- 1State Key Laboratory for Conservation and Utilization of Bio-Resources in Yunnan, School of Life Sciences, Yunnan University, Kunming, China
- 2Kunming Institute of Zoology, Chinese Academy of Sciences, Kunming, China
- 3Kunming College of Life Science, University of Chinese Academy of Sciences, Kunming, China
- 4Shanxi Institute of Zoology, Xi’an, China
- 5Shanxi Key Laboratory for Animal Conservation, Xi’an, China
- 6Gene Bank of Primates and Primate Genetics Laboratory, German Primate Center, Leibniz Institute for Primate Research, Göttingen, Germany
- 7Department of Laboratory Medicine and Pathobiology, University of Toronto, Toronto, ON, Canada
The research of phenotypic convergence is of increasing importance in adaptive evolution. Locomotory modes play important roles in the adaptive evolution of species in the Euarchontoglires, however, the investigation of convergent evolution of the locomotory modes across diverse Euarchontoglire orders is incomplete. We collected measurements of three phalangeal indices of manual digit III, including metacarpal of digit III (MC3), manus proximal phalanx of digit III (MPP3), and manus intermediate phalanx of digit III (MIP3), from 203 individuals of 122 Euarchontoglires species representing arboreal (orders Scandentia, Rodentia, and Primates), terrestrial (orders Scandentia and Rodentia), and gliding (orders Dermoptera and Rodentia) locomotory modes. This data can be separated into seven groups defined by order and locomotory mode. Based on combination of the three phalangeal indices, the Principle component analyses (PCA), phylomorphospace plot, and C-metrics analyses clustered the arboreal species of Scandentia, Rodentia, and Primates together and the terrestrial species of Scandentia and Rodentia together, showing the convergent signal in evolution of the arboreal (C1 = 0.424, P < 0.05) and terrestrial (C1 = 0.560, P < 0.05) locomotory modes in Euarchontoglires. Although the gliding species from Dermoptera and Rodentia did not cluster together, they also showed the convergent signal (C1 = 0.563, P < 0.05). Our work provides insight into the convergent evolution of locomotory modes in Euarchontoglires, and reveals that these three indices contribute valuable information to identify convergent evolution in Euarchontoglires.
Introduction
The relationship between morphology, locomotory modes and habitat has been receiving greater attention in evolutionary studies of phenotypic variation (Losos and Sinervo, 1989; Losos, 1990; Bonine and Garland, 1999; Vanhooydonck and Van Damme, 1999; Thorington and Santana, 2007; Goodman et al., 2008; Irschick et al., 2008; Pfaff et al., 2015; Kawashima et al., 2018). It has been observed that differences in habitat trigger divergence in the evolution of the morphology of locomotory systems across many different taxonomic taxa (Losos, 1990; Runestad and Ruff, 1995; Irschick et al., 2005; Calsbeek and Irschick, 2007; Sargis et al., 2007; Samuels and Van Valkenburgh, 2008; Schmidt, 2008), and that convergent evolution of locomotory modes for the same habitat niche adaptation in phylogenetically unrelated taxa can lead to similar morphological traits (Lemelin and Grafton, 1998; Kingsolver and Huey, 2003; Samuels and Van Valkenburgh, 2008; Gleiss et al., 2011; Losos, 2011; Edwards et al., 2012; Chen and Wilson, 2015; Morris et al., 2018; Grossnickle et al., 2019, 2020). The morphological convergence provides clues to better understanding functional adaptation and how distantly related species evolved in a similar way.
The mammalian superorder Euarchontoglires is comprised of five orders and includes Lagomorpha (rabbit, pika, and hare), Rodentia (mouse, rat, squirrel, and beaver), Dermoptera (flying lemur), Scandentia (tree shrew), and Primates (human, monkey, and ape) (Murphy et al., 2001; Kriegs et al., 2006; Vander Linden et al., 2019). A great diversity in locomotory modes is observed among and within the Euarchontoglire orders, including fossorial, ricochetal, arboreal, terrestrial, and gliding (Macphee, 1993). Interestingly, the same locomotory modes are found in different orders of Euarchontoglire, including the arboreal mode in Scandentia, Rodentia, and Primates, the terrestrial mode in Scandentia and Rodentia, and the gliding mode in Dermoptera and Rodentia (Kirk et al., 2008; Meng et al., 2017; Vander Linden et al., 2019; Grossnickle et al., 2020; Figure 1). The sharing of locomotory modes among orders makes Euarchontoglires an intriguing group for the investigation of morphological convergence with locomotory modes. Previous studies with Euarchontoglires have focused on the convergence of forelimb evolution with several locomotory modes, but these studies only examined species within Rodentia (Samuels and Van Valkenburgh, 2008) or on skeletal convergence with the gliding mode in Dermoptera and Rodentia (Grossnickle et al., 2020). Therefore, knowledge on the convergent evolution of locomotory modes across Euarchontoglire orders is very limited and it remains unclear whether the underlying skeletal morphology of terrestrial and arboreal species from the different Euarchontoglire orders have converged.
