleep occurred between astronomical dusk and dawn (Figure 4). Non-REM sleep constituted 81.3 – 85.6% of total sleep time and 15.2 – 21.0% of the 24 h day was spent in non-REM sleep. Daily trends in REM sleep appeared similar to non-REM sleep, with swans spending little of the day (2.3 – 3.6%) and more of the night (7.7 – 15.5 %) in REM sleep. The maximum recorded duration of REM sleep was 44 s; however, almost all other bouts of REM sleep were <12 s long (median duration = 4 s, 98% of bouts ≤ 12 s).
White Light at Night
When the male swan was exposed to a single night of white light, the time spent in non-REM and REM sleep at night decreased (Figure 4). Specifically, there was a 13% decrease in non-REM sleep and a 26% decrease in REM sleep during astronomical night of the white light treatment, relative to the previous (dark) night. This decline arose from a decrease in the number of bouts of non-REM and REM sleep, accompanied by an increase in the mean duration of wakefulness (Supplementary Figures S4–S6).
Relationship Between Rest and Sleep
We found a strong linear relationship between rest and sleep for both swans (Pearson’s correlation; male: r = 0.98; female: r = 0.99; Figure 5), which was highly significant (LM; male: t46 = 27.61, p < 0.01; female: t46 = 50.11, p < 0.01). This relationship applied to daytime (female: t36 = 37.95, p < 0.01; male: t36 = 25.36, p < 0.01), dark night-time (female: t8 = 54.25, p < 0.01; maledark control: t3 = 30.93, p < 0.01), and white light at night data (malewhite light: t3 = 10.54, p < 0.01). For the male swan, but not the female swan, the amount of time spent resting appeared to overestimate the amount of time spent sleeping by ∼10% (mean ± SE: 9.7 ± 1.0%). Interestingly, the male swan also seemed to spend more time inactive but awake during exposure to white light, with inactivity overestimating sleep by 18.3 ± 4.1% during this light treatment. This might suggest that the male was more vigilant during exposure to white light. Nevertheless, this overestimation of sleep would cause us to underestimate the disruptive effects of light pollution on sleep. As such, inactivity is a reasonable and conservative proxy for sleep within our study context.
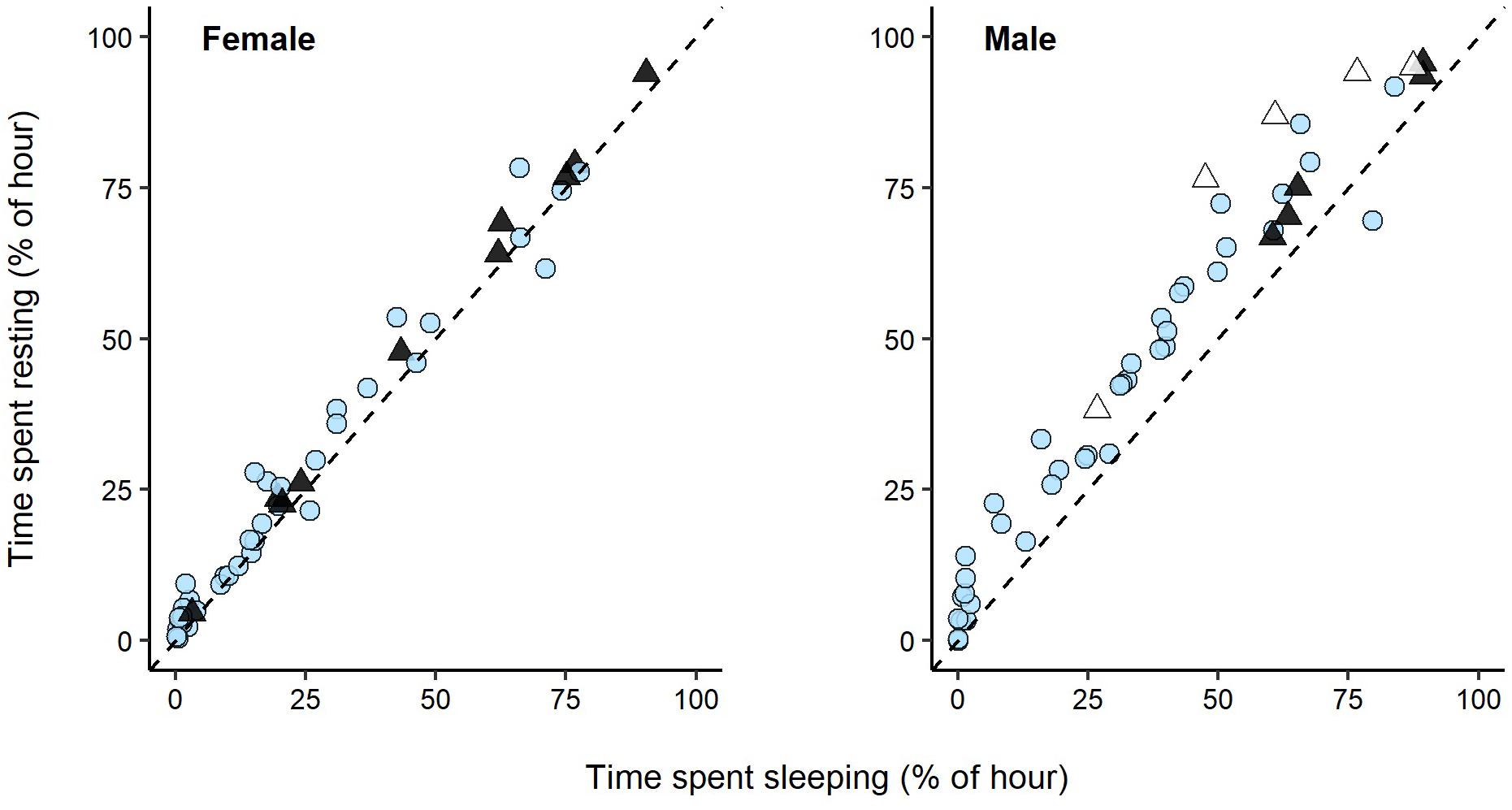
Figure 5. Relationship between time spent sleeping and resting in an adult female and male swan. Sleep and rest were recorded for 48 h during daytime (blue circles), astronomical night with streetlights off (solid triangles), and astronomical night with white streetlights on (open triangles). The dashed line represents the situation where a time spent resting equals time spent sleeping.
Melatonin
