- 1Department of Biology, CESAM - Centre for Environmental and Marine Studies, Aveiro, Portugal
- 2South Iceland Research Centre, University of Iceland, Laugarvatn, Iceland
For many migratory species, migration can represent a significant part of the annual cycle and the strategies used to move between the breeding and non-breeding areas vary considerably. Weather conditions are important during migration, particularly wind and temperature, and can play a crucial role in the timing of events during the annual cycle of migratory birds. When timing of specific events is important, for example spring arrival and laying dates, the effects of weather on the previous migration might lead to important fitness consequences, as it may alter migration speed. During spring, Icelandic whimbrels Numenius phaeopus islandicus display two main migratory behaviors: a direct flight from the wintering to the breeding sites (direct migration), or, more commonly, two flights with one stopover (stopover migration). We investigated how wind conditions, temperature and spring departure date may drive individuals to adopt either migratory behavior. Interestingly, we found no differences in wind support during migratory flights, in temperature closer to Iceland or on crosswinds experienced in the region before reaching the main stopover areas. However, when individuals undertook a direct flight, departure date from the wintering sites was on average later, but this was not explained by wind patterns over a period of 7 days prior to departure. In addition, we explored the variation at the individual level for three birds that changed migratory behavior between years. The differences in all variables for these individuals reflected the variation observed at the population level. Overall, in such long migrations, it seems advantageous to perform a shorter flight to a stopover area, from where the weather conditions in the breeding areas may be assessed and avoid the risk of facing stochastic inclement weather prior to breeding, while synchronizing time of arrival with conspecifics. In contrast, direct flights seem more common when individuals are time pressed.
Introduction
Migration can represent a significant part of an individual annual cycle and the strategies used to move between the breeding and non-breeding areas vary considerably (Newton, 2007; Hansson and Åkesson, 2014). Animals may take advantage of the flow of the medium where they move to support migration (e.g., air or water; Chapman et al., 2011) and optimal bird migration theory postulates that energy or time costs should be kept at a minimum (Alerstam and Lindström, 1990). Hence, weather conditions are often taken into consideration during migration, particularly wind and temperature, as these parameters have been shown to influence migration at different stages and in different ways (Liechti, 2006; Shamoun-Baranes et al., 2017). During migratory flight, wind can be favorable but also cause birds to extend the length of migration (Gill et al., 2014), drift due to crosswinds (Grönroos et al., 2013; Horton et al., 2016) or even force stops (Shamoun-Baranes et al., 2010; Klaassen et al., 2017); the effect of temperature on flight has been studied to less extent, but it has been shown to influence individuals’ flight altitude (Senner et al., 2018). At stationary locations (e.g., during wintering or stopover), individuals can either select favorable winds at departure (Schaub et al., 2004; Gill et al., 2014), or depart regardless of wind conditions (Thorup et al., 2006), and this can be context dependent (e.g., depending on subsequent flight length, season and migratory strategy; Dierschke and Delingat, 2001; Schmaljohann et al., 2012; Packmor et al., 2020); additionally, temperature can also influence the decision of when to initiate the migratory flight (Schmaljohann et al., 2013; Berchtold et al., 2017). Besides extrinsic factors, migratory behavior can be shaped by individual condition too. For example, individual fuel reserves at departure can influence where to stop next (Anderson et al., 2019), and departure probability can result from an interaction of fuel load and wind conditions (Arizaga et al., 2011). Weather can therefore play an important role on the annual cycle of migratory birds and changes in the atmospheric patterns at a large scale may influence whole populations or species, through costs of energy and time during migration (La Sorte and Fink, 2017; La Sorte et al., 2018). Understanding the mechanisms shaping migratory behavior can thus help predicting how these species will respond to variation in weather patterns.
When the timing of specific annual events is relevant for individual fitness, such as the timing of breeding (Perrins, 1970; Drent, 2006), the effect of weather during spring migration might have important consequences, as it can alter migration speed (Alerstam and Gudmundsson, 1999; Shamoun-Baranes et al., 2010). For example, if an individual encounters unfavorable weather conditions during spring migration, it may increase the duration of migration, leading to latter arrival at the breeding grounds, later breeding and ultimately result in lower breeding success than conspecifics breeding earlier (Drake et al., 2014). Therefore, it might be expected that birds will select the best wind support at departure so that at least the initial stages of migratory flight can take advantage of such subsidies. However, if wind conditions during the departure period are stable, the timing of departure should be independent of wind (Weber and Hedenström, 2000). In addition, there can be particular risks associated with long flights over unsuitable habitat for landing or feeding. Unfavorable weather conditions encountered en route may lead to higher energy expenditure, increasing the probability of depleting fuel stores before a suitable location for stopping is within reach and, in extreme conditions, result in mortality (Newton, 2006).
Icelandic whimbrels Numenius phaeopus islandicus typically complete their annual migrations in two or three flights (Alves et al., 2016; Carneiro et al., 2019a). After breeding in Iceland, autumn migration is commonly completed in one flight over open waters to the wintering sites, which are mostly located in West Africa. During spring, however, two main migratory behaviors have been identified, regarding route and number of stopovers: a direct migration, where whimbrels again fly non-stop to Iceland, and a stopover migration, where individuals travel first to a stopover site (usually in Britain and Ireland) and then fly to the breeding sites (7–17 days later; Carneiro et al., 2019a). Hence, these two migratory behaviors differ in travel duration from the winter sites to Iceland, but also in the timing of arrival at the breeding sites, with direct migrants arriving earlier than those undertaking a stopover (unpublished data). Arriving early to a region where favorable breeding conditions are available for a relatively short period can be important, as it may increase the probability of successful reproduction (Morrison et al., 2019). In fact, in Icelandic whimbrels, fledging success decreases with laying date (submitted). Despite this, stopover migration is more common, occurring in 80% of occasions (Carneiro et al., 2019a).
Here, we explore how wind conditions, temperature and departure date from winter location relate to the spring migratory behavior (direct vs. stopover) of Icelandic whimbrels. We envisage two non-mutually exclusive scenarios: (i) whimbrels adjust migratory behavior during flight, depending on conditions experienced en route up to the suitable stopover locations; (ii) make migratory decisions prior to departure, given local (weather) conditions. In order to assess the first hypothesis, we define a “decision latitude” where birds might change overall direction of migration, and test if wind support experienced until this latitude and the temperature at this latitude differs between individuals taking a direct or a stopover route. Additionally, we compare the zonal (longitudinal) wind conditions experienced between migratory behaviors when individuals approach the main stopover region. We expect that wind conditions experienced en route will be more favorable (e.g., more wind support, weak westerlies) and/or temperature higher when individuals perform a direct migration, as fuel reserves should be higher, and temperature can act as a cue that conditions in Iceland are likely to be favorable upon arrival. To investigate if migratory behavior is defined prior to departure, we test the role of departure date from the wintering sites, and the influence of wind support at this stage. Given previous evidence, we expect departure date to differ between migratory behaviors, and that whimbrel’s selection for wind support shall depend on the variability of wind conditions during the departure period (Weber and Hedenström, 2000; Thorup et al., 2006). In addition, using three repeatedly tracked birds that changed migratory behaviors over the years, we explore how individual level variation compares to population level variation on the factors mentioned above.