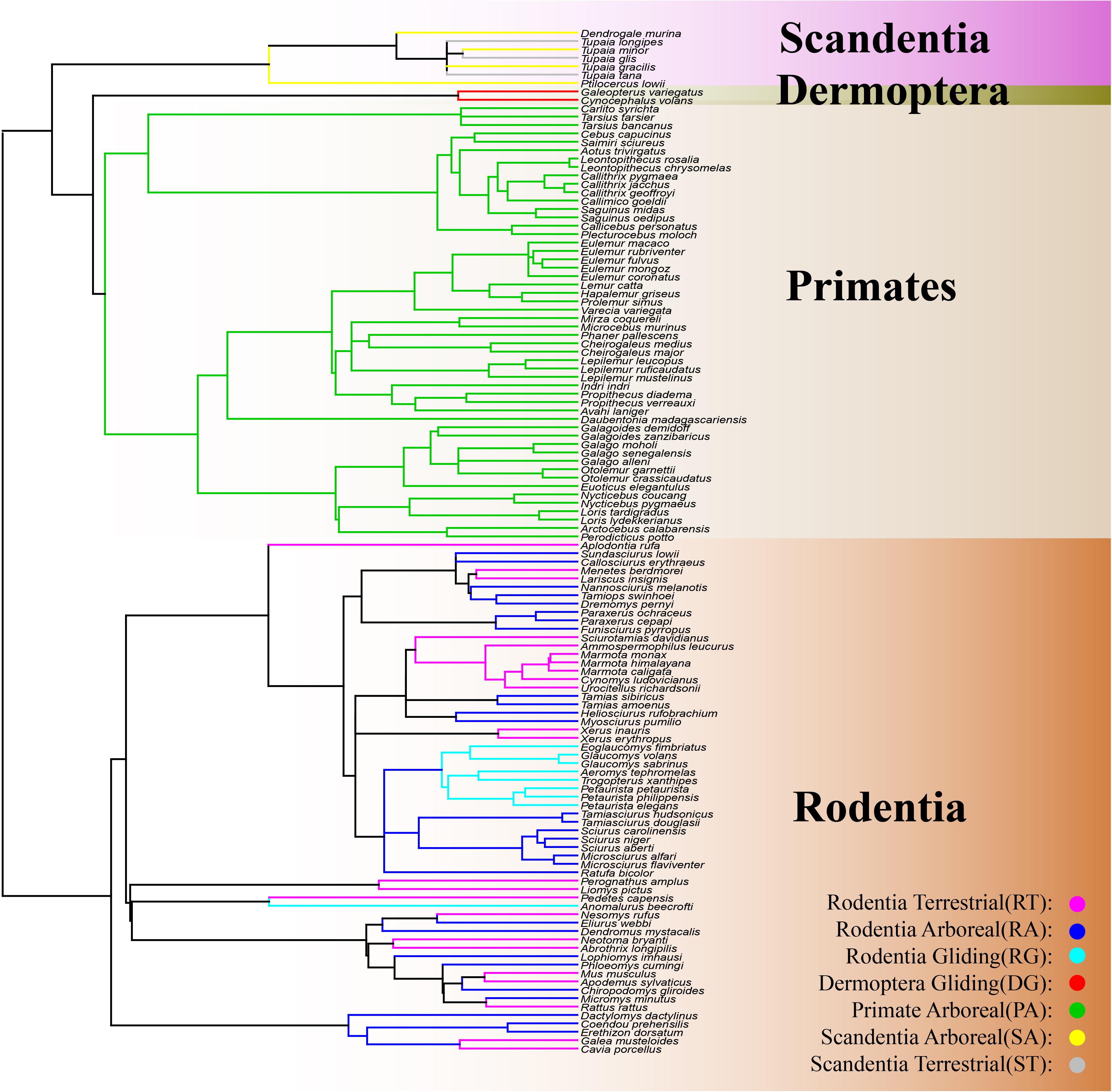
Figure 1. Phylogeny of the 203 individuals from 122 Euarchontoglire species included in this study. Rectangle with a gradient of light brown color represents the order Rodentia; the rectangle with a gradient of green color represents the order Dermoptera; the rectangle with a gradient of light-yellow color represents the order Primate; the rectangle with a gradient of purple color represents the order Scandentia. The yellow lines represent the Scandentia Arboreal (SA) species; the gray lines represent the Scandentia Terrestrial (ST) species; the red lines represent the Dermoptera Gliding (DG) species; the green lines represent the Primate Arboreal (PA) species; the purple lines represent the Rodentia Terrestrial (RT) species; the blue lines represent the Rodentia Arboreal (RA) species; the indigo lines represent the Rodentia Gliding (RG) species.
In the present study, we collected three phalangeal indices of manual digit III from 203 individuals of 122 Euarchontoglires species that represent the arboreal (orders Scandentia, Rodentia, and Primates), terrestrial (orders Scandentia and Rodentia), and gliding (orders Dermoptera and Rodentia) locomotory modes to investigate the convergent evolution of locomotory modes across these Euarchontoglire orders. The three phalangeal indices include metacarpal of digit III (MC3), manus proximal phalanx of digit III (MPP3), and manus intermediate phalanx of digit III (MIP3), which represent the inherent properties of the manus (Corruccini, 1995; Hamrick, 2001; Bloch et al., 2007; Kirk et al., 2008) and have been used to illustrate the locomotory modes of mammals (Lemelin, 1999; Hamrick, 2001; Meng et al., 2006, 2017; Bloch et al., 2007; Kirk et al., 2008; Samuels and Van Valkenburgh, 2008; Venkataraman et al., 2013; Zheng et al., 2013; Chen and Wilson, 2015; Grossnickle et al., 2020; Young and Chadwell, 2020). Our morphometric analyses based on quantitative methods provide insights into the convergent evolution of locomotory modes in Euarchontoglires, and moreover, evaluate the potential of these indices for studies on the convergent evolution of locomotory modes in Euarchontoglires.