Plasma melatonin concentrations in swans tended to be very low, irrespective of time of day [F(3,30) = 1.33, p = 0.28; Supplementary Figure S7]. We found no evidence for an effect of light treatment on circulating melatonin concentrations at night [F(5,47) = 1.23, p = 0.31]. We also found no effect of time between herding swans into the pen and collecting blood samples on plasma melatonin [time of day model: F(1,26) = 2.64, p = 0.12; light treatment model: F(1,44) = 0.11, p = 0.74].
Discussion
In this study, we provide evidence that streetlights disrupt night-time rest in urban birds in a naturalistic setting. Furthermore, we use electrophysiological data to verify that resting black swans are almost always sleeping (and vice versa), allowing us to infer impacts of streetlights on sleep in swans. We therefore refer to rest in swans as “sleep” from here onwards. We found that lighting of similar intensity, spectral composition, and spatial heterogeneity to real-world streetlighting reduced night-time sleep in black swans. Furthermore, the amount of night-time sleep was similar during exposure to amber (blue-reduced) and white (blue-rich) lights. As circulating melatonin concentrations were low, irrespective of time of day or light at night, it is unclear whether reductions in sleep were related to the suppression of melatonin. Streetlighting also did not appear to influence the total amount of sleep each day, with swans showing substantial variation in total sleep time and potentially recovering a small amount of lost sleep toward sunrise. Overall, our findings indicate that having streetlights switched on all night can alter sleep in urban birds and that, contrary to expectation, filtering out blue wavelengths of light might not mitigate these effects.
Disruption of sleep by light at night could have important implications (Aulsebrook et al., 2016). Sleep is important for early development (Kayser et al., 2014), learning (Derégnaucourt et al., 2005), memory (Vorster and Born, 2015), and immune function (Dinges et al., 1995), as well as for repairing DNA damage (Zada et al., 2019) and removing neurotoxic metabolites (Xie et al., 2013) in the brain. Although previous studies have found effects of light at night on night-time activity and behavior in birds (e.g., Dominoni et al., 2013a; Russ et al., 2014; Raap et al., 2015, 2016; Ouyang et al., 2017), this study provides the first evidence that increased night-time activity under artificial light at night (captured by accelerometry) reflects decreased sleep (based on the EEG) in a naturalistic environment. Interestingly, during exposure to white light at night, the male swan also spent more time inactive, yet awake. Our results therefore might even underestimate the suppressive effects of light at night on sleep. Furthermore, we found little evidence for habituation to light at night over multiple weeks of exposure. Together, these findings might suggest that long-term exposure to light from streetlights has negative consequences for waking performance and health in birds.
