- 1Department of Biology, Program in Ecology, Evolution and Conservation Biology, University of Nevada, Reno, NV, United States
- 2Xerces Society for Invertebrate Conservation, Portland, OR, United States
- 3Department of Ecology & Evolutionary Biology, The University of Tennessee, Knoxville, TN, United States
- 4Department of Entomology, Cornell University, Ithaca, NY, United States
Monarch butterflies (Danaus plexippus) are in decline in the western United States and are encountering a range of anthropogenic stressors. Pesticides are among the factors that likely contribute to this decline, although the concentrations of these chemicals in non-crop plants are not well documented, especially in complex landscapes with a diversity of crop types and land uses. In this study, we collected 227 milkweed (Asclepias spp.) leaf samples from 19 sites representing different land use types across the Central Valley of California. We also sampled plants purchased from two stores that sell plants to home gardeners. We found 64 pesticides (25 insecticides, 27 fungicides, and 11 herbicides, as well as 1 adjuvant) out of a possible 262 in our screen. Pesticides were detected in every sample, even at sites with little or no pesticide use based on information from landowners. On average, approximately 9 compounds were detected per plant across all sites, with a range of 1–25 compounds in any one sample. For the vast majority of pesticides detected, we do not know the biological effects on monarch caterpillars that consume these plants; however, we did detect a few compounds for which effects on monarchs have been experimentally investigated. Chlorantraniliprole in particular was identified in 91% of our samples and found to exceed a tested LD50 for monarchs in 58 out of 227 samples. Our primary finding is the ubiquity of pesticides in milkweeds in an early summer window of time that monarch larvae are likely to be present in the area. Thus, these results are consistent with the hypothesis that pesticide exposure could be a contributing factor to monarch declines in the western United States. This highlights the need for a greater understanding of both the lethal and sublethal effects of these compounds (individually, additively, and synergistically) and suggests the urgent need for strategies that reduce pesticide use and movement on the landscape.
Introduction
Widespread reports of declining insect populations have received considerable and increasing attention in recent years (Forister et al., 2010; Potts et al., 2010; Hallmann et al., 2017; Janzen and Hallwachs, 2019; Sánchez-Bayo and Wyckhuys, 2019; Wepprich et al., 2019). The causes of this phenomenon are multi-faceted, as species face correlated anthropogenic stressors that include climate change, habitat loss, and the use of pesticides (Deutsch et al., 2008; Goulson et al., 2015; Forister et al., 2019; Sánchez-Bayo and Wyckhuys, 2019). While the importance of each of these drivers will vary with context, just one or a combination of factors can disrupt population dynamics and lead to extirpation or extinction (Brook et al., 2008; Tylianakis et al., 2008; Potts et al., 2010; González-Varo et al., 2013). One potentially devastating combination of stressors is the historical loss of habitat to agricultural intensification and the contemporary use of pesticides on modified lands (Gibbs et al., 2009). To better understand the contribution of pesticides to long-term trends in insect populations, especially in heavily converted landscapes, we must identify the diversity of compounds, quantify their concentrations, and test how these affect insect survival and performance. Here we investigate the suite of pesticides that potentially contaminate milkweeds in the Central Valley of California, a large agricultural and urban landscape. It is our intention that the results reported here will provide critical data on field-realistic concentrations of pesticides in modified landscapes in order to better parameterize laboratory experiments on pesticide toxicity affecting non-target organisms.
Pesticides have long been discussed as drivers of ecosystem disruption and insect declines, especially in the context of agriculture (Epstein, 2014). Conventional agriculture employs a wide range of pesticides (including herbicides, insecticides, and fungicides) which can affect both target and non-target species (Abbes et al., 2015; Pisa et al., 2015). Insecticides and fungicides can have direct effects on insects (Sanchez-Bayo and Goka, 2014; Mulé et al., 2017), while herbicides are most often associated with indirect effects by altering the nearby plant community and floral resources; however, some recent research indicates that certain herbicides can also have direct effects on insects (Egan et al., 2014; Balbuena et al., 2015; Dai et al., 2018; Motta et al., 2018). Recently, much attention has been paid to neonicotinoids, a class of anticholinergic insecticides, whose use has dramatically increased over the past 20 years, such that they are now the most widely used class of insecticide in the world (Wood and Goulson, 2017). Neonicotinoids are water soluble and readily taken up by plant tissues, posing a risk to non-target insects as they can be found in all plant parts, including leaves, pollen, and nectar (Bonmatin et al., 2015; Wood and Goulson, 2017). Much research has focused on their impacts on bees (Whitehorn et al., 2012); however, their use is also associated with declines of dragonflies in Japan (Nakanishi et al., 2020), butterflies in Europe (Gilburn et al., 2015), and butterflies in the Central Valley (Forister et al., 2016). While individual pesticides can have lethal and sub-lethal effects (Pisa et al., 2015), plants sampled in agricultural landscapes often contain multiple compounds (Krupke et al., 2012; Olaya-Arenas and Kaplan, 2019). The literature on the additive or synergistic effects of pesticide combinations on non-target organisms is sparse; however, particular combinations have been shown to behave synergistically in insects broadly (Iwasa et al., 2004; Ahmed and Matsumura, 2013) and pest Lepidoptera specifically (Jones et al., 2012; Liu et al., 2018; Chen et al., 2019). By focusing on one or a few select pesticides or even a single class of pesticides, the realized risk of these chemicals on non-target insects is likely being underestimated.
Perhaps the most noted recent decline of any insect is that of the monarch butterfly (Danaus plexippus), whose reduced numbers have been observed in both the eastern (Stenoien et al., 2018) and western (Espeset et al., 2016; Schultz et al., 2017) North American populations. In the eastern United States, many hypotheses have been proposed to explain the monarch decline, including loss of critical overwintering habitat, natural enemies, climate, and various pesticides, especially herbicides, that have reduced milkweed abundance (Asclepias spp.) (Belsky and Joshi, 2018). In the west, monarch overwintering populations reached a historic low in 2018 (Pelton et al., 2019), and the causes appear to include loss of overwintering habitat and pesticides (Crone et al., 2019). There are few studies evaluating the direct (lethal and sub-lethal) effects of pesticides on the monarch (Krischik et al., 2015; Pecenka and Lundgren, 2015; James, 2019; Krishnan et al., 2020). Pecenka and Lundgren tested the toxicity of the neonicotinoid clothianidin and observed it in sub-lethal concentrations in milkweeds sampled in South Dakota, United States (Pecenka and Lundgren, 2015). Krischik et al. (2015) and James (2019) both assessed the effects of imidacloprid on monarchs. Krishnan et al. (2020) investigated the toxicity of five compounds on larval monarchs, including chlorantraniliprole, imidacloprid, and thiamethoxam, and found chlorantraniliprole to be highly toxic compared to imidacloprid and thiamethoxam. Further work in the mid-western United States sampled milkweeds and screened leaf samples for pesticides (Olaya-Arenas and Kaplan, 2019). A total of 14 pesticides were identified at various concentrations, including clothianidin, which was found in similar concentrations as those reported by Pecenka and Lundgren (2015). While these findings show that pesticides can be found at physiologically relevant concentrations in milkweeds in the eastern United States, we currently lack an understanding of pesticide contamination in the west and thus have no direct way to assess the potential contribution of pesticides to the decline of the western monarch.
The Central Valley of California is the largest cropped agricultural landscape of the western United States and is part of the migratory distribution and breeding ground for the western population of the monarch butterfly. Historically, one of the primary anthropogenic stressors in the Central Valley has been the loss of wetland habitat to agricultural intensification (Reiter et al., 2015). This change to the landscape reduced floral resources and introduced pesticides to large portions of the landscape (Wagner, 2019). While a major contributor, agriculture is not the only source of pesticides in the environment as pesticides are commonly sold for home and garden use (Atwood and Paisley-Jones, 2017). Over the past three decades, the Sacramento Valley, the largest metropolitan area in the Central Valley, has become increasingly developed (Theobald, 2005), and this urban growth may represent a second major source of contaminants in the region (Weston et al., 2009). Considering the history of the region, monarchs and other native and beneficial insects may be encountering a heterogeneous and toxic chemical landscape.