Materials and Methods
From 2012 to 2018, adult whimbrels were caught on the nest, in the southern lowlands of Iceland (63.8°N; 20.2°W), using a spring trap (Moudry TR601). Each bird was fitted with a combination of color rings and a geolocator attached to a leg flag (model Intigeo-W65A9RJ from 2012 to 2014 and Intigeo-C65 since; Migrate Technology Ltd.). Tagged individuals were caught using the same technique one or more years later in order to retrieve and replace the devices, allowing repeated tracking over the years. We deployed a total of 138 geolocators, and retrieved 66, from 39 individuals, despite the return rate to the breeding areas being higher (unpublished data). One device was damaged and contained no data, another stopped recording shortly after departure from Iceland, and a third in mid-winter. Bird sex was determined using biometrics following Katrínardóttir et al. (2013; n = 24), molecularly (as in Katrínardóttir et al., 2013; n = 9) or through behavioral observation (copulating position, assuming that males were on top; n = 3). In order to estimate geographical locations, light data recorded by the geolocators was analyzed as described in Carneiro et al. (2019a). Given that these data only allow a minimum temporal resolution of 12 h (two locations per day), we used geolocator recorded data on temperature, conductivity and wet contacts (recorded every 4 h) to refine timings of departure and arrival (Battley and Conklin, 2017; see details in Carneiro et al., 2019a). Stopover and winter locations were defined, for each individual and tracked year, as the average of all locations during each period. In five cases, a movement southward was observed at spring departure from the wintering sites. We considered this unlikely and due to geolocation precision errors through shading, and assumed migration from the first position on the track northwards. The location of arrival into Iceland was assumed to be the breeding region (63.8°N, 20.2°W).
Whimbrels are known to migrate in flocks (Piersma et al., 1990; Watts et al., 2017), therefore migratory decisions may or may not be taken independently by a given individual. Geolocator tracks allow individual migratory behavior to be related to phenology and external factors like weather, but migratory decisions such as whether to stopover may be made communally. However, all individuals in a given flock are subject to same external drivers during migration so relating individual movement patterns to external forces remains meaningful.
Temperature and Wind Data
Temperature and wind data were retrieved from the National Centres for Environmental Prediction (NCEP; Kanamitsu et al., 2002), using the R package “RNCEP” (Kemp et al., 2012a). Although bird migration can occur at higher altitudes (e.g., Alerstam and Gudmundsson, 1999), whimbrel flight altitude during migration is unlikely to be higher than 1,500 m above sea level (a. s. l.; Alves et al., 2016, unpublished data). Hence, data was retrieved for each location, at the air pressure of 1,000, 925, and 850 hPa, representing altitudes of 111, 762, and 1,457 m above sea level, respectively. In addition, temperature data during the departure period from the stopover site was also retrieved for the surface level.
Based on visual inspection of whimbrel tracks (Carneiro et al., 2019a), we defined a “decision latitude” at 42°N, where individuals might change the overall migration direction and stop, and a “decision window” between 37 and 50°N, i.e., the region before the main stopover locations in Britain and Ireland, where individuals might be influenced and pushed east by zonal winds to make a stopover, as westerlies prevail in this region. Additionally, given the subjectivity of the assumptions above and the error associated with geolocator positional data (ca. 200 km; Phillips et al., 2004; Shaffer et al., 2005; Fudickar et al., 2012), we also considered two more “decision latitudes” at 37 and 47°N. These latitudes are ca. 10% of the average distance between wintering and breeding sites (assuming a great circle route), to the south and north of 42°N. The locations and time when crossing 37, 42, and 47°N were interpolated linearly, assuming constant ground speed.
For all individuals, each location attained during migration, wintering and stopover sites, was annotated with the zonal (u) and meridional (v) wind components. In order to investigate wind support during migratory flight, we calculated the flow assistance (FA) as the tailwind component (Kemp et al., 2012b) and the air-to-ground speed ratio (AGR; Alerstam, 1979; Gill et al., 2014). The distance between positions was calculated as the great circle distance using the function “distCosine” of the R package “geosphere” (Hijmans, 2016). Ground speed was calculated as the speed between consecutive positions during migratory flights, and air speed as the difference of ground speed and the FA component. Hence, when AGR < 1 the individual is experiencing wind support, while AGR > 1 indicates that wind is an impediment to movement. The AGR value at wintering departure for one individual was an outlier (14.05). Since this could have been due to an erroneous location, either at departure or the following one, both locations and associated wind components were removed from further analysis.
Statistical Analysis
We built a generalized linear model (GLM; family binomial) in order to test whether migratory behavior (direct or stopover) varies with mean AGR until 42°N, air temperature at 42°N and departure date from the wintering site, for each air pressure (1,000, 925, and 850 hPa). In addition, we built similar models considering migration until latitudes 37 and 47°N.
In order to test for the influence of wind while crossing the region between 37 and 50°N, we restricted the data to locations within that window and built a GLM (family binomial) with migratory behavior as independent variable and the zonal wind component as explanatory variable, which represents east/west winds. As above, this model was performed for each air pressure (1,000, 925, and 850 hPa). Although variables such as sex, year, and individual may influence spring migratory behavior, these variables were not included in these models as random factors, because sample size limitations (i.e., observations biased toward stopover behavior, that is far more common; Carneiro et al., 2019b) and unbalanced samples across individuals prevented models to converge or led to overdispersion (Harrison et al., 2018). Nevertheless, in order to assess their possible effect, we constructed reduced models for all combinations of each independent and random variable, for migrations until 42°N and for the region between 37 and 50°N, at the air pressure of 1,000 hPa.
In order to assess if wind support at the wintering site can influence departure date, we retrieved the u and v wind components from NCEP (Kanamitsu et al., 2002; Kemp et al., 2012a), at the same altitudes as above, for the date and location of departure and for each of the previous 7 days (at the same time of the day as the day of departure). Icelandic whimbrels are consistent on departure date from the wintering sites, with a mean individual range of 3.6 days (Carneiro et al., 2019b), hence 7 days (i.e., a period twice as long) were considered to represent the period over which a decision to depart is likely to occur. Then, we calculated AGR and performed a Wilcoxon rank sum test to examine differences between migratory behavior at time of departure. In order to assess if whimbrels select certain wind support at departure, for each migratory behavior we performed a GLM (family binomial) with departure (depart or not) as independent variable and AGR as explanatory variable.
Among the 13 individuals with repeated tracks, only three changed migratory behavior, from direct to stopover. One individual was tracked during two spring migrations, and two individuals were tracked during five spring migrations. Using these data, we further explored individual variation between strategies using the same variables as above. Data were analyzed in software R (R Core Team, 2018) and results are reported as mean ± se, unless otherwise stated.
Results
We compared 9 direct to 48 stopover spring migrations, performed by 36 individuals (16 females and 20 males; Figure 1). Stopover sites were located along the Portuguese continental Atlantic coast (n = 3), northwest of France (n = 4), and Ireland and NW Britain (n = 41; Figure 1).
