Unraveling the World’s Longest Non-stop Migration: The Indian Ocean Crossing of the Globe Skimmer Dragonfly
- 1Centre for Animal Movement Research, Lund University, Lund, Sweden
- 2Centre for Ecology and Conservation, University of Exeter, Exeter, United Kingdom
- 3Department of Entomology, Nanjing Agricultural University, Nanjing, China
- 4Department of Zoology, Stockholm University, Stockholm, Sweden
- 5Animal Physiology and Biochemistry, Zoological Institute and Museum, University of Greifswald, Greifswald, Germany
- 6Manta Marine Pvt. Ltd., Malé, Maldives
- 7Environment and Sustainability Institute, University of Exeter, Exeter, United Kingdom
Insect migration redistributes enormous quantities of biomass, nutrients and species globally. A subset of insect migrants perform extreme long-distance journeys, requiring specialized morphological, physiological and behavioral adaptations. The migratory globe skimmer dragonfly (Pantala flavescens) is hypothesized to migrate from India across the Indian Ocean to East Africa in the autumn, with a subsequent generation thought to return to India from East Africa the following spring. Using an energetic flight model and wind trajectory analysis, we evaluate the dynamics of this proposed transoceanic migration, which is considered to be the longest regular non-stop migratory flight when accounting for body size. The energetic flight model suggests that a mixed strategy of gliding and active flapping would allow a globe skimmer to stay airborne for up to 230–286 h, assuming that the metabolic rate of gliding flight is close to that of resting. If engaged in continuous active flapping flight only, the flight time is severely reduced to ∼4 h. Relying only on self-powered flight (combining active flapping and gliding), a globe skimmer could cross the Indian Ocean, but the migration would have to occur where the ocean crossing is shortest, at an exceptionally fast gliding speed and with little headwind. Consequently, we deem this scenario unlikely and suggest that wind assistance is essential for the crossing. The wind trajectory analysis reveals intra- and inter-seasonal differences in availability of favorable tailwinds, with only 15.2% of simulated migration trajectories successfully reaching land in autumn but 40.9% in spring, taking on average 127 and 55 h respectively. Thus, there is a pronounced requirement on dragonflies to be able to select favorable winds, especially in autumn. In conclusion, a multi-generational, migratory circuit of the Indian Ocean by the globe skimmer is shown to be achievable, provided that advanced adaptations in physiological endurance, behavior and wind selection ability are present. Given that migration over the Indian Ocean would be heavily dependent on the assistance of favorable winds, occurring during a relatively narrow time window, the proposed flyway is potentially susceptible to disruption, if wind system patterns were to be affected by climatic change.
Introduction
Insect migration is a widespread natural phenomenon responsible for the distribution of thousands of tons of biomass and trillions of individuals each year (Hu et al., 2016; Florio et al., 2020). The annual and seasonal migration of insects translocates nutrients and ecological interactions, and has enormous influence on essential ecosystem functions such as pollination, herbivory and predation (Chapman et al., 2004; Krauel et al., 2015; Semmens et al., 2018; Wotton et al., 2019; Satterfield et al., 2020). These influences can occur over considerable ecological scales, as many insects are capable of long-distance migration covering thousands of kilometers and involving several generations (Zhan et al., 2011; Hallworth et al., 2018; Talavera et al., 2018; Gao et al., 2020a).
Migration in the insect order Odonata (dragonflies and damselflies) is relatively poorly studied (May, 2013), in spite of these insects’ conspicuousness and popularity (May, 2019). However, technological developments and an increasing interest in the use of stable isotope analysis has recently resulted in several noteworthy studies into the migratory dynamics of dragonflies (Hallworth et al., 2018; Knight et al., 2019; Borisov et al., 2020a; Hobson et al., 2021). Interestingly, potentially the longest non-stop migration of any dragonfly, indeed of any terrestrial animal when controlling for body length or mass (see Supplementary Table 1), is the proposed multi-generational, transoceanic migration of the globe skimmer dragonfly (Pantala flavescens), which has been postulated to migrate from India to Africa and back again (Anderson, 2009; Hobson et al., 2012).
At first consideration, a flight of > 2,000 km across the open ocean by an insect just 5 cm long and weighing just 300 mg may appear unlikely. Yet, there are numerous reports of the globally distributed globe skimmer being encountered at sea, and it has reached many isolated islands (see below), indicating that this species is not deterred from crossing large bodies of water, unlike other dragonflies (Wikelski et al., 2006). Monitoring of nocturnal insect migration over the Bohai Sea in China has recorded the species extensively (Feng et al., 2006), demonstrating its capacity for flying at least 150–400 km over the sea during a single night. Sightings hundreds of kilometers offshore were also noted by seafarers during the nineteenth and early twentieth centuries (Asahina and Turuoka, 1968; McLachlan, 1896; Davidson, 2012), and more recently, entered into online observation platforms by sailors (iNaturalist, 2018). The circum-tropical distribution of the globe skimmer, with individuals found on remote islands in the Pacific and Indian Oceans (Gibson-Hill, 1950; Samways, 1998; Samways and Osborn, 1998; Buden, 2010, 2018; Carr, 2020), provides further support to the idea that this species is capable of regularly carrying out long, cross-ocean flights. However, the most rigorous evidence of a re-occurring, seasonal transoceanic migration comes from regular monitoring of the globe skimmer’s annual mass appearance and departure from the Maldives, 430 km from the Indian sub-continent (Anderson, 2009). This hypothesized annual return migration between India and Africa has been frequently cited by researchers (Web of Science 80 peer-reviewed citations, as of 10th July 2021), however, the arrival of Indian globe skimmers in Africa in the autumn, as well as of African globe skimmers in India in the spring, has yet to be confirmed. Analyses investigating the eco-physiological dynamics and actual feasibility of the migration are also lacking.
Extreme endurance and long-distance migration in animals are subjects of considerable interest. Alongside the awe-inspiring journeys of long-distance migrants, such as the 8 days non-stop flight of the bar-tailed godwit Limosa lapponica (Gill et al., 2009), unraveling the complex adaptations necessary to accomplish such extreme feats of endurance is also central for our basic understanding of animal physiology, conservation and evolution, with further relevance for applied fields such as engineering and health (Hedenström, 2010; Jenni-Eiermann et al., 2014; Cooper-Mullin and McWilliams, 2016; Cheeseman et al., 2018). Bird migrants have been studied extensively, and individual-level studies in particular have revealed migratory adaptations, but until certain types of trackers are small and light enough to be carried by animals of < 1 g, migration in insects generally must be inferred remotely, via tools such as radar, modeling and stable isotopes.
One remote sensing tool that has been instrumental in the study of insect migration, is wind trajectory analysis. Wind patterns have pronounced effects on the movement of all aerial migrants (Liechti and Bruderer, 1998; Åkesson and Hedenström, 2000; Chapman et al., 2015), and similarly to birds, insects can utilize seasonally favorable winds for migration (Chapman et al., 2008, 2010). Indeed, depending on their size and migratory distance, they may rely solely on winds for their migration (Reynolds et al., 2016). Wind trajectory analysis can simulate the potential origins and destinations of insect migrations by input of regional and time specific wind data, while also allowing for species-specific parameters of migratory behavior. For example, it can be used to assess invasion risk of new pest species (Li et al., 2019; Wang et al., 2020), trace the likely departure point for specific migration events (Kim et al., 2010) and reconstruct dispersal pathways (Lander et al., 2014).
In the Indian Ocean region, the trade winds converge at an atmospheric “front” known as the Inter-Tropical Convergence Zone (ITCZ), which shifts seasonally, and influences rainfall and climate in association with monsoonal circulation. In autumn, the ITCZ moves south with winds blowing from the NNE from India, and in spring the ITCZ migrates northwards, shifting wind direction to India from the SSW (Barry and Chorley, 1992). Globe skimmers, as well as avian migrants, are believed to utilize these seasonal shifts in wind direction for their migration over the Indian Ocean (Moreau, 1938; Skerrett, 2008; Anderson, 2009).
Alongside adaptations pertaining to wind usage, insects performing long-distance migration may require specific adaptations in physiology and behavior, such as the ability to accumulate fat for energy storage (Blem, 1980; Hedenström, 2010), performing energy efficient flight (Gibo, 1981) and accurate navigational skills (Mouritsen and Frost, 2002; Dreyer et al., 2018). Dragonfly physiology has been studied extensively in the past (Kallapur and George, 1973; May, 1979, 1984, 1995), and considering the extreme energetic challenges facing a < 1 g migrant aiming to cross an ocean, we may use this knowledge to test how known physiological parameters can assist in the understanding of the feats and limitations of globe skimmer migration.
Here, we investigate the proposed multi-generational, long-distance transoceanic migration of the globe skimmer using energetic flight modeling and wind trajectory analysis. Energetic flight models apply estimates of metabolic rate, physiology and behavioral ecology to calculate theoretical flight durations and distances, while wind trajectory analyses simulate the possibility of wind-assisted migratory passage at different heights, departure sites and seasons. Specifically, we will address the following questions: (i) How long and how far can a globe skimmer stay airborne if just relying on accumulated body fat? (ii) Do favorable winds occur over the Indian Ocean that may be utilized for transoceanic migration by the globe skimmer? (iii) Where would a globe skimmer relying on wind assistance arrive? (iv) How long would a transoceanic migration relying on wind assistance take?
Materials and Methods
Study Species
The globe skimmer or wandering glider (Pantala flavescens, Libellulidae, Figure 1) is an obligate migrant dragonfly, believed to be specialized in utilizing seasonal wind systems to move between habitats where temporary rain pools are available (Corbet, 2004). It is widely distributed across all continents except Europe and the polar regions, and is usually common where it occurs (Corbet, 2004). The globe skimmer has a comparatively fast nymphal development time for a dragonfly, of approximately 40–60 days depending on water temperature (Van Damme and Dumont, 1999; Suhling et al., 2004; Ichikawa and Watanabe, 2016).
The globe skimmer is common in India, and occurs and breeds year-around in the very south (Corbet, 1988; Muthukumaravel et al., 2015). In the central part of India it peaks in abundance during the post-monsoon period (September–December), disappearing until the next heavy rains in May–July (Corbet, 1988; Sharma and Joshi, 2007; Kulkarni and Subramanian, 2013). The species is not resident in the Maldives, but starts to occur during migratory passage in October, peaking in November–December, with numbers trailing off in January–February, and with a small re-occurrence around May (Anderson, 2009; Hobson et al., 2012).
Globe skimmers have a mean body length of 50 cm and an approximate wingspan of 80 mm (Su et al., 2020). Individuals of the species typically weigh ∼300 mg (May, 1979, 1981; Ishizawa, 2007; Su et al., 2020), but mass ranges between 228 mg in small males and 553 mg in large females (Ishizawa, 2007). The anatomy of the globe skimmer indicates its adaptation for long, migratory flights. The surface of dragonfly wings is corrugated, an adaptation shown to counteract turbulence and facilitate lift (Obata and Shinihara, 2009) and the wings of globe skimmers in particular, present specialized anti-fatigue properties that are beneficial when alternating load during flight (Li et al., 2014). The globe skimmer has an expanded anal margin and broad hindwings with lobes, both features characteristic of species adapted to migration and gliding flight (Hankin, 1921; Corbet, 1962; Miller, 1962; Grabow and Rüppell, 1995; Johansson et al., 2009). The globe skimmer also has specialized thoracic musculature, which is assumed to improve long-distance flight (Bäumler et al., 2018).