In this study, we measured the concentration and diversity of pesticides found in Asclepias spp. leaves collected in the Central Valley of California. Over 4 days in late June of 2019, we sampled leaves from different land use types, including agriculture, wildlife refuges, urban parks and gardens, and plants sold in retail nurseries. The first objective of this study is to gather a snapshot picture of which pesticides are present on the landscape and in what concentrations they are found when monarch larvae are expected to be feeding. Second, we present an exploratory examination of contamination differences among land use types. Finally, we ask if the contamination levels detected could harm monarchs or other terrestrial insects, based on published data. Thus, this study is designed as a first look into what pesticides monarch larvae might be exposed to in the Central Valley and not to directly test if they are responsible for the ongoing decline of the western population.
Materials and Methods
Milkweed Sampling
Milkweed samples of Asclepias fascicularis (161 samples) and Asclepias speciosa (50), with fewer Asclepias eriocarpa (4) and Asclepias curassavica (12), were collected from sites in the Central Valley and purchased from retail nurseries from June 24 to 27, 2019 (Figure 1A). Our collection time was intended to overlap with monarch breeding in the Central Valley based on personal observations and historical data (Espeset et al., 2016). In total, we collected samples from 19 different sites: five sites were located in conventional farms, one in an organic farm, one in a milkweed establishment trial (grown for restoration), one in a roadside location (adjacent to an agriculture field), five in wildlife refuges, four in urban areas, and two from retail nurseries. Many of the agriculture sites are part of a Xerces Society project to implement on-farm invertebrate conservation and have made an effort to avoid bee-toxic pesticides. All agricultural locations (including the restoration trial and the roadside location) were treated in analyses as “agriculture” (since replication was not sufficient to parse further); thus, our main land use type categories were “agriculture,” “refuge,” “retail,” and “urban.” Sites were selected opportunistically, based on accessibility and in order to sample a diversity of landscapes. The identity of milkweed species is mostly confounded with sampling location (Supplementary Table S1), so our inferential ability is limited for differences in contamination among plant species. If sites contained fewer than 20 plants, all plants were surveyed, and if sites contained greater than 20 plants, individual plants were selected randomly within each patch, and leaf samples were collected and placed in bags. Clippers were cleaned with rubbing alcohol between cuttings. Samples were transported on ice, frozen and stored, and ultimately shipped to the Cornell University Chemical Ecology Core Facility lab on dry ice for analysis.
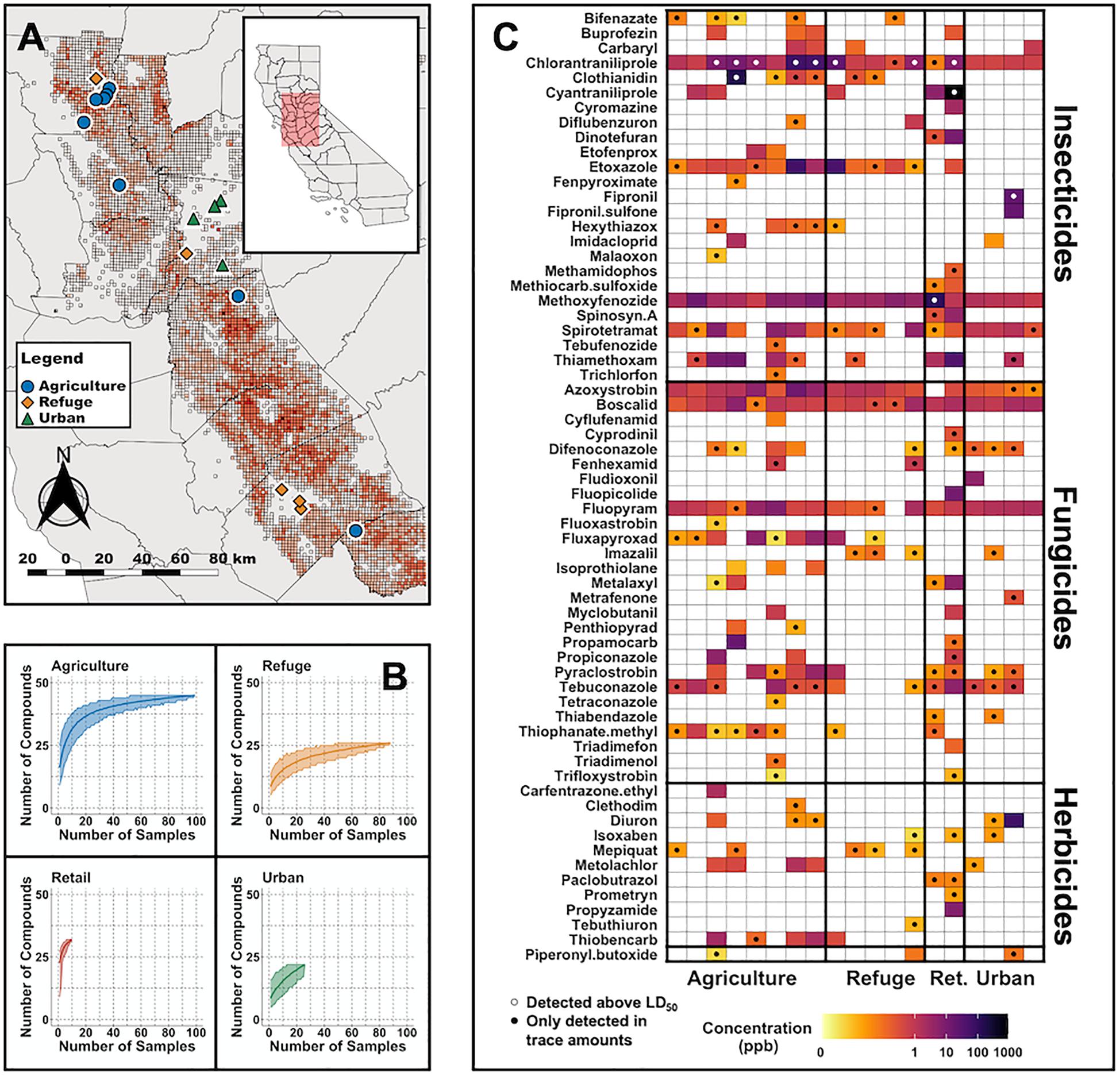
Figure 1. Overview of pesticide compounds and concentrations detected in the Central Valley. (A) Sampling locations colored by land use type. Red background indicates the number of compounds reported in the 2015–2017 California Department of Pesticide Regulation pesticide use data (the range is from 1 compound for the lightest gray to 113 for the darkest red cells). (B) Rarefaction curves for the number of pesticides detected by land use type. (C) Mean concentrations (per plant) of compounds at each site (see also Supplementary Table S7). Values are shown in parts per billion on a log scale. Black circles indicate compounds only detected in trace amounts (i.e., below the level of quantification). White circles indicate compounds found above a lepidopteran LD50.
Chemistry
Frozen milkweed leaves were extracted by a modified version of the EN 15662 QuEChERS procedure (European Committee for Standardization, 2008) and screened for 262 pesticides (including some metabolites and breakdown products) by liquid chromatography mass spectrometry (LC-MS/MS). Five grams of frozen leaves (5 grams was the target sample weight, samples ranged from 0.35 to 5.07 grams and were prepared accordingly) were mixed with 7 mL of acetonitrile and 5 mL of water. The leaves were then homogenized for 1 min using ceramic beads (2.8 mm diameter) and a Bead Ruptor 24 (OMNI International, United States). After complete homogenization, 6.5 mg of EN 15662 salts were added (4 g MgSO4; 1 g NaCl; 1 g sodium citrate tribasic dihydrate; 0.5 g sodium citrate dibasic sesquihydrate). Samples were then shaken and centrifuged at 7300 × g for 5 min. One milliliter of supernatant was collected and transferred into a d-SPE (dispersive solid phase extraction) tube containing 150 mg PSA and 900 mg MgSO4. After the d-SPE step, 496 μL of supernatant was collected and 4 μL of a solution of five internal standards spanning a wide range of polarity (d4-imidacloprid 0.07 ng/μL; d10-chlorpyrifos 0.2 ng/μL: d7-bentazon 0.1 ng/μL; d5-atrazine 0.02 ng/μL; d7-propamocarb 0.1 ng/μL) was added. Samples were then filtered (0.22 μm, PTFE) and stored at −20°C before analysis.