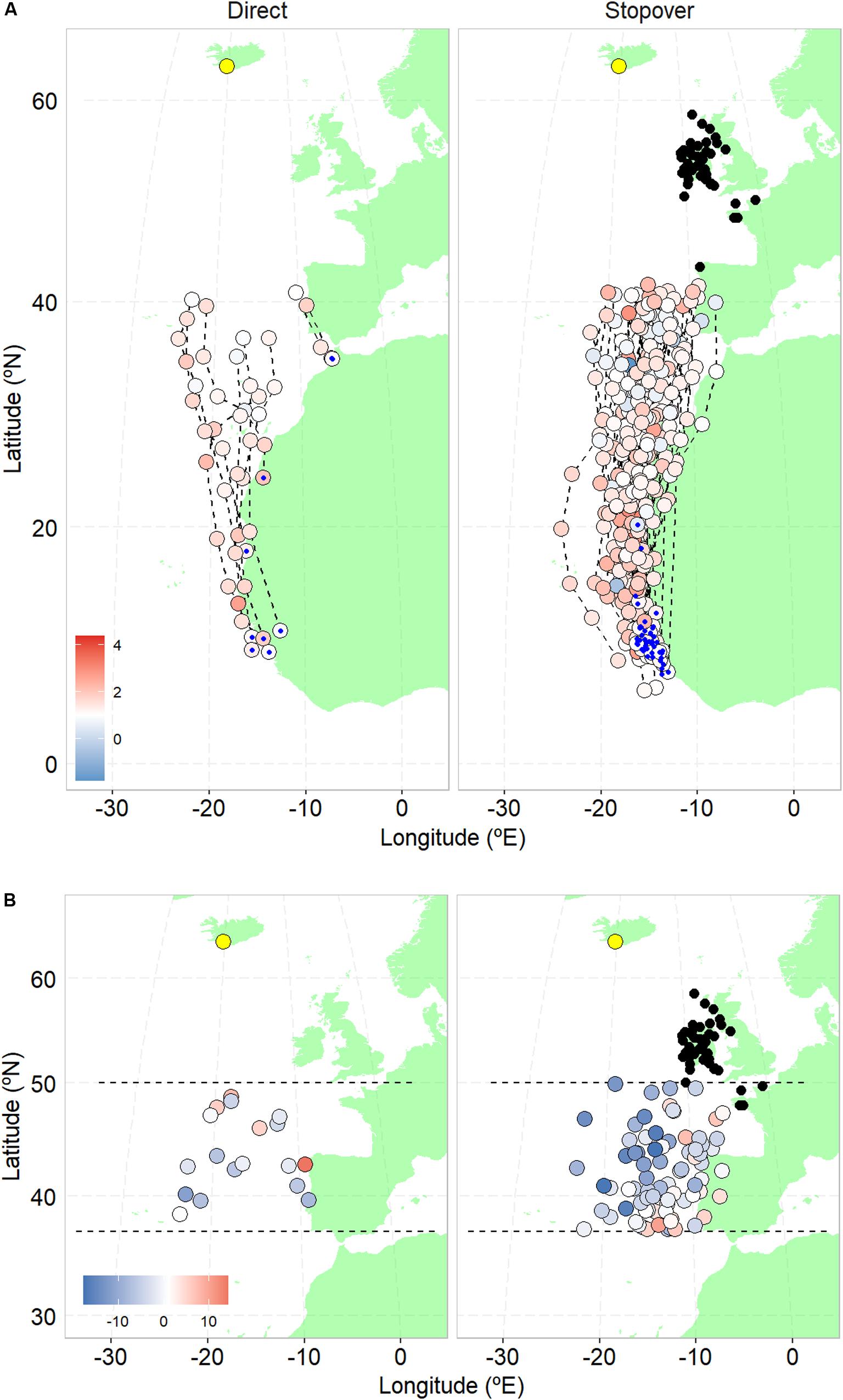
Figure 1. (A) Spring migration locations recorded with geolocators up to latitude 42°N, colored by air-to-ground speed ratio (at 1,000 hPa); blue represents wind support (values below one), whereas red represents wind impediment in the direction of movement (values above one); individual winter locations are given as blue dots. (B) Locations during spring migration between latitude 37 and 50°N, colored by zonal wind (u, in ms−1, at 1,000 hPa), where blue (negative) values represent westward winds and red (positive) values eastward winds. Circles depict stopover (black) and breeding (yellow) locations. Left panels show locations of individual whimbrels undertaking a direct migration (n = 9), and right panels a stopover migration (n = 48).
For the air pressure levels considered (representing altitudes of 111, 762, and 1,457 m a. s. l.), the mean air-to-ground speed ratio until 42°N and air temperature at that latitude, did not differ for whimbrels undertaking either migratory behavior (Table 1A and Figures 1A, 2A,B); but departure date from the wintering sites did, with birds that departed later tending to undertake a direct flight (direct migration: 27 April ± 1.7 days, n = 9; stopover migration: 19 April ± 0.6 days, n = 48; Table 1A and Figure 2C). When considering the “decision latitude” at 37°N or at 47°N, the results were similar (Supplementary Table S1).
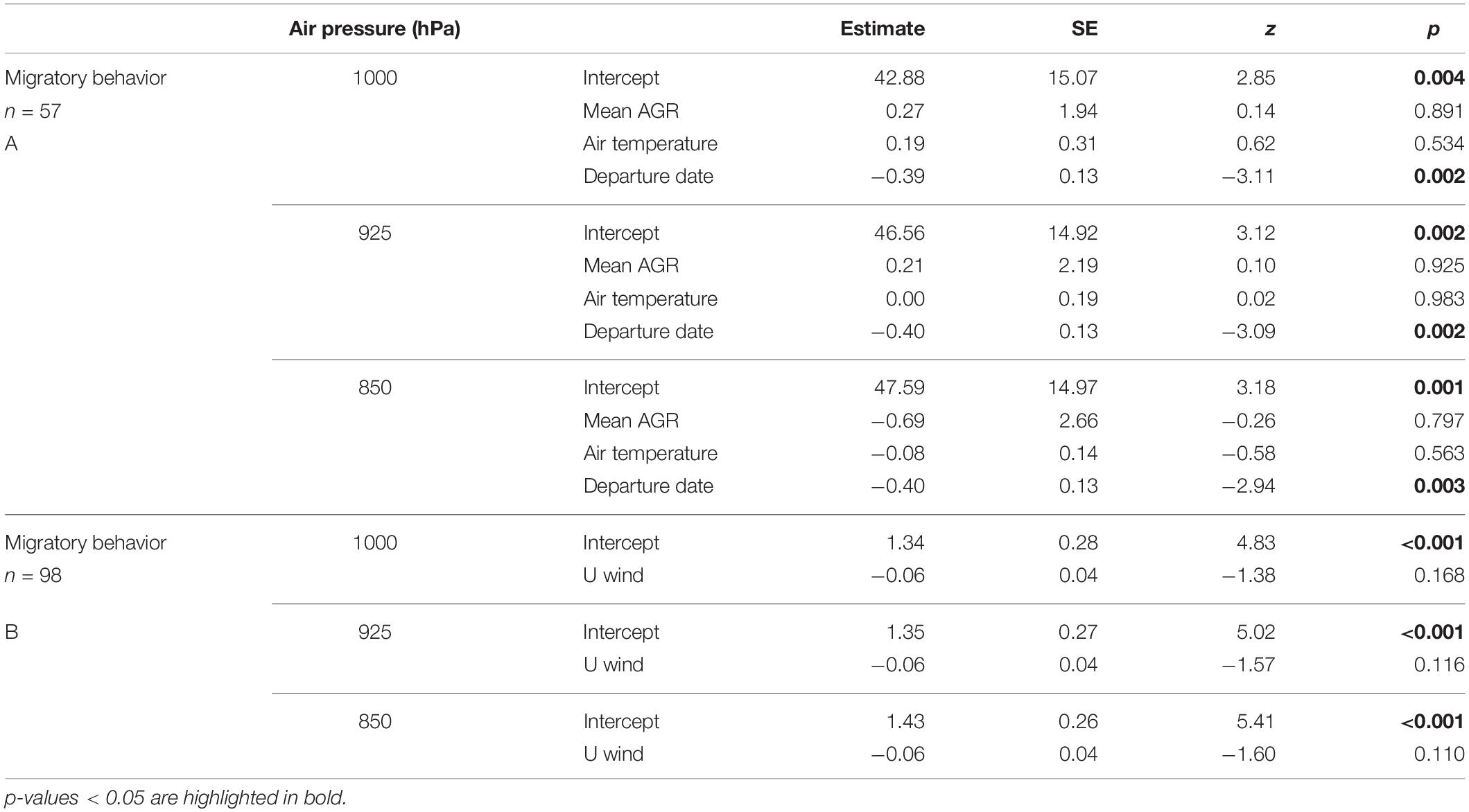
Table 1. Generalized linear models testing potential drivers of spring migratory behavior (direct vs. stopover): (A) individual mean air-to-ground speed ratio (AGR) experienced up to crossing 42°N, air temperature at 42°N and departure date from the wintering sites; and (B) zonal wind (east/westward) experienced between 37 and 50°N, for all locations recorded across individuals.