Materials and Methods
Data Collection
Publicly available data for the three phalangeal indices of manual digit III, including MC3, MPP3, and MIP3, from a total of 152 individuals from 122 Euarchontoglire species belonging to the orders Scandentia, Rodentia, Dermoptera, and Primates were collected from published sources (Kirk et al., 2008; Samuels and Van Valkenburgh, 2008; Chen and Wilson, 2015; Meng et al., 2017). These species represent arboreal (99 individuals from 83 species of Scandentia, Rodentia, and Primates), terrestrial (33 individuals from 28 species of Scandentia and Rodentia), and gliding (20 individuals from 11 species of Dermoptera and Rodentia) locomotory modes. In addition, three phalangeal indices of manual digit III were measured from 51 individuals of the gliding complex-toothed flying squirrel (Rodentia, Trogopterus xanthipes) that were bred in the Runxing Planting and Breeding Cooperative, Liquan County, Xi’an, Shaanxi Province in the present study and were added to the relatively limited data available to represent the gliding locomotory mode. The three phalangeal indices measured using the electronic digital indicator for Trogopterus xanthipes were shown in Supplementary Figure S1. Chordal nodes for each bone element were measured from the nearest to the farthest end, and from the parallel to the midline of the axis on the sagittal plane based on the methods of Kirk et al. (2008). In total, three phalangeal indices from 203 individuals of 122 species were collected for the present study (Supplementary Table S1). Individuals were divided into seven groups for analyses according to their taxonomic order and their locomotory mode. The groups are: Rodentia Gliding (RG; 64 individuals of 9 species), Dermoptera Gliding (DG; 7 individuals of 2 species), Scandentia Terrestrial (ST; 8 individuals of 3 species), Rodentia Terrestrial (RT; 25 individuals of 25 species), Scandentia Arboreal (SA; 6 individuals of 4 species), Rodentia Arboreal (RA; 34 individuals of 27 species), and Primate Arboreal (PA; 59 individuals of 52 species). For the present analysis, the MC3, MPP3, and MIP3 indices used were the percentage of the length of each indices of the total combined length for these three elements, i.e., MC3(p), MPP3(p), and MIP3(p), as previously used (Corruccini, 1995; Elissamburu and Vizcaino, 2004). Statistical differences within the 3 indices among the different groups were detected using the Wilcoxon test.
Morphometric Analyses
To avoid the possible bias of morphological variations resulted from the unbalanced individual numbers for each species, we calculated the average values per species to perform the morphometric analyses based on species level.
First, a principle component analyses (PCA) was conducted using the FactoMineR and factoextra packages in R (Version 3.6.2) to visualize the overall distribution of the groups in the morphospace.
Then, taking into the phylogenetic effect, the principal components of the Procrustes coordinates were projected in the phylomorphospace plot by using the phytools package in R (Version 3.6.2). A well-accepted mammalian species tree of 122 species in our study from TimeTree database1 was used as the input tree (Figure 1). This plot could detect the convergence based on the length and direction of branches in relation to their ancestral states (Feijó et al., 2020).
To statistically quantify the magnitude of convergence for the three locomotory modes from different orders, i.e., the gliding (RG and DG), arboreal (RA, PA, and SA) and terrestrial (ST and RT) modes, the distance-based metric (C1–C4) were calculated (Stayton, 2015) by using the “convrat” function from convevol package in R (Version 3.6.2). C1 is the distance between the convergent tips of the taxa of interest (Dtip) divided by the maximum distance between any ancestral nodes (Dmax), which represents the proportion of the maximum distance between two lineages. This change of this value from 0 to 1 means the degree of convergence signal from non-convergence to convergence. C2 is obtained by Dtip minus Dmax and represent the magnitude of convergent change. C3 and C4 are the standardized value of C2 which were calculated by dividing C2 by the total morphological branch length of convergent taxa of interest (C3) and the total amount of branch length in the entire clade (C4) (Stayton, 2015), respectively. The significance of the metrics was assessed by the “convratsig” function of the same package with 1000 iterations.
Results and Discussion
The three indices, i.e., MC3(p), MPP3(p), and MIP3(p), used for the seven groups, including Rodentia Gliding (RG), Dermoptera Gliding (DG), Scandentia Terrestrial (ST), Rodentia Terrestrial (RT), Scandentia Arboreal (SA), Rodentia Arboreal (RA), and Primate Arboreal (PA) are listed in Supplementary Table S1. Statistical tests for the three indices among the different groups revealed significant differences (p < 0.05) between most groups, with the exception of MIP3(p) for the same locomotory modes, including MIP3(p) between ST and RT (p = 0.4774), SA and RA (p = 0.3449), SA and PA (p = 0.7868), and RA and PA (p = 0.089), and MPP3(p) between RA and RG (p = 0.5345) (Supplementary Table S2).
A PCA of the three indices [MC3(p) + MPP3(p) + MIP3(p)] showed that PC1 explained 73.6% of variation and separated the terrestrial species of Scandentia and Rodentia (ST and RT groups) from the arboreal and gliding species (RA, PA, SA, RG, and DG groups) (p < 0.05). PC2 explained 26.4% of variation and separated the gliding species of Dermoptera (DG group) from the other species examined (p < 0.05) (Figure 2).