Still, decreased night-time sleep in swans might not necessarily have adverse implications. We found no evidence for effects of light at night on subsequent daytime behavior in swans, or their total amount of sleep each 24 h day. Swans also showed substantial inter-individual variation in total daily sleep, and even more substantial intra-individual variation, which surpassed any differences due to light at night. Such plasticity in sleep duration might mean that birds are well-adapted to varying their sleep in response to environmental change. For example, a recent study found striking variation in the daily amount of sleep in European starlings (Sturnus vulgaris) in relation to season and lunar phase (van Hasselt et al., 2020). Finally, swans may have also recovered sleep lost during the night by sleeping more intensely. Sleep intensity (or depth) is typically measured as the amount of EEG slow waves during non-REM sleep (slow wave activity; Tobler, 2011). Our EEG data did not allow us to fully explore this possibility, as we only obtained EEG data from one swan under light at night, who damaged his data logger the following morning. Future studies should test for sleep homeostasis following light-induced wakefulness.
Our study cannot determine the mechanisms by which light at night affected sleep, nor explain why amber and white light had such similar effects on sleep. Nevertheless, we can offer some possible explanations. Light at night affects physiology and visual capacity, both of which could influence sleep (Aulsebrook et al., 2018). Physiologically, light at night can disrupt sleep by suppressing the production of melatonin (Lewy et al., 1980; Zawilska, 1996; Cajochen et al., 2000), changing the phase relationship between activity and the circadian clock (Evans et al., 2005), and shifting the timing of the circadian clock (Boivin et al., 1996). These processes are most sensitive to short, blue wavelengths of light (Dominoni, 2015), which would suggest that blue-reduced amber lighting should affect sleep less than blue-rich white lighting. However, sustained exposures to long-wavelength red light can still affect melatonin production and circadian rhythms (Dauchy et al., 2015). While we found no effect of white or amber light at night on melatonin in our study, these results should be interpreted cautiously; melatonin concentrations were very low even under naturally dark conditions, which might have limited our ability to detect decreases under light at night. These results suggest that either swans produce little melatonin or that there was an issue with our blood sampling protocol, potentially because swans were active during the period they were herded for blood sampling (Fusani et al., 2011). The effects of white and amber lighting on physiology might also depend on other lighting characteristics, such as light intensity (de Jong et al., 2017), which warrants further investigation.
Another obvious impact of light at night is that it enhances the visual environment, particularly for diurnal animals. These visual changes might reduce night-time sleep by facilitating other behaviors at night, such as foraging (Russ et al., 2014) or anti-predator vigilance (Yorzinski et al., 2015). The effects of different types of lighting on sleep might therefore depend on how the animal perceives that lighting. While there is no information on the visual sensitivity of black swans, photopic vision in domestic ducks is more sensitive to light in the yellow to red range (577 to 633 nM) than it is for humans (Barber et al., 2006). For our study, this could mean that swans actually perceived the amber lighting as more “intense” than the white lighting, even though both lighting types provided similar illuminance as measured by lux (which is weighted toward the sensitivity of human daytime vision; Lucas et al., 2014). In addition, how animals perceive light at night – and their subsequent behavioral response – could influence the physiological impacts of light at night. For example, if swans avoided exposure to white light at night, then white lighting might have had relatively little effect on their physiology. Anecdotally, the swans in our study did not appear to avoid light at night, which is consistent with evidence from some other birds (Ulgezen et al., 2019) but not all (Dominoni et al., 2014; Yorzinski et al., 2015).
Given that the swans in our study were from urban areas, they may already be relatively tolerant of light at night, and thus our findings could underestimate the possible impact of light at night. Swans also display considerable flexibility in the timing of their behavior – they fly long distances at night (Frith, 1982) and alternate the timing of egg incubation with their partner. Because female swans primarily incubate their eggs at night (and males during the day), disruption of sleep by light at night could disproportionately affect one sex. Studies of the sleep and activity patterns of swans during various life history stages (e.g., breeding and incubation, as well as offspring development) could therefore offer further interesting insights. Moreover, other species that are less flexible than swans in their daily timing of behavior might experience greater impacts of light at night on sleep.
Light at night can have complex effects on wildlife (e.g., Witherington and Bjorndal, 1991; Sella et al., 2006), and use of amber lighting may be beneficial in some contexts (Longcore et al., 2018). However, our findings raise the possibility that filtering streetlights to reduce emission of blue light may not help reduce impacts of light at night on sleep in birds. This is in direct contrast to studies of humans (Santhi et al., 2012; Ayaki et al., 2016), highlighting the need to consider species-specific impacts of both the intensity and color of streetlights. Our research further demonstrates that streetlights can disrupt night-time sleep even in urban-tolerant birds. Although we found no further adverse effects on total sleep, behavior or circulating melatonin, our results and the findings of other studies nevertheless suggest that land managers should consider switching off lights when they are not in use, particularly around parks, reserves and other wildlife habitat.