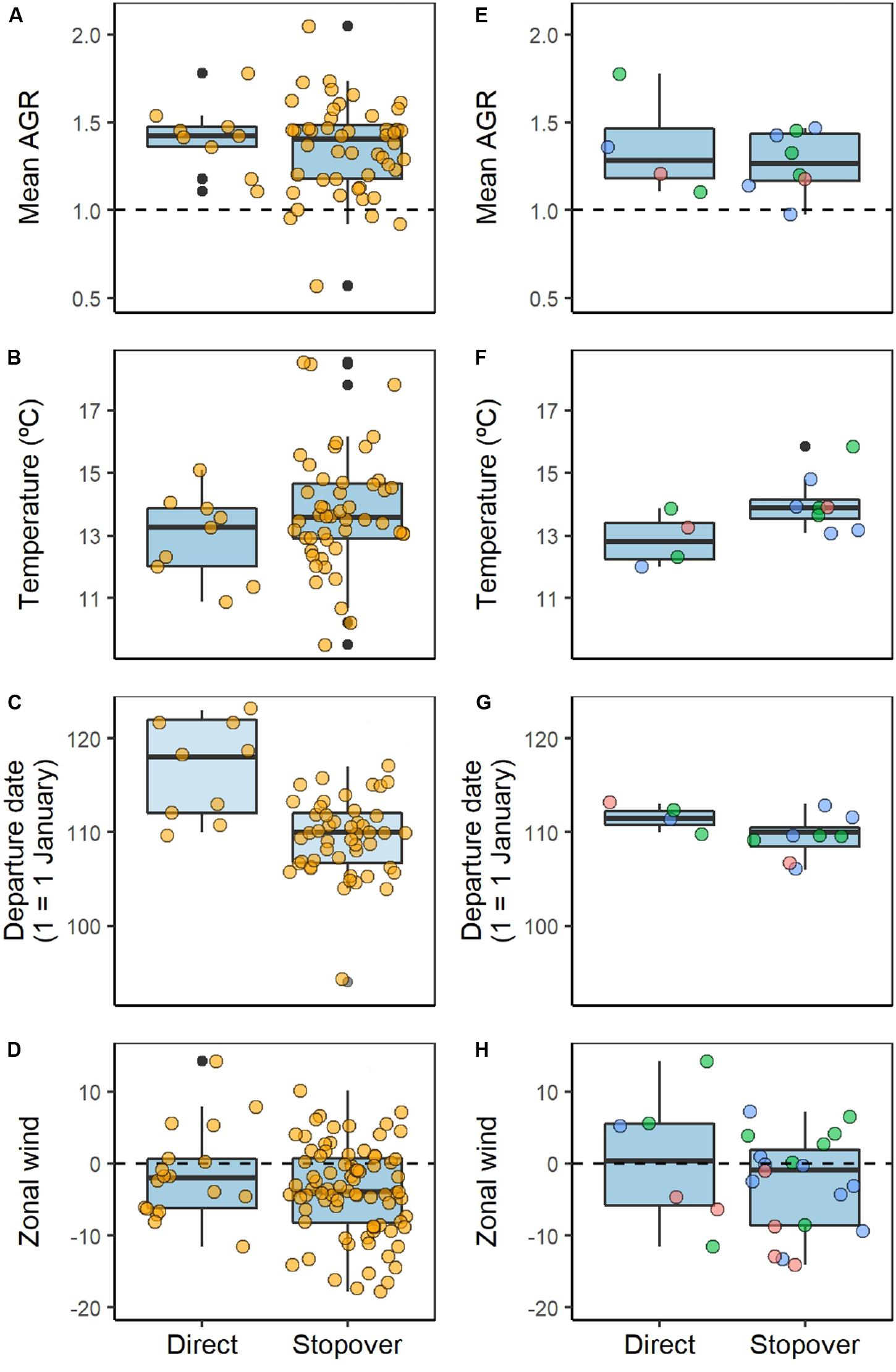
Figure 2. Variation in potential drivers of spring migratory behavior (direct vs. stopover) of Icelandic whimbrels at the population (left column; A–D; n = 9 direct and 48 stopover migrations), and individual levels (right column, E–H). The three individuals that changed spring migratory behavior between years (right column) are coded by color. (A,E) Mean individual air-to-ground speed ratio (AGR) from departure up to latitude 42°N (values below one represent wind support, while values above one mean impediment in the direction of movement). (B,F) Air temperature experienced by each individual at latitude 42°N. (C,G) Departure date from the wintering site. (D,H) Zonal wind (u) component, (ms−1) between 37 and 50°N, where negative values represent westward winds and positive values eastward winds (note that between those latitudes the number of locations per track can be more than one, hence the number of locations is higher than the number of migrations; n = 18 for direct and n = 80 for stopover migrations). All data refers to air pressure 1,000 hPa (i.e., 111 m a. s. l.). Boxplots show the median and 25 and 75% quartiles, whiskers extending up to 1.5 times the inter quartile range from the median and points beyond that are individually marked in black.
The zonal wind component when crossing the region from latitude 37°N to 50°N was not different between migratory behaviors at any air pressure level considered (Table 1B and Figures 1B, 2D), and was predominantly westward (e.g., for 1,000 hPa, direct migration: −1.49 ± 1.52, n = 18; stopover migration −3.79 ± 0.70, n = 80).
The reduced models provided the same results when considering a possible effect of year or sex on migratory behavior (Table 1 and Supplementary Table S2). However, in a reduced model with departure date as explanatory variable for migratory behavior and individual as random term, we did not find a significant effect (Supplementary Table S2), likely due to the low sample size.
Wind support at departure from the wintering sites, measured as AGR at an air pressure of 1,000 hPa did not differ between strategies (Wilcoxon rank sum test: W = 276, p = 0.156; direct: 1.45 ± 0.15, n = 9; stopover: 1.22 ± 0.05, n = 47), although it did at higher altitudes, for 925 hPa (Wilcoxon rank sum test: W = 329, p = 0.007; direct: 1.41 ± 0.15, n = 9; stopover: 1.07 ± 0.03, n = 47) and 850 hPa (Wilcoxon rank sum test: W = 352, p = 0.001; direct: 1.22 ± 0.10, n = 9; stopover: 0.92 ± 0.03, n = 47), being higher (i.e., higher impediment for movement) for birds that flew directly. For each migratory behavior, the AGR at departure and during the previous 7 days had no effect on departure, at any altitude considered, suggesting no or little selection for wind conditions to initiate the flight (Table 2).
