- 1Laboratory of Applied Microbiology, University of Applied Sciences and Arts of Southern Switzerland, Bellinzona, Switzerland
- 2School of Biological Sciences, University of Bristol, Bristol, United Kingdom
- 3Insect Division, Department of Life Sciences, Natural History Museum, London, United Kingdom
Bats and moths provide a textbook example of predator-prey evolutionary arms races, demonstrating adaptations, and counter adaptations on both sides. The evolutionary responses of moths to the biosonar-led hunting strategies of insectivorous bats include convergently evolved hearing structures tuned to detect bat echolocation frequencies. These allow many moths to detect hunting bats and manoeuvre to safety, or in the case of some taxa, respond by emitting sounds which startle bats, jam their biosonar, and/or warn them of distastefulness. Until now, research has focused on the larger macrolepidoptera, but the recent discovery of wingbeat-powered anti-bat sounds in a genus of deaf microlepidoptera (Yponomeuta), suggests that the speciose but understudied microlepidoptera possess further and more widespread anti-bat defences. Here we demonstrate that wingbeat-powered ultrasound production, likely providing an anti-bat function, appears to indeed be spread widely in the microlepidoptera; showing that acoustically active structures (aeroelastic tymbals, ATs) have evolved in at least three, and likely four different regions of the wing. Two of these tymbals are found in multiple microlepidopteran superfamilies, and remarkably, three were found in a single subfamily. We document and characterise sound production from four microlepidopteran taxa previously considered silent. Our findings demonstrate that the microlepidoptera contribute their own unwritten chapters to the textbook bat-moth coevolutionary arms race.
Introduction
Roeder’s seminal discovery of anti-bat hearing (Roeder and Treat, 1957) sparked research into the defences of nocturnal moths against echolocating bats in the bat-moth acoustic evolutionary arms race. Many nocturnal insects, including moths, have evolved hearing structures to detect bats (e.g., Miller and Surlykke, 2001) and the Arctiinae (tiger moths) are well known for their defensive sounds that function through startling their predators, acoustic aposematism (warning sounds), and/or echolocation jamming (e.g., Corcoran et al., 2010). However, a recent surge of new discoveries has arisen in this arms race: taxa other than the Arctiinae have been shown to produce anti-bat sounds (Barber and Kawahara, 2013; Corcoran and Hristov, 2014; O’Reilly et al., 2019), the hindwing “tails” of some moths have been discovered to act as acoustic decoys (Barber et al., 2015; Lee and Moss, 2016), and the acoustic absorptive power of moth scales as acoustic metamaterials has emerged as a fascinating and complex new area of research (Zeng et al., 2011; Ntelezos et al., 2017; Shen et al., 2018; Neil et al., 2020a, b). This spate of recent discoveries suggests the true extent of moth anti-bat adaptations might substantially exceed current knowledge.
Lepidoptera have been crudely divided by their size into two suborders: the smaller micro- and the larger macrolepidoptera. Most research into the anti-bat defences of moths has focussed on the macrolepidoptera, yet preferred prey size varies both within (Waters et al., 1995) and between bat species. Some species such as Myotis septentrionalis, rely heavily on microlepidoptera as dietary constituents (e.g., Dodd et al., 2012). Microlepidoptera are therefore also under significant predation pressure from bats.
It would seem highly likely that such pressure on the microlepidoptera would lead to the evolution of anti-bat defences analogous to those found in macrolepidoptera. However, research into such defences has seemingly just recently begun, with only two studies, other than those investigating the well-known pyralid hearing (e.g., Skals and Surlykke, 2000), addressing the subject. Firstly, Kovalev (2016) suggested that the feather-like wing plumes of Alucita hexadactyla (Alucitidae) may have evolved to reduce its echo intensity, and secondly O’Reilly et al. (2019) discovered that the hyaline (transparent) hindwing patches of the microlepidopteran genus Yponomeuta (Yponomeutidae) are wingbeat-powered aeroelastic tymbals (ATs) that render these deaf moths acoustic Müllerian mimics of aposematic Arctiinae.
Like the sound-producing tymbals located on the thorax of the macrolepidopteran Arctiinae, Yponomeuta ATs produce two bursts (one longer and one shorter burst) of ultrasonic clicks through buckling of a series of striations. However, unlike arctiine sound production, AT clicks are not produced upon detection of an approaching bat. Instead, they occur during every wingbeat, one burst per wing stroke. As these moths are deaf and unable to detect and respond to hunting bats, these structures allow them to bypass predator detection by constantly producing warning sounds. Yponomeuta provide the first example of constitutive acoustic aposematism in the bat-moth arms race (O’Reilly et al., 2019). This elegant defence solution for unpalatable, deaf microlepidoptera is unlikely to be exclusive to the Yponomeutidae and here we specifically investigate whether other microlepidopteran taxa possess yet undocumented defences based on ATs.
As the AT of Yponomeuta reveals itself as a hyaline patch in the wing, hyaline patches in other microlepidopteran taxa might suggest similar acoustic functionality. The presence of hyaline wing patches is indeed not exclusive to Yponomeuta. For example, Monopis, Crypsithyrodes, and Crypsithyris (Tineidae) species are characterised by a subhyaline patch in the discal cell of the forewing (Robinson, 1980; Xiao and Li, 2005; Lee et al., 2016), and members of the Tinea pellionella species complex (Tineidae) possess hyaline/subhyaline patches at the base of the forewing, just below the subcosta (Robinson, 1979). Generally, hyaline wing patches, such as the above examples, are only documented in the literature if they serve as identification features.
Given that microlepidoptera are under significant predation pressure from echolocating bats, and that ATs provide an elegant method of passive acoustic protection, we anticipated that these structures would be taxonomically widespread. Thus, through a comprehensive morphological assay as well as acoustic characterisation, we investigated our prediction that ATs have convergently evolved throughout the microlepidoptera.
Materials and Methods
Phylogenetic Spread of Candidate ATs
Image Analysis
For each of our two phylogenetic analyses we assessed taxa for the presence of known and candidate ATs. This was primarily achieved by examining online image databases of microlepidoptera. The majority of photographs were assessed from the Barcode of Life Database (BOLD) (Ratnasingham and Hebert, 2007), but microscopic assessment of specimens from the Bristol Museum and Art Gallery and the Natural History Museum, London was also used.
A known AT was defined as a hyaline patch in the same position on the wing as related taxa known to produce wingbeat-powered sound, e.g., a hindwing hyaline patch in an Yponomeuta species or relative. A candidate AT was defined as a hyaline patch on the wing with no obvious other function. If possible, for every species suspected of possessing an AT, multiple specimens were assessed to confirm the presence of the structure. This aided in preventing false positives due to symmetrical specimen damage.
Candidate ATs in the Microlepidoptera
This comprehensive assessment of the presence of ATs includes all microlepidopteran taxa from the 11 superfamilies in a recent molecular phylogeny of the Lepidoptera (Regier et al., 2013), from Nepticuloidea up to and including Gelechioidea. Despite the Pyraloidea being considered microlepidoptera, they were excluded from this analysis, because they have ultrasound sensitive ears, which constant sound production by ATs would excite and habituate, rendering the combination of ears and AT counterproductive. Furthermore, any transparent areas of the wings of the Sesiidae were not considered as potential ATs as they more likely function as part of their visual mimicry of Hymenoptera.
We used a simplified phylogenetic tree based on Figure S1 from Regier et al. (2013) for this study, to identify likely points of independent evolution of ATs within the microlepidoptera. The genus Monopis (Tineidae) and the Scaeosophinae (Cosmopterigidae) were not included in the original phylogeny (Regier et al., 2013) but were found to possess candidate ATs, so they were includes in our analysis.
Candidate ATs in the Tineidae
Photographs of 751 species (148 genera) of Tineidae were assessed. Between one and 20 photographs (individuals) were assessed per species for the presence of hyaline patches on either the forewing or hindwing. In the case of the genus Chrypsithyris (four species) images and species descriptions were used from Xiao and Li (2005). Images for Niditinea sabroskyi were used from (Metz et al., 2018).
Phylogenetic Analysis
As far as we are aware, a detailed molecular phylogeny of the Tineidae does not exist; thus, using publicly available data, a phylogenetic tree was inferred using the Cytochrome Oxidase Subunit 1 (COI) amino acid sequence from 90 species from 19 genera of the Tineidae family. Dolophilodes distinctus (Philopotamidae: Trichoptera) was used as an outgroup. Sequences were downloaded from BOLD Systems (Ratnasingham and Hebert, 2007), aligned using MAFFT version 7 (Katoh and Standley, 2013), and the alignments were trimmed using BMGE 4.0, using a BLOSUM62 matrix to remove poorly aligned positions (Criscuolo and Gribaldo, 2010). The phylogenetic tree was inferred using IQ-Tree (Nguyen et al., 2015), and the best-fitting substitution model (LG+C60+G) was selected by Bayesian Information Criterion (Le and Gascuel, 2008). Moreover, empirical profile mixture models were used to improve model fit (Quang et al., 2008). The bootstrap supports were estimated using UFBoot2 (Minh et al., 2013). Trees were visualised and edited in ITOL (Letunic and Bork, 2016). For links to software used see Table 1.
Sound Production by Candidate ATs
Species
We gained access to live specimens of five relevant species to test in laboratory conditions: two Tineinae (Tineidae) species possessing candidate ATs (Monopis crocicapitella, Clemens, 1859 n = 2; and T. pellionella, Linnaeus, 1758 n = 7), one Tineinae species lacking candidate ATs (Tineola bisselliella, Hummel, 1823 n = 6), one Oecophoridae species possessing candidate ATs (Endrosis sarcitrella, Linnaeus, 1758 n = 4) and one lacking them (Hofmannophila pseudospretella, Stainton, 1849 n = 2), which were all tested for sound production. All T. pellionella specimens were wild caught from three houses in Bristol, United Kingdom, all T. bisselliella specimens were taken from a wicker basket found in Bristol, United Kingdom, both M. crocicapitella specimens were caught at one location in Weston-Super-Mare, United Kingdom using a mercury vapour moth trap in a suburban garden, and all E. sarcitrella and H. pseudospretella were caught from two houses within Bristol, United Kingdom. Moths were either acoustically assessed immediately or kept in a refrigerator between 4 and 6°C for up to 24 h before beginning assessment. If refrigerated, moths were kept at room temperature for at least 2 h prior to testing.