Physiology of Long-Distance Migration
To assess the physiological potential and constraints of globe skimmer migration, parameters of the species ecology, behavior and physiology were obtained from the literature. Specifically, empirical data was sought on (i) species-specific flight speeds at different flight modes, in order to estimate distance that could be traveled; (ii) metabolism and energy storage capacity, in order to estimate energy expenditure at different flight modes and the distance that could be traveled on certain amounts of accumulated fat; and (iii) environmental and behavioral factors affecting these features, i.e., to identify species tendency to use or alternate between different flight modes and whether certain conditions, e.g., temperature, would influence flying capacity or flight mode. The information gathered was then entered into an energetic flight model (see below).
Flight Speed
Dragonfly flight is aerodynamically advanced, and great maneuverability and different flight modes can be achieved by the precise angling and independent motion of the fore- and hindwings (Salami et al., 2019; Liu et al., 2021). When migrating, globe skimmers have been observed to travel at velocities of 3.1–7 m/s depending on tailwind (Srygley, 2003; Feng et al., 2006). Also known as the wandering glider, the globe skimmer is often observed engaging in gliding flight for long periods of time (Hankin, 1921; Scorer, 1954; Corbet, 2004), and using thermal lift for soaring (Gibo, 1981). During gliding and soaring, the globe skimmer’s wings are outstretched but immobile, suggesting that energy expenditure is very low, potentially nearing that of resting metabolism (Gibo, 1981). However, to counteract instability and rolling movements when gliding, dragonflies hold their wings in a shallow dihedral (Wakeling and Ellington, 1997), which suggests that some energy is utilized to keep the wings in position. Studying gliding speeds in Odonates, Wakeling and Ellington (1997) found that prolonged gliding speeds outside the range of 0.6–0.9 m/s should not be aerodynamically efficient, as energy expenditure would increase because of drag. However, the authors did record speeds ranging from 0.5 to 2.6 m/s, and it is known that gliding dragonflies tend to combine gliding with shorter bouts of flapping (pers. obs.; Wakeling and Ellington, 1997; Gibo, 1981; Obata et al., 2014), so as to maintain altitude and speed when little wind is available to uphold gliding.
Metabolism
When estimating energy expenditure during rest in a globe skimmer weighing 330 mg, May (1979) recorded a metabolic rate of 0.0024 W (W = joules/s). For other behaviors, such as active flying, metabolic rate is only available for other species. In the green darner (Anax junius), which weighs ∼1,000 mg, active feeding flight is achieved at 0.26–0.43 W (May, 1995). Long-range migratory flights in dragonflies should predominantly be achieved through gliding, as this flight mode uses less energy (Gibo and Pallett, 1979; Gibo, 1981; Corbet, 2004; Vargas et al., 2008). When flight mode type was scored in migrating monarch butterflies, they were observed to soar 83.5% of the time, and only to engage in powered active flight 15.3% of the time (Gibo and Pallett, 1979). A high proportion of time spent gliding was also observed in migrating dragonflies, in relation to other flight modes (May, 1995). Unfortunately, the metabolic rate of gliding flight in dragonflies is not known empirically, but when measured in birds, has been shown to be similar to baseline energy consumption (Duriez et al., 2014), or twice as high (Sapir et al., 2010).
Fueling Flight
Similar to birds, insects are known to gain body mass by fat accumulation in preparation for migration (Blem, 1980; Corbet, 1984; Weber, 2009) and experiments on globe skimmers have shown that prolonged flight decreases lipid reserves (Kallapur and George, 1973). However, actual estimation of fat content in dragonflies, measured in species actively migrating, is absent from the literature, with one exception. During autumn migration in North America, it was estimated that up to 27% of the body mass in the green darner dragonfly can consist of fat reserves (May and Matthews, 2008). This fat percentage places dragonflies in the middle of the range measured in other migrant insects (Blem, 1980; Brower et al., 2006). Importantly, all fat stored in an insect’s body will not be available as fuel during migration, as lipids are essential for other physiological functions, for example cell membrane structure and reproduction (Arrese and Soulages, 2010).
Energetic Flight Model
An energetic flight model was constructed based on the available information presented above. All simulations were run considering a standard individual of 330 mg containing 27% fat, of which 90% was available for use to power flight (with the remaining 10% reserved for other physiological functions). All energy consumption for flight was assumed to be performed through the burning of fat, at the rate of 38 kJ/g (Berg et al., 2002). Two flight modes requiring different rates of energy consumption were considered. Active flapping flight was set at a rate of 0.19 W, inferred from the regression in Figure 7 of May (1995) for similarly sized dragonflies. Gliding flight was set at a rate of 0.002904 W, which is the resting metabolic rate in a 330 mg globe skimmer (0.0024 W, May, 1979) increased by 20%. There is no reference for the metabolic rate for gliding in the literature, but as it is suggested to be near to that of resting (Gibo, 1981), but still has to account for energy required to angle the wings optimally (Wakeling and Ellington, 1997; Obata et al., 2014), we chose an increase of 20%.
Three metrics were calculated: (1) total flight duration assuming active flight, gliding flight and a combination of active and gliding flight modes (80% gliding, 20% active flight); (2) total flight distance assuming no wind assistance; and (3) total flight distance with wind assisted flight. Flight duration analysis and distance modeling were performed using MATLAB R2019a. A flowchart visualizing the input data and resulting estimations can be viewed in Supplementary Figure 1.
Wind Trajectory Analysis
To simulate possible migratory routes of globe skimmers departing on autumn and spring migration across the Indian Ocean, we developed a numerical trajectory model that considered flight behavior and self-powered flight vectors (see for example Wu et al., 2018). The trajectory calculations were driven by high spatio-temporal resolution weather conditions, simulated by the Weather Research and Forecasting (WRF) model (version 3.8)1. The WRF is developed for research on actual or idealized atmospheric conditions, and is well adapted for meso-scale numerical forecasting applications. In this study, the dimensions of our trajectory model domain were 160 × 200 grid points at a resolution of 60 km. Thirty-two vertical layers were available and the model ceiling was 100 hPa. The model was set to adhere to the following rules: (i) trajectories were enforced to follow the wind direction downwind at all times; (ii) if the trajectories experienced temperatures under 5°C they were suspended; (iii) if windspeeds < 5 m/s were experienced, the trajectories compensated with simulated self-powered flight to uphold a minimum speed of 5 m/s.
Three departure sites for the model were set for autumn, spanning the western seaboard of the Indian subcontinent and the Maldives: (1a) Gwarka lighthouse, Gujarat, northern India (22° 14′ 18.5922″, 68° 57′ 34.3902″); (1b) Vasco da Gama, Goa, western India (15° 23′ 9.7182″, 73° 50′ 38.544″); and (1c) Malé, the Maldives (4° 6′ 10.5408″, 73° 18′ 11.5086″). The Maldives was chosen as it is the only location in the region where regular migratory movements of globe skimmers have been documented and where monitoring has been performed long-term (Anderson, 2009). The remaining two sites were chosen to acquire evenly spaced departure sites at additional locations along the Indian western coastline. As wind speeds typically increase with height above ground, insect migration that does not rely solely on self-powered flight often takes place above the flight boundary layer at high altitude (Wilson, 1995; Reynolds et al., 2016), and thus the trajectories were initiated at three different heights: 500, 1,000, and 1,500 m. Trajectories were run for a simulation period of 10 days until suspended, starting once every day at 07.00 local time for all days during the period September–December 2019, generating in total 1,098 migration events.
Two departure sites were set for the model for spring: (2a) Guardafui lighthouse, Somalia (11° 45′ 3.2394″, 51° 15′ 21.2394″); and (2b) Shakani, Kenya (−1° 39′ 39.6″, 41° 33′ 29.5194″). Trajectories were again initiated at three heights (500, 1,000, and 1,500 m) and were run for 6 days until they were suspended (they were run for less time than the autumn simulations, as the eastward migration was found to take considerably less time than the westwards migration), starting once every day at 07.00 local time for all days during the period May–July in 2019, generating in total 366 migration events.
Results
Energetic Flight Model
When engaged in 100% active, flapping flight (metabolic rate of 0.19 W), the energy reserves of a standard individual globe skimmer last just 4.39 h, in stark contrast to 286.96 h if engaged only in gliding flight (metabolic rate of 0.002904 W). Considering a combination of flight modes (Gibo and Pallett, 1979), consisting of 20% active flight and 80% gliding flight, the corresponding flight duration is 230.45 h, which is equivalent to 9.6 days (Supplementary Figure 1). Importantly, these are the absolute maximum flight durations, estimated at the limit of energetic capacity, whereby a standard individual transforms 90% of its stored lipids into movement energy. This caveat must also be kept in mind for estimates of flight distance.
Flight distance was estimated for a standard individual employing self-powered flight consisting of a combination of flight modes (20% active flight and 80% gliding flight) under self-powered speeds of 1.4, 1.8, and 2.6 m/s (Figure 2 and Supplementary Figure 1). These three speeds are based on the assumption of a speed of 5 m/s for active flight (20% of the time) and three differing gliding speeds (80% of the time): 0.5, 1, or 2 m/s. Gliding speeds without wind assistance have been suggested to be aerodynamically effective only in the range of 0.6–0.9 m/s (Wakeling and Ellington, 1997), thus our highest modeled gliding speed (2 m/s) may be in excess of gliding speeds obtainable without wind assistance. Flight distance was also estimated for a standard individual assisted by differing wind speeds ranging from 1 to 15 m/s (Figure 3).
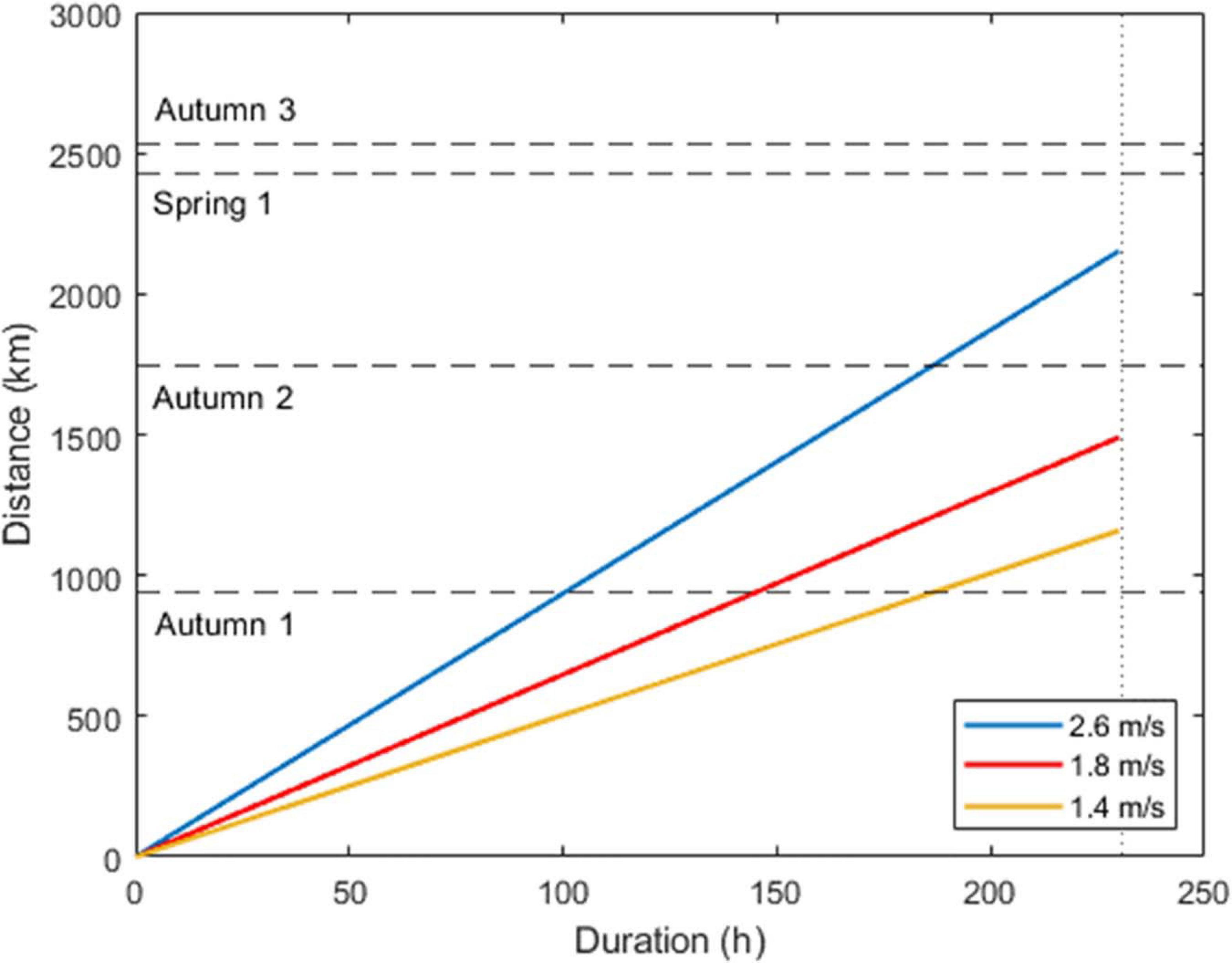
Figure 2. Estimated flight distances assuming a combination of 20% active flight and 80% gliding flight. Colored lines reflect different self-powered speeds, and the figure assumes no contribution of wind. Self-powered speeds are based on the assumption of 5 m/s for active flight (20% of the time) and three differing gliding speeds (80% of the time): 0.5, 1, and 2 m/s. The dotted vertical line denotes the estimated maximum flight duration (230.45 h). The dashed horizontal lines reflect hypothetical flight distances during autumn and spring migration, based on the shortest distance from the wind trajectory departure sites to land across the Indian Ocean (Autumn 1: 938 km; Autumn 2: 1,744 km; Autumn 3: 2,536 km; and Spring 1: 2,430 km, see Table 2).