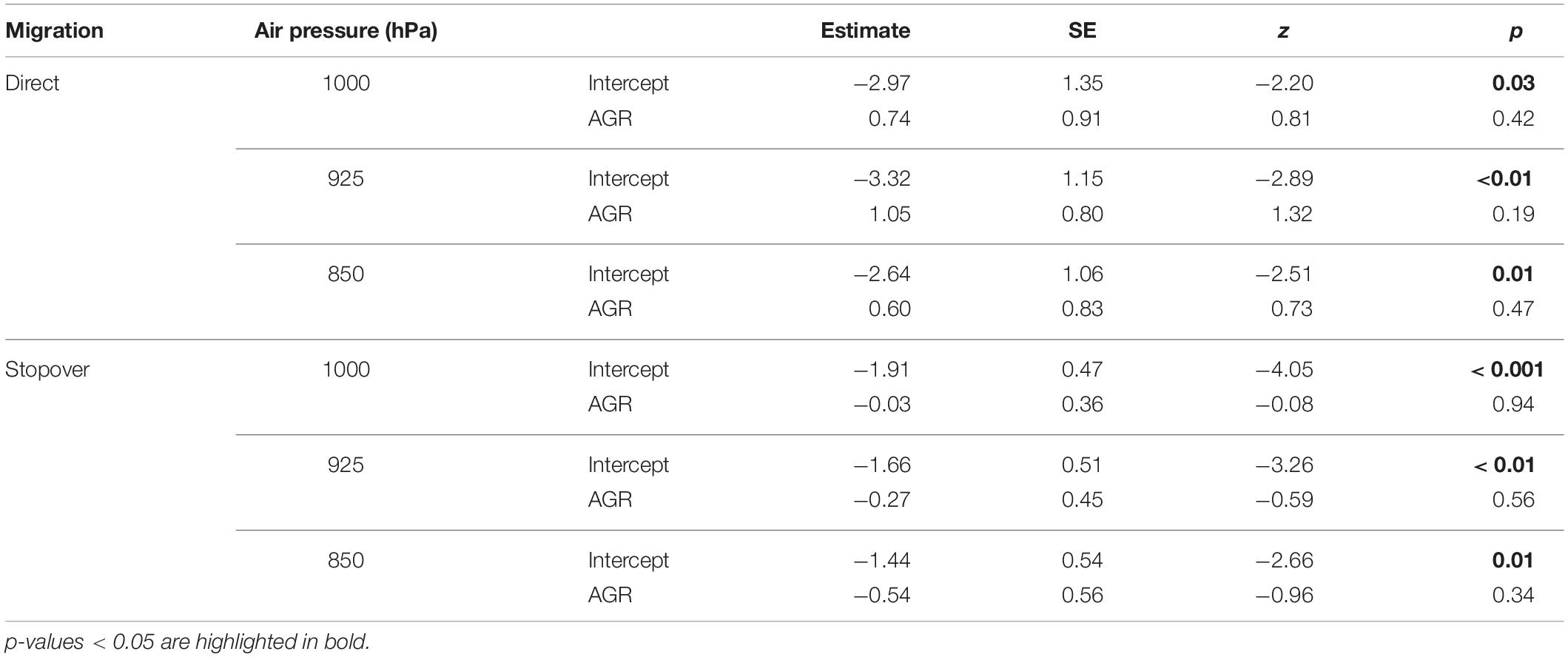
Table 2. Generalized linear models testing the influence of air-to-ground speed ratio (AGR) at the wintering site, on the departure decision of Icelandic whimbrels undertaking a direct (n = 72) or stopover migration (n = 376).
For the three individuals that changed migratory behavior, from direct to stopover (Supplementary Figure S2), the overall pattern of mean AGR, temperature, departure date and zonal winds was similar to that recorded at the population level (Figures 2E–H).
Discussion
During spring migration, Icelandic whimbrels display two main migratory behaviors: a direct flight from the wintering to the breeding sites (direct migration), or two flights with one stopover (stopover migration). We investigated how wind conditions, temperature, and spring departure date may drive individuals to adopt each behavior, and found no differences in wind support during migration, on temperature closer to Iceland or on crosswinds experienced in the region before reaching the main stopover sites. However, departure date from the wintering sites was on average later for individuals performing a direct flight to the breeding areas.
Departure date can be influenced by weather conditions, as birds often select favorable winds (Ma et al., 2011; Gill et al., 2014). Long-distance migrating bar-tailed godwits Limosa lapponica baueri generally experience favorable conditions during the departure period from the wintering sites, creating few opportunities for wind assistance selectivity. Yet, most off-schedule individuals tend to depart when wind assistance is maximized (Conklin and Battley, 2011). In the case of Icelandic whimbrels, wind conditions at departure were not different between migratory behaviors for 1,000 hPa (i.e., 111 m a. s. l.), suggesting that a direct migratory flight is not taken when wind conditions render more support at low altitudes. However, we found differences at higher altitudes (925 hPa and 850 hPa, i.e., 762 and 1,457 m a. s. l, respectively), in which birds migrating directly would experience more impediment of movement than those performing a stopover. Whimbrels have been observed circling and gaining height at departure from the south coast of Iceland in autumn (TGG pers. obs., but see Piersma et al., 1990), indicating that they may sample the wind conditions at different altitudes. However, it seems unlikely that individuals would sample hundreds of meters above sea level and select worse conditions for a longer migratory flight (i.e., direct to Iceland). A study to determine if, and how high, whimbrels sample wind conditions prior to departure, would help clarify if the differences found here have biological meaning. More importantly, wind conditions at departure were not different from those recorded during the previous week, and were predominantly unfavorable, suggesting none to low selectivity for wind assistance. However, other factors may also influence the departure decision of individuals, such as the amount of fuel reserves (Sjöberg et al., 2015), temperature (Schmaljohann et al., 2013; Berchtold et al., 2017) and joining a flock of departing conspecifics, which may be important in orientation during flight (Alerstam et al., 1990).
Given the apparent stable conditions departure and the importance of breeding timing in this system (submitted), the lack of wind selectivity is not surprising and could explain the direct migration when individuals depart later. By undertaking a direct migration, individuals arrive earlier to the breeding sites in relation to undertaking a stopover (unpublished data), which may allow to compensate for a potential delay. However, the laying date does not seem to differ between migratory behaviors (unpublished data), suggesting that a direct migration may in fact not always translate into higher breeding success.
If conditions during spring migration flight are not different between migratory behaviors (until the same region of migration) and there are no clear advantages for an early arrival after a direct migration, then why performing a stopover is predominant across this population? The main stopover sites are in Britain and Ireland, which are relatively close to the breeding sites, in Iceland. Hence, individuals might be able to assess the weather conditions at the breeding locations during stopover, adjust arrival date into Iceland to when those are favorable, and avoid the risk of stochastic inclement weather prior to breeding (Newton, 2006). In fact, temperature at departure from the stopover locations tends to be positively correlated to the temperature in the breeding areas at that time (Spearman r = 0.575, S = 7830, p < 0.001, n = 48) and with the temperature upon arrival (Spearman r = 475, S = 9672, p < 0.001, n = 48), suggesting that if whimbrels depart when weather is good at the stopover site, they are likely to find favorable conditions upon arrival in Iceland. Similar behavior has been suggested for Icelandic black-tailed godwits L. limosa islandica (Gunnarsson and Tómasson, 2011; Alves et al., 2012), that first fly to Britain and Ireland from southern wintering areas in the Iberian Peninsula, before resuming migration to Iceland. Hence, in both species, individuals move to a region where they are likely able to adjust their arrival timing into the breeding sites. Furthermore, given that most individuals stop, a stopover might also allow timing synchronization among couples and the pair bond in the breeding grounds is maintained (Handel and Gill, 2000; Gunnarsson et al., 2004).