Data Availability Statement
The datasets generated for this study are available on request to the corresponding author.
Ethics Statement
All methods were approved by The University of Melbourne Animal Ethics Committee (1513532.1), La Trobe University Animal Ethics Committee (AEC13-42.4), Department of Environment, Land, Water, and Planning (permit: 10008176), and the Australian Bird and Bat Banding Scheme (Authority 1405).
Author Contributions
AA, TJ, RM, and JL conceived and designed the study. AA performed the data collection and data analysis with assistance from JL, TJ, and RM and those mentioned in the acknowledgments. JL conducted surgeries with assistance from AA and those mentioned in the acknowledgments. WG facilitated and interpreted the hormone analysis. AV developed and provided Neurologgers. AA wrote the manuscript. All authors commented on the manuscript.
Funding
This research was funded by the Hermon Slade Foundation (awarded to TJ), Australian Research Council (DP170101003 awarded to JL), Holsworth Wildlife Research Endowment (Equity Trustees Charitable Foundation & the Ecological Society of Australia awarded to AA), Australasian Society for the Study of Animal Behaviour (awarded to AA), and BirdLife Australia (awarded to AA).
Conflict of Interest
The authors declare that the research was conducted in the absence of any commercial or financial relationships that could be construed as a potential conflict of interest.
Acknowledgments
The research was conducted at Serendip Wildlife Sanctuary with the permission and support of Parks Victoria. We thank Monika Trappschuh for performing the hormone analysis, Tara Egan and Pat Macwhirter for providing veterinary expertise during surgeries, Rowan Jacques-Hamilton for assisting with data analysis, and Cameron Patrick (Melbourne Statistical Consulting Platform) for providing statistical advice. We also thank the many volunteers who assisted with the research, particularly Farley Connelly, Ashton Dickerson, Gill Holmes, Jason Lacson, Guille Mayor, and Maria Ospina for their help with the daily care of the swans. We are also grateful to Jesko Partecke for providing light loggers and Advanced Lighting Technologies for providing lights for the study.
Supplementary Material
The Supplementary Material for this article can be found online at: https://www.frontiersin.org/articles/10.3389/fevo.2020.00131/full#supplementary-material
Footnotes
References
Alaasam, V. J., Duncan, R., Casagrande, S., Davies, S., Sidher, A., Seymoure, B., et al. (2018). Light at night disrupts nocturnal rest and elevates glucocorticoids at cool color temperatures. J. Exp. Zool. A Ecol. Integr. Physiol. 329, 465–472. doi: 10.1002/jez.2168
Anisimov, V. N., Herbst, J. A., Abramchuk, A. N., Latanov, A. V., Hahnloser, R. H., and Vyssotski, A. L. (2014). Reconstruction of vocal interactions in a group of small songbirds. Nat. Methods 11, 1135–1137. doi: 10.1038/nmeth.3114
Aulsebrook, A. E., Jones, T. M., Mulder, R. A., and Lesku, J. A. (2018). Impacts of artificial light at night on sleep: a review and prospectus. J. Exp. Zool. A Ecol. Integr. Physiol. 329, 409–418. doi: 10.1002/jez.2189
Aulsebrook, A. E., Jones, T. M., Rattenborg, N. C., Roth, T. C. II, and Lesku, J. A. (2016). Sleep ecophysiology: integrating neuroscience and ecology. Trends Ecol. Evol. 31, 590–599. doi: 10.1016/j.tree.2016.05.004
Ayaki, M., Hattori, A., Maruyama, Y., Nakano, M., Yoshimura, M., Kitazawa, M., et al. (2016). Protective effect of blue-light shield eyewear for adults against light pollution from self-luminous devices used at night. Chronobiol. Int. 33, 134–139. doi: 10.3109/07420528.2015.1119158
Barber, C. L., Prescott, N. B., Jarvis, J. R., Le Sueur, C., Perry, G. C., and Wathes, C. M. (2006). Comparative study of the photopic spectral sensitivity of domestic ducks (Anas platyrhynchos domesticus), turkeys (Meleagris gallopavo gallopavo) and humans. Br. Poultry Sci. 47, 365–374. doi: 10.1080/00071660600753870
Bates, D., Mächler, M., Bolker, B., and Walker, S. (2015). Fitting linear mixed-effects models using lme4. J. Stat. Softw. 67, 1–48. doi: 10.18637/jss.v067.i01
Bennie, J., Duffy, J. P., Davies, T. W., Correa-Cano, M. E., and Gaston, K. J. (2015). Global trends in exposure to light pollution in natural terrestrial ecosystems. Rem. Sens. 7, 2715–2730. doi: 10.3390/rs70302715
Boivin, D. B., Duffy, J. F., Kronauer, R. E., and Czeisler, C. A. (1996). Dose-response relationships for resetting of human circadian clock by light. Nature 379, 540–542. doi: 10.1038/379540a0
Cajochen, C., Zeitzer, J. M., Czeisler, C. A., and Dijk, D. J. (2000). Dose-response relationship for light intensity and ocular and electroencephalographic correlates of human alertness. Behav. Brain Res. 115, 75–83. doi: 10.1016/s0166-4328(00)00236-9
Caorsi, V., Sprau, P., Zollinger, S. A., and Brumm, H. (2019). Nocturnal resting behaviour in urban great tits and its relation to anthropogenic disturbance and microclimate. Behav. Ecol. Sociobiol. 73:1.