Tethering Method
Moths were first recorded in free flight, if they did not produce sound, they were not studied further, if they did then they were subsequently tethered. Due to their small size, we tethered them following O’Reilly et al. (2019): a 0.14 mm diameter insect pin was inserted into the dorsal meso/prothorax until the tip just punctured the ventral side. Like the moths tested in O’Reilly et al. (2019), all test specimens flew for prolonged periods post tethering.
The head of the tether (insect pin) was inserted into modelling clay attached to a flexible arm (Manfrotto + Co. Spa, Cassola, Italy), which allowed flexible positioning of the moth. We positioned the moth upside down, which elicited more prolonged flight compared to normal orientation. This stronger flight is probably due to the unusual gravitational pull on the insect causing it to try and return itself to its natural flight orientation.
Audio Recordings
All audio recordings of M. crocicapitella, T. pellionella, and E. sarcitrella (16bit, sampling rate 500 kHz) were made using USG Omnidirectional Electret Ultrasound Knowles FG-O microphones connected to an UltraSoundGate 1216H200 recorder, run through Avisoft Recorder USGH software (all Avisoft Bioacoustics, Berlin, Germany). Recordings were made in a semi-anechoic chamber (Industrial Acoustics Company Ltd., Winchester, United Kingdom).
Individual moths were initially placed in a 24″ × 24″ × 24″ BugDorm-1 Insect Rearing Cage (Megaview Science Co., Ltd., Taichung City, Taiwan) with one microphone positioned through a central sleeved hole on one side of the cage. Flight was initiated through flicking or tapping the cage where the insect was at rest. These free-flight recordings were initially analysed for the presence of any acoustic signal. If sound production was discovered, tethered recordings were subsequently made. For tethered recordings, the insect was positioned 30–50 mm from a microphone oriented perpendicular to the centre of the lateral axis of the moth. To reliably initiate flight, tethered moths were first given a small (∼5 mm diameter) ball of paper or foam to hold, this was removed when flight was required.
Additionally, one Monopis cf monachella (Hübner, 1796, collected in Germany) was assessed for free-flight sound production using an ultrasound bat detector, and one Ethmia bicolorella (Guenée, 1879, Depressariidae, collected in Kenya) specimen was recorded in free-flight using a USB ultrasonic microphone (Ultramic250K, Dodotronic, Italy) in 16 bit using a 250 kHz sampling rate. This recording was made in the field, as such it was only analysed for the presence/absence of ultrasonic click production. Four other Ethmia (E. sabiella, E. oculigera, E. cascineutis, and E. livida; Felder et al., 1875; Möschler, 1883; Meyrick, 1913; Zeller, 1852, respectively) species were collected and recorded in South Africa using the same methods. Recordings of these species were assessed for the presence or absence of ultrasonic clicks.
Ablation Experiments
Ablation of the candidate ATs was attempted on all individuals of M. crocicapitella, T. pellionella, and E. sarcitrella, however, due to the small size of the moths and their hyaline patches, this proved difficult. In all but one individual of each of the two Tineinae species the ablation attempt resulted in enough damage to the wings to render them unable to fly. Therefore, ablation results were only taken from one individual of each Tineinae species. E. sarcitrella patches were more fragile than those of the Tineinae, therefore a cruder method of ablation (removal of the hindwings) was initially used to confirm the general location of the sound producer (n = 1). More specific ablation attempts, similar to those used on Tineinae, were unsuccessful in the three remaining E. sarcitrella individuals. For the successful ablations of M. crocicapitella and T. pellionella, recordings were made from two treatments for each moth, firstly the right hyaline patch was ablated, and secondly the left hyaline patch was ablated.
Tineinae ablation was achieved using a size 0.14 mm diameter insect pin under a 50× magnification dissection microscope (Leica EZ5 Stereo Microscope, Leica Microsystems, Wetzlar, Germany). Moths were anaesthetised using CO2 and secured to foam by placing insect pins in a cross over (not penetrating) both the abdomen and head of the insect, as well as individual pins over the fore and hindwings to hold them extended from the body, thus exposing the hyaline patches. The patch was then punctured with an insect pin and the membrane removed using fine forceps and microdissection scissors. All pins were removed, and the insect was positioned within the recording set-up, holding a small piece of paper or foam. It was left for between 15 and 120 min to recover and checked every 15 min for pre-ablation flight behaviour, and post-ablation recordings were subsequently made.
Acoustic Analysis
We analysed all acoustic recordings using Avisoft SASLab Pro (version 5.2.07, Avisoft Bioacoustics, Berlin, Germany), measuring the following acoustic characteristics for each species: source level, peak frequency, high and low frequency (bandwidth), click detection distance, shorter click burst click duration, longer click burst click duration, duty cycle, number of clicks per burst, and number of clicks per wingbeat. We analysed 10 consecutive wingbeats from a steady flight period with consistently high amplitude click bursts for each individual.
Acoustic characteristics were determined using the following methods. For each individual, click bursts from ten consecutive wingbeats were analysed. There are two click bursts per wingbeat cycle, defined here as longer and shorter as we did not confirm which burst occurred during the up- and down-stroke, respectively. We counted all clicks and further analysed the loudest click from each longer click burst. Click number was determined by totalling the number of clicks discernible in waveform for each of the two click bursts per wingbeat. The duration of each individual click was measured from the waveform. Click amplitude was determined following O’Reilly et al. (2019), by initially calculating the peak-to-peak sound pressure and converting it to dB peSPL, using a calibration tone from a signal generator which produced a constant tone of known amplitude at 40 kHz (Avisoft Bioacoustics, Berlin, Germany) and the following formula:
Clicks were extracted from the waveform individually for spectral analysis including 0.05 ms of margin noise, with silent margins added (zero padding) and linearly ramped into the noise. Peak frequency and bandwidth (high and low frequencies) were measured from power spectra (Hamming window size 1024), high and low frequencies were determined as the frequencies −15 dB either side of the peak frequency.
Monopis crocicapitella, T. pellionella, and E. sarcitrella click detection distances by bats were calculated from the loudest click from each of the longer bursts from ten successive wingbeats. Following O’Reilly et al. (2019) click peak frequency and amplitude (dB peSPL) were used to calculate the distance at which these sounds could be detected by bats, using a 10 dB SPL bat hearing threshold.
Additionally, video footage was taken using a smartphone at 120 fps of T. pellionella, M. crocicapitella, and E. sarcitrella in order to determine their wingbeat frequency, so it could be linked to the frequency of click burst production. The quality of these videos was sufficient to determine wingbeat frequency, but did not provide clarity or frame rates capable of creating useful synchronised high-speed video and audio recordings.
Hearing Tests
Prior to free-flight recordings, every moth in the flight cage was exposed to an ultrasonic stimulus known to elicit the anti-bat behaviours of moths with hearing capabilities (St. Juliana et al., 2007), at a distance of around one metre from the centre of the cage. Moths were exposed to the stimulus both at rest and during flight, and their behaviours observed. The observer was not blind to the treatment, as personnel availability and time constraints with limited numbers of live animals made this impractical. A Dazer II Ultrasonic Dog Deterrent (Dazer International, London, United Kingdom) was used as the stimulus; it produces a 25 kHz tone at 118.1 dB SPL (at 0.1 m). Reactions were defined as a sudden cessation of flight, or any other typical anti-bat escape/avoidance manoeuvre (Miller and Surlykke, 2001), or twitching, commencement of flight, or dropping from its perch if the moth was at rest.
If multiple individuals of the same species were caught on the same day, they were placed in the BugDorm-1 together and their behaviour in response to flight, and therefore sound production, of other individuals was observed. This was possible once each for M. crocicapitella (two individuals), T. pellionella (two individuals), and E. sarcitrella (two individuals).
Results
Phylogenetics
Phylogenetic Spread of ATs in the Microlepidoptera
Photographs or museum specimens of 19,596 species (2,440 genera, 50 families, 11 superfamilies) were morphologically examined for the presence of candidate ATs in the form of hyaline patches. The results were plotted on a simplified version of Regier et al.’s (2013) lepidopteran phylogeny (Figure 1). Candidate ATs were found throughout the microlepidoptera in nine of the eleven superfamilies assessed (Figure 1).