Undertaking a stopover might bring other benefits, for example, if individuals can attain a higher resource intake rate at the stopover than at the breeding sites (Alerstam, 2006) and arrive in better body condition (with higher energy reserves) than those that flew directly. Despite later arrival, individuals that make a stopover might then save time at the breeding sites by starting energy-demanding activities quickly (e.g., display flights; Davidson and Evans, 1988; Gudmundsson et al., 1991). On the other hand, a direct migration and early arrival might be beneficial, by guaranteeing that the partner will be met and the pair bond maintained (Ens et al., 1996). However, the relatively lower body condition of direct flight migrants might prevent earlier laying.
At the individual level, for the three birds that changed migratory behavior, the variation in all variables between direct and stopover migrations reflects the patterns also observed at the population level (i.e., tracked individuals). For example, in years when individuals performed a direct flight, departure tended to be later than when a stopover was undertaken (Figure 2). However, the variation within strategy is smaller, as would be expected because Icelandic whimbrels tend to be consistent in their timing, particularly at spring departure (Carneiro et al., 2019b). Given the consistency in spring departure date, the differences between direct and stopover migratory behavior at the individual level, further support the role of departure date in the migratory behavior displayed. Additionally, the fact that individuals only changed from a direct to a stopover migration, hints toward an individual refinement of behavior (Supplementary Figure S3). Since there appears to be no clear advantage from a direct migration, a shorter flight to a stopover site might involve fewer risks due to unpredictable weather conditions at arrival and fuel depletion, while still allowing a timely arrival at the breeding sites.
Our analyses suggest that weather conditions encountered during migration are not the main driver of different spring migratory behavior in Icelandic whimbrels, but these results need to be considered with caution and may require further investigation. First, due to the naturally low prevalence of direct spring migrations, the sample size is skewed toward stopover, which may lead to type II errors, despite the good fit of our models to the data, as assessed by Hosmer & Lemeshow goodness of fit test. Second, in our main statistical models, individual identity was not included as a random effect (see section Materials and Methods), but a reduced model of migratory behavior in function of departure date suggests that individual identity may explain some of the variation in behavior, although the reduced size likely limits detection of the main effect. Nevertheless, despite being highly consistent (Carneiro et al., 2019b), individuals can perform both behaviors and vary in departure time in the same way as the population, e.g., by showing a later departure during direct migrations (Figure 2). Third, given that whimbrels tend to depart in flocks from the wintering sites (Piersma et al., 1990), individual decision may be influenced by group decision. However, there is evidence of flocks breaking at departure (Piersma et al., 1990), suggesting dissimilarity in group and individual behavior. In our dataset, only in four occasions whimbrels may have departed on the same flock (four times two individuals), and in two of those they clearly took different flight routes either due to departing in different flocks, or to the flock breaking up following departure (Supplementary Figure S4). Hence, the effects of weather and departure date are unlikely to be biased by non-independence between tracked individuals. Fourth, there is error associated with locations extracted from geolocators (Phillips et al., 2004; Shaffer et al., 2005; Fudickar et al., 2012), and also on the interpolated weather variables (Kemp et al., 2012a), which may influence the direction and speed calculated, and consequently influencing FA and AGR. However, given the amount of locations during migration available in our dataset, if wind conditions had an important effect, it should still be detected. Fifth, we used FA as the tailwind component, which is a method with few assumptions that ignores perpendicular wind flow in relation to the direction of the birds movement (Kemp et al., 2012b), therefore simplifying a likely more complex behavior. Lastly, as the flight altitude of the individuals tracked here is unknown, we assumed whimbrels fly at constant altitude which restricts the natural dynamics of flight.
Several studies have investigated the role of weather conditions on the decision to depart from stopover locations (e.g., Schaub et al., 2004; Grönroos et al., 2012; Schmaljohann et al., 2013; Packmor et al., 2020), but less has been done concerning their role in the decision to stop during migratory flight (Beekman et al., 2002; Anderson et al., 2019). We add knowledge on the latter, by showing that weather conditions experienced during flight appear to be irrelevant for Icelandic whimbrels, as individuals seem to define a strategy prior to or at departure from the wintering sites. Whether departure date is a cause or a consequence of a direct or stopover spring migration remains to be investigated, but records of whimbrels’ body condition prior to departure from the wintering grounds will likely help to clarify this question.
Data Availability Statement
The datasets generated for this study are available on request to the corresponding author.
Ethics Statement
The studies involving whimbrels were reviewed and approved by the Bird Ringing Unit of the Icelandic Institute of Natural History according to Act no. 64/1994.
Author Contributions
CC performed the analysis and led the writing with substantial discussion and inputs from TG and JA. All authors designed and carried the study, read and approved the final version.
Funding
This work was funded by RANNIS (Grant Nos: 130412-052 and 152470-052), the University of Iceland Research Fund, FCT/MCTES through national funds to CESAM (UIDP/50017/2020 + UIDB/50017/2020), CC (PD/BD/113534/2015), and JA (SFRH/BPD/91527/2012) and ProPolar.
Conflict of Interest
The authors declare that the research was conducted in the absence of any commercial or financial relationships that could be construed as a potential conflict of interest.
Acknowledgments
We are very thankful for the logistic support of the Icelandic Soil Conservation Service, particularly to Anne Bau and Jóna Maria; Verónica Méndez and Borgný Katrínardóttir for fieldwork support; our group members in Iceland and Portugal for fruitful discussions; Kristinn Jónsson for kindly allowing us to work on his land and CM Alcochete for facilities. Last, we are thankful to Cas Eikenaar and Andrea Ferretti for useful suggestions that improved the manuscript.
Supplementary Material
The Supplementary Material for this article can be found online at: https://www.frontiersin.org/articles/10.3389/fevo.2020.00145/full#supplementary-material
Footnotes
References
Alerstam, T. (1979). Wind as selective agent in bird migration. Ornis Scand. 10:76. doi: 10.2307/3676347
Alerstam, T. (2006). Strategies for the transition to breeding in time-selected bird migration. Ardea 94, 347–357.