Da Silva, A., Samplonius, J. M., Schlicht, E., Valcu, M., and Kempenaers, B. (2014). Artificial night lighting rather than traffic noise affects the daily timing of dawn and dusk singing in common European songbirds. Behav. Ecol. 25, 1037–1047. doi: 10.1093/beheco/aru103
Dauchy, R. T., Wren, M. A., Dauchy, E. M., Hoffman, A. E., Hanifin, J. P., Warfield, B., et al. (2015). The influence of red light exposure at night on circadian metabolism and physiology in Sprague-Dawley rats. J. Am. Assoc. Lab. Anim. Sci. 54, 40–50.
de Jong, M., Caro, S. P., Gienapp, P., Spoelstra, K., and Visser, M. E. (2017). Early birds by light at night: effects of light color and intensity on daily activity patterns in blue tits. J. Biol. Rhythms 32, 323–333. doi: 10.1177/0748730417719168
de Jong, M., Jeninga, L., Ouyang, J. Q., van Oers, K., Spoelstra, K., and Visser, M. E. (2016). Dose-dependent responses of avian daily rhythms to artificial light at night. Physiol. Behav. 155, 172–179. doi: 10.1016/j.physbeh.2015.12.012
Derégnaucourt, S., Mitra, P. P., Fehér, O., Pytte, C., and Tchernichovski, O. (2005). How sleep affects the developmental learning of bird song. Nature 433, 710–716. doi: 10.1038/nature03275
Dinges, D. F., Douglas, S. D., Hamarman, S., Zaugg, L., and Kapoor, S. (1995). Sleep deprivation and human immune function. Adv. Neuroimmunol. 5, 97–110. doi: 10.1016/0960-5428(95)00002-j
Dominoni, D. M. (2015). The effects of light pollution on biological rhythms of birds: an integrated, mechanistic perspective. J. Ornithol. 156, 409–418. doi: 10.1007/s10336-015-1196-3
Dominoni, D. M., Borniger, J. C., and Nelson, R. J. (2016). Light at night, clocks and health: from humans to wild organisms. Biol. Lett. 12:20160015. doi: 10.1098/rsbl.2016.0015
Dominoni, D. M., Carmona-Wagner, E. O., Hofmann, M., Kranstauber, B., and Partecke, J. (2014). Individual-based measurements of light intensity provide new insights into the effects of artificial light at night on daily rhythms of urban-dwelling songbirds. J. Anim. Ecol. 83, 681–692. doi: 10.1111/1365-2656.12150
Dominoni, D. M., Goymann, W., Helm, B., and Partecke, J. (2013a). Urban-like night illumination reduces melatonin release in European blackbirds (Turdus merula): implications of city life for biological time-keeping of songbirds. Front. Zool. 10:60. doi: 10.1186/1742-9994-10-60
Dominoni, D. M., Helm, B., Lehmann, M., Dowse, H. B., and Partecke, J. (2013b). Clocks for the city: circadian differences between forest and city songbirds. Proc. R. Soc. B Biol. Sci. 280:20130593. doi: 10.1098/rspb.2013.0593
Evans, J. A., Elliott, J. A., and Gorman, M. R. (2005). Circadian entrainment and phase resetting differ markedly under dimly illuminated versus completely dark nights. Behav. Brain Res. 162, 116–126. doi: 10.1016/j.bbr.2005.03.014
Falchi, F., Cinzano, P., Duriscoe, D., Kyba, C. C. M., Elvidge, C. D., Baugh, K., et al. (2016). The new world atlas of artificial night sky brightness. Sci. Adv. 2:e1600377. doi: 10.1126/sciadv.1600377
Field, A. P., and Wilcox, R. R. (2017). Robust statistical methods: a primer for clinical psychology and experimental psychopathology researchers. Behav. Res. Ther. 98, 19–38. doi: 10.1016/j.brat.2017.05.013