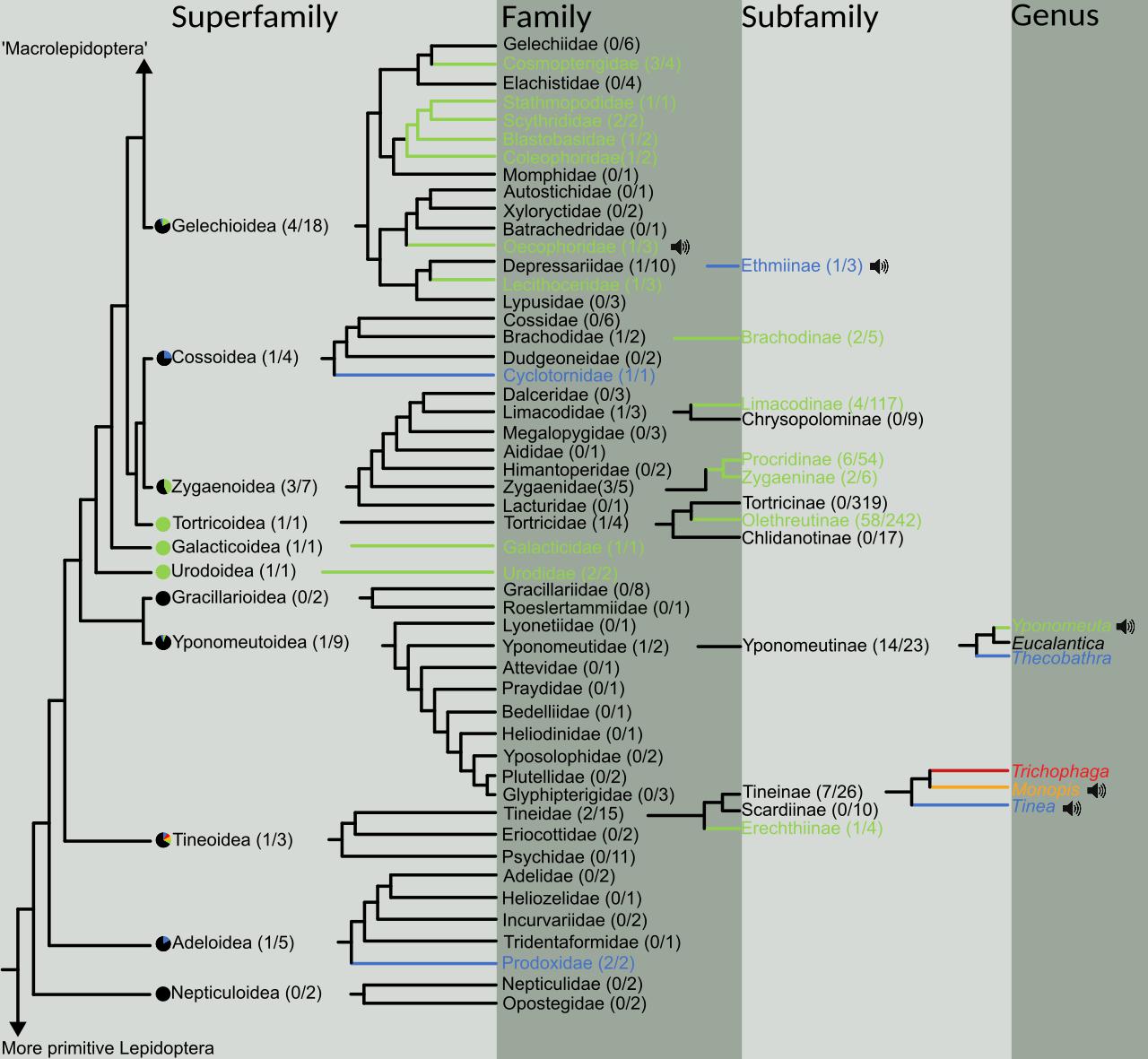
Figure 1. Phylogeny of the “microlepidoptera” (here defined as taxa below and including the superfamily Gelechioidea, and above and including Nepticuloidea) adapted from Regier et al. (2013). The spread of aeroelastic tymbals (ATs) is represented at various taxonomic levels, beginning with superfamilies and ending in genera. For each taxonomic level above genus, the fraction of subtaxa possessing ATs is given in parentheses. Following superfamily, if ATs are present, all families are presented, and then only relevant subfamilies (i.e., possessing ATs or showing evolutionary relationships). In subfamilies with multiple types of AT (see Figure 2), a genus tree is presented to show evolutionary relationships. Pie charts before the superfamilies represent the ratio of families containing ATs. Slice colours correspond to the location of the AT on the wing and match Figure 2, and black represents no obviously detectable structure. Taxa names and their branches are coloured if they contain examples of ATs, and colours again correspond to the locations in Figure 2. Black speaker icons indicate that those AT-possessing taxa have been recorded producing sound in flight (see Figure 4).
We identified ATs in four different locations on microlepidopteran wings (Figure 2) and named them as follows: (1) Forewing Subcostal Tymbal (FST) at the forewing base between the subcostal and radial veins in the cell directly above the discal cell (blue); (2) Forewing Discal Tymbal (FDT) directly within the apex of the discal cell itself (orange); (3) Forewing Cubital Tymbal (FCT) in the cell directly below the first cubital veins (red); and (4) Hindwing Cubital Tymbal (HCT) at the base of the hindwing in the cell directly below first cubital veins (green).
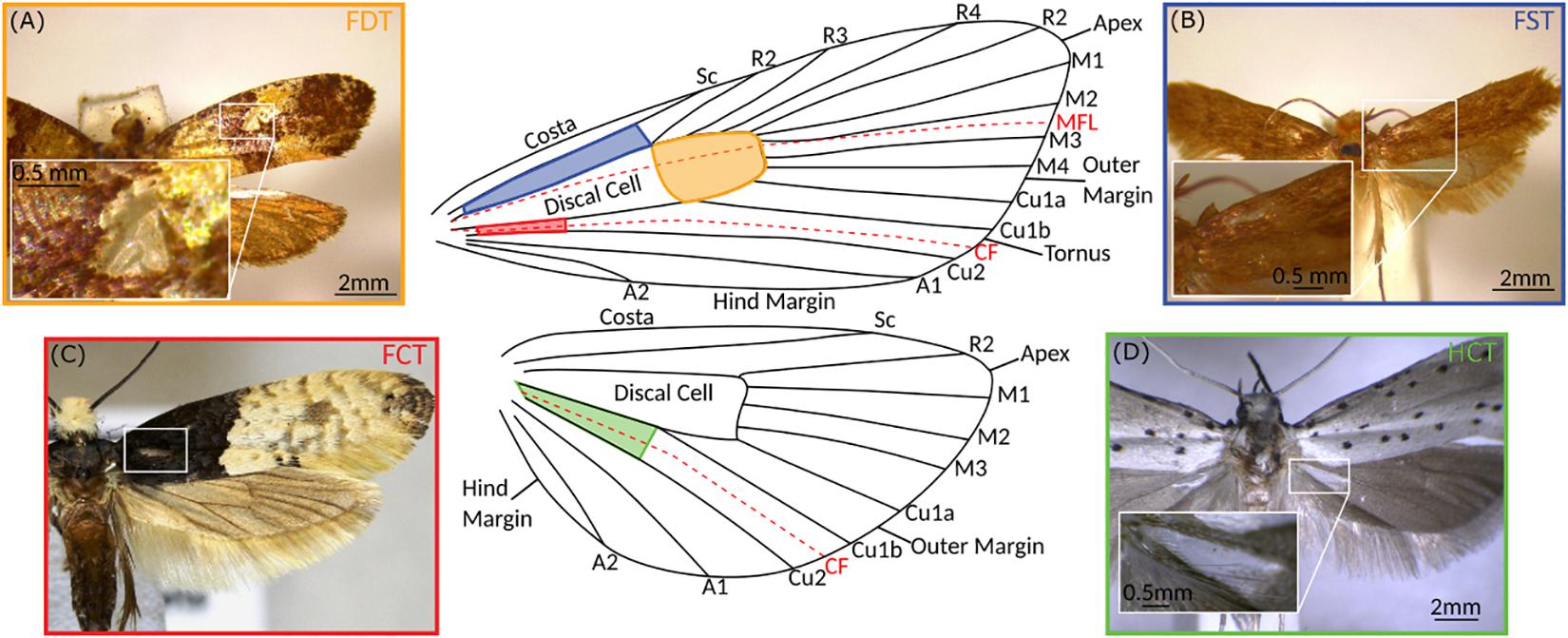
Figure 2. Aeroelastic tymbals of the microlepidoptera. Typical examples of macrolepidopteran aeroelastic tymbals for each of the four locations in which they are found on the wings. (A) Forewing Discal Tymbal (FDT) represented by Monopis crateroxantha (Meyrick, 1927; Tineidae), (B) Forewing Subcostal Tymbal (FST) represented by Tinea pellionella (Linnaeus, 1758; Tineidae), (C) Forewing Cubital Tymbal (FCT) represented by Trichophaga tapetzella (Linnaeus, 1758; Tineidae), and (D) Hindwing Cubital Tymbal (HCT) represented by Yponomeuta cagnagella (Hübner, 1813; Yponomeutidae). The colours of the photograph borders correspond to the location of the tymbal on the generalised Lepidopteran wing in the centre (modified from Watson and Dallwitz, 2003 onward), as well as the colours used in Figures 1, 3. Shaded areas show locations of tymbals, and dashed red lines represent flexion lines in the wing, the median flexion line (MFL) or the “fold” and the claval furrow (CF). Vein labelling: Sc (Subcosta), R (Radial), M (Medial), Cu (Cubital), and A (Anal), followed by vein number. Photograph (C) was taken from BOLD Systems (Ratnasingham and Hebert, 2007), the photographer was Marko and the image has a CC0 licence.
Phylogenetic Spread of ATs in the Tineidae
Analysis of 751 species from 148 genera in 14 subfamilies (as assigned by BOLD systems) of the Tineidae revealed that hyaline patches, likely to be ATs, were present in at least 46 species in eight genera, within the family, seven of which were in the subfamily Tineinae. The Tineidae contain examples of ATs in all four wing locations.
Forewing Subcostal Tymbals are present in 11 of 38 species of Tinea as well as one of two Praeacedes and four of five Niditinea species examined, including the newly discovered species N. sabroskyi (Metz et al., 2018). FSTs in the Tineinae vary in relative size, with the more conspicuous examples being found in Tinea steueri (Robinson, 1979), whereas, species such as Tinea dubiella possess much smaller structures.
Forewing Discal Tymbals are present in all Monopis analysed (26 species) as well as the genera Crypsithyrodes (one species) and Crypsithyris (four species), Tinea unomaculella possesses a light spot in the same area but we believe this is colouration not a tymbal. FDTs can vary in their size, shape (relatively round to elongated), and their location on the wing in terms of their position along the wing tip to base axis. Nevertheless, the structures always appear to be situated within the discal cell of the forewing and their position is likely due to differences in the length of this cell.
Forewing Cubital Tymbals are small (∼1 mm in length) hyaline patches near the base of the forewing, likely between veins Cu1b and Cu2 (Figures 2, 3), and are limited to the genus Trichophaga, present in at least three of the six species analysed. Additionally, the previous analysis using the Regier et al. (2013) phylogeny revealed HCTs in the Erechthiinae genus Erechthias. This genus was not, however, included in our phylogenetic analysis of the Tineidae.