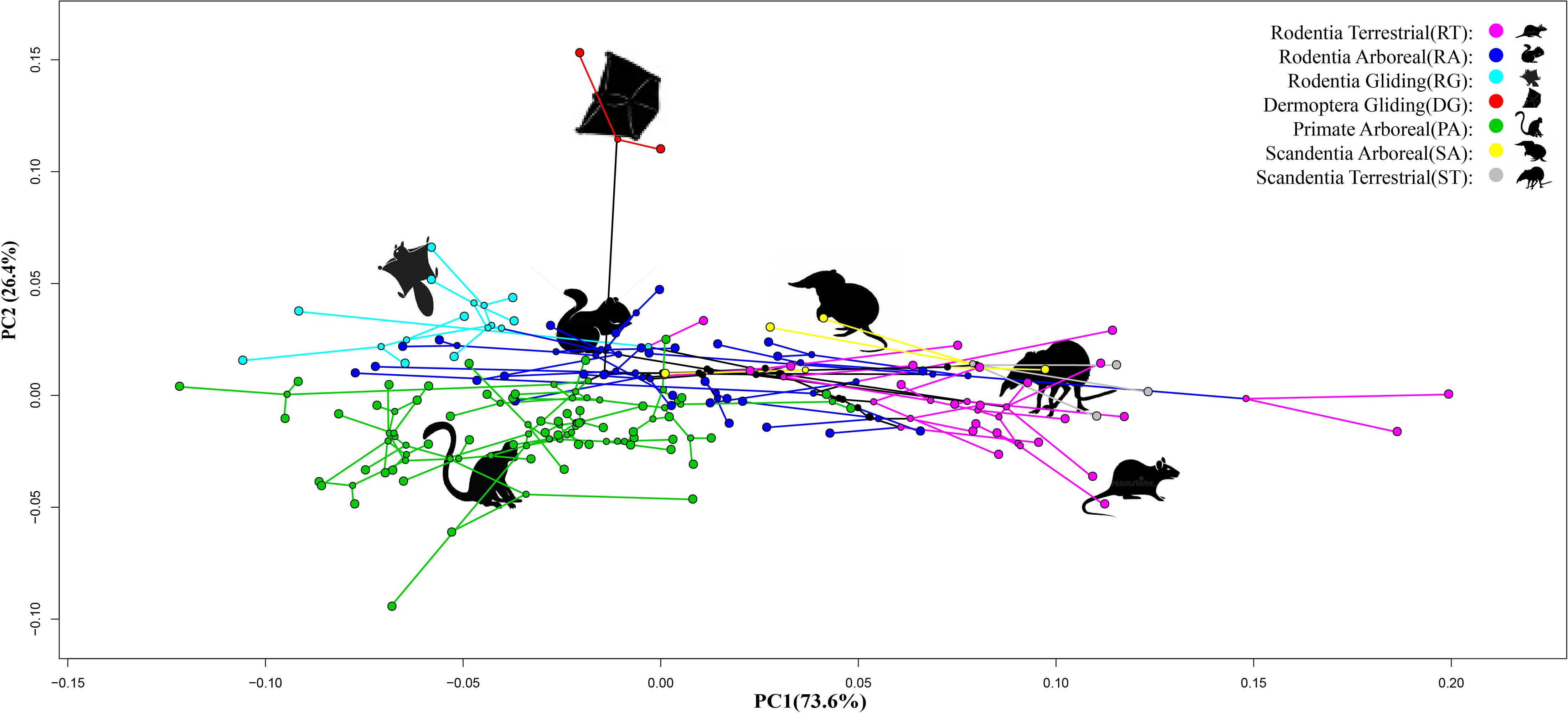
Figure 2. Phylomorphospace plot of 122 Euarchontoglire species based on the scores of a principle component analysis (PCA) of three phalangeal indices of manual digit III. The yellow color represents the Scandentia Arboreal (SA) species; the gray color represents the Scandentia Terrestrial (ST) species; the red color represents the Dermoptera Gliding (DG) species; the green color represent the Primate Arboreal (PA) species; the purple color represents the Rodentia Arboreal (RT) species; the blue color represents the Rodentia Terrestrial (RT) species; the indigo color represents the Rodentia Gliding (RG) species.
As seen from the phylomorphospace plot, the terrestrial species from Scandentia (ST group) and Rodentia (RT group) clustered together, suggesting that their similar phalangeal morphology may contribute to adapt to the same ground habitat. Likewise, the arboreal species from Scandentia (SA group), Rodentia (RA group), and Primate (PA group) clustered together, suggesting that their similar phalangeal morphology help them adapt to their grasping behavior. These results indicate the similarity of these morphological traits in the terrestrial and arboreal locomotory modes in Euarchontoglires. Interestingly, we found that the gliding species from Rodentia (RG group) were located between the arboreal species (RA, PA, and SA groups) and the gliding species from Dermoptera (DG group), consistent with the observation that the RG group species have evolved their morphological characteristics by not only adapting to tree-dwelling and branch-grasping life, but also to the gliding movement (Samuels and Van Valkenburgh, 2008; Grossnickle et al., 2020). The gliding species from Dermoptera are most distantly separated from the other species. Although the gliding species of Dermoptera (DG group) did not cluster with those from Rodentia (RG group) in the phylomorphospace plot (Figure 2), these two groups possess the same gliding locomotory mode and show a trend of closer proximity.