Alerstam, T., and Gudmundsson, G. A. (1999). Migration patterns of tundra birds: tracking radar observations along the Northeast Passage. Arctic 52, 346–371. doi: 10.14430/arctic941
Alerstam, T., Gudmundsson, G. A., Jonsson, P. E., Karlsson, J., and Lindstrom, A. (1990). Orientation, migration routes and flight behaviour of knots, turnstones and brant geese departing from Iceland in spring. Arctic 43, 201–214. doi: 10.14430/arctic1613
Alerstam, T., and Lindström, Å. (1990). “Optimal bird migration: the relative importance of time, energy, and safety,” in Bird Migration: Physiology and Ecophysiology, ed. E. Gwinner (Berlin: Springer), 331–351. doi: 10.1007/978-3-642-74542-3_22
Alves, J. A., Dias, M. P., Méndez, V., Katrínardóttir, B., and Gunnarsson, T. G. (2016). Very rapid long-distance sea crossing by a migratory bird. Sci. Rep. 6:38154. doi: 10.1038/srep38154
Alves, J. A., Gunnarsson, T. G., Potts, P. M., Gélinaud, G., Sutherland, W. J., and Gill, J. A. (2012). Overtaking on migration: does longer distance migration always incur a penalty? Oikos 121, 464–470. doi: 10.1111/j.1600-0706.2011.19678.x
Anderson, A. M., Duijns, S., Smith, P. A., Friis, C., and Nol, E. (2019). Migration distance and body condition influence shorebird migration strategies and stopover decisions during southbound migration. Front. Ecol. Evol. 7:251. doi: 10.3389/fevo.2019.00251
Arizaga, J., Belda, E. J., and Barba, E. (2011). Effect of fuel load, date, rain and wind on departure decisions of a migratory passerine. J. Ornithol. 152, 991–999. doi: 10.1007/s10336-011-0685-2
Battley, P. F., and Conklin, J. R. (2017). Geolocator wetness data accurately detect periods of migratory flight in two species of shorebird. Wader Study 124, 112–119. doi: 10.18194/ws.00068
Beekman, J. H., Nolet, B. A., and Klaassen, M. (2002). Skipping swans: fuelling rates and wind conditions determine differential use of migratory stopover sites of Bewick’s Swans Cygnus bewickii. Ardea 90, 437–460.
Berchtold, A., Nightingale, I., Vandermeer, C., and MacDougall-Shackleton, S. A. (2017). Experimental temperature manipulations alter songbird autumnal nocturnal migratory restlessness. Anim. Migr. 4, 1–7. doi: 10.1515/ami-2017-0001
Carneiro, C., Gunnarsson, T. G., and Alves, J. A. (2019a). Faster migration in autumn than in spring: seasonal migration patterns and non-breeding distribution of Icelandic whimbrels Numenius phaeopus islandicus. J. Avian Biol. 50, 1–8. doi: 10.1111/jav.01938
Carneiro, C., Gunnarsson, T. G., and Alves, J. A. (2019b). Why are whimbrels not advancing their arrival dates into iceland? exploring seasonal and sex-specific variation in consistency of individual timing during the annual cycle. Front. Ecol. Evol. 7:248. doi: 10.3389/fevo.2019.00248
Chapman, J. W., Klaassen, R. H. G., Drake, V. A., Fossette, S., Hays, G. C., Metcalfe, J. D., et al. (2011). Animal orientation strategies for movement in flows. Curr. Biol. 21, R861–R870. doi: 10.1016/j.cub.2011.08.014
Conklin, J. R., and Battley, P. F. (2011). Impacts of wind on individual migration schedules of New Zealand bar-tailed godwits. Behav. Ecol. 22, 854–861. doi: 10.1093/beheco/arr054
Davidson, N. C., and Evans, P. R. (1988). Prebreeding accumulation of fat and muscle protein by Arctic-breeding shorebirds. Proc. Int. Ornithol. Congr. 19, 342–352.
Dierschke, V., and Delingat, J. (2001). Stopover behaviour and departure decision of northern wheatears, Oenanthe oenanthe, facing different onward non-stop flight distances. Behav. Ecol. Sociobiol. 50, 535–545. doi: 10.1007/s002650100397
Drake, A., Rock, C. A., Quinlan, S. P., Martin, M., and Green, D. J. (2014). Wind speed during migration influences the survival, timing of breeding, and productivity of a neotropical migrant, Setophaga petechia (N Saino, Ed.). PLoS One 9:e97152. doi: 10.1371/journal.pone.0097152
Drent, R. H. (2006). The timing of birds’ breeding seasons: the Perrins hypothesis revisited especially for migrants. Ardea 94, 305–322.
Ens, B. J., Choudhury, S., and Black, J. M. (1996). “Mate fidelity and divorce in monogamous birds,” in Partnerships in Birds: The Study of Monogamy, ed. J. M. Black (Oxford: Oxford University Press), 344–401.
Fudickar, A. M., Wikelski, M., and Partecke, J. (2012). Tracking migratory songbirds: accuracy of light-level loggers (geolocators) in forest habitats. Methods Ecol. Evol. 3, 47–52. doi: 10.1111/j.2041-210X.2011.00136.x
Gill, R. E., Douglas, D. C., Handel, C. M., Tibbitts, T. L., Hufford, G., and Piersma, T. (2014). Hemispheric-scale wind selection facilitates bar-tailed godwit circum-migration of the Pacific. Anim. Behav. 90, 117–130. doi: 10.1016/j.anbehav.2014.01.020
Grönroos, J., Green, M., and Alerstam, T. (2012). To fly or not to fly depending on winds: shorebird migration in different seasonal wind regimes. Anim. Behav. 83, 1449–1457. doi: 10.1016/j.anbehav.2012.03.017
Grönroos, J., Green, M., and Alerstam, T. (2013). Orientation of shorebirds in relation to wind: both drift and compensation in the same region. J. Ornithol. 154, 385–392. doi: 10.1007/s10336-012-0902-7
Gudmundsson, G. A., Lindström, Å, and Alerstam, T. (1991). Optimal fat loads and long-distance flights by migrating Knots Calidris canutus, Sanderlings C. alba and Turnstones Arenaria interpres. IBIS 133, 140–152. doi: 10.1111/j.1474-919X.1991.tb04825.x
Gunnarsson, T. G., Gill, J. A., Sigurbjornsson, T., and Sutherland, W. J. (2004). Arrival synchrony in migratory birds. Nature 413:646. doi: 10.1038/nature03163
Gunnarsson, T. G., and Tómasson, G. (2011). Flexibility in spring arrival of migratory birds at northern latitudes under rapid temperature changes. Bird Study 58, 1–12. doi: 10.1080/00063657.2010.526999
Handel, C. M., and Gill, R. E. (2000). Mate fidelity and breeding site tenacity in a monogamous sandpiper, the black turnstone. Anim. Behav. 60, 471–481. doi: 10.1006/anbe.2000.1505
Hansson, L.-A., and Åkesson, S. (2014). Animal Movement Across Scales. Oxford: Oxford University Press.