Sample analysis was carried out with a Vanquish Flex UHPLC system (Dionex Softron GmbH, Germering, Germany) coupled with a TSQ Quantis mass spectrometer (Thermo Scientific, San Jose, CA, United States). The UHPLC was equipped with an Accucore aQ column (100 mm × 2.1 mm, 2.6 μm particle size). The mobile phase consisted of (A) Methanol/Water (2:98, v/v) with 5 mM ammonium formate and 0.1% formic acid and (B) Methanol/Water (98:2, v/v) with 5 mM ammonium formate and 0.1% formic acid. The temperature of the column was maintained at 25°C throughout the run and the flow rate was 300 μL/min. The elution program was the following: 1.5 min equilibration (0% B) prior to injection, 0–0.5 min (0% B, isocratic), 0.5–7 min (0–70% B, linear gradient), 7–9 min (70–100% B, linear gradient), 9–12 min (100% B, column wash), 12–12.1 min (100–0% B, linear gradient), 12.1–14.5 min (0% B, re-equilibration). The flow from the LC was directed to the mass spectrometer through a Heated Electrospray probe (H-ESI). The settings of the H-ESI were: spray voltage 3700 V for positive mode and 2500 V for negative mode, Sheath gas 35 (arbitrary unit), Auxiliary gas 8 (arbitrary unit), Sweep gas 1 (arbitrary unit), Ion transfer tube temperature 325°C, Vaporizer temperature 350°C.
The MS/MS detection was carried out using the selected reaction monitoring (SRM) mode. Two transitions were monitored for each compound: one for quantification and the other for confirmation. The SRM parameters for each individual pesticide are summarized in Supplementary Table S2. The resolution of both Q1 and Q3 was set at 0.7 FWHM, the cycle time was 0.5 s, and the pressure of the collision gas (argon) was set at 2 mTorr.
Statistical Analyses
The chemical screening was able to classify concentrations into four categories. The first was when the chemical was below the level of detection and these were treated as zeros. Second was when the chemical was detected, but the concentrations were too low to be quantified, these samples were labeled as “trace.” In these cases, we used a known lower limit of detection for the observed value. Third was if the chemical could be detected and quantified. Finally, there were a few cases in which chemicals were found in too high of concentrations to be quantified. In these cases, we used the upper limit of detection as the observed value. The lower and upper limits of detection are known values which vary by compound; thus, even if a compound was only found in trace amounts, we can still draw some inference about relative concentrations.
Sampling sites were classified into agricultural, retail, refuge, or urban for statistical analysis, as described above. To examine total pesticide richness and diversity in each land use type, we performed sample-based rarefaction. To directly compare compositional differences in pesticides between different land use types, we calculated the effective number of pesticides for each sample using different Hill numbers (q = 0, q = 1, and q = 2). Using this approach to diversity, the sensitivity to rare compounds changes as a function of the parameter q: q = 0 weights all compounds equally (richness), q = 1 weights all compounds by their relative abundance (exponential of Shannon entropy), and q = 2 down-weights rarer compounds (inverse Simpson’s index) (Hill, 1973; Jost, 2006). We also performed this same diversity analysis, but on data that were rarefied to match the land use type with the lowest sampling effort (retail, 11 samples).
Dissimilarity of pesticides detected among milkweeds from each of the land use types was then visualized using a distance-based redundancy analysis (dbRDA) (Legendre and Legendre, 2012). The distance matrix was constructed using the quantitative generalization of Jaccard dissimilarity (Ružička index) with land use types as the constraining factors (Schubert and Telcs, 2014). The dbRDA was implemented using the R package vegan v2.5-4 (Oksanen et al., 2019). Associations between each pesticide and land use types were examined using the group-equalized point serial correlation (De Cáceres and Legendre, 2009). We explored associations allowing pesticides to be indicative of combinations of land use types. Statistical significance (α = 0.05) of the strongest association for each pesticide with land use types was determined using 9999 permutations of the data. These indicator analyses were conducted using functions from the R package indicspecies (De Cáceres et al., 2020).
Literature Search
To examine biological importance of the detected concentrations, we compared our findings to published LD50 data for honeybees and Lepidoptera. LD50 data (both contact and oral where available) for honeybees were collected from EPA records in the ECOTOX and PubChem databases and the University of Hertfordshire’s Pesticide Properties Database (Supplementary Table S3). One strength of these data is their standardized collection and thus ease of use for comparison across compounds in examining collective (or additive) effects. To do this, we calculated the hazard quotient for each compound, by dividing the detected concentration by the LD50, and then summed this across all compounds in each sample (Stoner et al., 2019). This approach has an important drawback in that it assumes all lethal effects are additive when that may not be the case, as residue combinations could result in either less toxicity (antagonism) or more toxicity (synergism) (Zhu et al., 2014). Additionally, while the EPA uses honeybees as a surrogate species for other insect pollinators in pesticide risk assessments, these data are not directly applicable to lepidopterans and many other insects. Furthermore, toxicity tests are performed on adult honeybees which are, of course, different from caterpillars, and this is especially true considering that some insecticides are designed specifically to affect caterpillars. Thus, we only use the honeybee LD50 data in the most general sense to establish a benchmark of concentrations where these compounds could have a biological effect on non-target terrestrial invertebrates. To better apply our findings directly to the monarch butterfly, we also conducted a literature review of papers that have studied the compounds we detected and have reported LD50 concentrations for lepidopterans (Supplementary Table S4). The literature search was performed in January 2020 using ISI Web of Science with the terms (lepidopt∗ OR butterfl∗ OR moth∗) and (compound) and was repeated for all compounds identified in our samples.
Results
A total of 64 compounds were identified in at least one leaf sample out of 262 possible compounds in our test panel. Of these, 25 were insecticides (including two insecticide metabolites), 27 were fungicides, 11 were herbicides, and 1 was a common adjuvant (Figure 1C). An adjuvant is a compound designed to enhance the effect of other compounds. Seven compounds were detected in over 50% of collected samples and 17 compounds were detected in over 10% of samples. Methoxyfenozide and chlorantraniliprole were the most prevalent compounds, which were found in 96% and 91% of samples, respectively. Detected concentrations across all compounds range from below 1 ppb to above 900 ppb. In some samples, compounds were detected, but the concentration was too low to be quantified (Figure 1C). In these cases, we used the limit of detection value for that pesticide, as the actual concentration would be above the limit of detection but below the limit of quantification.
Generally, higher numbers of pesticides (distinct compounds) were found in agricultural and retail samples than refuge or urban samples; however, we detected considerable variation among plants and locations, and pesticides were present in all land use types (Figures 1B, 2, and Supplementary Figure S1). Diversity analyses suggest especially high numbers of compounds in retail samples, and this appears to be driven by “rare” compounds (found in only one or a few samples), as effective numbers of compounds dramatically decline between Hill numbers generated at q = 0 and q = 1 (Figure 2). The other three land use types contained fewer rare pesticides and were similar to each other in the proportion of rare compounds. This pattern is maintained even when samples are rarefied to match the low sampling effort of the retail samples (Supplementary Figure S1). There was substantial variation in the mean number of compounds among milkweed species; however, as previously noted, species are confounded with sampling sites as most sites had only one species present (Supplementary Figure S2). This is especially true for A. curassavica and A. eriocarpa, which were almost exclusively found in retail and agricultural sites, respectively (Supplementary Table S1). When examining site dissimilarity across all compounds, there is clustering based on land use type in ordination space (Figure 3). In general, retail and agricultural samples are the most similar, but there are also refuge sites that are chemically similar to agriculture and retail sites (Figure 3). Many specific chemicals are associated with agricultural sites including chlorantraniliprole, clothianidin, imidacloprid, and azoxystrobin (Supplementary Figure S3 and Table 1). Methoxyfenozide and thiamethoxam are associated with retail samples; however, it is important to note the low sample size of retail compared to other land use types. We have stronger evidence supporting associations with agriculture than associations with retail.