The C-metrics analyses of all three locomotory modes showed statistically significant convergence [gliding (DG and RG), C1 = 0.563, P < 0.05], arboreal (RA, PA, and SA), C1 = 0.424, P < 0.05) and terrestrial (ST and RT), C1 = 0.560, P < 0.05)], which indicate that evolution has reduced the distances in the gliding species and terrestrial species by showing more than 50% similarity than expected by chance, and in the arboreal species by more than 40% (Table 1). In addition, we also explore the potential species that show more signals of convergence for the three locomotory modes, as used by the method of Stayton (2015). The phylomorphospace plot analyses and the C-metrics analyses identified and quantified at least 72 species in total showing more signals of convergence for the three locomotory modes, respectively (Supplementary Table S3 and Supplementary Figure S2).
Therefore, our morphometric analyses based on quantitative methods provide insight into the convergent evolution of terrestrial, arboreal, and gliding locomotory modes in Euarchontoglires. In our analyses, the terrestrial species (ST and RT groups) demonstrate a relatively long metacarpal (MC3) and short phalange (MPP3 and MIP3) compared with the arboreal and gliding species (P < 0.05) (Figure 3), which would help increase their speed for hunting or feeding on the ground (Peng et al., 1991; Kirk et al., 2008; Samuels and Van Valkenburgh, 2008; Fuchs and Corbach-Söhle, 2010). In addition, previous studies have reported the possible genetic mechanisms at the gene level involving muscle contraction and skeletal morphogenesis for locomotory adaptation of terrestrial in Scandentia group (Fan et al., 2014). Our study not only confirmed the long metacarpal and short phalange in the terrestrial species of both Scandentia and Rodentia, but also demonstrated convergent evolution with the terrestrial locomotory mode in these two orders of Euarchontoglire.
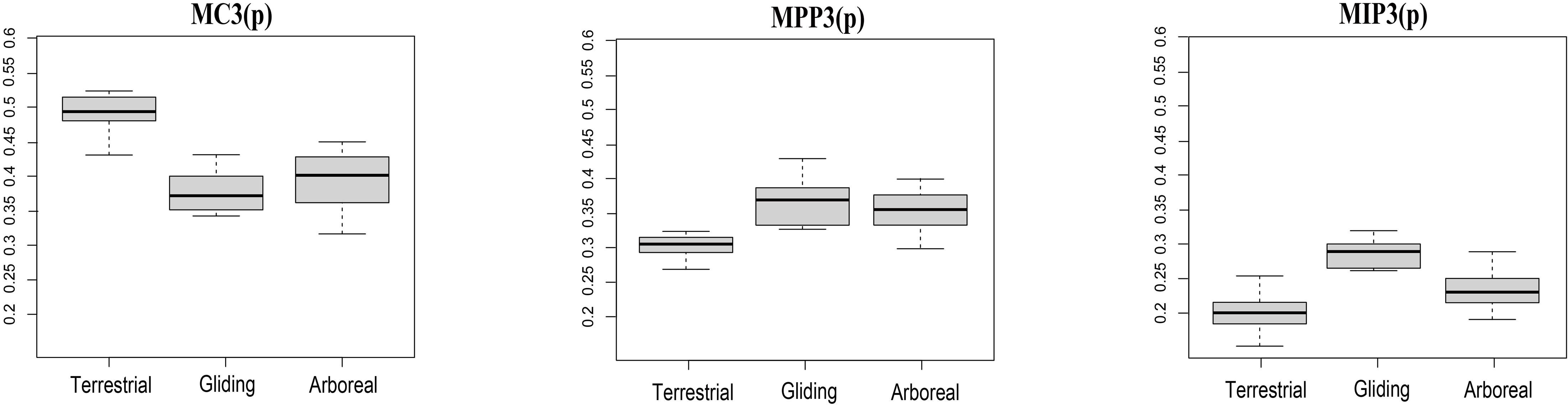
Figure 3. The distribution of the three indices among each locomotory mode. The abscissa represents the locomotory modes and the ordinate represents the ratios of the indices. MC3(p): The proportion of MC3 in the total combined length of the three elements; MPP3(p): The proportion of MPP3 in the total combined length of the three elements; MIP3(p): The proportion of MIP3 in the total combined length of the three elements. Terrestrial mode includes ST and RT groups; Arboreal mode includes SA, RA, and PA groups; Gliding mode includes DG and RG groups. Wilcoxon tests of the three indices among each locomotry mode all show significant P-values (P < 0.05).
In comparison, the arboreal species (RA, PA, and SA groups) show an elongated phalange MPP3 and MIP3 compared with terrestrial species (P < 0.05) (Figure 3), which would help them grasp branches while they are moving. This phenomenon has been demonstrated in arboreal Primates (Lemelin, 1999; Hamrick, 2001; Bloch et al., 2007; Kirk et al., 2008; Young and Chadwell, 2020) and Rodentia (Kirk et al., 2008; Samuels and Van Valkenburgh, 2008). Our study not only observed this in the arboreal species of Scandentia, Rodentia, and Primates, but also demonstrated convergent evolution with the arboreal locomotory mode in these three orders of Euarchontoglire.