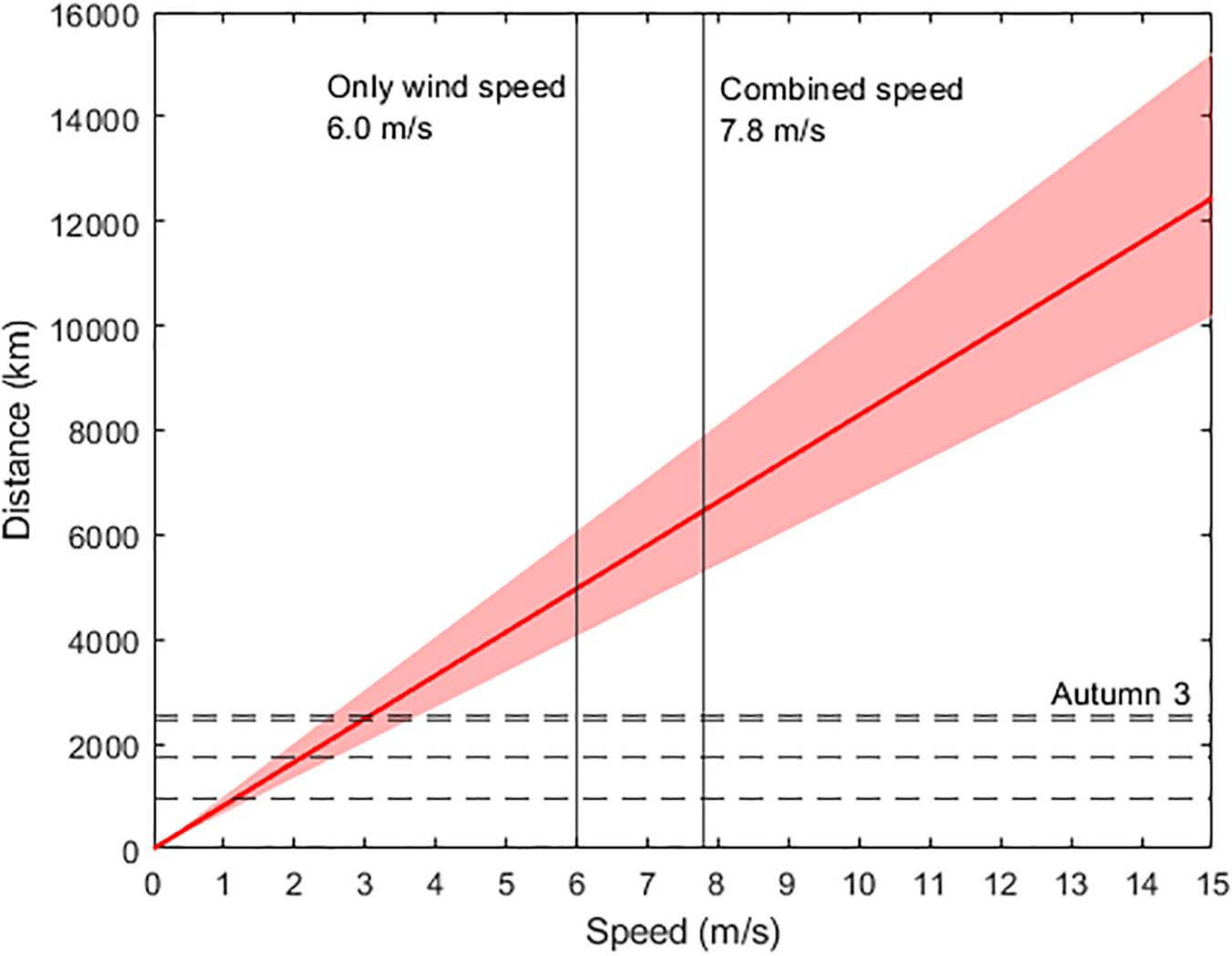
Figure 3. Estimated flight distance assuming a combination of unaided flight and wind. The red line reflects a standard individual, and the shaded area reflects simulated variation of ± 10% in input variables (energy available and metabolic rate). The horizontal dashed lines denote various flight distances during the autumn and spring migration (Autumn 1: 938 km; Autumn 2: 1,744 km; Autumn 3: 2,536 km; and Spring 1: 2,430 km, see Table 2), with the longest migration labeled (Autumn 3: 2,536 km). The vertical, solid lines denote mean wind speed during the autumn migration based on wind trajectory analyses (6 m/s), and mean wind speed during the autumn migration with the addition of the mean unaided flight speed (1.8 + 6 = 7.8 m/s).
The minimum distances a globe skimmer would have to cover to cross the Indian Ocean, from any of the departure sites used in the wind trajectory analyses (Table 1), are given the IDs Autumn 1–3 (938, 1,744, and 2,536 km) and Spring 1 (2,430 km), and used to illustrate globe skimmer migratory capacity in Figure 2 and Supplementary Figure 2. (Note here that only one departure site is considered in spring, the reason for this is further explained in the section on the results of the wind trajectory analysis). The shortest distance, Autumn 1 (938 km), is within the 230.45 h flight duration threshold for all three assumed self-powered flight speeds of a standard individual employing a combined active-gliding flight (Figure 2). However, only a speed of at least 2.6 m/s would ensure that a standard individual can cover the distance of “Autumn 2” (1,744 km) within the maximum flight duration of 230.45 h, with the two remaining distances (2,536 and 2,430 km) taking longer than this upper threshold when relying on self-powered flight alone.
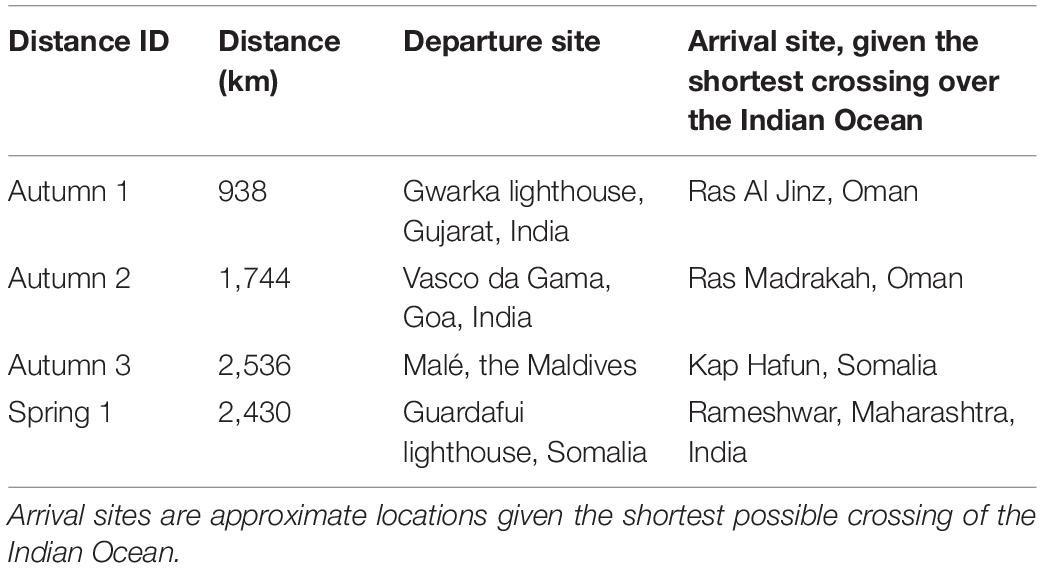
Table 1. Four minimum distances across the Indian Ocean, based on starting points corresponding to the four departure sites used in the wind trajectory analyses of potential globe skimmer autumn and spring migration routes.
We find that a windspeed of as low as 1.5 m/s is sufficient to enable a standard individual to cross the shortest proposed distance over the Indian Ocean, Autumn 1 (Figure 3), while tailwinds of at least 2.5–4 m/s are required to cross the longest distance, Autumn 3 (Figure 3). Natural conditions may not provide consistent windspeeds for many hours, or favorable wind assistance in the optimum direction. These nuances are clear from the results of our wind trajectory analyses (see below, Figures 4–9). Thus, the assistance of greater wind speeds may be necessary. In the section below on the results of the wind trajectory analysis, we report that simulated migration events in fact are assisted by winds > 4 m/s (e.g., mean wind speed across all sites and heights = 6.0 m/s in autumn and 9.7 m/s in spring).