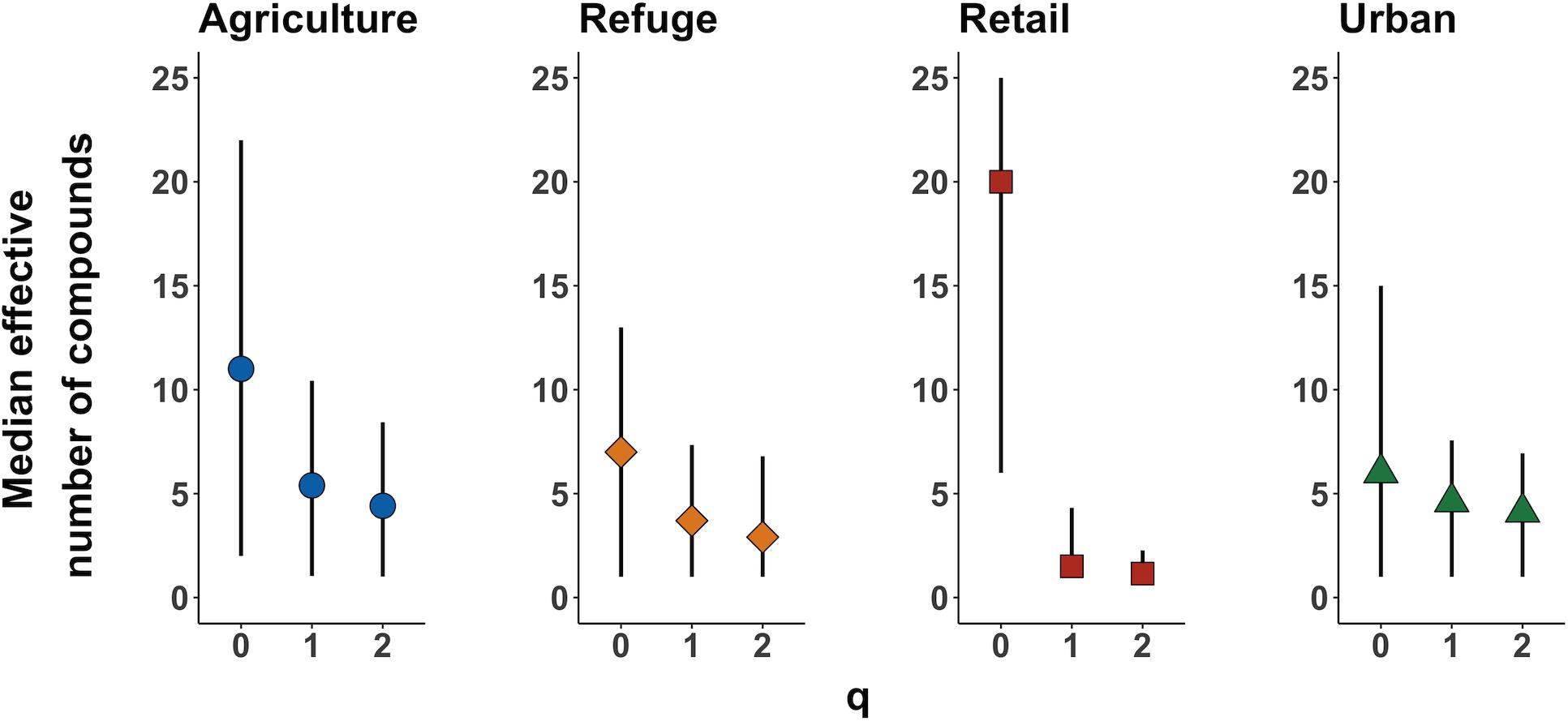
Figure 2. Effective numbers of pesticides per sample by land use type using Hill numbers generated across a range of q-values that place different weights on rare versus common compounds (at q = 0 all compounds have equal weight and higher q-values place more weight on relatively abundant compounds, see text for additional details). Points show the median number of compounds per sample. Bars show the full range across samples within one land use type. The values shown here are estimates of pesticide diversity; for concentrations, see Figure 1C.
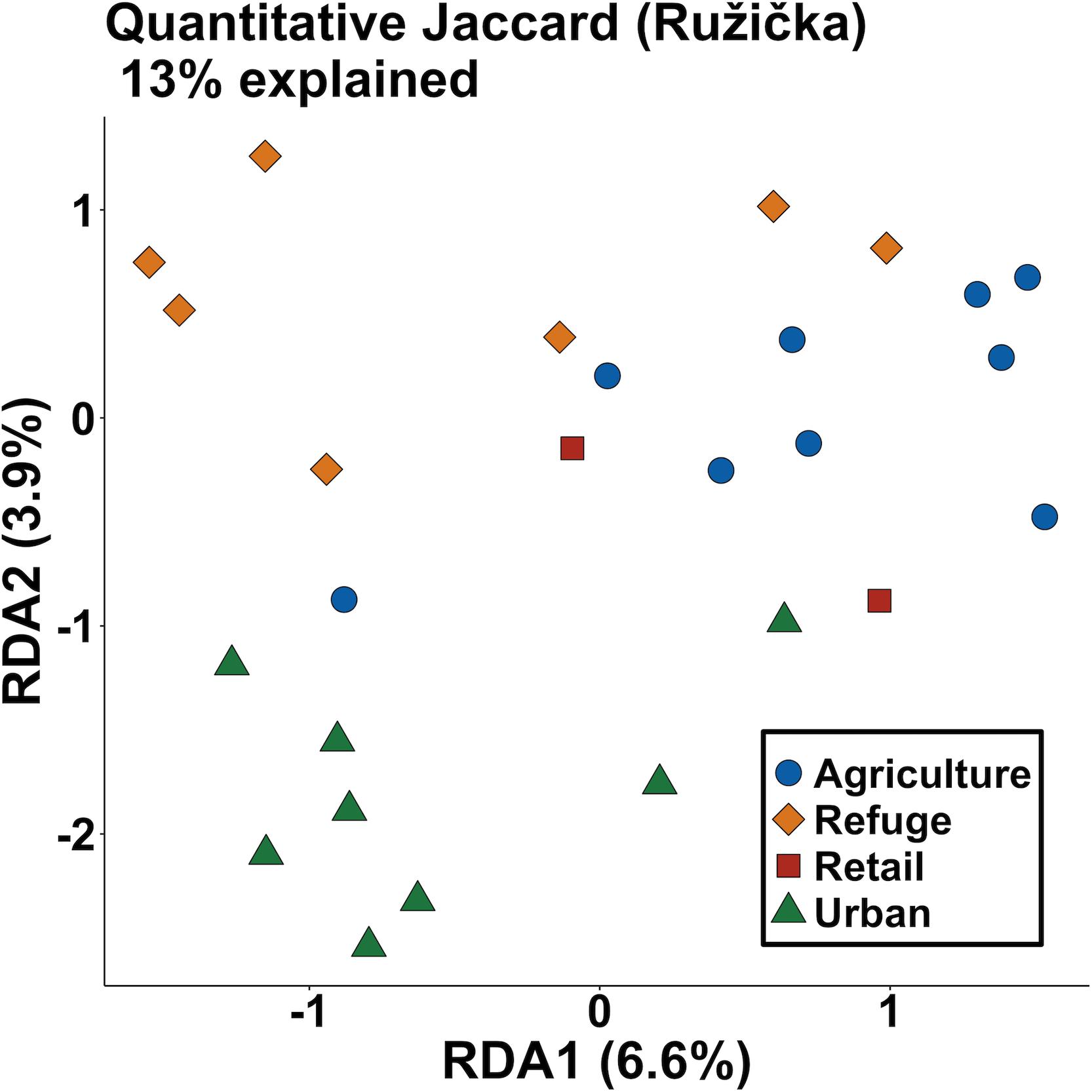
Figure 3. Ordination of the constrained axes from distance-based redundancy analysis based upon chemical dissimilarity between sampling sites (variation explained by axis indicated after each axis label). Points indicate the mean score for each sampling site; colors and shapes indicate land use type. Points that are close in ordination space have similar pesticide compositions.
Of the 64 detected compounds, we acquired contact and oral honeybee LD50 concentrations for 62 compounds (data were not available for the two insecticide metabolites). When looking at each compound individually, there were 27 exceedances of a contact LD50 and 52 exceedances of an oral LD50. These 79 total exceedances were from 5 compounds and occurred in 36 individual plant samples (out of 227) from seven sites. Calculating collective risk across all detected compounds in a sample (by dividing the observed value by the LD50 and then summing across the sample) identified the same 36 samples, and thus, it appears that single compounds are driving the exceedances of honeybee LD50 concentrations. These samples primarily came from agricultural or retail samples; however, one urban backyard sample also exceeded an oral LD50. Information about exceedances of specific compounds can be found in Supplementary Table S5.