The gliding species (DG and RG groups) demonstrated an elongation of the phalangeal MIP3 compared with arboreal and terrestrial species (Figure 3), which is likely associated with their unique attachment of the patagia to adapt the gliding locomotory habitat (Macphee, 1993) or to their roosting behavior (Grossnickle et al., 2020). Although they both possessed patagium, but they are different in that the former shows the patagium attachment by the styliform cartilages from the wrist or elbow while the latter’s patagium are attached to the digits (Jackson, 2012). Further examination of the gliding species from these two orders showed that the gliding species from Rodentia have MPP3 lengths similar to those of arboreal species and have elongated MIP3, while the gliding species from Dermoptera have the shortest MPP3 and the most elongated MIP3 compared with species of the other two locomotory modes and the gliding species from Rodentia (Supplementary Table S1). According to these above observations, we speculate that the similarities and differences between these two orders make them both distant from the other locomotory modes, but prevent their clustering like that seen for the other two locomotory modes, although they do show a trend of having closer proximity in the phylomorphospace plot and do show the significant convergence signal in C-metrics analyses. Besides the phalangeal indices of manual digit III examined here, the gliding mammals also developed other morphological modifications for gliding adaptation, including the relatively elongated neck, longer forearms, lumbar, and shorter thoracic vertebrae, hands, feet bony as well as the more sensitivity of bony labyrinth morphometry in the inner ear (Thorington and Santana, 2007; Pfaff et al., 2015; Kawashima et al., 2018). So we hypothesized that additional morphological traits, in addition to the three indices used in the present study, are also necessary to address the convergence in the gliding locomotory mode. Indeed, a recent phylogenetic comparative analysis of 31 skeletal traits from the gliding mammals has observed convergence, including the gliding Dermoptera and Rodentia (Grossnickle et al., 2020).
Conclusion
In the present study, we used morphometric analyses based on quantitative methods to explore the convergence of locomotory modes across diverse Euarchontoglires orders. Our results showed that the phalangeal morphology of species with terrestrial, arboreal, and gliding locomotory modes from multiple Euarchontoglire orders are convergent, supporting the convergent evolution of these three locomotory modes. This study adds evidence of phenotypic convergent evolution of the locomotory modes in Euarchontoglires by extending the investigation into the terrestrial, arboreal, and gliding locomotory modes across four Euarchontoglires orders, which had previously only been studied for the gliding mode of Euarchontoglires or for the other modes only within Primates and Rodentia. With these findings we provide a framework for future work on convergence in mammals, and the application of the phalangeal indices used here to allow the inference and interpretation of locomotory modes of unknown or fossil species.
Data Availability Statement
The original contributions presented in the study are included in the article/Supplementary Material, further inquiries can be directed to the corresponding author/s.
Ethics Statement
The animal study was reviewed and approved by the Committee on Animal Research and Ethics of Yunnan University.
Author Contributions
LY designed the study. W-HG, L-FC, TZ, XW, and RL measured and collected the data. X-PW and W-HG performed the data analyses. LY, X-PW, and W-HG wrote the manuscript. CR and DI revised the manuscript. All authors read and approved the final manuscript.
Funding
This work was supported by a grant from the National Natural Science Foundation of China (31760619) to XW, an Applied Basic Research General Project of Yunnan Science and Technology Department (202001BB050058) to XW and “One Institute One Product” Special Project of Shaanxi Academy of Sciences (2020K-02) to L-FC.
Conflict of Interest
The authors declare that the research was conducted in the absence of any commercial or financial relationships that could be construed as a potential conflict of interest.
The reviewer, Y-GY, declared a shared affiliation with one of the authors, W-HG, to the handling editor at the time of review.
Supplementary Material
The Supplementary Material for this article can be found online at: https://www.frontiersin.org/articles/10.3389/fevo.2020.615862/full#supplementary-material
Footnotes
References
Bloch, J. I., Silcox, M. T., Boyer, D. M., and Sargis, E. J. (2007). New Paleocene skeletons and the relationship of plesiadapiforms to crown-clade primates. Proc. Natl. Acad. Sci. U.S.A. 104, 1159–1164. doi: 10.1073/pnas.0610579104
Bonine, K. E., and Garland, T. (1999). Sprint performance of phrynosomatid lizards, measured on a high-speed treadmill, correlates with hindlimb length. J. Zool. 248, 255–265. doi: 10.1111/j.1469-7998.1999.tb01201.x