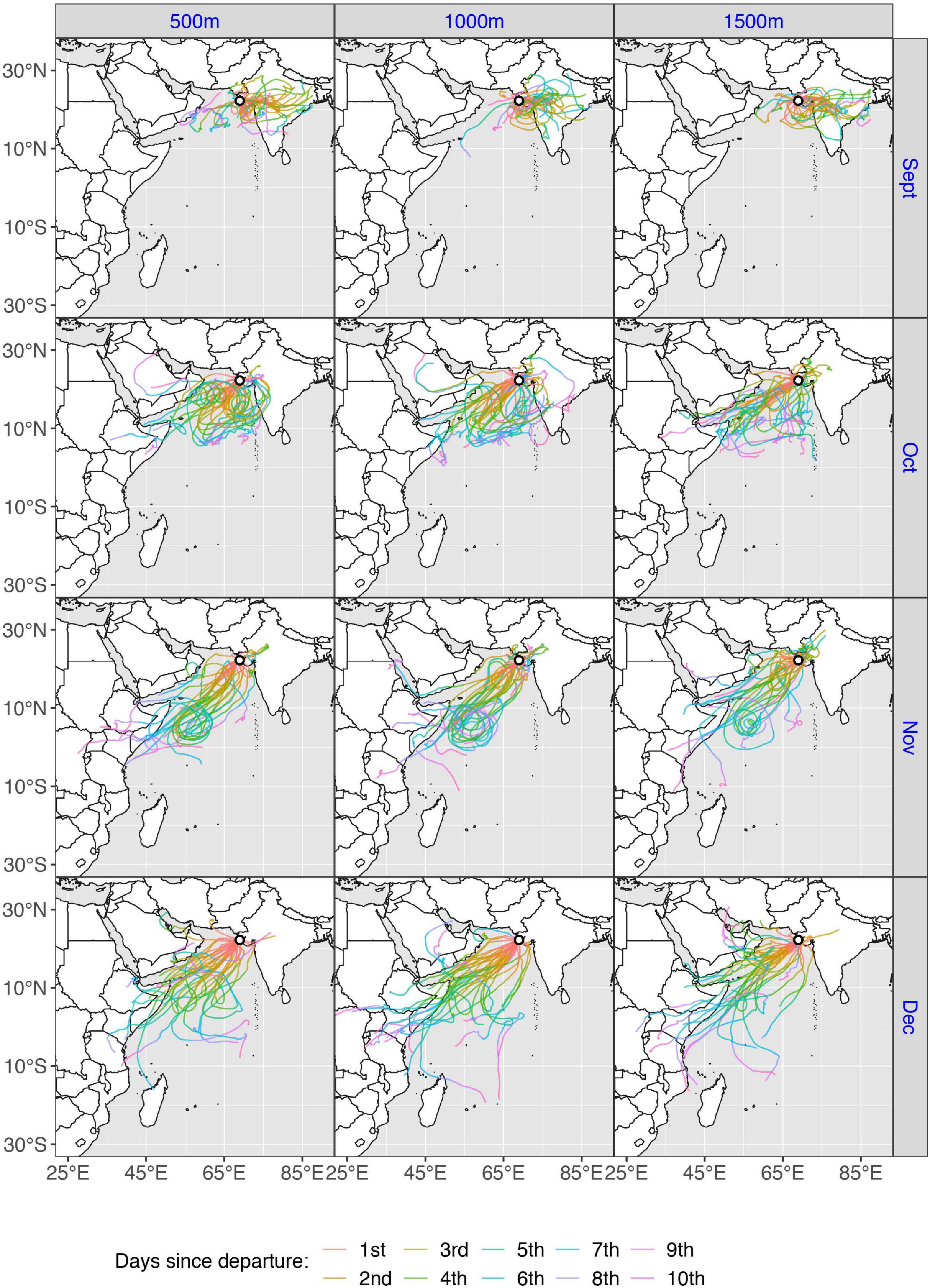
Figure 4. Autumn wind trajectories (N = 366), during September–December, for three starting heights (500, 1,000, and 1,500 m) from the departure site Gwarka lighthouse, Gujarat, northern India. Colors denote the number of days since departure (1–10). As the model adheres to a limited geographical scope, trajectories that exceeded the boundary (approximately 87°E longitude) will stop, thus trajectories traveling eastward across the Bengal Sea are cut short.
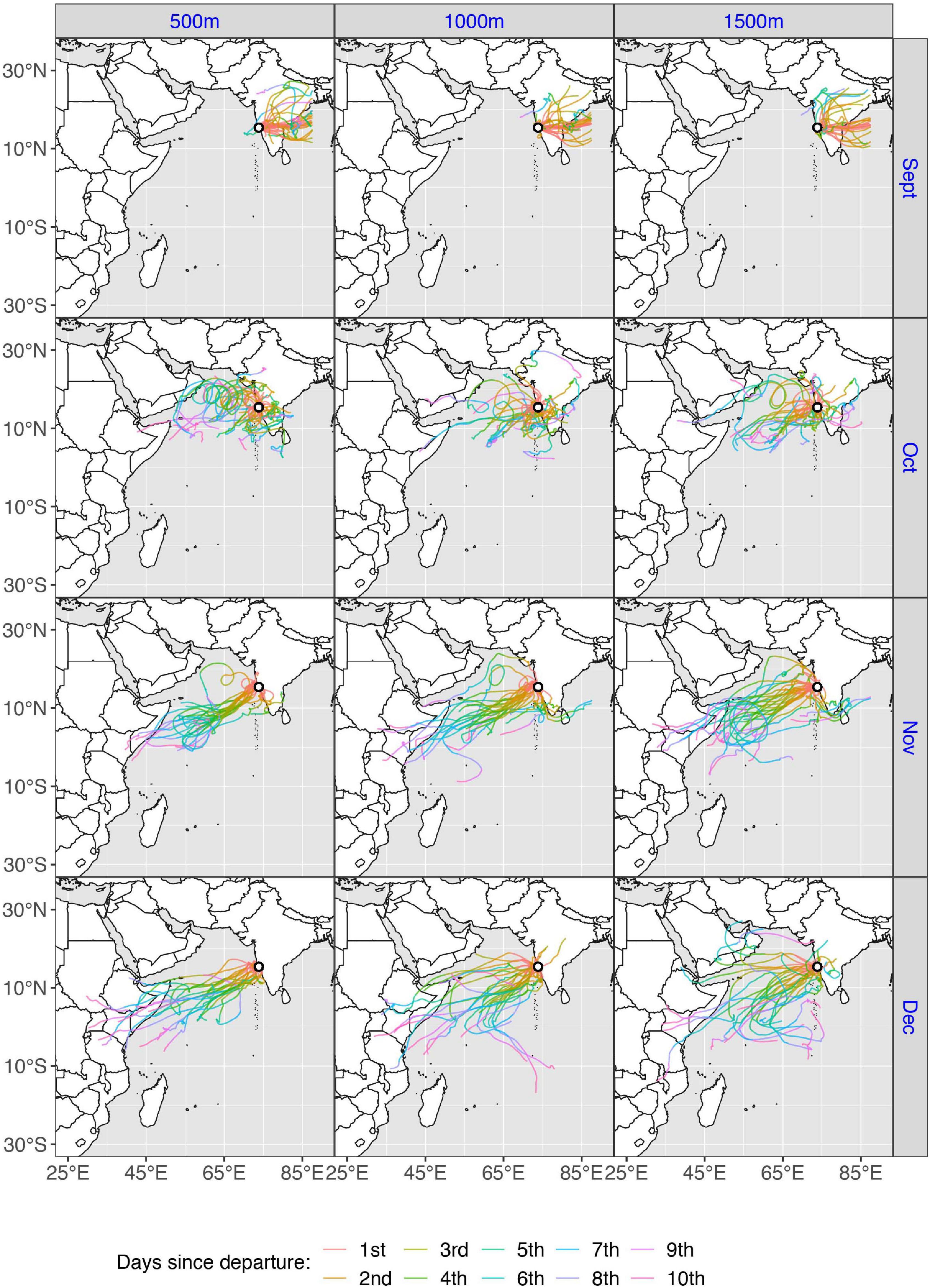
Figure 5. Autumn wind trajectories (N = 366), during September–December, for three starting heights (500, 1,000, and 1,500 m) from the departure site Vasco da Gama, Goa, western India. Colors denote the number of days since departure (1–10). As the model adheres to a limited geographical scope, trajectories that exceeded the boundary (approximately 87°E longitude) will stop, thus trajectories traveling eastward across the Bengal Sea are cut short.
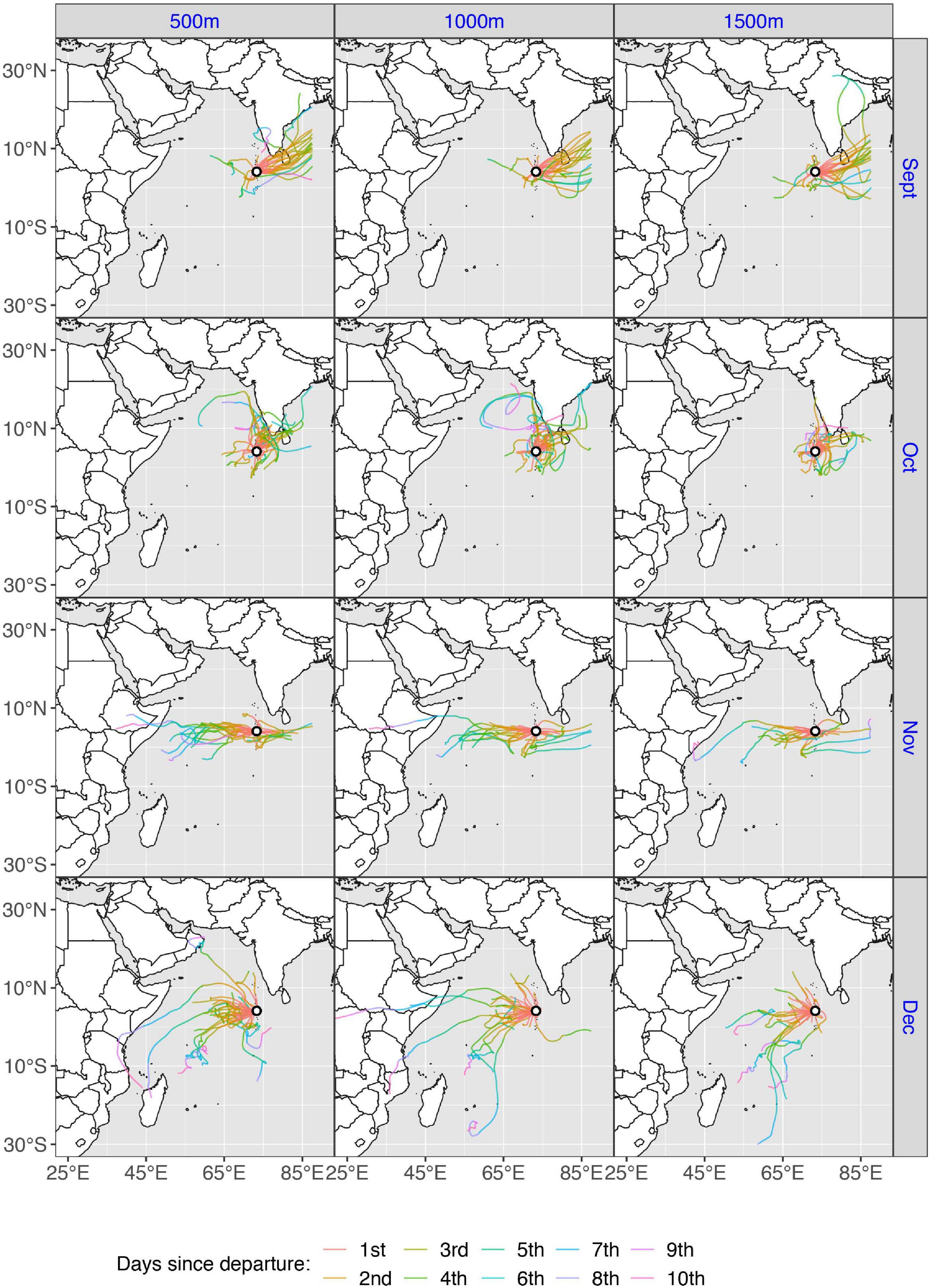
Figure 6. Autumn wind trajectories (N = 366), during September–December, for three starting heights (500, 1,000, and 1,500 m) from the departure site Malé, the Maldives. Colors denote the number of days since departure (1–10). As the model adheres to a limited geographical scope, trajectories that exceeded the boundary (approximately 87°E longitude) will stop, thus trajectories traveling eastward across the Bengal Sea are cut short.