The literature search for Lepidoptera and pesticides generated 44 studies with published lethal doses for the compounds we detected (Supplementary Table S4). Pest species dominated the literature as only eight non-pest papers (including the four aforementioned monarch papers) were found. The majority of compounds had none or a single study. Reported LD50 concentrations for a compound often varied between lepidopteran species by multiple orders of magnitude. Generally, insecticides had lower LD50 values (and thus are more directly toxic) than fungicides and herbicides. An additional axis of variation in the literature was exposure time, which varied from under 30 min to 3 weeks; however, the range of 24–72 h was most common. Using the published lepidopteran data, 47% of samples exceeded published LD50 values for a lepidopteran. Of these, 68% (32% of all samples) contained a pesticide above a published LD50 for monarchs. These exceedances were observed in 10 sites across all land use types; however, agriculture and refuge contained the highest number of raw exceedances (they are also the most sampled) (Figure 1B). The most notable individual compound is chlorantraniliprole, which was found above a published LD50 for monarchs in 26% of all samples and above an LD10 in 78% of all samples. Clothianidin was recorded above a monarch LD50 in 15 samples (and above the LD90 in 11); however, these all came from one agricultural site. Other compounds that exceeded an LD50 were cyantraniliprole, fipronil, and methoxyfenozide which came from retail and urban samples. A full overview of all of the exceedances and their associated land use type can be seen in Figure 1 and in a Supplementary Table S6.
Discussion
Insects are facing many stressors simultaneously, especially in areas where habitat has already been converted from a natural state and fragmented. Identifying various stressors and quantifying their implications for population dynamics are critical for fully understanding how insects are responding to the Anthropocene. In the Central Valley, pesticides likely represent an important stressor, as they were detected in all land use types sampled. Agricultural and retail samples tended to have more compounds in higher concentrations; however, our choice of sampling locations was not random, nor comprehensive, and thus, our ability to make direct land use type comparisons is limited. In general, we suspect that our results may be conservative. Agricultural samples were primarily collected from farmers who are already working with the Xerces Society to implement on-farm invertebrate conservation, many of whom have made an effort to avoid bee-toxic pesticides. Likewise, the backyard samples were taken at the homes of Xerces employees where pesticides have not knowingly been applied recently. Still, both of our backyard sites had pesticide detections, including one site with residues from an application of fipronil made more than 6 years before sampling. Numerous pesticides were also detected in wildlife refuges, although some herbicides known to be used on portions of the refuges were not detected. All of the refuges sampled are surrounded by agricultural fields. In combination with the backyard samples, this demonstrates the presence of pesticides in areas where they are not expected or generally used and are likely coming from adjacent areas.
Another reason to suspect that our results are conservative comes from the chemical screening process itself. There are several pesticides that would likely have been identified if they had been part of the panel that was used in screening. Pyrethroid insecticides, including bifenthrin, and some fungicides, including chlorothalonil, could not be detected with the lab methods used, but are commonly applied to crops in the Central Valley and are toxic to non-target insects (Wolfenbarger et al., 2008). Overall, the clearest pattern in these data is the ubiquity of pesticide presence in milkweeds across the Central Valley, which may impact local and migratory insects (monarch caterpillars are not the only insects that interact with these plants) as they are very likely being exposed to many contaminants. This is true whether a caterpillar is consuming a milkweed leaf in a wildlife refuge, a backyard, or near a conventional agricultural field.
While compounds and concentrations were highly variable, a few notable pesticides warrant further discussion. Chlorantraniliprole was the second most common pesticide, identified in 91% of samples. In the counties we sampled, chlorantraniliprole is most commonly applied to tree nut crops (including almond, pistachio, and walnut) with most applications during May, June, July, and August (California Department of Pesticide Regulation, 2019). Krishnan et al. (2020) recently studied the toxicity of this specific compound in different instars of monarchs. They found chlorantraniliprole to be highly toxic when compared to imidacloprid and thiamethoxam. Chlorantraniliprole’s LD50 was lowest (and thus most toxic) in second instar caterpillars. The number of exceedances we report for this compound used this second instar value. We also found a high number of exceedances of the reported LD10 for second instars. These lower doses are often used as a benchmark for sub-lethal effects (Perveen, 2000; Hummelbrunner and Isman, 2001), thus raising the possibility that the majority of our samples contained residues of chlorantraniliprole that could impact the biology of the overall monarch population, while not directly causing mortality. Clothianidin was detected well above lethal concentrations for larval monarchs at one site. It is interesting to note that we have anecdotally linked this finding to an application in the weeks preceding sampling by the landowner to a nearby field, thus providing further evidence of movement of compounds on the landscape. Another compound of note was methoxyfenozide, which was the most frequently detected compound across samples. This compound is an insect growth regulator that targets caterpillars and is most commonly applied to tree nuts and wine grapes with the heaviest use during May, June, and July in the counties we sampled (California Department of Pesticide Regulation, 2019). Methoxyfenozide accelerates molting in lepidopteran species, and while they have not been directly tested, monarch butterflies have been predicted to be susceptible to this class of pesticides (LaLone et al., 2014).
There are some notable caveats when applying the above studies to our findings. First, these studies exposed caterpillars at various instars and for different exposure times. It is not clear how an LD50 of one compound over 36 h compares with an LD50 of a different compound over 48 h, and what either of these can tell us about risk in the field. A larval monarch will consume a plant for much longer than 48 h, and generally longer exposure times will decrease survival (Abivardi et al., 1999; Yue et al., 2003; Wang et al., 2009, 2013; Nasr et al., 2010; Rehan and Freed, 2015; Ahmed et al., 2016; Liu et al., 2018). Thus, considering shorter exposure times is likely to be a conservative approach which underestimates risk. We should also consider temporal issues from the perspective of plants. Pesticides are not static in leaves and concentrations will dissipate over time. The half-lives for some of these compounds have been investigated in different plants and there is high variation (Fantke and Juraske, 2013; Fantke et al., 2014). Reported half-lives range from shorter than a day to longer than the life of a monarch caterpillar. Given that the LD50 values we obtained have shorter exposure lengths compared to the feeding time of monarch caterpillars, these LD50 values may better account for reduced exposure due to pesticide turnover in plant tissue. Additionally, our sampling timing certainly impacted the chemicals and concentrations we found. It is likely that we would have detected different pesticides had we sampled in late July or August instead of June. While we specifically planned our timing to be during the period that a larval monarch could be present in the Central Valley, monarch larvae can be found from spring through summer. A final point of uncertainty worth noting is behavior: monarchs are known to express oviposition preferences among different species of milkweed (Pocius et al., 2018), but it is currently unknown whether pesticide contamination can be a factor in this decision. Despite these uncertainties, we think that these reported LD50 concentrations and the exceedances across land use types offer compelling evidence that certain compounds are being found at biologically meaningful concentrations, with possible regicidal (or sub-regicidal) implications for larval monarchs in the Central Valley.
With the exception of the already mentioned compounds, we are not able to speculate how the concentrations we detected for most compounds directly impact larval monarchs. Overall, most of the concentrations we observed were below reported LD50 values for other lepidopterans and honeybees; however, there are numerous reasons why most reported LD50 values may not be reliable for monarchs or other non-target butterflies and moths. The vast majority of studies on the compounds we detected are focused on lepidopteran pest species, and many of these studies investigate lethal concentrations on populations suspected to display pesticide resistance. A study on a resistant population will inflate the reported lethal doses, and thus, these studies likely do not reflect the risk of pesticides for non-target insects. Additionally, most studies have the same exposure time drawback already discussed, namely short exposures. This common experimental design is ideal for determining the potency of a chemical; however, it is not a good benchmark for understanding the risk these contaminants pose to non-target insects in the field. Chronic exposure studies are more applicable for this question. It is critical that future research continues to quantify the toxicity of these compounds, especially for field realistic concentrations and exposure times, for monarchs and other insects for which we currently have no data.