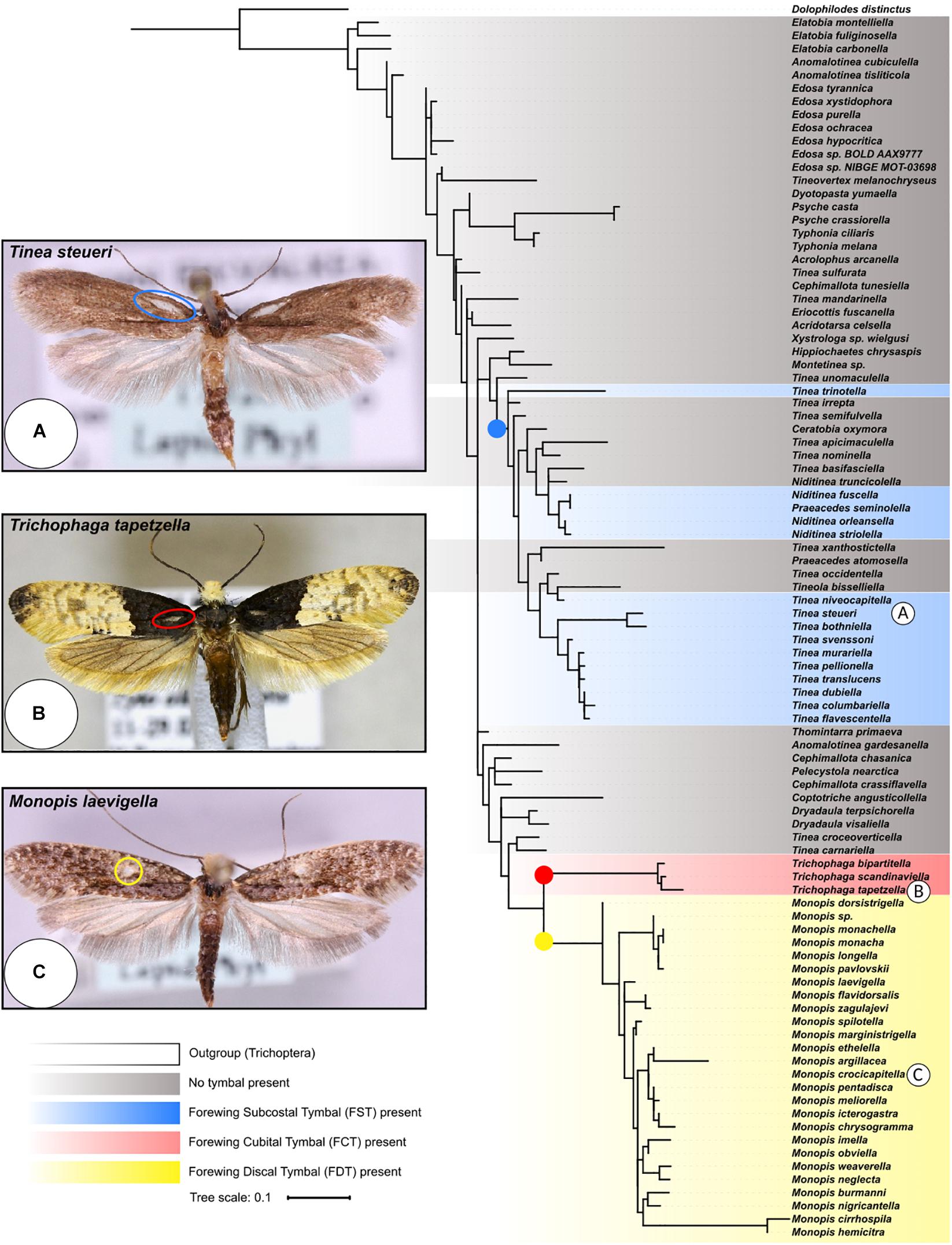
Figure 3. Rooted phylogenetic tree of Cytochrome Oxidase Subunit 1 (COI) gene from the Tineidae family. The maximum likelihood tree was inferred in IQ-tree (Nguyen et al., 2015) from an amino acid alignment of the COI gene from 90 species of moth and one caddis fly - Dolophilodes distinctus (Philopotamidae: Trichoptera) was used as an outgroup to root the tree. Bayesian Inference Criterion was used to select the best-fitting substitution model (LG + C60 + G), and bootstrap supports from branches were calculated using UFBoot2 (Minh et al., 2013). The three Tineinae ATs (FSTs, FCTs, and FDTs) are labelled on an example species of each (A–C are Tinea steueri, Petersen, 1966; Trichophaga tapetzella, Linnaeus, 1758; and Monopis laevigella, Denis and Schiffermüller, 1775 respectively). Coloured nodes indicate likely origins of the three ATs. Colours correspond to the AT position on the wing detailed in Figure 2. Photographs taken from BOLD Systems (Ratnasingham and Hebert, 2007), all are CC0 licence and taken by Marko Mutanen.
The maximum likelihood tree (Figure 3), groups all Monopis as a single clade, with the three Trichophaga species forming a sister clade. The FST-possessing species do not form a single clade but are instead split into two main clades with one species, T. trinotella, placed away from these two groups. The first of these two clades exclusively contains Niditinea and Praeacedes species, and the second exclusively Tinea species. The second, with the addition of T. columbariella and T. niveocapitella, consists of the already established T. pellionella species complex (Robinson, 1979). Tineola bisselliella (no hyaline patch) is placed as the only non-Tinea species in a clade containing mostly FST-possessing species.
Acoustics
Microlepidopteran Sound Production
Live specimens from three Tineinae and two Oecophoridae species were available for acoustic testing. All three species possessing hyaline patches, M. crocicapitella (forewing patch), T. pellionella (forewing patch), and E. sarcitrella (Yponomeuta-like hindwing patch), produced two bursts of broadband ultrasonic clicks with every wingbeat (Figure 4). These clicks are acoustically similar to the in-flight clicks produced by the ATs of Yponomeuta species (Yponomeutidae) (O’Reilly et al., 2019) in that they show a bimodal regularity (likely two different bursts per wingbeat, one on the up- and one on the down-stroke), they exclusively occur during flight, and the two bursts differ in duration (Figure 4).
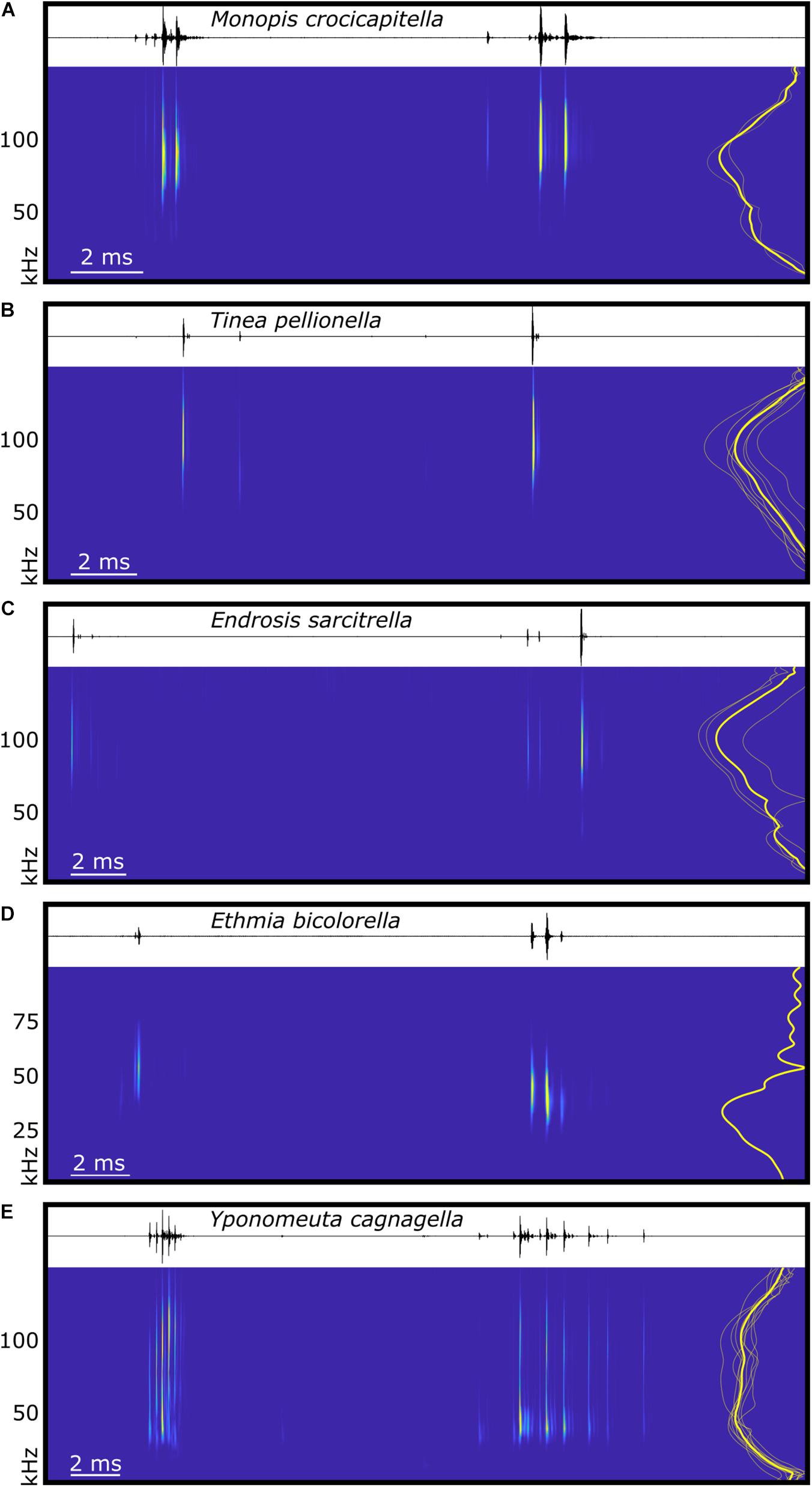
Figure 4. Spectral and temporal characteristics of microlepidoptera sounds. The waveform and spectrogram (derived from continuous wavelet transformation using the Morse wavelet) of typical examples of in-flight acoustic emissions of five species of microlepidoptera, (A) Monopis crocicapitella (Tineidae), (B) Tinea pellionella (Tineidae), (C) Endrosis sarcitrella (Oecophoridae), (D) Ethmia bicolorella (Depressariidae), and (E) Yponomeuta cagnagella (Hübner, 1813; Yponomeutidae). Each panel represents one full wingbeat showing the two bursts of clicks produced with each wingbeat cycle, beginning with the first click of the burst with the shortest inter-click interval, and ending immediately prior to the first click of the next equivalent burst. To the right of each spectrogram is a power spectrum showing the normalised click amplitude for the species mean (thick yellow line) and individuals (thin translucent yellow lines, for each species n see section “Materials and Methods”). Time scales vary between plots, (D) uses a different frequency scale, and spectrograms are not calibrated for amplitude.