Fusani, L., Cardinale, M., Schwabl, I., and Goymann, W. (2011). Food availability but not melatonin affects nocturnal restlessness in a wild migrating passerine. Horm. Behav. 59, 187–192. doi: 10.1016/j.yhbeh.2010.11.013
Gandhi, A. V., Mosser, E. A., Oikonomou, G., and Prober, D. A. (2015). Melatonin is required for the circadian regulation of sleep. Neuron 85, 1193–1199. doi: 10.1016/j.neuron.2015.02.016
Gaston, K. J., Bennie, J., Davies, T. W., and Hopkins, J. (2013). The ecological impacts of nighttime light pollution: a mechanistic appraisal. Biol. Rev. Camb. Philos. Soc. 88, 912–927. doi: 10.1111/brv.12036
Goymann, W., Trappschuh, M., and Fusani, L. (2008). A gentler method to raise melatonin levels in birds. J. Biol. Rhythms 23, 274–277. doi: 10.1177/0748730408316349
Guay, P. J., and Mulder, R. A. (2009). Do neck-collars affect the behaviour and condition of black swans (Cygnus atratus)? Emu 109, 248–251. doi: 10.1071/mu09020
Hölker, F., Wolter, C., Perkin, E. K., and Tockner, K. (2010). Light pollution as a biodiversity threat. Trends Ecol. Evol. 25, 681–682. doi: 10.1016/j.tree.2010.09.007
Jones, T. M., Durrant, J., Michaelides, E. B., and Green, M. P. (2015). Melatonin: a possible link between the presence of artificial light at night and reductions in biological fitness. Philos. Trans. R. Soc. B Biol. Sci. 370:20140122. doi: 10.1098/rstb.2014.0122
Kayser, M. S., Yue, Z. F., and Sehgal, A. (2014). A critical period of sleep for development of courtship circuitry and behavior in Drosophila. Science 344, 269–274. doi: 10.1126/science.1250553
Kempenaers, B., Borgström, P., Loës, P., Schlicht, E., and Valcu, M. (2010). Artificial night lighting affects dawn song, extra-pair siring success, and lay date in songbirds. Curr. Biol. 20, 1735–1739. doi: 10.1016/j.cub.2010.08.028
Koller, M. (2016). robustlmm: an R package for robust estimation of linear mixed-effects models. J. Stat. Softw. 75, 1–24. doi: 10.18637/jss.v075.i06
Kuznetsova, A., Brockhoff, P. B., and Christensen, R. H. B. (2017). lmerTest package: tests in linear mixed effects models. J. Stat. Softw. 82, 1–26. doi: 10.18637/jss.v082.i13
Lenth, R. (2018). emmeans: Estimated Marginal Means, aka Least-Squares Means. R package version 1.3.1. Available online at: https://CRAN.R-project.org/package=emmeans (accessed April 26, 2019).
Lesku, J. A., Meyer, L. C. R., Fuller, A., Maloney, S. K., Dell’Omo, G., Vyssotski, A. L., et al. (2011a). Ostriches sleep like platypuses. PLoS One 6:e23203. doi: 10.1371/journal.pone.0023203
Lesku, J. A., and Rattenborg, N. C. (2014). Avian sleep. Curr. Biol. 24, R12–R14. doi: 10.1016/j.cub.2013.10.005
Lesku, J. A., Rattenborg, N. C., Valcu, M., Vyssotski, A. L., Kuhn, S., Kuemmeth, F., et al. (2012). Adaptive sleep loss in polygynous pectoral sandpipers. Science 337, 1654–1658. doi: 10.1126/science.1220939
Lesku, J. A., Vyssotski, A. L., Martinez-Gonzalez, D., Wilzeck, C., and Rattenborg, N. C. (2011b). Local sleep homeostasis in the avian brain: convergence of sleep function in mammals and birds? Proc. R. Soc. B Biol. Sci. 278, 2419–2428. doi: 10.1098/rspb.2010.2316
Lewy, A. J., Wehr, T. A., Goodwin, F. K., Newsome, D. A., and Markey, S. P. (1980). Light suppresses melatonin secretion in humans. Science 210, 1267–1269. doi: 10.1126/science.7434030