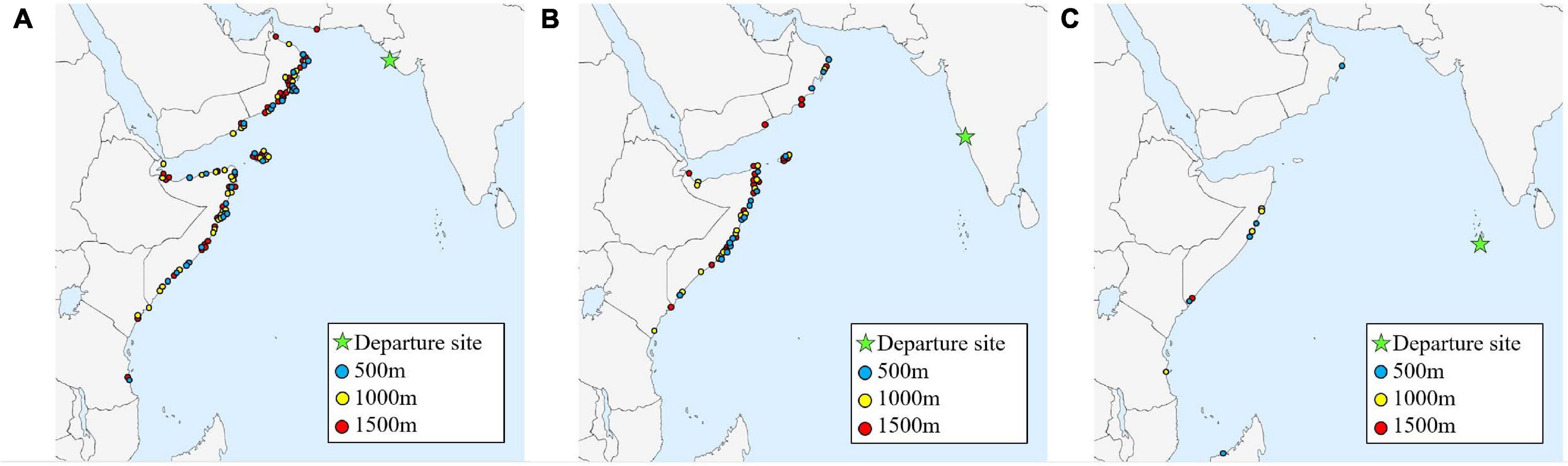
Figure 7. Arrival sites for all successful autumn trajectories (N = 167), for each starting height (500, 1,000, and 1,500 m), and three departure sites: (A) Gwarka lighthouse, northern India (N = 105); (B) Vasco da Gama, western India (N = 53); and (C) Malé, the Maldives (N = 9).
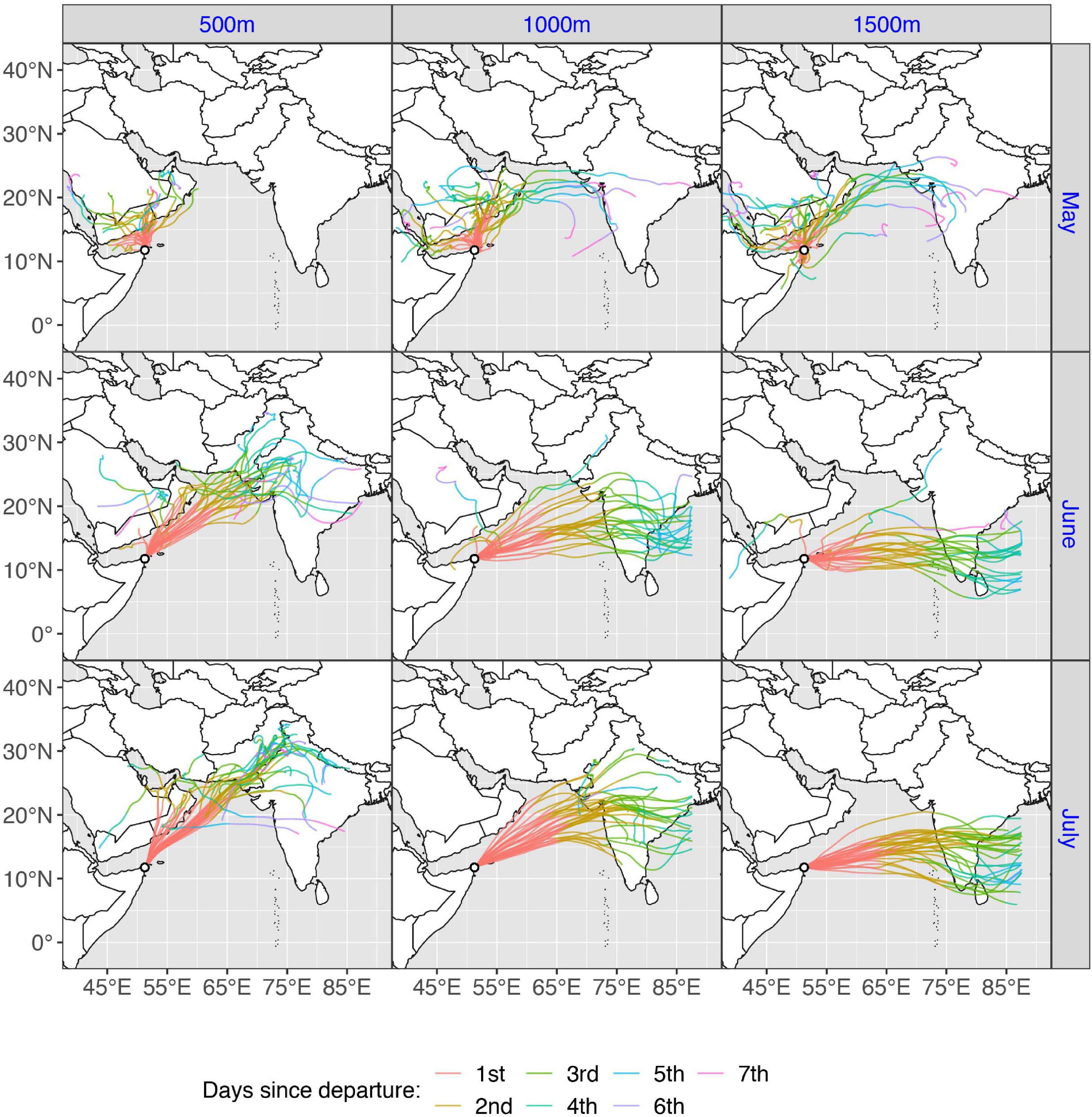
Figure 8. Spring wind trajectories (N = 366) for one departure site, Guardafui lighthouse, Somalia, during May–July, for three starting heights (500, 1,000, and 1,500 m). Colors denote the number of days (flystage) since departure (1–6). As the model adheres to a limited geographical scope, trajectories that exceeded the boundary (approximately 87°E longitude) will stop, thus trajectories traveling eastward across the Bengal Sea are cut short.
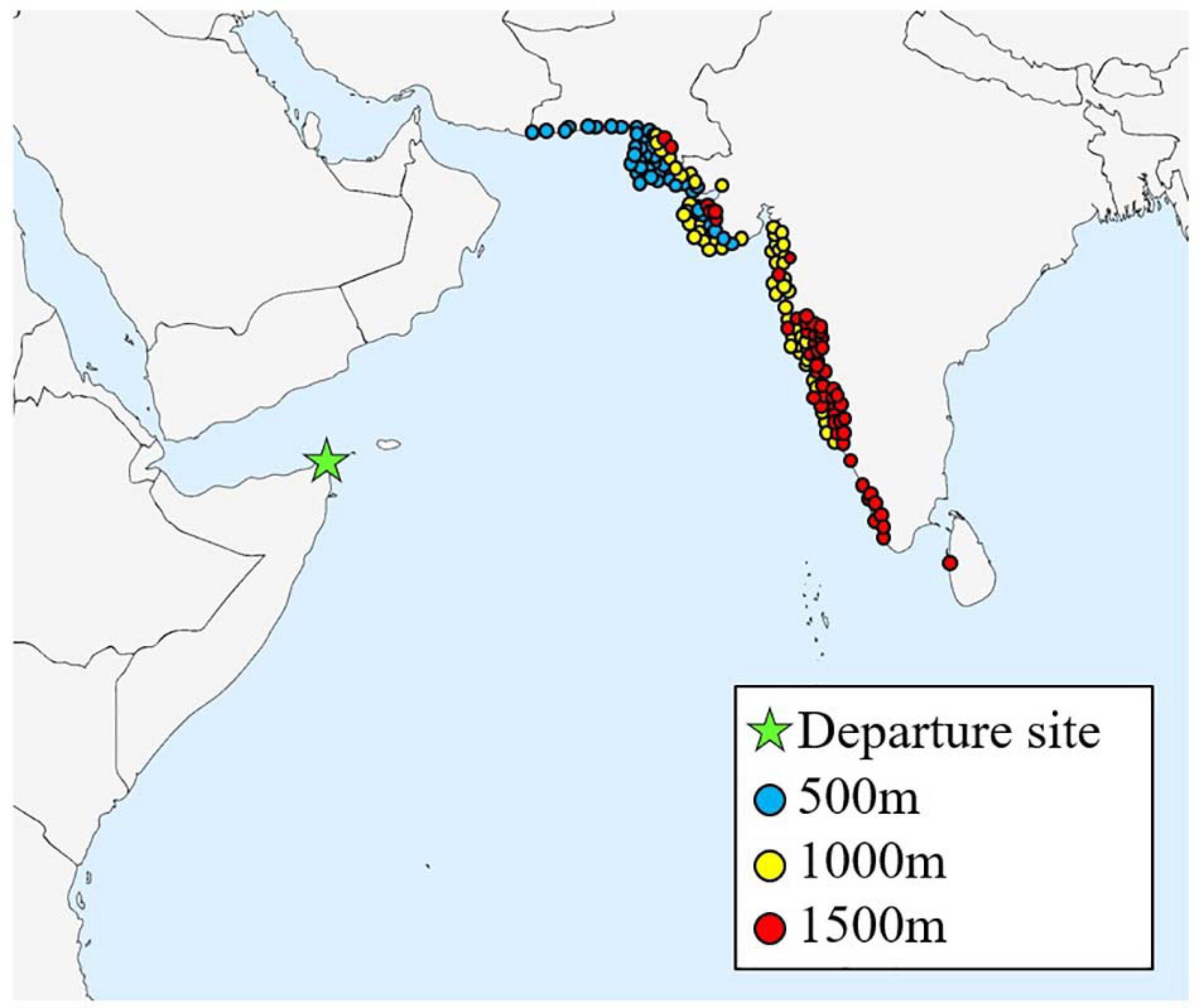
Figure 9. Map showing arrival sites for all successful spring trajectories (N = 150), for each starting height (500, 1,000, and 1,500 m), for the departure site Guardafui lighthouse, Somalia.
Wind Trajectory Analysis
A total of 1,098 trajectories were run to simulate autumn migratory flights across the Indian Ocean (Figures 3–5), equating to one trajectory for each starting height (500, 1,000, and 1,500 m), for each of the three departure sites, every day during the specified time window. An autumn trajectory was deemed successful if it reached any country in Africa, the Arabian Peninsula or Iran. A total of 180 trajectories fulfilled these requirements, but 13 were removed as they experienced temperatures under 10°C (due to high altitude), which is presumed to be too close to a temperature threshold where flight can be adequately sustained (Corbet, 1957; Rowe and Winterbourn, 1981; Dumont, 1988; Sformo and Doak, 2006). The remaining successful trajectories (N = 167, 15.2%) took on average 127.2 h ± 3.8 to cross the Indian Ocean (Figures 7A–C). Separated by departure sites, a transoceanic crossing took on average 114.2 h ± 4.5 from Gwarka lighthouse (Gujarat, northern India), 146.82 h ± 6.6 from Vasco da Gama (Goa, western India), and 164.22 h ± 10.23 from Malé, the Maldives (Table 2). Thus, flight time was in general shorter for trajectories setting out from Gwarka lighthouse, and the longest for trajectories setting out from the Maldives, which correlates with the distance required to cross the ocean to land in each case. The departure site at Gwarka lighthouse also had the greatest number of successful trajectories (N = 105, 62% of all successful), followed by the departure site in Vasco da Gama (N = 53, 31%) and lastly The Maldives (N = 9, 5%). The arrival sites of all successful trajectories in autumn are illustrated in Figures 7A–C. Most autumn wind trajectories reached land during December (N = 67), followed by November (N = 49), October (N = 34), January (N = 15), and September (N = 2). Note that arrival in January 2020 is due to the fact that trajectories starting late dates in December, running for 10 days, would finish their routes the following month. The mean wind speed across all autumn trajectories was 6.0 m/s, with a slightly higher speed measured for the departure sites of Gwarka lighthouse and Vasco da Gama (6.1 m/s), followed by The Maldives (5.9 m/s).