Both species lacking hyaline patches, T. bisselliella and H. pseudospretella, did not produce any acoustic emissions during flight. Males of T. bisselliella are known to produce low frequency substrate-borne sounds (Takács et al., 2003), yet we were not attempting to record such substrate-borne vibrations.
Successful ablation of the hyaline patches of both M. crocicapitella and T. pellionella (n = 1) eliminated sound production, whilst ablation of one hyaline patch, leaving the other intact, effectively halved the number of clicks produced per wingbeat, 22.95 ± 3.4 and 3.8 ± 0.9 pre ablation, and 12.9 ± 1.1 and 2.2 ± 0.4 post ablation (mean ± SD) for M. crocicapitella and T. pellionella, respectively. Removal of both E. sarcitrella hindwings eliminated sound production.
One Monopis cf monachella individual was found to produce ultrasonic emissions during flight using an ultrasonic bat detector, and recordings from one E. bicolorella in free flight in Kenya confirm alternating bursts of ultrasonic clicks characteristic of AT sound production (Figure 4D). Additionally, all four South African Ethmia species recorded in free flight produces similar bursts of clicks (data not shown).
Acoustic Characterisation of AT Sounds
We analysed 20 bursts and 10 individual clicks from M. crocicapitella, T. pellionella, and E. sarcitrella for amplitude, spectral, temporal, and duration information. In addition, we calculated the distance at which bats could detect these clicks (Table 2). All three species produce relatively loud (64.6, 56.9, and 54.0 dB peSPL at 0.1 m, respectively) ultrasonic, broadband clicks (41.2–111.7, 54.3–125.1, and 45.8–128.9 kHz, respectively) with high peak frequencies (88.1, 92.1, and 100.0 kHz, respectively) (Table 2).
The sounds of all three moth species fall within the known frequency range of anti-bat sounds of the Arctiinae, Sphingidae, Geometridae, and Yponomeutinae (Corcoran et al., 2010; Barber and Kawahara, 2013; Corcoran and Hristov, 2014; O’Reilly et al., 2019). Their low duty cycles (Table 2) are also similar to some of the aposematic signalling Arctiinae such as Cosmosoma stibasticta and Amplicincia near mixta (Corcoran et al., 2010). Example ultrasonic click burst recrodings of Endrosis sarcitrella, Ethmia bicolorella, Monopis crocicapitella, and Tinea pellionella can be found in Supplementary Audio 1–4 respectively.
Hearing Tests
All live individuals (excluding E. bicolorella) were exposed to a sound source known to elicit the anti-bat behaviours in insects with hearing capabilities (St. Juliana et al., 2007). No individual of any species showed any reaction, such as cessation or initiation of flight, sudden movement, or any change in flight direction. Additionally, when the insects were obtained as groups of two or more individuals, they were housed together and no individual was observed reacting to flight, and therefore sound production, of the other (n = 2 for all tested species).
Discussion
Distribution of ATs in the Microlepidoptera
Morphological analysis of 11 superfamilies highlighted that ATs are widely distributed in microlepidoptera (Figure 1). However, when we analysed the presence and absence of ATs on a subfamily and genus level it was apparent that ATs were a lineage-specific innovation, and that ATs can vary in both location and size. The exact number of evolutionary events is unclear; however, given we showed four different ATs in four locations on the wing (Figures 1, 2). When mapped onto a phylogenetic tree, the distribution of ATs either suggests multiple evolutionary events, or significant lineage specific losses of this organ, with the former being the most parsimonious explanation for the evolutionary history. We could confirm sound production in five independent ATs (Yponomeutinae, T. pellionella and E. bicolorella FSTs, Monopis FDTs, and E. sarcitrella HCTs). These ATs were found in three different wing regions, two of which had not been documented as sound producing structures before. Our analysis suggests a remarkable example for multiple convergent evolution. The fact that all candidate ATs we were able to test with live specimens indeed produced sound, inspires confidence in the validity of our approach to identify ATs using hyaline wing patches. This does not confirm that a lack of scales is a prerequisite for an AT though.
Aeroelastic Tymbal Morphology and Function
The positioning of ATs on the wing may provide some insight into how they function. Interestingly, all four AT locations place them near flexion lines (Figure 2). Flexion lines are lines along which an insect wing shows flexibility (folding) during the wingbeat. The claval furrow is a flexion line found in most insect wings, and the median flexion line (sometimes referred to as the “fold”) is found in the forewing (and occasionally hindwing) of many insect taxa, and usually runs between the medial and radial veins (Dudley, 2000).
The hindwing claval furrow appears to play a role in Yponomeuta HCT actuation (O’Reilly et al., 2019), and thus it is reasonable to assume it has similar importance in other taxa with HCTs. FCTs are located analogously to Yponomeuta ATs but in the fore- not hindwing, and thus, if these are sound producers, the claval furrow is again likely to play a role in actuation.
The median flexion line is not always present in insect wings, and its position on the wing when present can vary between taxa (Wootton, 1979); however, its normal location transects the discal cell and therefore FDTs. Additionally, FSTs are near the median flexion line if it passes through the discal cell, but there is also the possibility that in these taxa it may be situated even closer to the tymbal. It is therefore reasonable to predict that FDT and FST actuation is facilitated by this flexion line.
Aeroelastic tymbal location seems to be associated with flexion lines, but strong supporting structures appear important too, as Monopis, Crypsithyrodes, and Crypsithyris discal cells have thickened veins surrounding their ATs (Robinson, 1980; Huang et al., 2011). This apparent importance of flexion lines and strong supporting structures in AT location, and probably in actuation, provides important indicators for mechanical modelling of these novel sound-producing systems.
Other than the known tymbals of Amyna natalis (Noctuidae), which are used for sexual communication and are not perpetually active during wing movement (Heller and Achmann, 1993), there are no obvious AT candidates in the macrolepidoptera. This exclusivity and convergence of ATs within the microlepidoptera suggests that a property of their wings gives them a propensity to evolve into sound producers. Therefore, differences between macro- and microlepidoptera in wing elastodynamics and structure of wing and wing membrane would be another important area of investigation. The most obvious difference between macro- and microlepidoptera is their size and we believe this is the most likely morphological factor facilitating sound production. Wing cell size may be of particular importance, as the smaller spaces between wing veins could allow for the formation of appropriately sized tymbals in micro- but not macrolepidoptera. Tymbal size is likely to be a factor in determining acoustic characteristics such as frequency; thus, the cell sizes in macrolepidopteran wings may not allow for ATs that produce clicks with frequencies appropriate for their function, e.g., anti-bat sound production.
Acoustics
All species possessing candidate ATs we were able to assess produced ultrasonic clicks linked to their wingbeat. The oecophorid species E. sarcitrella produces its sounds using its hindwings and has a hyaline patch at the same position as the known Yponomeuta HCT. T. pellionella and M. crocicapitella (Tineinae; Tineidae) have hyaline/subhyaline patches on their forewings that produce sound during wingbeats, most likely functioning similar to the hindwing HCTs of Yponomeuta and its relatives (O’Reilly et al., 2019). The Ethmia species assessed (Depressariidae) also produce sounds during flight and possess a subhyaline patch in a similar position to T. pellionella, but a lack of ablation tests prevented confirmation that this is the sound producer.
Several further lines of evidence corroborate that hyaline/subhyaline patches of T. pellionella, M. crocicapitella, and E. sarcitrella are functioning as ATs: firstly, only the species possessing candidate structures produced sounds, the two species lacking structures were silent. Secondly, like Yponomeuta sounds, the clicks of all these moths occur in two bursts every wingbeat, with one burst likely occurring during the upstroke and the other during the downstroke. Thirdly, for both Tineinae species, ablation of both hyaline patches eliminated sound production, and ablation of one of the two patches did not result in a change in the periodicity of the click bursts, instead halving the total number of clicks per wingbeat. This demonstrates that each tymbal is producing half the total number of clicks per wingbeat, that each tymbal contributes to both click bursts, and that the body of the moth does not prevent clicks from one wing reaching the opposite side. Although specific ablation of the hyaline patches of E. sarcitrella was unsuccessful, the removal of the hindwings eliminated sound production, and there is no other obvious candidate structure on these wings. Additional support comes from the location of the E. sarcitrella hyaline patch being indistinguishable from that of Yponomeuta HCTs.
We believe that, like other tymbals, ATs produce sound through bimodal buckling, and that the two click bursts each moth produces per full wingbeat are the two stages of its ATs buckling and then returning to their resting state. The exact biomechanical mechanism by which these tymbals are actuated was beyond the scope of this study and requires complex modelling, but we propose that, similarly to Yponomeuta HCTs (O’Reilly et al., 2019), twisting and folding of the wing (likely along flexion lines, e.g., claval furrow or median flexion line) during flight are important, as are strong supporting structures such as thickened veins.
Structurally, all three tymbals resemble Yponomeuta HCTs; they consist of similarly sized hyaline patches with few or no scales between two often strong veins. However, unlike Yponomeuta HCTs, they do not possess obvious microtymbals. Microtymbals are striations running the length of a tymbal, each functioning to produce an individual click in sequence following tymbal actuation, resulting in the production of bursts (trains) of clicks. Following initial tymbal buckling each microtymbal buckles in sequence producing a train of individual clicks, and then upon the return of the tymbal to its resting state the same process occurs in reverse order, producing a second click train.
The low click number in T. pellionella and E. sarcitrella click bursts is consistent with a lack of microtymbals; however, the higher click number in M. crocicapitella bursts suggests that this species may possess an alternative mechanism. Raised “bumps” are visible on the FDTs of some Monopis species, which may be analogues of microtymbals (Figure 2A).
Function of Sounds
The acoustic emissions of all recorded species most likely function as anti-bat sounds. The ultrasonic, broadband nature of the clicks is similar to the known anti-bat sounds of other moths (Corcoran et al., 2010; Barber and Kawahara, 2013; Corcoran and Hristov, 2014; O’Reilly et al., 2019) and they are loud enough to be detected by bats.
The maximum distances over which these sounds will be audible to bats is lower than Yponomeuta clicks (5.9, 4.1, and 3.4 m for M. crocicapitella, T. pellionella, and E. sarcitrella, respectively compared to 10.5 m for Yponomeuta cagnagella; O’Reilly et al., 2019). This is due to increased atmospheric attenuation of the sounds due to much higher peak frequencies, and for T. pellionella and E. sarcitrella sounds, lower source levels.