Longcore, T., Rodríguez, A., Witherington, B., Penniman, J. F., Herf, L., and Herf, M. (2018). Rapid assessment of lamp spectrum to quantify ecological effects of light at night. J. Exp. Zool. A Ecol. Integr. Physiol. 329, 511–521. doi: 10.1002/jez.2184
Lucas, R. J., Peirson, S. N., Berson, D. M., Brown, T. M., Cooper, H. M., Czeisler, C. A., et al. (2014). Measuring and using light in the melanopsin age. Trends Neurosci. 37, 1–9. doi: 10.1016/j.tins.2013.10.004
Martinez-Gonzalez, D., Lesku, J. A., and Rattenborg, N. C. (2008). Increased EEG spectral power density during sleep following short-term sleep deprivation in pigeons (Columba livia): evidence for avian sleep homeostasis. J. Sleep Res. 17, 140–153. doi: 10.1111/j.1365-2869.2008.00636.x
Mulder, R. A., Guay, P.-J., Wilson, M., and Coulson, G. (2010). Citizen science: recruiting residents for studies of tagged urban wildlife. Wildl. Res. 37, 440–446. doi: 10.1071/wr10007
Ouyang, J. Q., de Jong, M., van Grunsven, R. H. A., Matson, K. D., Haussmann, M. F., Meerlo, P., et al. (2017). Restless roosts: light pollution affects behavior, sleep, and physiology in a free-living songbird. Glob. Change Biol. 23, 4987–4994. doi: 10.1111/gcb.13756
Pandi-Perumal, S. R., Srinivasan, V., Maestroni, G. J. M., Cardinali, D. P., Poeggeler, B., and Hardeland, R. (2006). Melatonin – nature’s most versatile biological signal? FEBS J. 273, 2813–2838. doi: 10.1111/j.1742-4658.2006.05322.x
R Development Core Team (2018). R: A Language and Environment for Statistical Computing. Vienna: R Foundation for Statistical Computing.
Raap, T., Pinxten, R., and Eens, M. (2015). Light pollution disrupts sleep in free-living animals. Sci. Rep. 5:13557. doi: 10.1038/srep13557
Raap, T., Pinxten, R., and Eens, M. (2016). Artificial light at night disrupts sleep in female great tits (Parus major) during the nestling period, and is followed by a sleep rebound. Environ. Pollut. 215, 125–134. doi: 10.1016/j.envpol.2016.04.100
Raap, T., Pinxten, R., and Eens, M. (2018). Cavities shield birds from effects of artificial light at night on sleep. J. Exp. Zool. A Ecol. Integr. Physiol. 329, 449–456. doi: 10.1002/jez.2174
Rattenborg, N. C., de la Iglesia, H. O., Kempenaers, B., Lesku, J. A., Meerlo, P., and Scriba, M. F. (2017). Sleep research goes wild: new methods and approaches to investigate the ecology, evolution and functions of sleep. Philos. Trans. R. Soc. B Biol. Sci. 372:20160251. doi: 10.1098/rstb.2016.0251
Reiter, R. J., Tan, D. X., and Fuentes-Broto, L. (2010). Melatonin: a multitasking molecule. Prog. Brain Res. 181, 127–151. doi: 10.1016/s0079-6123(08)81008-4
Russ, A., Rüger, A., and Klenke, R. (2014). Seize the night: European blackbirds (Turdus merula) extend their foraging activity under artificial illumination. J. Ornithol. 156, 123–131. doi: 10.1007/s10336-014-1105-1
Santhi, N., Thorne, H. C., van der Veen, D. R., Johnsen, S., Mills, S. L., Hommes, V., et al. (2012). The spectral composition of evening light and individual differences in the suppression of melatonin and delay of sleep in humans. J. Pineal Res. 53, 47–59. doi: 10.1111/j.1600-079X.2011.00970.x
Sella, K. N., Salmon, M., and Witherington, B. E. (2006). Filtered streetlights attract hatchling marine turtles. Chelonian Conserv. Biol. 5, 255–261.