Calsbeek, R., and Irschick, D. J. (2007). The quick and the dead: correlational selection on morphology, performance, and habitat use in island lizards. Evolution 61, 2493–2503. doi: 10.1111/j.1558-5646.2007.00206.x
Chen, M., and Wilson, G. P. (2015). A multivariate approach to infer locomotor modes in Mesozoic mammals. Paleobiology 41, 280–312. doi: 10.1017/pab.2014.14
Corruccini, R. S. (1995). Of ratios and rationality. Am. J. Phys. Anthropol. 96, 189–191. doi: 10.1002/ajpa.1330960209
Edwards, S., Vanhooydonck, B., Herrel, A., Measey, G. J., and Tolley, K. A. (2012). Convergent evolution associated with habitat decouples phenotype from phylogeny in a clade of lizards. PLoS One 7:e51636. doi: 10.1371/journal.pone.0051636
Elissamburu, A., and Vizcaino, S. F. (2004). Limb proportions and adaptations in caviomorph rodents (Rodentia : Caviomorpha). J. Zool. 262, 145–159. doi: 10.1017/S0952836903004485
Fan, Y., Yu, D. D., and Yao, Y. G. (2014). Positively selected genes of the Chinese tree shrew (Tupaia belangeri chinensis) locomotion system. Zool. Res. 35, 240–248. doi: 10.11813/j.issn.0254-5853.2014.3.240
Feijó, A., Ge, D. Y., Wen, Z. X., Xia, L., and Yang, Q. S. (2020). Divergent adaptations in resource-use traits explain how pikas thrive on the roof of the world. Funct. Ecol. 34, 1826–1838. doi: 10.1111/1365-2435.13609
Fuchs, E., and Corbach-Söhle, S. (2010). “Tree shrews,” in The UFAW Handbook on the Care and Management of Laboratory and Other Research Animals, eds R. Hubrecht and J. Kirkwood (Oxford: Wiley-Blackwell), 262–275. doi: 10.1002/9781444318777.ch20
Gleiss, A. C., Jorgensen, S. J., Liebsch, N., Sala, J. E., Norman, B., Hays, G. C., et al. (2011). Convergent evolution in locomotory patterns of flying and swimming animals. Nat. Commun. 2:352. doi: 10.1038/ncomms1350
Goodman, B. A., Miles, D. B., and Schwarzkopf, L. (2008). Life on the rocks: habitat use drives morphological and performance evolution in lizards. Ecology 89, 3462–3471. doi: 10.1890/07-2093.1
Grossnickle, D. M., Chen, M., Wauer, J. G. A., Pevsner, S. K., Weaver, L. N., Meng, Q. J., et al. (2020). Incomplete convergence of gliding-mammal skeletons. Evolution doi: 10.1111/evo.14094 [Online ahead of print].
Grossnickle, D. M., Smith, S. M., and Wilson, G. P. (2019). Untangling the multiple ecological radiations of early mammals. Trends Ecol. Evol. 34, 936–949. doi: 10.1016/j.tree.2019.05.008
Hamrick, M. W. (2001). Primate origins: evolutionary change in digital ray patterning and segmentation. J. Hum. Evol. 40, 339–351. doi: 10.1006/jhev.2001.0467
Irschick, D. J., Herrel, A. V., Vanhooydonck, B., Huyghe, K., and Van Damme, R. (2005). Locomotor compensation creates a mismatch between laboratory and field estimates of escape speed in lizards: a cautionary tale for performance-to-fitness studies. Evolution 59, 1579–1587. doi: 10.1111/j.0014-3820.2005.tb01807.x
Irschick, D. J., Meyers, J. J., Husak, J. F., and Le Galliard, J. F. (2008). How does selection operate on whole-organism functional performance capacities? A review and synthesis. Evol. Ecol. Res. 10, 177–196. doi: 10.1111/j.1558-5646.2007.00298.x
Kawashima, T., Thorington, R. W. Jr., Bohaska, P. W., and Sato, F. (2018). Variability and constraint of vertebral formulae and proportions in colugos, tree shrews, and rodents, with special reference to vertebral modification by aerodynamic adaptation. Folia Morphol. 77, 44–56. doi: 10.5603/FM.a2017.0064
Kingsolver, J. G., and Huey, R. B. (2003). Introduction: the evolution of morphology, performance, and fitness. Integr. Comp. Biol. 43, 361–366. doi: 10.1093/icb/43.3.361
Kirk, E. C., Lemelin, P., Hamrick, M. W., Boyer, D. M., and Bloch, J. I. (2008). Intrinsic hand proportions of euarchontans and other mammals: implications for the locomotor behavior of plesiadapiforms. J. Hum. Evol. 55, 278–299. doi: 10.1016/j.jhevol.2008.02.008
Kriegs, J. O., Churakov, G., Kiefmann, M., Jordan, U., Brosius, J., and Schmitz, J. (2006). Retroposed elements as archives for the evolutionary history of placental mammals. PloS. Biol. 4:e91. doi: 10.1371/journal.pbio.0040091
Lemelin, P. (1999). Morphological correlates of substrate use in Didelphid marsupials: implications for primate origins. J. Zool. 247, 165–175. doi: 10.1017/S0952836999002046
Lemelin, P., and Grafton, B. W. (1998). “Grasping performance in Saguinus midas and the evolution of hand prehensility in primates,” in Primate Locomotion:Recent Advances, eds E. Strasser, J. Fleagle, A. Rosenberger, and H. McHenry (New York, NY: Plenum Press), 131–144. doi: 10.1007/978-1-4899-0092-0_8
Losos, J. B. (1990). Concordant evolution of locomotor behavior, display rate and morphology in Anolis lizards. Anim. Behav. 39, 879–890. doi: 10.1016/S0003-3472(05)80952-2
Losos, J. B. (2011). Convergence, adaptation, and constraint. Evolution 65, 1827–1840. doi: 10.1111/j.1558-5646.2011.01289.x
Losos, J. B., and Sinervo, B. (1989). The effects of morphology and perch diameter on sprint performance of Anolis lizards. J. Exp. Biol. 145, 23–30.
Macphee, R. D. E. (1993). Primates and Their Relatives in Phylogenetic Perspective. New York, NY: Plenum Press, 63–90.