The different number of clicks per wingbeat might not necessarily have substantial biological relevance. T. pellionella and E. sarcitrella produce much fewer, lower amplitude clicks per burst (normally one or two, but occasionally more, see Figure 4) than M. crocicapitella. However, producing fewer clicks does not mean that these sounds are less likely to function as a bat defence. Within the tymbal-possessing Arctiinae, many species produce click bursts but others, including the sympatric Arctia caja, do not possess microtymbals and thus produce one defensive click per tymbal buckling event (Fenton and Roeder, 1974; Surlykke and Miller, 1985).
Additional support for these sounds having an anti-bat function is the lack of any reaction from the moths to ultrasonic stimuli, whether generated artificially or by another individual. Although tympana have been reported in the Tineidae, this is a defining feature of the subfamily Harmacloninae (Davis, 1998), and there is no evidence they are present in the Tineinae. Similarly, there is no evidence in the literature that E. sarcitrella possesses hearing capabilities. Therefore, these moths cannot be communicating with conspecifics using airborne sounds. Constantly producing ultrasonic clicks detectable by bats that serve no communication purpose, seems counterintuitive, unless the sounds act as acoustic defence.
The precise defensive mechanism of these sounds for each species remains unclear, with relevant unknowns including moth toxicity and their propensity to spend time on the wing producing sound. It is clear though that the low duty cycles of all their sounds cannot jam bat echolocation (Table 2), as this requires a duty cycle of at least 20% (Corcoran et al., 2010; Conner and Corcoran, 2012). It is also unlikely that bats will be startled by these sounds as such a defence tends to be ephemeral and only effective against naïve bats (Bates and Fenton, 1990; Hristov and Conner, 2005). This suggests that the sounds function as aposematic signals, as either Batesian (imposter) or Müllerian (true) mimics of acoustically aposematic moths such as the Arctiinae and Yponomeuta.
Phylogenetic Spread of ATs Within the Tineinae
There are two lines of support for the convergent evolution of anti-bat sound production by ATs within the Tineinae subfamily. Firstly, the structures are morphologically similar in many aspects, but are sufficiently different in shape and position on the wing to suggest multiple evolutionary origins. Secondly, based on their phylogeny, the distinctly separate Monopis, Trichophaga, and Tinea-like clades suggests three points of evolutionary origin for Tineinae ATs (Figure 3). The Tinea-like clade is particularly interesting as FSTs appear in three distinct lineages. The ancestor of both the Tricophaga and Monopis lineages probably had no AT, suggesting that the FCT and FDT structures found in these lineages evolved independently. Moreover, the structures are found on different locations on the wing, providing further evidence they are not homologous, and thus, the result of convergent evolution. Additionally, the distribution FSTs found in the Tinea and Niditinea species suggests either the common ancestor of all these species possessed an FST and there has been lineage specific loss, or that there has been a minimum of three independent evolutionary events of this structure, and convergent evolution has occurred. The phylogeny from which we draw our conclusions was constructed with a single marker gene, leading to species level branches only having weak bootstrap support. Thus, the conclusions on a species level must be re-assessed when more data become available and more robust phylogenies can be constructed. The distribution of FST, FCT, and FTD possessing species on the phylogenetic tree clearly indicates three independent evolutionary events of sound producing structures, highlighting remarkable convergent evolution on a subfamily level.
Anti-bat sound production in the Tineinae is exceptionally diverse as it has evolved convergently several times within one subfamily. Convergent evolution of bat defences in the Lepidoptera is common, and has occurred in terms of hearing, sound production, and hindwing decoys (Corcoran and Hristov, 2014; Barber et al., 2015; ter Hofstede and Ratcliffe, 2016), but it rarely, occurs between such closely related taxa as within one subfamily. Similar levels of convergence appear to have occurred within Saturniidae (silkmoth) subfamilies with regards to acoustic wing decoys (Rubin et al., 2018). These two examples of subfamily level converge in moths reiterate how important the bat-moth coevolutionary arms race is as a case study for evolutionary principals.
Thoughts on the Evolution of ATs in Cave-Dwelling Taxa
Records of troglophilic (cave-dwelling) invertebrates from various cave systems globally indicate that Tineidae are widely present, particularly in tropical and subtropical regions of the Americas as well as the Balkan states and Australia (Barr and Reddell, 1967; Hamilton-Smith, 1967; Peck, 1975, 1974; Robinson, 1980; Trajano, 2000; Humphreys and Eberhard, 2001; Cokendolpher and Polyak, 2004, 1996; László, 2004; Wynne and Pleytez, 2005; Wynne et al., 2005; Polak et al., 2012; Byun et al., 2014; Eberhard et al., 2014; Pape, 2014; Silva and Ferreira, 2015; Turbanov et al., 2016; Jakšić, 2017). The Tineidae is a cosmopolitan lepidopteran family (Slootmaekers, 2013), and so it is highly likely that tineids are present in cave systems globally, but records are lacking.
Larvae of the subfamily Tineinae feed on animal detritus including bat guano, resulting in independence from green plants. This independence allows these moths to permanently inhabit environments such as caves. Indeed, at least 11 Tineidae species (including species of the Tineinae genera Monopis, Crypsithyrodes, Crypsithyris, Tinea, Niditinea, and Praeacedes) are known to spend their entire lives within caves feeding as larvae on bat guano or the fungi that grow on it (Robinson, 1980). In addition, E. sarcitrella is also found in caves and bat roosts (Mosconi, 2011; Centelles Bascuas, 2015). E. sarcitrella is a pest of stored grain but is known to be able to subsist on guano and other organic matter (Carter, 1984).
All these troglophilic moth species thus exist alongside bats, feeding on the faeces of their potential predators, which puts them at a perpetual risk of predation. It seems counterintuitive for moths to have initially adapted to live on the faeces of their predators; indeed, guanophagy in cave-dwelling microlepidoptera may have originated before bats, with moths perhaps feeding on bird guano.
Guanophagous moths will indiscriminately feed on bird or bat guano, and sometimes other animal products, including bird feathers (Robinson, 1980). With birds having evolved considerably earlier than bats (Kumar and Hedges, 1998), it is plausible that the ancestral cave-dwelling, guanophagous tineid shared its abode with cave-roosting birds, like extant swiftlets or oilbirds. A cave can provide a geographic mating barrier to populations, and as multiple Tineinae species can spend their entire lives living in caves (Robinson, 1980), ancestral moth populations could have become isolated in caves, leading to speciation. Then, following the evolution of echolocating bats and their colonisation of caves, this strong predation pressure, the geographic isolation, and an apparent propensity for wings to be sound producers could have resulted in the convergent evolution of ATs in the Tineinae.
Cave-Dwelling Microlepidopteran Acoustics
We already established that the sounds produced by the species we recorded most likely function as acoustic aposematic signals, but whether these moths are Batesian or Müllerian mimics of other aposematic moths depends on their toxicity which is unknown. Toxicity in Lepidoptera is derived from sequestering secondary metabolites from food and/or synthesising compounds (Rothschild et al., 1970). Both faeces and the fungi that grow on it could conceivably provide noxious compounds to sequester for the acoustically active Tineinae and E. sarcitrella, or equally they could synthesise such compounds.
Bats will learn over time to ignore acoustic Batesian signals (Barber and Conner, 2007), so if these cave-dwelling moths are palatable, they then risk becoming acoustically conspicuous targets. Therefore, the persistence and convergent evolution of sound production within this group of moths suggests that they are truly aposematic. An interesting thought is that naïve juvenile bats might first learn to avoid clicking moths from within their roosts.
Alternatively, reducing the exposure of bats to these acoustic signals could allow Batesian mimicry to persist. If these moths preferentially avoid flight, and instead crawl atop the guano, they will avoid sound production. This would prevent saturating the bats with a potentially Batesian signal and therefore reducing the effectiveness of sound production as a defence. The lower detection range of the clicks we recorded compared to non-cavernicolous Yponomeuta and macrolepidoptera (e.g., Corcoran et al., 2010; O’Reilly et al., 2019) may be beneficial in this respect; limiting their detectability to a distance at which bats are close enough to pose a threat.
A second, not necessarily separate, scenario in which Batesian mimicry could persist may arise if the ratio of Müllerian to Batesian mimics bats encounter is so high it is not worth risking attacking any clicking target. If the amount of sound producing moths in the bats’ hunting environment is above a certain threshold, then they will be regularly exposed to true aposematic signallers when foraging, reinforcing the effectiveness of acoustic aposematism. Within the roost, palatable cave-dwelling microlepidoptera could then “piggyback” on the protection afforded by ultrasound production, and reduced acoustic conspicuousness and/or flight could maintain the effectiveness of their signals.
Everything considered, based on the similarities of their sounds with those of aposematic moths, their lack of both hearing and, therefore, intraspecific communication, as well their unusual feeding ecology in close proximity to bats, we conclude that M. crocicapitella, T. pellionella, their tymbal-possessing relatives (Figure 3), and E. sarcitrella are mimics of acoustically aposematic moths. We cannot, however, state with confidence whether they are Batesian or Müllerian mimics.
Conclusion
The bat-moth evolutionary arms race is an area of much research interest for both sensory ecologists and evolutionary biologists, and yet a huge number of taxa remain underrepresented in the current literature. Microlepidoptera are largely ignored in terms of this topic, and our findings highlight that this suborder is greatly understudied. The remarkable level of convergence in anti-bat sound producing structures is further evidence in support of microlepidoptera being under significant selection pressure from bat predation. As a result of this pressure, the array of acoustic defences these moths possess are probably just as complex and diverse as their larger cousins, and they undoubtedly deserve increased research attention. Here, we begin a new chapter in the bat-moth coevolutionary arms race; the acoustic anti-bat defences of the microlepidoptera.
Data Availability Statement
The original contributions presented in the study are included in the article/Supplementary Material, further inquiries can be directed to the corresponding author.