Spoelstra, K., Verhagen, I., Meijer, D., and Visser, M. E. (2018). Artificial light at night shifts daily activity patterns but not the internal clock in the great tit (Parus major). Proc. R. Soc. B Biol. Sci. 285:20172751. doi: 10.1098/rspb.2017.2751
Stevens, R. G., and Zhu, Y. (2015). Electric light, particularly at night, disrupts human circadian rhythmicity: is that a problem? Philos. Trans. R. Soc. B Biol. Sci. 370:20140120. doi: 10.1098/rstb.2014.0120
Tobler, I. (2011). “Phylogeny of sleep regulation,” in Principles and Practice of Sleep Medicine, eds M. H. Kryger, T. Roth, and W. C. Dement, (Philadelphia, PA: Elsevier Health Sciences), 112–125. doi: 10.1016/b978-1-4160-6645-3.00009-8
Ulgezen, Z. N., Kapyla, T., Meerlo, P., Spoelstra, K., Visser, M. E., and Dominoni, D. M. (2019). The preference and costs of sleeping under light at night in forest and urban great tits. Proc. R. Soc. B Biol. Sci. 286:20190872. doi: 10.1098/rspb.2019.0872
van Hasselt, S. J., Rusche, M., Vyssotski, A. L., Verhulst, S., Rattenborg, N. C., and Meerlo, P. (2020). Sleep time in the European starling is strongly affected by night length and moon phase. Curr. Biol. doi: 10.1016/j.cub.2020.02.052
CrossRef Full Text [Epub ahead of print], | PubMed Abstract | Google Scholar
Vorster, A. P., and Born, J. (2015). Sleep and memory in mammals, birds and invertebrates. Neurosci. Biobehav. Rev. 50, 103–119. doi: 10.1016/j.neubiorev.2014.09.020
Vyssotski, A. L., Dell’Omo, G., Dell’Ariccia, G., Abramchuk, A. N., Serkov, A. N., Latanov, A. V., et al. (2009). EEG responses to visual landmarks in flying pigeons. Curr. Biol. 19, 1159–1166. doi: 10.1016/j.cub.2009.05.070
Wilson, R. P., White, C. R., Quintana, F., Halsey, L. G., Liebsch, N., Martin, G. R., et al. (2006). Moving towards acceleration for estimates of activity-specific metabolic rate in free-living animals: the case of the cormorant. J. Anim. Ecol. 75, 1081–1090. doi: 10.1111/j.1365-2656.2006.01127.x
Witherington, B. E., and Bjorndal, K. A. (1991). Influences of wavelength and intensity on hatchling sea turtle phototaxis: implications for sea-finding behavior. Copeia 1991, 1060–1069.
Xie, L. L., Kang, H. Y., Xu, Q. W., Chen, M. J., Liao, Y. H., Thiyagarajan, M., et al. (2013). Sleep drives metabolite clearance from the adult brain. Science 342, 373–377. doi: 10.1126/science.1241224
Yorzinski, J. L., Chisholm, S., Byerley, S. D., Coy, J. R., Aziz, A., Wolf, J. A., et al. (2015). Artificial light pollution increases nocturnal vigilance in peahens. PeerJ 3:e1174. doi: 10.7717/peerj.1174
Zada, D., Bronshtein, I., Lerer-Goldshtein, T., Garini, Y., and Appelbaum, L. (2019). Sleep increases chromosome dynamics to enable reduction of accumulating DNA damage in single neurons. Nat. Commun. 10:895. doi: 10.1038/s41467-019-08806-w
Zawilska, J. B. (1996). Melatonin as a chemical indicator of environmental light-dark cycle. Acta Neurobiol. Exp. 56, 757–767.
Zawilska, J. B., Bereziñska, M., Rosiak, J., Vivien-Roels, B., Skene, D. J., Pévet, P., et al. (2003). Daily variation in the concentration of melatonin and 5-methoxytryptophol in the goose pineal gland, retina, and plasma. Gen. Comp. Endocrinol. 134, 296–302. doi: 10.1016/s0016-6480(03)00269-7
Keywords: accelerometry, artificial light at night, blue light, EEG, elecrophysiology, light pollution, light spectra
Citation: Aulsebrook AE, Lesku JA, Mulder RA, Goymann W, Vyssotski AL and Jones TM (2020) Streetlights Disrupt Night-Time Sleep in Urban Black Swans. Front. Ecol. Evol. 8:131. doi: 10.3389/fevo.2020.00131
Received: 06 December 2019; Accepted: 21 April 2020;
Published: 19 May 2020.
Edited by:
Davide M. Dominoni, University of Glasgow, United KingdomReviewed by:
Kevin Wood, Wildfowl & Wetlands Trust, United KingdomJacqueline Van Der Meij, Radboud University Nijmegen, Netherlands
Erica Stuber, Cornell Lab of Ornithology, United States
Copyright © 2020 Aulsebrook, Lesku, Mulder, Goymann, Vyssotski and Jones. This is an open-access article distributed under the terms of the Creative Commons Attribution License (CC BY). The use, distribution or reproduction in other forums is permitted, provided the original author(s) and the copyright owner(s) are credited and that the original publication in this journal is cited, in accordance with accepted academic practice. No use, distribution or reproduction is permitted which does not comply with these terms.
*Correspondence: Anne E. Aulsebrook, YXVsc2Vicm9va2FAZ21haWwuY29t