Meng, J., Hu, Y., Wang, Y., Wang, X., and Li, C. (2006). A Mesozoic gliding mammal from northeastern China. Nature 444, 889–893. doi: 10.1038/nature05234
Meng, Q. J., Grossnickle, D. M., Liu, D., Zhang, Y. G., Neander, A. I., Ji, Q., et al. (2017). New gliding mammaliaforms from the Jurassic. Nature 548, 291–296. doi: 10.1038/nature23476
Morris, P. J. R., Cobb, S. N. F., and Cox, P. G. (2018). Convergent evolution in the Euarchontoglires. Biol. Lett. 14:20180366. doi: 10.1098/rsbl.2018.0366
Murphy, W. J., Eizirik, E., O’brien, S. J., Madsen, O., Scally, M., Douady, C. J., et al. (2001). Resolution of the early placental mammal radiation using Bayesian phylogenetics. Science 294, 2348–2351. doi: 10.1126/science.1067179
Peng, Y. Z., Ye, Z. Z., Zou, R. J., Wang, Y. X., Tian, B. P., Ma, Y. Y., et al. (1991). Biology of Chinese Tree Shrews (Tupaia belangeri chinensis). Kunming: Yunnan Science and Technology Press.
Pfaff, C., Martin, T., and Ruf, I. (2015). Bony labyrinth morphometry indicates locomotor adaptations in the squirrel-related clade (Rodentia, Mammalia). Proc. R. Soc. B Biol. Sci. 282:20150744. doi: 10.1098/rspb.2015.0744
Runestad, J. A., and Ruff, C. B. (1995). Structural adaptations for gliding in mammals with implications for locomotor behavior in Paromomyids. Am. J. Phys. Anthropol. 98, 101–119. doi: 10.1002/ajpa.1330980202
Samuels, J. X., and Van Valkenburgh, B. (2008). Skeletal indicators of locomotor adaptations in living and extinct rodents. J. Morphol. 269, 1387–1411. doi: 10.1002/jmor.10662
Sargis, E. J., Boyer, D. M., Bloch, J. I., and Silcox, M. T. (2007). Evolution of pedal grasping in Primates. J. Hum. Evol. 53, 103–107. doi: 10.1016/j.jhevol.2007.01.008
Schmidt, M. (2008). Forelimb proportions and kinematics: how are small primates different from other small mammals? J. Exp. Biol. 211, 3775–3789. doi: 10.1242/jeb.019802
Stayton, C. T. (2015). The definition, recognition, and interpretation of convergent evolution, and two new measures for quantifying and assessing the significance of convergence. Evolution 69, 2140–2153. doi: 10.1111/evo.12729
Thorington, R., and Santana, E. (2007). How to make a flying squirrel: Glaucomys anatomy in phylogenetic perspective. J. Mammal. 88, 882–896. doi: 10.1644/06-Mamm-S-325r2.1
Vander Linden, A., Hedrick, B. P., Kamilar, J. M., and Dumont, E. R. (2019). Atlas morphology, scaling and locomotor behaviour in primates, rodents and relatives (Mammalia: Euarchontoglires). Zool. J. Linn. Soc. 185, 283–299. doi: 10.1093/zoolinnean/zly042
Vanhooydonck, B., and Van Damme, R. (1999). Evolutionary relationships between body shape and habitat use in lacertid lizards. Evol. Ecol. Res. 1, 785–805.
Venkataraman, V. V., Rolian, C., Gordon, A. D., and Patel, B. A. (2013). A resampling approach and implications for estimating the phalangeal index from unassociated hand bones in fossil primates. Am. J. Phys. Anthropol. 151, 280–289. doi: 10.1002/ajpa.22278
Young, J. W., and Chadwell, B. A. (2020). Not all fine-branch locomotion is equal: grasping morphology determines locomotor performance on narrow supports. J. Hum. Evol. 142:102767. doi: 10.1016/j.jhevol.2020.102767
Keywords: convergent evolution, Euarchontoglires, metacarpal, manus proximal phalanx, manus intermediate phalanx, locomotory modes
Citation: Geng W-h, Wang X-p, Che L-f, Wang X, Liu R, Zhou T, Roos C, Irwin DM and Yu L (2020) Convergent Evolution of Locomotory Modes in Euarchontoglires. Front. Ecol. Evol. 8:615862. doi: 10.3389/fevo.2020.615862
Received: 10 October 2020; Accepted: 02 December 2020;
Published: 23 December 2020.
Edited by:
Deyan Ge, Chinese Academy of Sciences (CAS), ChinaReviewed by:
Anderson Feijo, Chinese Academy of Sciences (CAS), ChinaYong-Gang Yao, Kunming Institute of Zoology, China
Jia-Tang Li, Chinese Academy of Sciences, China
Copyright © 2020 Geng, Wang, Che, Wang, Liu, Zhou, Roos, Irwin and Yu. This is an open-access article distributed under the terms of the Creative Commons Attribution License (CC BY). The use, distribution or reproduction in other forums is permitted, provided the original author(s) and the copyright owner(s) are credited and that the original publication in this journal is cited, in accordance with accepted academic practice. No use, distribution or reproduction is permitted which does not comply with these terms.
*Correspondence: Li Yu, eXVsaUB5bnUuZWR1LmNu
†These authors have contributed equally to this work