Author Contributions
LO conceived the study, performed all images, and museum specimen analysis, all laboratory-based acoustic recordings and analysis, and wrote the original manuscript draft. DA made acoustic recordings of Ethmia species in Kenya and South Africa, provided live Tineidae specimens, and provided advice on microlepidoptera. BH performed the phylogenetic analysis of the Tineidae and created the cladogram in Figure 3, as well as providing advice on the other phylogenetic aspects of the project. MH provided funding for LO, research methods and equipment, confirmed sound production in Monopis cf monachella, offered advice on acoustic analysis and contributed to later drafts of the manuscript. All authors contributed to the article and approved the submitted version.
Funding
LO was supported during this project by the University of Bristol Graduate Teaching Assistant Ph.D. Scholarship awarded to MH and is currently supported by the Swiss National Science Foundation Spark grant CRSK-2_190855. BH was supported by a New Phytologist Trust Ph.D. studentship. MH was supported by the Biotechnology and Biological Sciences Research Council (grant BB/N009991/1) and the Engineering and Physical Sciences Research Council (grant EP/T002654/1).
Conflict of Interest
The authors declare that the research was conducted in the absence of any commercial or financial relationships that could be construed as a potential conflict of interest.
Acknowledgments
For allowing us to examine their Lepidopteran collections, we would like to thank Bristol Museum and Art Gallery and the Natural History Museum, London, and their trustees. We offer particular thanks to Ray Barnett, Rhian Rowson, and David Lees.
Supplementary Material
The Supplementary Material for this article can be found online at: https://www.frontiersin.org/articles/10.3389/fevo.2021.648223/full#supplementary-material
Supplementary Audio 1–4 | Recordings of in-flight microlepidopteran sounds. Audio 1–4 contain multiple ultrasonic click bursts from Endrosis sarcitrella, Ethmia bicolorella, Monopis crocicapitella, and Tinea pellionella respectively.
References
Barber, J. R., and Conner, W. E. (2007). Acoustic mimicry in a predator-prey interaction. Proc. Natl. Acad. Sci. U. S. A. 104, 9331–9334. doi: 10.1073/pnas.0703627104
Barber, J. R., and Kawahara, A. Y. (2013). Hawk moths produce anti-bat ultrasound. Biol. Lett. 9, 1–5.
Barber, J. R., Leavell, B. C., Keener, A. L., Breinholt, J. W., Chadwell, B. A., McClure, C. J. W., et al. (2015). Moth tails divert bat attack: evolution of acoustic deflection. Proc. Natl. Acad. Sci.U.S.A. 112, 2812–2816. doi: 10.1073/pnas.1421926112
Barr, T. C., and Reddell, J. R. (1967). The arthropod cave fauna of the carlsbad caverns region, New Mexico. Southwest. Nat. 12, 253–273. doi: 10.2307/3669113
Bates, D. L., and Fenton, M. B. (1990). Aposematism or startle? Predators learn their responses to the defenses of prey. Can. J. Zool. 68, 49–52. doi: 10.1139/z90-009
Byun, B. K., Shin, S. B., Bae, Y. S., Kim, D. S., and Choi, Y. G. (2014). First discovery of a cave-dwelling tineid moth (Lepidoptera, tineidae) from East Asia. J. For. Res. 25, 647–651. doi: 10.1007/s11676-014-0503-9
Carter, D. J. (1984). in Pest Lepidoptera of Europe With Special Reference to the British Isles, ed. W. Junk (Netherlands: Springer).
Centelles Bascuas, R. (2015). Les papillons des grottes Essai d’inventaire français et européen et indications bibliographiques. Spelunca 140, 1–3.
Clemens, B. (1859). “Contributions to american lepidopterology,” in Proceedings of the Academy of Natural Sciences of Philadelphia, Philadelphia, 256–262.
Cokendolpher, J. C., and Polyak, V. J. (1996). Biology of the caves at Sinkhole Flat, Eddy County, New Mexico. J. Cave Karst Stud. 58, 181–192.
Cokendolpher, J. C., and Polyak, V. J. (2004). Macroscopic invertebrates of Hidden and Hidden Chimney caves, Eddy County, New Mexico. Texas Meml. Museum Speleol. Monogr. 6, 175–198.
Conner, W. E., and Corcoran, A. J. (2012). Sound strategies: the 65-million-year-old battle between bats and insects. Annu. Rev. Entomol. 57, 21–39. doi: 10.1146/annurev-ento-121510-133537
Corcoran, A. J., Conner, W. E., and Barber, J. R. (2010). Anti-bat tiger moth sounds: form and function. Curr. Zool. 56, 358–369. doi: 10.1093/czoolo/56.3.358
Corcoran, A. J., and Hristov, N. I. (2014). Convergent evolution of anti-bat sounds. J. Comp. Physiol. A 200, 811–821. doi: 10.1007/s00359-014-0924-0
Criscuolo, A., and Gribaldo, S. (2010). BMGE (block mapping and gathering with entropy): a new software for selection of phylogenetic informative regions from multiple sequence alignments. BMC Evol. Biol. 10:210. doi: 10.1186/1471-2148-10-210
Davis, D. R. (1998). A world classification of the Harmacloninae, a new subfamily of Tineidae (Lepidoptera: tineoidea). Smithson. Contrib. to Zool. 597, 1–81. doi: 10.5479/si.00810282.597
Denis, M., and Schiffermüller, I. (1775). Ankündung eines systematischen Werkes von den Schmetterlingen der Wienergegend.
Dodd, L. E., Chapman, E. G., Harwood, J. D., Lacki, M. J., and Rieske, L. K. (2012). Identification of prey of myotis septentrionalis using DNA-based techniques. J. Mammal. 93, 1119–1128. doi: 10.1644/11-MAMM-A-218.1
Dudley, R. (2000). The Biomechanics of Insect Flight. Princeton, NJ: Princeton University Press, doi: 10.2307/j.ctv301g2x
Eberhard, S. M., Smith, G. B., Gibian, M. M., Smith, H. M., and Gray, M. R. (2014). Invertebrate Cave Fauna of Jenolan. Proc. Linn. Soc. NSW 136, 35–67. doi: 10.1109/FUZZ-IEEE.2017.8015520
Felder, C., Felder, R., and Rogenhofer, A. F. (1875). Reise der österreichischen Fregatte Novara um die Erde in den Jahren 1857, 1858, 1859 unter den Befehlen des Commodore B. von Wüllerstorf-Urbair. Zoologischer Theil. Zweiter Band. Abtheilung 2, Heft 4, Lepidoptera. Atlas der Heterocera.
Fenton, M. B., and Roeder, K. D. (1974). The microtymbals of some arctiidae. J. Lepid. Soc. 28, 205–211.
Hamilton-Smith, E. (1967). The Arthropoda of Australian Caves. J. Aust. Entomol. Soc. 6, 103–118. doi: 10.1111/j.1440-6055.1967.tb02123.x
Heller, K., and Achmann, R. (1993). The ultrasonic song of the moth Amyna natalis (Lepidoptera: noctuidae: acontiinae). Bioacoustics 5, 89–97. doi: 10.1080/09524622.1993.9753231
Hristov, N. I., and Conner, W. E. (2005). Sound strategy: acoustic aposematism in the bat-tiger moth arms race. Naturwissenschaften 92, 164–169. doi: 10.1007/s00114-005-0611-7
Huang, G.-H., Chen, L.-S., Hirowatari, T., Nasu, Y., and Wang, M. (2011). A revision of the Monopis monachella species complex (Lepidoptera: Tineidae) from China. Zool. J. Linn. Soc. 163, 1–14. doi: 10.1111/j.1096-3642.2011.00704.x
Humphreys, W. F., and Eberhard, S. (2001). Subterranean Fauna of Christmas Island, Indian Ocean. Helictite 37, 59–74.
Jakšić, P. (2017). Cave moth and butterfly fauna (Insecta: Lepidoptera) of serbia: current state and future prospects. Univ. Thought - Publ. Nat. Sci. 7, 8–12. doi: 10.5937/univtho7-14038
Katoh, K., and Standley, D. M. (2013). MAFFT multiple sequence alignment software version 7: improvements in performance and usability. Mol. Biol. Evol. 30, 772–780. doi: 10.1093/molbev/mst010
Kovalev, I. S. (2016). Stealth moths: the multi-plumed wings of the moth alucita hexadactyla may decrease the intensity of their echo to simulated bat echolocation cries. Entomol. News 126, 204–212. doi: 10.3157/021.126.0306
Kumar, S., and Hedges, S. B. (1998). A molecular timescale for vertebrate evolution. Nature 392, 917–920. doi: 10.1038/31927
Le, S. Q., and Gascuel, O. (2008). An improved general amino acid replacement matrix. Mol. Biol. Evol. 25, 1307–1320. doi: 10.1093/molbev/msn067
Lee, D. J., Ju, Y. D., Bayarsaikhan, U., Park, B. S., Na, S. M., Kim, J. W., et al. (2016). First report on two species of genus Monopis (Lepidoptera, tineidae) collected by feather trap in Korea. J. Asia Pacific Biodivers. 9, 215–218. doi: 10.1016/j.japb.2016.02.007
Lee, W., and Moss, C. F. (2016). Can the elongated hindwing tails of fluttering moths serve as false sonar targets to divert bat attacks? J. Acoust. Soc. Am. 139, 2579–2588. doi: 10.1121/1.4947423
Letunic, I., and Bork, P. (2016). Interactive tree of life (iTOL) v3: an online tool for the display and annotation of phylogenetic and other trees. Nucleic Acids Res. 44, W242–W245. doi: 10.1093/nar/gkw290
Metz, M. A., Davis, D. R., and Davis, M. M. (2018). A new species of niditinea (Tineidae: Tineinae) with a preference for bird nests, and the known larval habitats of the species in the United States. Proc. Entomol. Soc. Washingt. 120, 153–166. doi: 10.4289/0013-8797.120.1.153
Meyrick, E. (1913). Descriptions of South African Micro-Lepidoptera. IV. Ann. Transvaal Museum 3, 267–336.
Miller, L. A., and Surlykke, A. (2001). How some insects detect and avoid being eaten by bats: tactics and countertactics of prey and predator. Bioscience 51, 570–581. doi: 10.1641/0006-3568(2001)051[0570:hsidaa]2.0.co;2
Minh, B. Q., Nguyen, M. A. T., and Von Haeseler, A. (2013). Ultrafast approximation for phylogenetic bootstrap. Mol. Biol. Evol. 30, 1188–1195. doi: 10.1093/molbev/mst024
Möschler, H. B. (1883). Contributions to the butterfly fauna of the Kaffir country. Negot. Imp. Zool. Soc. 33.