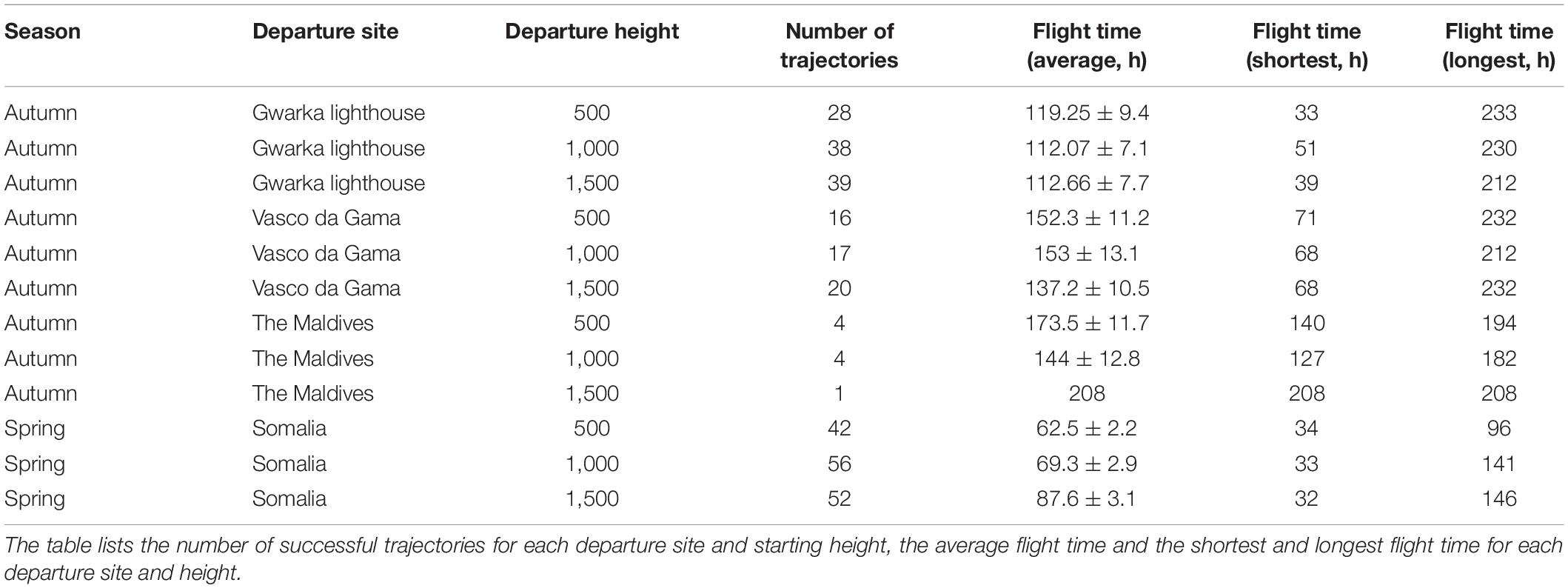
Table 2. Successful autumn and spring simulated migrations events from the wind trajectory analysis.
During spring migration, a total of 366 trajectories were run for each departure site, equating to one trajectory for each starting height (500, 1,000, and 1,500 m), every day during the specified time window (Figure 8). However, as the trajectories departing from Kenya stayed over land and did not cross the ocean until they reached Somalia, hence overlapping with the Somalian trajectories (Supplementary Figure 2), the Kenyan trajectories are not discussed further. A spring trajectory was deemed successful if it reached Pakistan, India, Sri Lanka or the Maldives. A total of 162 of 366 spring migration trajectories departing from Somalia were successful in crossing the Indian Ocean and reaching land, but of these 12 were removed as they experienced temperatures too low for flight (<10°C), leaving 150 successful trajectories (40.9%) (Figure 9). Successful spring trajectories took on average 55.24 h ± 1.6 to reach land (Table 2), a considerably shorter amount of time than required during the simulated autumn migration. Notably, spring trajectories that reached land displayed geographical segregation based on starting altitude (Figure 9), with those initiated at 500 m predominantly arriving to Pakistan and northern India, and the higher starting altitudes progressively being distributed southwards along the western Indian coastline. Most spring wind trajectories reached land during July (N = 80), followed by June (N = 56), May (N = 11), and August (N = 3). Note that arrival in August is due to the fact that trajectories starting late dates in July, running for 6 days, would finish their routes the following month. The mean wind speed across all spring trajectories departing from Somalia was 9.7 m/s, considerably faster than the mean estimated for the autumn crossing.
Discussion
Globe skimmer departure from the Asian continent toward The Maldives, and the subsequent passage across Maldives, are well-documented (Anderson, 2009; Hobson et al., 2012). However, whether this represents a regular, deliberate, transoceanic migration from India to Africa in the autumn, followed by a return migration in spring, is yet to be fully confirmed. Here, we have investigated the physiological, environmental and behavioral factors that govern the feasibility of this proposed migration. We posed four questions to frame our investigation. We applied an energetic flight model to answer the first question, which queried how long a globe skimmer could stay airborne and how far it could travel if relying solely on accumulated fat and self-powered flight (i.e., without wind assistance). The results suggest a wide range of ∼4–287 h for globe skimmer flight duration, depending on flight mode metabolics, and that a maximum distance of ∼2,250 km could be flown, given exceptionally favorable conditions, and a high self-powered gliding speed of 2.6 m/s. In summary, these measurements confirm that a self-powered migration over the Indian Ocean is possible, but only if it would take place at crossings less than 2,250 km and with exceptionally high gliding speed. These caveats suggest that wind assistance would substantially increase the likelihood of a successful ocean crossing, and is further discussed below.
The three remaining questions concerned the availability and performance of favorable winds, and we obtained answers using wind trajectory analysis. We showed, answering the second question, that favorable winds are available to the globe skimmer during both autumn and spring migration, but they vary considerably in availability over time. Thirdly, migration simulations that successfully crossed the Indian Ocean were demonstrated to arrive most often in Somalia and Oman during the autumn, and northwest India during spring. And fourth, we find that a transoceanic migration assisted by winds would take an estimated average of 127 h in autumn but only 55 h in spring. Summarizing these findings, we show that variability in the availability of favorable winds supposes a requirement for wind selectivity on the dragonfly, if it is to successfully migrate across the Indian Ocean. Furthermore the simulated migration events have several interesting implications, which will be addressed in the following sections.
According to our estimates, active flight will deplete the energy reserves of a standard globe skimmer within just 4.39 h. This energy expenditure appears rapid when compared to exhaustion trials in other flying insects (Tigreros and Davidowitz, 2019). However, this figure represents a conservative estimate of flight duration based on the upper limits of metabolic rate (0.19 W). Similarly, our assumption that gliding metabolic rate is low and near that of resting (0.002904 W) should be considered a minimum estimate. Thus, in reality, flight capacity likely lies somewhere within our upper and lower estimations. Nevertheless, with this consideration in mind we conclude that self-powered flight relying solely on a flight mode of continuous flapping flight, requiring high energy expenditure, will deplete reserves too quickly to allow the completion of a migratory journey across the Indian Ocean. Even if active flight is reduced to 20% and combined with a flight mode of low energy expenditure (i.e., gliding), this is unlikely to be sufficient to carry a globe skimmer across even the shortest distance crossing (i.e., Autumn 1), unless it experiences no headwind, and can operate at near its maximum metabolic rate constantly or at exceptionally high gliding speeds. Thus, we conclude that globe skimmer migration across the Indian Ocean would require wind assistance.
Our wind trajectory analyses clearly demonstrate that there are both intra- and inter-seasonal differences in favorable wind availability, suggesting that optimal, or even feasible, migration over the Indian Ocean requires the ability to select favorable winds. Indeed, only 15.2% of all simulated migration events in autumn were successful in reaching land, taking approximately 127 h to do so. Dragonfly migration is often reported to be associated with persistent winds, weather fronts and even storms (Corbet, 1962; Easton and Liang, 2000; Moskowitz et al., 2001; Srygley, 2003; Ries et al., 2018), and the globe skimmer is referred to as an obligate migrant of the ITCZ winds (Corbet, 2004). Indeed, there are numerous studies on insect migration suggesting that larger migrants (> 10 mg) have the ability to select winds, choosing to migrate in fast high-altitude airstreams, compensate for cross-wind drift, and select winds that will transport them in seasonally favorable directions (Chapman et al., 2008, 2010; Stefanescu et al., 2013; Wotton et al., 2019; Gao et al., 2020b). Compensating for cross-wind drift has been confirmed in the globe skimmer (Srygley, 2003), and it is likely that a species of this size, that is highly adapted for migration, would possess the ability to select winds. The ability and opportunity to select winds would be further increased if the dragonfly has physiological adaptations allowing it to remain airborne in unfavorable conditions, or when wind assistance is lacking. Consequently, investigating the mechanisms used by globe skimmers to select favorable winds for migration represents an interesting future research avenue.
According to our autumn wind trajectories in September, the month when reports of globe skimmer migration usually start in India (Fraser, 1924, 1954; Mitra, 1974, 1994), favorable winds across the Indian Ocean are almost completely absent, irrespective of departure site. These poor conditions prevail through October, at least for the two more southern departure sites, while the most favorable months to depart are November and December. In agreement with this finding, these later months coincide with the observed peak of globe skimmer passages across the Maldives (Anderson, 2009).
Within the African continent, globe skimmers appear in connection with seasonal rains and the movement of the ITCZ, in both northern and southern Africa (Corbet, 1962; Stortenbeker, 1967; Happold, 1968; Suhling and Martens, 2007; Corso et al., 2017). Unfortunately, associating the timing of the autumn wind trajectories with African observations is not possible, since there are no published observations of migrating globe skimmers arriving to, or departing from, East-African coastlines. There are, however, records from Indian Ocean islands situated east of the African coastline documenting the arrival of globe skimmers, for example in December in the Seychelles (Campion, 1913) and in October–November in the Chagos Archipelago (Carr, 2020).
The regular appearance of thousands of dragonflies on the Maldives brings in to question why so few trajectories (9 out of 366) were successful in their simulated migration from the Maldives. If our simulations were truly representative of the migratory effort, that would imply very high mortality of globe skimmers transiting the Maldives. Such prodigal waste of life, as assumed for example by Fraser (1954), may be an inevitable consequence of an obligate migratory lifestyle governed by the ITCZ (Corbet, 2004) or by migration in general (Sillett and Holmes, 2002). The globe skimmer may benefit from moving on seasonal winds for migration throughout most of its range (e.g., over mainland continents), but could face a higher likelihood of failure in certain areas (e.g., over the Pacific and Indian Ocean). In fact, stable isotope analysis of individuals arriving on the Maldives indicate that their origin is northern India (Hobson et al., 2012), and having already traveled > 2,000 km, they could be at the limit of their migratory effort.