Harrison, X. A., Donaldson, L., Correa-Cano, M. E., Evans, J., Fisher, D. N., Goodwin, C. E. D., et al. (2018). A brief introduction to mixed effects modelling and multi-model inference in ecology. PeerJ 6;e4794. doi: 10.7717/peerj.4794
Horton, K. G., Van Doren, B. M., Stepanian, P. M., Hochachka, W. M., Farnsworth, A., and Kelly, J. F. (2016). Nocturnally migrating songbirds drift when they can and compensate when they must. Sci. Rep. 6:21249. doi: 10.1038/srep21249
Kanamitsu, M., Ebisuzaki, W., Woollen, J., Yang, S.-K., Hnilo, J. J., Fiorino, M., et al. (2002). NCEP–DOE AMIP-II Reanalysis (R-2). Bull. Am. Meteorol. Soc. 83, 1631–1644. doi: 10.1175/BAMS-83-11-1631
Katrínardóttir, B., Pálsson, S., Gunnarsson, T. G., and Sigurjónsdóttir, H. (2013). Sexing Icelandic Whimbrels Numenius phaeopus islandicus with DNA and biometrics. Ringing Migr. 28, 43–46. doi: 10.1080/03078698.2013.811160
Kemp, M. U., Emiel van Loon, E., Shamoun-Baranes, J., and Bouten, W. (2012a). RNCEP: global weather and climate data at your fingertips. Methods Ecol. Evol. 3, 65–70. doi: 10.1111/j.2041-210X.2011.00138.x
Kemp, M. U., Shamoun-Baranes, J., van Loon, E. E., McLaren, J. D., Dokter, A. M., and Bouten, W. (2012b). Quantifying flow-assistance and implications for movement research. J. Theor. Biol. 308, 56–67. doi: 10.1016/j.jtbi.2012.05.026
Klaassen, R. H. G., Schlaich, A. E., Bouten, W., and Koks, B. J. (2017). Migrating Montagu’s harriers frequently interrupt daily flights in both Europe and Africa. J. Avian Biol. 48, 180–190. doi: 10.1111/jav.01362
La Sorte, F. A., and Fink, D. (2017). Projected changes in prevailing winds for transatlantic migratory birds under global warming. J. Anim. Ecol. 86, 273–284. doi: 10.1111/1365-2656.12624
La Sorte, F. A., Horton, K. G., Nilsson, C., and Dokter, A. M. (2018). Projected changes in wind assistance under climate change for nocturnally migrating bird populations. Glob. Chang. Biol. 25, 589–601. doi: 10.1111/gcb.14531
Liechti, F. (2006). Birds: blowin’ by the wind? J. Ornithol. 147, 202–211. doi: 10.1007/s10336-006-0061-9
Ma, Z., Hua, N., Zhang, X., Guo, H., Zhao, B. I. N., Ma, Q., et al. (2011). Wind conditions affect stopover decisions and fuel stores of shorebirds migrating through the south Yellow Sea. IBIS 2011, 755–767. doi: 10.1111/j.1474-919x.2011.01164.x
Morrison, C. A., Alves, J. A., Gunnarsson, T. G., Thorisson, B., and Gill, J. A. (2019). Why do earlier-arriving migratory birds have better breeding success? Ecol. Evol. 9, 8856–8864. doi: 10.1002/ece3.5441
Newton, I. (2006). Can conditions experienced during migration limit the population levels of birds? J. Ornithol. 147, 146–166. doi: 10.1007/s10336-006-0058-4
Packmor, F., Klinner, T., Woodworth, B. K., Eikenaar, C., and Schmaljohann, H. (2020). Stopover departure decisions in songbirds: do long-distance migrants depart earlier and more independently of weather conditions than medium-distance migrants? Mov. Ecol. 8, 1–14. doi: 10.1186/s40462-020-0193-1
Perrins, C. M. (1970). The timing of birds breeding seasons. IBIS 112, 242–255. doi: 10.1111/j.1474-919X.1970.tb00096.x
Phillips, R. A., Silk, J. R. D., Croxall, J. P., Afanasyev, V., and Briggs, D. R. (2004). Accuracy of geolocation estimates for flying seabirds. Mar. Ecol. Prog. Ser. 266, 265–272. doi: 10.3354/meps266265
Piersma, T., Zwarts, L., and Bruggemann, J. H. (1990). Behavioural aspects of the departure of waders before long-distance flights: flocking, vocalizations, flight paths and diurnal timing. Ardea 78, 157–184.
R Core Team (2018). R: A Language and Environment for Statistical Computing. Vienna: R Foundation for Statistical Computing.
Schaub, M., Liechti, F., and Jenni, L. (2004). Departure of migrating European robins, Erithacus rubecula, from a stopover site in relation to wind and rain. Anim. Behav. 67, 229–237. doi: 10.1016/j.anbehav.2003.03.011
Schmaljohann, H., Fox, J. W., and Bairlein, F. (2012). Phenotypic response to environmental cues, orientation and migration costs in songbirds flying halfway around the world. Anim. Behav. 84, 623–640. doi: 10.1016/j.anbehav.2012.06.018
Schmaljohann, H., Korner-Nievergelt, F., Naef-Daenzer, B., Nagel, R., Maggini, I., Bulte, M., et al. (2013). Stopover optimization in a long-distance migrant: the role of fuel load and nocturnal take-off time in Alaskan northern wheatears (Oenanthe oenanthe). Front. Zool. 10:26. doi: 10.1186/1742-9994-10-26
Senner, N. R., Stager, M., Verhoeven, M. A., Cheviron, Z. A., Piersma, T., and Bouten, W. (2018). High-altitude shorebird migration in the absence of topographical barriers: avoiding high air temperatures and searching for profitable winds. Proc. R. Soc. B Biol. Sci. 285:20180569. doi: 10.1098/rspb.2018.0569
Shaffer, S. A., Tremblay, Y., Awkerman, J. A., Henry, R. W., Teo, S. L. H., Anderson, D. J., et al. (2005). Comparison of light- and SST-based geolocation with satellite telemetry in free-ranging albatrosses. Mar. Biol. 147, 833–843. doi: 10.1007/s00227-005-1631-8
Shamoun-Baranes, J., Leyrer, J., van Loon, E., Bocher, P., Robin, F., Meunier, F., et al. (2010). Stochastic atmospheric assistance and the use of emergency staging sites by migrants. Proc. Biol. Sci. 277, 1505–1511. doi: 10.1098/rspb.2009.2112
Shamoun-Baranes, J., Liechti, F., and Vansteelant, W. M. G. (2017). Atmospheric conditions create freeways, detours and tailbacks for migrating birds. J. Comp. Physiol. A Neuroethol. Sensory, Neural, Behav. Physiol. 203, 509–529. doi: 10.1007/s00359-017-1181-9
Sjöberg, S., Alerstam, T., Åkesson, S., Schulz, A., Weidauer, A., Coppack, T., et al. (2015). Weather and fuel reserves determine departure and flight decisions in passerines migrating across the Baltic Sea. Anim. Behav. 104, 59–68. doi: 10.1016/j.anbehav.2015.02.015
Thorup, K., Alerstam, T., Hake, M., and Kjellén, N. (2006). Traveling or stopping of migrating birds in relation to wind: an illustration for the osprey. Behav. Ecol. 17, 497–502. doi: 10.1093/beheco/arj054
Watts, B. D., Smith, F. M., and Truitt, B. R. (2017). Departure patterns of Whimbrels using a terminal spring staging area. Wader Study 124, 141–146. doi: 10.18194/ws.00075
Keywords: Numenius phaeopus, whimbrel, migration, migration strategy, wind, wader, shorebird, flight behavior
Citation: Carneiro C, Gunnarsson TG and Alves JA (2020) Linking Weather and Phenology to Stopover Dynamics of a Long-Distance Migrant. Front. Ecol. Evol. 8:145. doi: 10.3389/fevo.2020.00145
Received: 22 February 2020; Accepted: 28 April 2020;
Published: 04 June 2020.
Edited by:
Andreas Nord, Lund University, SwedenReviewed by:
Cas Eikenaar, Institute of Avian Research, GermanyAndrea Ferretti, University of Vienna, Austria
Copyright © 2020 Carneiro, Gunnarsson and Alves. This is an open-access article distributed under the terms of the Creative Commons Attribution License (CC BY). The use, distribution or reproduction in other forums is permitted, provided the original author(s) and the copyright owner(s) are credited and that the original publication in this journal is cited, in accordance with accepted academic practice. No use, distribution or reproduction is permitted which does not comply with these terms.
*Correspondence: Camilo Carneiro, Y2FtaWxvZmNhcm5laXJvQGdtYWlsLmNvbQ==