Mosconi, F. (2011). Biologia Comparata Dei Princpali Lepidotteri Cavernicoli Italiani Nella Loro Ecofase Sotterranea. Ph. D. Thesis. Rome: Sapienza Università di Roma.
Neil, T. R., Shen, Z., Robert, D., Drinkwater, B. W., and Holderied, M. W. (2020a). Thoracic scales of moths as a stealth coating against bat biosonar. J. R. Soc. Interface 17:20190692. doi: 10.1098/rsif.2019.0692
Neil, T. R., Shen, Z., Robert, D., Drinkwater, B. W., and Holderied, M. W. (2020b). Moth wings are acoustic metamaterials. Proc. Natl. Acad. Sci. U.S.A. 117, 31134–31141. doi: 10.1073/pnas.2014531117
Nguyen, L. T., Schmidt, H. A., Von Haeseler, A., and Minh, B. Q. (2015). IQ-TREE: a fast and effective stochastic algorithm for estimating maximum-likelihood phylogenies. Mol. Biol. Evol. 32, 268–274. doi: 10.1093/molbev/msu300
Ntelezos, A., Guarato, F., and Windmill, J. F. C. (2017). The anti-bat strategy of ultrasound absorption: the wings of nocturnal moths (Bombycoidea: Saturniidae) absorb more ultrasound than the wings of diurnal moths (Chalcosiinae: Zygaenoidea: Zygaenidae). Biol. Open 6, 109–117. doi: 10.1242/bio.021782
O’Reilly, L. J., Agassiz, D. J. L., Neil, T. R., and Holderied, M. W. (2019). Deaf moths employ acoustic Müllerian mimicry against bats using wingbeat-powered tymbals. Sci. Rep. 9, 1–9. doi: 10.1038/s41598-018-37812-z
Pape, R. B. (2014). Biology and ecology of bat cave, grand canyon national park, arizona. J. Cave Karst Stud. 76, 1–13. doi: 10.4311/2012LSC0266
Peck, S. B. (1974). The invertebrate fauna of tropical american caves, part ii: puerto rico, an ecological and zoogeographic analysis the invertebrate fauna of tropical american caves, part ii: puerto rico, an ecological and zoogeographic analysis. Biotropica 6, 14–31. doi: 10.2307/2989693
Petersen, G. (1966). Über einige Tineiden aus Th ringen, gesammelt von Dr. H. Steuer. Entomol. Dresden: Nachrichten, 10.
Peck, S. B. (1975). The invertebrate fauna of tropical American caves, part III: Jamaica, an introduction. Int. J. Speleol. 7, 303–326. doi: 10.5038/1827-806X.7.4.1
Polak, S., Bedek, J., and Ozimec, R. (2012). Subterranean fauna of twelve istrian caves. Ann. Ser. Hist. Nat. 22, 7–24.
Quang, L. S., Gascuel, O., and Lartillot, N. (2008). Empirical profile mixture models for phylogenetic reconstruction. Bioinformatics 24, 2317–2323. doi: 10.1093/bioinformatics/btn445
Ratnasingham, S., and Hebert, P. D. N. (2007). Bold: the barcode of life data system (http://www.barcodinglife.org). Mol. Ecol. Notes 7, 355–364. doi: 10.1111/j.1471-8286.2006.01678.x
Regier, J. C., Mitter, C., Zwick, A., Bazinet, A. L., Cummings, M. P., Kawahara, A. Y., et al. (2013). A large-scale, higher-level, molecular phylogenetic study of the insect order lepidoptera (moths and butterflies). PLoS One 8:e58568. doi: 10.1371/journal.pone.0058568
Robinson, G. S. (1979). Clothes-moths of the Tinea pellionella complex: a revision of the world’s species (Lepidoptera: Tineidae). Bull. Br. Museum. 38, 57–128. doi: 10.5962/bhl.part.785
Robinson, G. S. (1980). Cave-dwelling tineid moths: a taxonomic review of the world species (Lepidoptera: Tineidae). Trans. Br. Cave Res. Assoc. 7, 83–120.
Roeder, K. D., and Treat, A. E. (1957). Ultrasonic reception by the tympanic organ of noctuid moths. J. Exp. Zool. 134, 127–157. doi: 10.1002/jez.1401340107
Rothschild, M., Reichstein, T., von Euw, J., Aplin, R., and Harman, R. R. M. (1970). Toxic Lepidoptera. Toxicon 8, 293–299. doi: 10.1016/0041-0101(70)90006-1
Rubin, J. J., Hamilton, C. A., McClure, C. J. W., Chadwell, B. A., Kawahara, A. Y., and Barber, J. R. (2018). The evolution of anti-bat sensory illusions in moths. Sci. Adv. 4, 1–10. doi: 10.1126/sciadv.aar7428
Shen, Z., Neil, T. R., Robert, D., Drinkwater, B. W., and Holderied, M. W. (2018). Biomechanics of a moth scale at ultrasonic frequencies. Proc. Natl. Acad. Sci. U.S.A. 115, 12200–12205. doi: 10.1073/pnas.1810025115
Silva, M. S., and Ferreira, R. L. (2015). Cave invertebrates in Espírito Santo state, Brazil: a primary analysis of endemism, threats and conservation priorities. Subterr. Biol. 16, 79–102. doi: 10.3897/subtbiol.16.5227
Skals, N., and Surlykke, A. (2000). Hearing and evasive behaviour in the greater wax moth, Galleria mellonella (Pyralidae). Physiol. Entomol. 25, 354–362. doi: 10.1046/j.1365-3032.2000.00204.x
Slootmaekers, D. (2013). Infurcitinea ignicomella (Lepidoptera: Tineidae, Meessiinae), new to the Belgian fauna. Phegea 41, 17–18.
St. Juliana, J. R., Fenton, B. M., Korine, C., Pinshow, B., Wojciechowski, M., and Kravchenko, V. (2007). Note: a field assessment of the defensive responses of moths to an auditory stimulus. Isr. J. Ecol. Evol. 53, 173–177. doi: 10.1560/IJEE.53.2.173
Surlykke, A., and Miller, L. A. (1985). The influence of arctiid moth clicks on bat echolocation; jamming or warning? J. Comp. Physiol. A 156, 831–843. doi: 10.1007/bf00610835
Takács, S., Mistal, C., and Gries, G. (2003). Communication ecology of webbing clothes moth: attractiveness and characterization of male-produced sonic aggregation signals. J. Appl. Entomol. 127, 127–133. doi: 10.1046/j.1439-0418.2003.00724.x
ter Hofstede, H. M., and Ratcliffe, J. M. (2016). Evolutionary escalation: the bat - moth arms race. J. Exp. Biol. 219, 1589–1602. doi: 10.1242/jeb.086686
Trajano, E. (2000). Cave faunas in the atlantic tropical rain forest: composition. Ecol. Conservat. Bio. 32, 882–893. doi: 10.1646/0006-3606(2000)032[0882:cfitat]2.0.co;2
Turbanov, I. S., Palatov, D. M., and Golovatch, S. I. (2016). The state of the art of biospeleology in Russia and other countries of the former Soviet Union: a review of the cave (endogean) invertebrate fauna. 3. references. Entomol. Rev. 96, 1297–1333. doi: 10.1134/S0013873816090128
Waters, D. A., Rydell, J., and Jones, G. (1995). Echolocation call design and limits on prey size: a case study using the aerial-hawking bat Nyctalus leisleri. Behav. Ecol. Sociobiol. 37, 321–328. doi: 10.1007/BF00174136
Watson, L., and Dallwitz, M. (2003). Insects of Britain and Ireland: the Genera of Grass Moths (Pyralidae- Crambidae and Schoenobiinae), Version: 12th February 2019. [WWW Document]. Available online at: delta-intkey.com (accessed May 5, 2019).
Wootton, R. J. (1979). Function, homology and terminology in insect wings. Syst. Entomol. 4, 81–93. doi: 10.1111/j.1365-3113.1979.tb00614.x
Wynne, J. J., Drost, C. A., Cobb, N. S., and Rihs, J. R. (2005). “Cave-dwelling invertebrates of grand canyon national park,” in Proceedings of the 8th Biennial Conference of Research on the Colorado Plateau, (Tucson: University of Arizona Press), 235–246.
Wynne, J. J., and Pleytez, W. (2005). Sensitive ecological areas and species inventory of Actun Chapat Cave, Vaca Plateau, Belize. J. Cave Karst Stud. 67, 148–157.
Xiao, Y. L., and Li, H. H. (2005). A systematic study on the genus Crypsithyris Meyrick, 1907 from China (Lepidoptera: Tineidae). Shil. Rev. Lepidopterol. 33, 17–23.
Zeller, P. C. (1852). Lepidoptera Microptera, quae J. A. Wahlberg in Caffrorum terra collegit. Kongliga Sven. Vetenskaps-Akademiens Nye Handl. 3, 1–120.
Keywords: bat-moth arms race, acoustic mimicry, micromoths, Tineidae, Oecophoridae, Depressariidae, Yponomeuta
Citation: O’Reilly LJ, Harris BJ, Agassiz DJL and Holderied MW (2021) Convergent Evolution of Wingbeat-Powered Anti-Bat Ultrasound in the Microlepidoptera. Front. Ecol. Evol. 9:648223. doi: 10.3389/fevo.2021.648223
Received: 31 December 2020; Accepted: 06 April 2021;
Published: 03 May 2021.
Edited by:
Fernando Montealegre-Z, University of Lincoln, United KingdomReviewed by:
William Conner, Wake Forest University, United StatesJames A. Simmons, Brown University, United States
Copyright © 2021 O’Reilly, Harris, Agassiz and Holderied. This is an open-access article distributed under the terms of the Creative Commons Attribution License (CC BY). The use, distribution or reproduction in other forums is permitted, provided the original author(s) and the copyright owner(s) are credited and that the original publication in this journal is cited, in accordance with accepted academic practice. No use, distribution or reproduction is permitted which does not comply with these terms.
*Correspondence: Marc Wilhelm Holderied, bWFyYy5ob2xkZXJpZWRAYnJpc3RvbC5hYy51aw==