The low success rate of trajectories starting in the Maldives may also reflect model inadequacy. Our simulations are (mainly) based on wind data from 2019, which was an atypical year, with regional oceanography and meteorology dominated by a positive phase of the Indian Ocean Dipole (Ratna et al., 2021). Furthermore, the wind trajectory model assumed migration downwind, but depending on the extent of globe skimmer wind selection ability, it could optimize wind usage further, for example by actively seeking out and exploiting rising air (Gibo, 1981) thus enabling faster and longer journeys. For example, great frigatebirds (Fregata minor) may stay aloft for months over the Indian Ocean using thermal lift, carefully selecting favorable winds and tracking the convections of the ITCZ (Weimerskirch et al., 2016). Thus, as most dragonflies leave the Maldives, it is possible that the archipelago functions as a stop-over and refueling site, and that many transit migrants do in fact make it across the ocean.
On the Arabian Peninsula, globe skimmer migratory movements are noted annually and regularly in October and November (Feulner, 2003; Campbell, 2009; Porter, 2011), including observations of large swarms occurring along the coast of UAE, the Island of Socotra (Yemen), and Oman (Campbell, 2009; Porter, 2011; Lambret et al., 2017). Successful wind trajectories departing from Gwarka lighthouse, northern India, did reach land in this region, resembling, in part, a proposed migration scenario suggested by Borisov et al. (2020b). But whether this confirms that Indian globe skimmer migrants do cross the Indian Ocean aiming for the Arabian Peninsula remains speculative until more detailed field studies are carried out. Nevertheless, our results identify this as a feasible migratory route for the species, in addition to routes further south directly across the Indian Ocean or via the Maldives.
In spring, wind trajectories demonstrate a much faster transoceanic crossing than in autumn, carrying a globe skimmer across the Indian Ocean within ∼55 h during May–August. This period follows the globe skimmer’s second flight period in The Arabian Peninsula (March–April) (Waterston and Pittaway, 1991), suggesting this area as a possible origin of migrants departing for India. The monsoonal rains return to India in May, making the area suitable for globe skimmer reproduction again (Corbet, 1988). Reappearances of the globe skimmer in India following these rains are sometimes described as “sudden” (Corbet, 1988), and considering the highly favorable winds available to transport migrants from eastern Africa at this time, it is possible that at least some of the individuals that suddenly appear in India are transoceanic migrants (Anderson, 2009). An alternative origin is wetter areas in Sri Lanka and within India itself, for example the states of Kerala and Tamil Nadu, where globe skimmers are on the wing year-around (Corbet, 1988; Muthukumaravel et al., 2015).
A third potential source of globe skimmers appearing in India in May–July is the vast areas of rice paddy fields in Southeast Asia. For comparison, recent research on the brown planthopper (Nilaparvata lugens) has revealed substantial exchange between populations from India, Southeast Asia and temperate East Asia (Hu et al., 2019; Gao et al., 2020a). Similar migratory routes and population exchange could take place in the globe skimmer. Indeed, the seasonal globe skimmer migrations through China, Russia, Southeast Asia and Japan, already suggest substantial population mixing throughout Asia (Gibson-Hill, 1950; Feng et al., 2006; Borisov and Malikova, 2019; Hobson et al., 2021), and the origin of globe skimmers arriving on the Maldives has been traced to NE India or beyond (Hobson et al., 2012). Mapping the migratory movements of globe skimmers within Asia, and between Asia and other continents, thus presents an excellent framework for the study of large-scale migration in a common and ecologically influential, but non-pest, insect.
Besides the globe skimmer, several bird species are known or presumed to migrate over the Indian Ocean (Gaston, 1976; British Trust For Ornithology, 2020), including the Amur falcon Falco amurensis (Dixon et al., 2011; The Amur Falcon Partnership, 2016; Meyburg et al., 2017). Comparing actual GPS-tracked routes of Amur falcons (Dixon et al., 2011; The Amur Falcon Partnership, 2016), with our autumn wind trajectories departing from Vasco da Gama (Goa), the routes correspond extremely well. Similarly, in spring, Amur falcon departure appears to hug the coastline of Somalia, with birds arriving into north India (The Amur Falcon Partnership, 2016; Meyburg et al., 2017), in clear agreement with our spring wind trajectories, especially the 500 m simulations. These similarities suggest the possibility that the Amur falcon co-migrates with the globe skimmer, selects similar, favorable winds, and potentially even utilizes migrating dragonflies as a prey resource during the crossing (Anderson, 2009).
Our results for aerial migration over the Indian Ocean reinforce the importance of seasonally favorable winds, and that a whole flyway system, involving several trophic levels, may be susceptible to disruption if wind patterns alter. Models of climatic change have indicated that the positioning of the ITCZ, and the behavior of the trade winds, can shift with pronounced temperature change within timescales as short as decades (Broccoli et al., 2006; Green et al., 2017). The implications of pronounced wind system change are currently unclear. However, there is a potential to disrupt migratory flyways and dislodge ecological exchange between and within ecosystems, emphasizing the importance of further research into the dynamics of wind-assisted migratory dynamics.
To conclude, our simulations estimate the circumstances whereby globe skimmers can successfully migrate over the Indian Ocean. In the few occasions where field observations are available, these correspond well with our wind trajectories. Thus, the open question “do dragonflies migrate over the western Indian Ocean?” (Anderson, 2009), can receive a cautious confirmation from a physiological and meteorological perspective. However, there is still several aspects of this proposed bidirectional transoceanic migration in need of verification: i.e., confirmed arrival of Indian migrants to reproductive habitats across the Indian Ocean, and the departure and arrival of a subsequent generation back to India. Expanding insect radar monitoring in Africa, as well as in India, is one suggested future research aim that would greatly increase our knowledge on the movement patterns of this and other important species. Another interesting avenue to explore would be life-cycle modeling, where benefits and costs of migration could be studied through population dynamics, as has been previously done for avian migrants. As the globe skimmer is a common and highly migratory species with a worldwide distribution, it is a promising model system for movement ecology research, and the Indian Ocean migration is just one of its many noteworthy flyways worth detailed investigation.
Data Availability Statement
The raw data supporting the conclusions of this article will be made available by the authors, without undue reservation.
Author Contributions
JH, JC, and RCA developed the concept and scope, as well as main text of this study. HL, PL, and GH developed and executed the modeling. JH interpreted the model results. JH, HL, and PL designed and produced the figures and tables. All authors contributed to the article and approved the submitted version.
Funding
HL and GH were supported by the National Natural Science Foundation of China (31822043). JH was funded by Formas, the Swedish Research Council for sustainable development.
Conflict of Interest
RCA was a director of the company Manta Marine Pvt. Ltd.
The remaining authors declare that the research was conducted in the absence of any commercial or financial relationships that could be construed as a potential conflict of interest.
Publisher’s Note
All claims expressed in this article are solely those of the authors and do not necessarily represent those of their affiliated organizations, or those of the publisher, the editors and the reviewers. Any product that may be evaluated in this article, or claim that may be made by its manufacturer, is not guaranteed or endorsed by the publisher.
Acknowledgments
We thank Dr. Alex Hayward for his valuable comments on the drafts of this manuscript and Dr. Eva Ehrnsten for her technical support in developing the energetic flight model. Several older references were obtained from the Biodiversity Heritage Library, and we are grateful for this online library making biodiversity literature openly available.
Supplementary Material
The Supplementary Material for this article can be found online at: https://www.frontiersin.org/articles/10.3389/fevo.2021.698128/full#supplementary-material
Footnotes
References
Åkesson, S., and Hedenström, A. (2000). Wind selectivity of migratory flight departures in birds. Behav. Ecol. Soc. 47, 140–144. doi: 10.1007/s002650050004
Anderson, R. C. (2009). Do dragonflies migrate across the western Indian Ocean? J. Trop. Ecol. 25, 347–358. doi: 10.1017/S0266467409006087
Arrese, E. L., and Soulages, J. L. (2010). Insect fat body: Energy, metabolism, and regulation. Ann. Rev. Entomol. 55, 207–225. doi: 10.1146/annurev-ento-112408-085356
Asahina, S., and Turuoka, Y. (1968). Records of the insects visited a weather ship located at the Ocean Weather Station “Tango” on the Pacific. II. Kontyû 36, 190–202.
Barry, R. G., and Chorley, R. J. (1992). Atmosphere, Weather and Climate, 6th Edn. Abingdon: Routledge, 392.
Bäumler, F., Gorb, S. N., and Büsse, S. (2018). Comparative morphology of the thorax musculature of adult Anisoptera (Insecta: Odonata): Functional aspects of the flight apparatus. Arthrop. Struct. Dev. 47, 430–441. doi: 10.1016/j.asd.2018.04.003
Berg, J. M., Tymoczko, J. L., and Stryer, L. (2002). Biochemistry, 5th Edn. New York, NY: Freeman and Company.
Blem, C. (1980). “The energetics of migration,” in Animal Migration, Orientation and Navigation, ed. S. Gauthreaux (Cambridge, MA: Academic Press).
Borisov, S. N., Iakovlev, I. K., Borisov, A. S., Ganin, M. Y., and Tiunov, A. V. (2020a). Seasonal migrations of Pantala flavescens (Odonata: Libellulidae) in middle asia and understanding of the migration model in the afro-asian region using stable isotopes of hydrogen. Insects 11, 1–12. doi: 10.3390/insects11120890
Borisov, S. N., Iakovlev, I. K., Borisov, A. S., Zuev, A. G., and Tiunov, A. V. (2020b). Isotope evidence for latitudinal migrations of the dragonfly Sympetrum fonscolombii (Odonata: Libellulidae) in Middle Asia. Ecolog. Entomol. 45, 1445–1456. doi: 10.1111/een.12930
Borisov, S. N., and Malikova, E. I. (2019). Distribution and migration strategy of Pantala flavescens (Fabricius, 1798) (Odonata. Libellulidae) near the northern limit of the range in Transbaikalia and in the Far East of Russia. Eur. Entomol. J. 18, 155–162. doi: 10.15298/euroasentj.18.3.01
British Trust For Ornithology. (2020). International Cuckoo tracking projects. Thetford: British Trust For Ornithology
Broccoli, A. J., Dahl, K. A., and Stouffer, R. J. (2006). Response of the ITCZ to Northern Hemisphere cooling. Geophys. Res. Lett. 33:L01702. doi: 10.1029/2005GL024546
Brower, L. P., Fink, L. S., and Walford, P. (2006). Fueling the fall migration of the monarch butterfly. Integr. Compar. Biol. 46, 1123–1142. doi: 10.1093/icb/icl029
Buden, D. W. (2010). Pantala flavescens (Insecta: Odonata) rides west winds into Ngulu Atoll, Micronesia: evidence of seasonality and wind-assisted dispersal. Pacific Sci. 64, 141–143. doi: 10.2984/64.1.141
Buden, D. W. (2018). Dragonflies and Damselflies (Insecta: Odonata) of the Republic of the Marshall Islands. Pacific Sci. 72, 373–387. doi: 10.2984/72.3.8
Campbell, O. (2009). Migration of Blue-spotted Arabs Colitis phisadia and associated insects at Sila’a, Western Abu Dhabi in November 2008. Tribulus. J. Emir. Nat. Hist. Group 18:66.