Moving beyond individual compounds, these findings raise the possibility of harmful effects from combinations of multiple compounds, even if each is present at low levels. We explored collective (or additive) effects of compounds using honeybee data, which are highly standardized and allow for comparison of compounds within one sample. High risk samples were typically driven by a single compound in high concentration with little contribution from all of the others. We have already stated the assumed additive relationship of this calculation and the lack of applicability of bee data for caterpillars (especially since we only assayed leaves), but this nevertheless allowed for some quantification of collective effects. This does not mean that the low concentration of many compounds is not important, as they could act additively, synergistically, or antagonistically. There are far fewer studies on interactions of multiple compounds; however, synergistic effects have been identified in Lepidoptera for thiamethoxam, chlorantraniliprole, imidacloprid, and methoxyfenozide (Jones et al., 2012; Liu et al., 2018; Chen et al., 2019), all of which we detected. These findings suggest possible negative effects on lepidopterans; however, it is clear that more research is needed to understand the synergistic effects of field-relevant concentrations on non-target insects.
This is now the second study in the past year that has found pesticide contamination in milkweeds that could be used by breeding monarchs. Olaya-Arenas and Kaplan (2019) also found that pesticides were present in milkweed samples collected near agricultural fields in the mid-western United States. That study found a total of 14 compounds; however, the authors screened for different and fewer compounds than this study. When directly comparing 30 compounds that both studies looked for, Olaya-Arenas and Kaplan found 12 compounds while we detected 14 out of 30. This result is unexpected as that study was concentrated in corn and soybean fields, while our study covered many different land use types and agricultural areas with higher crop diversity. That study collected more than five times as many samples over 2 years, which may account for the similar number of compounds despite less land use diversity. Similar to our study, Olaya-Arenas and Kaplan were not able to definitively conclude that the pesticides they observed are negatively impacting monarchs, as we currently lack the appropriate data; however, it is likely that monarch caterpillars are encountering biologically meaningful concentrations of these contaminants in the landscape.
Pesticides are frequently discussed as a driver of insect declines, which have been reported in the Central Valley for butterflies in general (Forister et al., 2016) and for monarchs in particular (Espeset et al., 2016). Notably, while monarchs are in decline in the region, many other butterfly species show even steeper declines (Nice et al., 2019). We are not suggesting that pesticides are solely responsible or even the most important factor in these declines; however, our findings demonstrate the potential for pesticides to play a role. Insecticides, fungicides, and herbicides were found in milkweeds at all sampling sites, even in locations we know have not been directly treated. Compounds were also detected in milkweeds purchased from commercial suppliers used by the general public for plantings intended to support butterfly conservation. We are not aware if our findings apply to other butterfly host plants in the region; however, given our knowledge that many of these exposures are caused by off-site movement, similar contamination can be expected on other plants found throughout this highly developed landscape. Much more research will be needed to understand how these different concentrations impact monarchs (and other pollinators and beneficial insects), and we hope that our data provide a useful starting place for future experimental designs. We also hope that the results presented here emphasize the need for additional research on practices that reduce pesticide use and movement across landscapes with many uses, including habitat for native insects.
Data Availability Statement
The datasets generated for this study are available on request to the corresponding author.
Author Contributions
CH, SH, and other Xerces staff collected the samples. NB performed the chemical analysis. JF, CH, and MF performed statistical analyses. All authors wrote the manuscript.
Funding
This work was funded through the generous support of Linda S. Raynolds, who donated to the Xerces Society. MF was supported by a Trevor James McMinn professorship.
Conflict of Interest
The authors declare that the research was conducted in the absence of any commercial or financial relationships that could be construed as a potential conflict of interest.
Acknowledgments
We thank Linda S. Raynolds for the generous support of this project; Maggie Douglas for help in gathering the bee toxicity data; and Jaret Daniels, Tom Dilts, Ian Kaplan, Christy Leppanen, and Beth Pringle for discussion of results. We also thank the two reviewers for their helpful comments and suggestions.
Supplementary Material
The Supplementary Material for this article can be found online at: https://www.frontiersin.org/articles/10.3389/fevo.2020.00162/full#supplementary-material
References
Abbes, K., Biondi, A., Kurtulus, A., Ricupero, M., Russo, A., Siscaro, G., et al. (2015). Combined non-target effects of insecticide and high temperature on the parasitoid Bracon nigricans. PLoS One 10:e0138411. doi: 10.1371/journal.pone.0138411
Abivardi, C., Weber, D. C., and Dorn, S. (1999). Effects of carbaryl and cyhexatin on survival and reproductive behaviour of Cydia pomonella (Lepidoptera: Tortricidae). Ann. Appl. Biol. 134, 143–151. doi: 10.1111/j.1744-7348.1999.tb05250.x
Ahmed, M. A. I., and Matsumura, F. (2013). Synergistic actions of formamidine insecticides on the activity of pyrethroids and neonicotinoids against Aedes aegypti (Diptera: Culicidae). J. Med. Entomol. 49, 1405–1410. doi: 10.1603/me12030
Ahmed, M. A. I., Temerak, S. A. H., Abdel-Galil, F. A. K., and Manna, S. H. M. (2016). Susceptibility of field and laboratory strains of cotton leafworm, Spodoptera littoralis (Boisd.) (Lepidoptera: Noctuidae) to spinosad pesticide under laboratory conditions. Plant Prot. Sci. 52, 128–133. doi: 10.17221/5/2015-PPS
Atwood, D., and Paisley-Jones, C. (2017). Pesticides Industry Sales and Usage 2008-2012 Market Estimates. Washington, DC: United States Environmental Protection Agency.
Balbuena, M. S., Tison, L., Hahn, M. L., Greggers, U., Menzel, R., and Farina, W. M. (2015). Effects of sublethal doses of glyphosate on honeybee navigation. J. Exp. Biol. 218, 2799–2805. doi: 10.1242/jeb.117291
Belsky, J., and Joshi, N. K. (2018). Assessing role of major drivers in recent decline of monarch butterfly population in North America. Front. Environ. Sci. 6:86. doi: 10.3389/fenvs.2018.00086
Bonmatin, J. M., Giorio, C., Girolami, V., Goulson, D., Kreutzweiser, D. P., Krupke, C., et al. (2015). Environmental fate and exposure; neonicotinoids and fipronil. Environ. Sci. Pollut. Res. 22, 35–67. doi: 10.1007/s11356-014-3332-7
Brook, B. W., Sodhi, N. S., and Bradshaw, C. J. A. (2008). Synergies among extinction drivers under global change. Trends Ecol. Evol. 28, 453–460. doi: 10.1016/j.tree.2008.03.011
California Department of Pesticide Regulation (2019). Summary of Pesticide Use Report Data - 2017. Sacramento, CA: California Department of Pesticide Regulation.
Chen, J., Jiang, W., Hu, H., Ma, X., Li, Q., Song, X., et al. (2019). Joint toxicity of methoxyfenozide and lufenuron on larvae of Spodoptera exigua Hübner (Lepidoptera: Noctuidae). J. Asia Pac. Entomol. 22, 795–801. doi: 10.1016/j.aspen.2019.06.004
Crone, E. E., Pelton, E. M., Brown, L. M., Thomas, C. C., and Schultz, C. B. (2019). Why are monarch butterflies declining in the West? Understanding the importance of multiple correlated drivers. Ecol. Appl. 29:e01975. doi: 10.1002/eap.1975
Dai, P., Yan, Z., Ma, S., Yang, Y., Wang, Q., Hou, C., et al. (2018). The herbicide glyphosate negatively affects midgut bacterial communities and survival of honey bee during larvae reared in vitro. J. Agric. Food Chem. 66, 7786–7793. doi: 10.1021/acs.jafc.8b02212
De Cáceres, M., Jansen, F., and Dell, N. (2020). indicspecies: Relationship Between Species and Groups of Sites. Available online at: https://cran.r-project.org/package=indicspecies (accessed February 4, 2020).
De Cáceres, M., and Legendre, P. (2009). Associations between species and groups of sites: indices and statistical inference. Ecology 90, 3566–3574. doi: 10.1890/08-1823.1
Deutsch, C. A., Tewksbury, J. J., Huey, R. B., Sheldon, K. S., Ghalambor, C. K., Haak, D. C., et al. (2008). Impacts of climate warming on terrestrial ectotherms across latitude. Proc. Natl. Acad. Sci. U.S.A. 105, 6668–6672. doi: 10.1073/pnas.0709472105
Egan, J. F., Bohnenblust, E., Goslee, S., Mortensen, D., and Tooker, J. (2014). Herbicide drift can affect plant and arthropod communities. Agric. Ecosyst. Environ. 185, 77–87. doi: 10.1016/j.agee.2013.12.017
Epstein, L. (2014). Fifty years since silent spring. Annu. Rev. Phytopathol. 52, 377–402. doi: 10.1146/annurev-phyto-102313-045900
Espeset, A. E., Harrison, J. G., Shapiro, A. M., Nice, C. C., Thorne, J. H., Waetjen, D. P., et al. (2016). Understanding a migratory species in a changing world: climatic effects and demographic declines in the western monarch revealed by four decades of intensive monitoring. Oecologia 181, 819–830. doi: 10.1007/s00442-016-3600-y
European Committee for Standardization (2008). Foods of Plant Origin -Determination of Pesticide Residues Using GC-MS and/or LC-MS/MS Following Acetonitrile Extraction/Partitioning and Cleanup by Dispersive SPE—QuEChERS-Method. Brussels: European Committee for Standardization.
Fantke, P., Gillespie, B. W., Juraske, R., and Jolliet, O. (2014). Estimating half-lives for pesticide dissipation from plants. Environ. Sci. Technol. 48, 8588–8602. doi: 10.1021/es500434p
Fantke, P., and Juraske, R. (2013). Variability of pesticide dissipation half-lives in plants. Environ. Sci. Technol. 47, 3548–3562. doi: 10.1021/es303525x
Forister, M. L., Cousens, B., Harrison, J. G., Anderson, K., Thorne, J. H., Waetjen, D., et al. (2016). Increasing neonicotinoid use and the declining butterfly fauna of lowland California. Biol. Lett. 12:20160475. doi: 10.1098/rsbl.2016.0475
Forister, M. L., McCall, A. C., Sanders, N. J., Fordyce, J. A., Thorne, J. H., O’Brien, J., et al. (2010). Compounded effects of climate change and habitat alteration shift patterns of butterfly diversity. Proc. Natl. Acad. Sci. U.S.A. 107, 2088–2092. doi: 10.1073/pnas.0909686107
Forister, M. L., Pelton, E. M., and Black, S. H. (2019). Declines in insect abundance and diversity: we know enough to act now. Conserv. Sci. Pract. 1:e80. doi: 10.1111/csp2.80
Gibbs, K. E., MacKey, R. L., and Currie, D. J. (2009). Human land use, agriculture, pesticides and losses of imperiled species. Divers. Distrib. 15, 242–253. doi: 10.1111/j.1472-4642.2008.00543.x
Gilburn, A. S., Bunnefeld, N., Wilson, J. M., Botham, M. S., Brereton, T. M., Fox, R., et al. (2015). Are neonicotinoid insecticides driving declines of widespread butterflies? PeerJ 3:e1402. doi: 10.7717/peerj.1402
González-Varo, J. P., Biesmeijer, J. C., Bommarco, R., Potts, S. G., Schweiger, O., Smith, H. G., et al. (2013). Combined effects of global change pressures on animal-mediated pollination. Trends Ecol. Evol. 28, 524–530. doi: 10.1016/j.tree.2013.05.008
Goulson, D., Nicholls, E., Botías, C., and Rotheray, E. L. (2015). Bee declines driven by combined stress from parasites, pesticides, and lack of flowers. Science 347:1255957. doi: 10.1126/science.1255957
Hallmann, C. A., Sorg, M., Jongejans, E., Siepel, H., Hofland, N., Schwan, H., et al. (2017). More than 75 percent decline over 27 years in total flying insect biomass in protected areas. PLoS One 12:e0185809. doi: 10.1371/journal.pone.0185809
Hill, M. O. (1973). Diversity and evenness: a unifying notation and its consequences. Ecology 54, 427–432. doi: 10.2307/1934352
Hummelbrunner, L. A., and Isman, M. B. (2001). Acute, sublethal, antifeedant, and synergistic effects of monoterpenoid essential oil compounds on the tobacco cutworm, Spodoptera litura (Lep., Noctuidae). J. Agric. Food Chem. 49, 715–720. doi: 10.1021/jf000749t
Iwasa, T., Motoyama, N., Ambrose, J. T., and Roe, R. M. (2004). Mechanism for the differential toxicity of neonicotinoid insecticides in the honey bee, Apis mellifera. Crop Prot. 23, 371–378. doi: 10.1016/j.cropro.2003.08.018
James, D. G. (2019). A neonicotinoid insecticide at a rate found in nectar reduces longevity but not oogenesis in monarch. Insects 10:E276. doi: 10.3390/insects10090276
Janzen, D. H., and Hallwachs, W. (2019). Perspective: where might be many tropical insects? Biol. Conserv. 223, 102–108. doi: 10.1016/j.biocon.2019.02.030
Jones, M. M., Robertson, J. L., and Weinzierl, R. A. (2012). Toxicity of thiamethoxam and mixtures of chlorantraniliprole plus acetamiprid, esfenvalerate, or thiamethoxam to neonates of oriental fruit moth (Lepidoptera: Tortricidae). J. Econ. Entomol. 105, 1426–1431. doi: 10.1603/ec11349
Krischik, V., Rogers, M., Gupta, G., and Varshney, A. (2015). Soil-applied imidacloprid translocates to ornamental flowers and reduces survival of adult Coleomegilla maculata, Harmonia axyridis, and Hippodamia convergens lady beetles, and larval Danaus plexippus and Vanessa cardui butterflies. PLoS One 10:e0119133. doi: 10.1371/journal.pone.0119133
Krishnan, N., Zhang, Y., Bidne, K. G., Hellmich, R. L., Coats, J. R., and Bradbury, S. P. (2020). Assessing field-scale risks of foliar insecticide applications to monarch butterfly (Danaus plexippus) larvae. Environ. Toxicol. Chem. 39, 923–941. doi: 10.1002/etc.4672
Krupke, C. H., Hunt, G. J., Eitzer, B. D., Andino, G., and Given, K. (2012). Multiple routes of pesticide exposure for honey bees living near agricultural fields. PLoS One 7:e29268. doi: 10.1371/journal.pone.0029268
LaLone, C., Villeneuve, D., Kristina, G., Tollefsen, K., and Ankley, G. (2014). Conservation of lepidopteran ecdysteroid receptor provides evidence for butterfly susceptibility to diacylhydrazine and bisacylhydrazine chemicals. Soc. Environ. Toxicol. Chem.
Legendre, P., and Legendre, L. (2012). Numerical Ecology. Developments in Environmental Modeling. New York, NY: Springer.
Liu, Y., Zhang, H., He, F., Li, X., Tan, H., and Zeng, D. (2018). Combined toxicity of chlorantraniliprole, lambda-cyhalothrin, and imidacloprid to the silkworm Bombyx mori (Lepidoptera: Bombycidae). Environ. Sci. Pollut. Res. 25, 22598–22605. doi: 10.1007/s11356-018-2374-7
Motta, E. V. S., Raymann, K., and Moran, N. A. (2018). Glyphosate perturbs the gut microbiota of honey bees. Proc. Natl. Acad. Sci. U.S.A. 115, 10305–10310. doi: 10.1073/pnas.1803880115
Mulé, R., Sabella, G., Robba, L., and Manachini, B. (2017). Systematic review of the effects of chemical insecticides on four common butterfly families. Front. Environ. Sci. 5:32. doi: 10.3389/fenvs.2017.00032
Nakanishi, K., Uéda, T., Yokomizo, H., and Hayashi, T. I. (2020). Effects of systemic insecticides on the population dynamics of the dragonfly Sympetrum frequens in Japan: statistical analyses using field census data from 2009 to 2016. Sci. Total Environ. 703:134499. doi: 10.1016/j.scitotenv.2019.134499
Nasr, H. M., Badawy, M. E. I., and Rabea, E. I. (2010). Toxicity and biochemical study of two insect growth regulators, buprofezin and pyriproxyfen, on cotton leafworm Spodoptera littoralis. Pestic. Biochem. Physiol. 98, 198–205. doi: 10.1016/j.pestbp.2010.06.007
Nice, C. C., Forister, M. L., Harrison, J. G., Gompert, Z., Fordyce, J. A., Thorne, J. H., et al. (2019). Extreme heterogeneity of population response to climatic variation and the limits of prediction. Glob. Change Biol. 25, 2127–2136. doi: 10.1111/gcb.14593
Oksanen, J., Blanchet, F. G., Friendly, M., Kindt, R., Legendre, P., McGlinn, D., et al. (2019). vegan: Community Ecology Package. Available online at: https://cran.r-project.org/package=vegan (accessed September 1, 2019).
Olaya-Arenas, P., and Kaplan, I. (2019). Quantifying pesticide exposure risk for monarch caterpillars on milkweeds bordering agricultural land. Front. Ecol. Evol. 7:223. doi: 10.3389/fevo.2019.00223
Pecenka, J. R., and Lundgren, J. G. (2015). Non-target effects of clothianidin on monarch butterflies. Sci. Nat. 102:19. doi: 10.1007/s00114-015-1270-y
Pelton, E. M., Schultz, C. B., Jepsen, S. J., Black, S. H., and Crone, E. E. (2019). Western monarch population plummets: status, probable causes, and recommended conservation actions. Front. Ecol. Evol. 7:258. doi: 10.3389/fevo.2019.00258
Perveen, F. (2000). Sublethal effects of chlorfluazuron on reproductivity and viability of Spodoptera litura (F.) (Lep., Noctuidae). J. Appl. Entomol. 124, 223–231. doi: 10.1046/j.1439-0418.2000.00468.x
Pisa, L. W., Amaral-Rogers, V., Belzunces, L. P., Bonmatin, J. M., Downs, C. A., Goulson, D., et al. (2015). Effects of neonicotinoids and fipronil on non-target invertebrates. Environ. Sci. Pollut. Res. 22, 68–102. doi: 10.1007/s11356-014-3471-x
Pocius, V. M., Debinski, D. M., Pleasants, J. M., Bidne, K. G., and Hellmich, R. L. (2018). Monarch butterflies do not place all of their eggs in one basket: oviposition on nine Midwestern milkweed species. Ecosphere 9:e02064. doi: 10.1002/ecs2.2064
Potts, S. G., Biesmeijer, J. C., Kremen, C., Neumann, P., Schweiger, O., and Kunin, W. E. (2010). Global pollinator declines: trends, impacts and drivers. Trends Ecol. Evol. 25, 345–353. doi: 10.1016/j.tree.2010.01.007
Rehan, A., and Freed, S. (2015). Fitness cost of methoxyfenozide and the effects of its sublethal doses on development, reproduction, and survival of Spodoptera litura (Fabricius) (Lepidoptera: Noctuidae). Neotrop. Entomol. 44, 513–520. doi: 10.1007/s13744-015-0306-5
Reiter, M. E., Wolder, M. A., Isola, J. E., Jongsomjit, D., Hickey, C. M., Carpenter, M., et al. (2015). Local and landscape habitat associations of shorebirds in wetlands of the Sacramento valley of California. J. Fish Wildl. Manag. 6, 29–43. doi: 10.3996/012014-JFWM-003
Sanchez-Bayo, F., and Goka, K. (2014). Pesticide residues and bees - A risk assessment. PLoS One 9:e94482. doi: 10.1371/journal.pone.0094482
Sánchez-Bayo, F., and Wyckhuys, K. A. G. (2019). Worldwide decline of the entomofauna: a review of its drivers. Biol. Conserv. 232, 8–27. doi: 10.1016/j.biocon.2019.01.020
Schubert, A., and Telcs, A. (2014). A note on the Jaccardized Czekanowski similarity index. Scientometrics 98, 1397–1399. doi: 10.1007/s11192-013-1044-2
Schultz, C. B., Brown, L. M., Pelton, E., and Crone, E. E. (2017). Citizen science monitoring demonstrates dramatic declines of monarch butterflies in western North America. Biol. Conserv. 214, 343–346. doi: 10.1016/j.biocon.2017.08.019
Stenoien, C., Nail, K. R., Zalucki, J. M., Parry, H., Oberhauser, K. S., and Zalucki, M. P. (2018). Monarchs in decline: a collateral landscape-level effect of modern agriculture. Insect Sci. 25, 528–541. doi: 10.1111/1744-7917.12404
Stoner, K. A., Cowles, R. S., Nurse, A., and Eitzer, B. D. (2019). Tracking pesticide residues to a plant genus using palynology in pollen trapped from honey bees (Hymenoptera: Apidae) at ornamental plant nurseries. Environ. Entomol. 48, 351–362. doi: 10.1093/ee/nvz007
Theobald, D. M. (2005). Landscape patterns of exurban growth in the USA from 1980 to 2020. Ecol. Soc. 10:32.
Tylianakis, J. M., Didham, R. K., Bascompte, J., and Wardle, D. A. (2008). Global change and species interactions in terrestrial ecosystems. Ecol. Lett. 11, 1351–1363. doi: 10.1111/j.1461-0248.2008.01250.x
Wagner, S. (2019). Study 310: Surface Water Monitoring for Pesticides in Agricultural Areas of Northern California, 2019. Sacramento, CA: Department of Pesticide Regulation.
Wang, D., Gong, P., Li, M., Qiu, X., and Wang, K. (2009). Sublethal effects of spinosad on survival, growth and reproduction of Helicoverpa armigera (Lepidoptera: Noctuidae). Pest Manag. Sci. 65, 223–227. doi: 10.1002/ps.1672
Wang, D., Wang, Y.-M., Liu, H.-Y., Xin, Z., and Xue, M. (2013). Lethal and sublethal effects of spinosad on Spodoptera exigua (Lepidoptera: Noctuidae). J. Econ. Entomol. 106, 1825–1831. doi: 10.1603/ec12220
Wepprich, T., Adrion, J. R., Ries, L., Wiedmann, J., and Haddad, N. M. (2019). Butterfly abundance declines over 20 years of systematic monitoring in Ohio, USA. PLoS One 14:e0216270. doi: 10.1371/journal.pone.0216270
Weston, D. P., Holmes, R. W., and Lydy, M. J. (2009). Residential runoff as a source of pyrethroid pesticides to urban creeks. Environ. Pollut. 157, 287–294. doi: 10.1016/j.envpol.2008.06.037
Whitehorn, P. R., O’connor, S., Wackers, F. L., and Goulson, D. (2012). Neonicotinoid pesticide reduces bumble bee colony growth and queen production. Science 336, 351–352. doi: 10.5061/dryad.1805c973
Wolfenbarger, L. L. R., Naranjo, S. E., Lundgren, J. G., Bitzer, R. J., and Watrud, L. S. (2008). Bt crop effects on functional guilds of non-target arthropods: a meta-analysis. PLoS One 3:e2118. doi: 10.1371/journal.pone.0002118
Wood, T. J., and Goulson, D. (2017). The environmental risks of neonicotinoid pesticides: a review of the evidence post 2013. Environ. Sci. Pollut. Res. 24, 17285–17325. doi: 10.1007/s11356-017-9240-x
Yue, B., Wilde, G. E., and Arthur, F. (2003). Evaluation of thiamethoxam and imidacloprid as seed treatments to control European corn borer and Indianmeal moth (Lepidoptera: Pyralidae) Larvae. J. Econ. Entomol. 96, 503–509. doi: 10.1093/jee/96.2.503
Keywords: monarch, milkweed, pesticides, non-target insects, butterflies
Citation: Halsch CA, Code A, Hoyle SM, Fordyce JA, Baert N and Forister ML (2020) Pesticide Contamination of Milkweeds Across the Agricultural, Urban, and Open Spaces of Low-Elevation Northern California. Front. Ecol. Evol. 8:162. doi: 10.3389/fevo.2020.00162
Received: 09 March 2020; Accepted: 08 May 2020;
Published: 08 June 2020.
Edited by:
Laura A. Brannelly, The University of Melbourne, AustraliaReviewed by:
Francisco Sánchez-Bayo, The University of Sydney, AustraliaJacob Pecenka, Purdue University, United States
Copyright © 2020 Halsch, Code, Hoyle, Fordyce, Baert and Forister. This is an open-access article distributed under the terms of the Creative Commons Attribution License (CC BY). The use, distribution or reproduction in other forums is permitted, provided the original author(s) and the copyright owner(s) are credited and that the original publication in this journal is cited, in accordance with accepted academic practice. No use, distribution or reproduction is permitted which does not comply with these terms.
*Correspondence: Matthew L. Forister, Zm9yaXN0ZXJAZ21haWwuY29t