Campion, H. (1913). No. XXVII. Odonata. First Percy Sladen Trust Expedition to the Indian Ocean. Transactions of the Linnean Society of London. 2nd Series. Zoology 1913:15,
Carr, P. (2020). Odonata of the Chagos Archipelago, central Indian Ocean: an update. Notul. Odonatolog. 9, 229–235. doi: 10.5281/zenodo.4268581
Chapman, J. W., Nesbit, R., Burgin, L., Reynolds, D., Smith, A., Middleton, D., et al. (2010). Flight Orientation Behaviors Promote Optimal Migration Trajectories in High- Flying Insects. Science 327, 682–685. doi: 10.1126/science.1182990
Chapman, J. W., Nilsson, C., Lim, K. S., Bäckman, J., Reynolds, D. R., Alerstam, T., et al. (2015). Detection of flow direction in high-flying insect and songbird migrants. Curr. Biol. 25, R751–R752. doi: 10.1016/j.cub.2015.07.074
Chapman, J. W., Reynolds, D. R., Mouritsen, H., Hill, J. K., Riley, J. R., Sivell, D., et al. (2008). Wind Selection and Drift Compensation Optimize Migratory Pathways in a High-Flying Moth. Curr. Biol. 18, 514–518. doi: 10.1016/j.cub.2008.02.080
Chapman, J. W., Reynolds, D. R., and Smith, A. D. (2004). Migratory and foraging movements in beneficial insects: A review of radar monitoring and tracking methods. Internat. J. Pest Manag. 50, 225–232. doi: 10.1080/09670870410001731961
Cheeseman, S., Owen, S., Truong, V. K., Meyer, D., Ng, S. H., Vongsvivut, J., et al. (2018). Pillars of Life: Is There a Relationship between Lifestyle Factors and the Surface Characteristics of Dragonfly Wings? ACS Omega 3, 6039–6046. doi: 10.1021/acsomega.8b00776
Cooper-Mullin, C., and McWilliams, S. R. (2016). The role of the antioxidant system during intense endurance exercise: Lessons from migrating birds. J. Exp. Biol. 2016:123992. doi: 10.1242/jeb.123992
Corbet, P. (1984). Orientation and reproductive condition of migrating dragonflies (Anisoptera). Odonatologica 13, 81–88.
Corbet, P. (1988). “Current topics in dragonfly biology, a discussion focusing on the seasonal ecology of Pantala flavescens in the Indian subcontinent”, in The 9th International Symposium of Odonatology at Madurai (India), 1988.
Corbet, P. (2004). Dragonflies. Behaviour and ecology of Odonata. Comstock Publishing Associates. New York, NY: Cornell University Press.
Corbet, P. (1957). The life-history of the emperor dragonfly Anax imperator Leach (Odonata: Aeshnidae). J. Anim. Ecol. 26, 1–69.
Corso, A., Janni, O., Pavesi, M., and Viganò, M. (2017). Update to the status of Pantala flavescens (Fabricius, 1798) and Trithemis kirbyi Selys, 1891 for Italy and Central Mediterranean basin (Odonata Libellulidae). Biodiv. J. 8, 33–38.
Dixon, A., Batbayar, N., and Purev-Ochir, G. (2011). Autumn migration of an Amur falcon Falco amurensis from Mongolia to the Indian Ocean tracked by satellite. Forktail 27, 86–89.
Dreyer, D., Frost, B., Mouritsen, H., Günther, A., Green, K., Whitehouse, M., et al. (2018). The Earth’s Magnetic Field and Visual Landmarks Steer Migratory Flight Behavior in the Nocturnal Australian Bogong Moth. Curr. Biol. 28, 2160–2166. doi: 10.1016/j.cub.2018.05.030
Dumont, H. J. (1988). Hemianax ephippiger (Burmeister) in the northern Algerian Sahara in winter (Anisoptera: Aeshnidae). Notul. Odonatol. 3, 17–36.
Duriez, O., Kato, A., Tromp, C., Dell’Omo, G., Vyssotski, A. L., Sarrazin, F., et al. (2014). How Cheap Is Soaring Flight in Raptors? A preliminary investigation in freely-flying vultures. PLoS One 9:e84887. doi: 10.1371/journal.pone.0084887
Easton, E. R., and Liang, G.-Q. (2000). The odonata of Macao. Souther China. Notul. Odonatolog. 5, 69–84.
Feng, H. Q., Wu, K. M., Ni, Y. X., Cheng, D. F., and Guo, Y. Y. (2006). Nocturnal migration of dragonflies over the Bohai Sea in northern China. Ecol. Entomol. 31, 511–520. doi: 10.1111/j.1365-2311.2006.00813.x
Florio, J., Verú, L. M., Dao, A., Yaro, A. S., Diallo, M., Sanogo, Z. L., et al. (2020). Diversity, dynamics, direction, and magnitude of high-altitude migrating insects in the Sahel. Sci. Rep. 10, 1–14. doi: 10.1038/s41598-020-77196-7
Fraser, F. C. (1924). A survey of the odonate (dragonfly) fauna of western India with special remarks on the genera Macromia and Idionyx and descriptions of thirty new species. Rec. Indian Museum 26, 423–522.
Fraser, F. C. (1954). The origin and relationship of the odonate fauna of the Belgian Congo. Annales Du Musée Du Congo Belge, Tervuren, Miscellanea Zoologica 1, 368–370.
Gao, B., Hedlund, J., Reynolds, D. R., Zhai, B., Hu, G., and Chapman, J. W. (2020a). The ‘migratory connectivity’ concept, and its applicability to insect migrants. Move. Ecol. 8, 1–13. doi: 10.1186/s40462-020-00235-5
Gao, B., Wotton, K. R., Hawkes, W. L. S., Menz, M. H. M., Reynolds, D. R., Zhai, B. P., et al. (2020b). Adaptive strategies of high-flying migratory hoverflies in response to wind currents. Proc. Biol. Sci. 287:20200406. doi: 10.1098/rspb.2020.0406
Gaston, A. (1976). Brood Parasitism by the Pied Crested Cuckoo Clamator jacobinus. J. Anim. Ecol. 45, 331–348.
Gibo, D. L. (1981). Some Observations on Slope Soaring in Pantala flavescens (Odonata: Libellulidae). J. N. York Entomol. Soc. 89, 184–187.
Gibo, D. L., and Pallett, M. J. (1979). Soaring flight of monarch butterflies, Danaus plexippus (Lepidoptera: Danaidae), during the late summer migration in southern Ontario. Can. J. Zool. 57, 1393–1401. doi: 10.1139/z79-180
Gibson-Hill, C. (1950). Notes on the insects taken on Cocos-Keeling Islands. Bull. Raffles Museum 22, 149–165.
Gill, R. E., Tibbitts, T. L., Douglas, D. C., Handel, C. M., Mulcahy, D. M., Gottschalck, J. C., et al. (2009). Extreme endurance flights by landbirds crossing the Pacific Ocean: Ecological corridor rather than barrier? Proc. R. Soc. B: Biol. Sci. 2009:1142. doi: 10.1098/rspb.2008.1142
Grabow, K., and Rüppell, G. (1995). Wing loading in relation to size and flight characteristics of European Odonata. Odonatologica 24, 175–186.
Green, B., Marshall, J., and Donohoe, A. (2017). Twentieth century correlations between extratropical SST variability and ITCZ shifts. Geophys. Res. Lett. 44, 9039–9047. doi: 10.1002/2017GL075044
Hallworth, M. T., Marra, P. P., McFarland, K. P., Zahendra, S., and Studds, C. E. (2018). Tracking dragons: Stable isotopes reveal the annual cycle of a long-distance migratory insect. Biol. Lett. 2018:14. doi: 10.1098/rsbl.2018.0741
Happold, D. (1968). Seasonal distribution of adult dragonflies at Khartoum. Sudan. Revue de Zoologie et de Botan. Afr. 77, 50–61.
Hedenström, A. (2010). Extreme endurance migration: What is the limit to non-stop flight? PLoS Biol. 8, 2–9. doi: 10.1371/journal.pbio.1000362
Hobson, K. A., Anderson, R. C., Soto, D. X., and Wassenaar, L. I. (2012). Isotopic evidence that dragonflies (Pantala flavescens) migrating through the Maldives come from the Northern Indian subcontinent. PLoS One 7, 9–12. doi: 10.1371/journal.pone.0052594
Hobson, K. A., Jinguji, H., Ichikawa, Y., Kusack, J. W., and Anderson, R. C. (2021). Long-distance migration of the globe skimmer dragonfly to Japan revealed using stable hydrogen (δ2H) Isotopes. Env. Entomol. 50, 247–255. doi: 10.1093/ee/nvaa147
Hu, G., Lim, K. S., Horvitz, N., Clark, S. J., Reynolds, D. R., Sapir, N., et al. (2016). Mass seasonal bioflows of high-flying insect migrants. Science 354, 1584–1586. doi: 10.1126/science.aah4379
Hu, G., Lu, M. H., Reynolds, D., Wang, H. K., Chen, X., Liu, W. C., et al. (2019). Long-term seasonal forecasting of a major migrant insect pest: the brown planthopper in the Lower Yangtze River Valley. J. Pest Sci. 2019:9. doi: 10.1007/s10340-018-1022-9
Ichikawa, Y., and Watanabe, M. (2016). Daily egg production in Pantala flavescens in relation to food intake (Odonata: Libellulidae). Odonatologica 45, 107–116. doi: 10.5281/zenodo.50853
iNaturalist (2018). Pantala flavescens observation by user gmucientes. Available online at: www.inaturalist.org/observations/14645949
Ishizawa, N. (2007). Morphological variations in relation to maturation in Pantala flavescens in central Japan (Fabricius) (Anisoptera: Libellulidae). Odonatologica 36, 147–157.
Jenni-Eiermann, S., Jenni, L., Smith, S., and Costantini, D. (2014). Oxidative stress in endurance flight: An unconsidered factor in bird migration. PLoS One 2014:97650. doi: 10.1371/journal.pone.0097650
Johansson, F., Söderquist, M., and Bokma, F. (2009). Insect wing shape evolution: Independent effects of migratory and mate guarding flight on dragonfly wings. Biolog. J. Linnean Soc. 97, 362–372. doi: 10.1111/j.1095-8312.2009.01211.x
Kallapur, V. L., and George, C. J. (1973). Fatty acid oxidation by the flight muscles of the dragonfly, Pantala flavescens. J. Insect Physiol. 19, 1035–1040. doi: 10.1016/0022-1910(73)90029-2
Kim, K. S., Jones, G. D., Westbrook, J. K., and Sappington, T. W. (2010). Multidisciplinary fingerprints: Forensic reconstruction of an insect reinvasion. J. R. Soc. Interface 7, 677–686. doi: 10.1098/rsif.2009.0345
Knight, S. M., Pitman, G. M., Flockhart, D. T. T., and Norris, D. R. (2019). Radio-tracking reveals how wind and temperature influence the pace of daytime insect migration. Biol. Lett. 2019:15. doi: 10.1098/rsbl.2019.0327
Krauel, J. J., Westbrook, J. K., and Mccracken, G. F. (2015). Weather-driven dynamics in a dual-migrant system: Moths and bats. J. Anim. Ecol. 84, 604–614. doi: 10.1111/1365-2656.12327
Kulkarni, A. S., and Subramanian, K. A. (2013). Habitat and seasonal distribution of Odonata (Insecta) of Mula and Mutha river basins. Maharashtra, India. J. Threat. Taxa 5, 4084–4095. doi: 10.11609/jott.o3253.4084-95
Lambret, P., Chelmick, D., De Knijf, G., Durand, E., and Judas, J. (2017). Odonata surveys 2010–2016 in the United Arab Emirates and the Sultanate of Oman, with emphasis on some regional heritage species. Odonatologica 46, 153–205. doi: 10.5281/zenodo.1040296
Lander, T. A., Klein, E. K., Oddou Muratorio, S., Candau, J. N., Gidoin, C., Chalon, A., et al. (2014). Reconstruction of a windborne insect invasion using a particle dispersal model, historical wind data, and Bayesian analysis of genetic data. Ecol. Evol. 4, 4609–4625. doi: 10.1002/ece3.1206
Li, X., Wu, M., Ma, J., Gao, B., Wu, Q., Chen, A. D., et al. (2019). Prediction of migratory routes of the invasive fall armyworm in eastern China using a trajectory analytical approach. Pest Manag. Sci. 76, 454–463. doi: 10.1002/ps.5530
Li, X. J., Zhang, Z. H., Liang, Y. H., Ren, L. Q., Jie, M., and Yang, Z. G. (2014). Antifatigue properties of dragonfly Pantala flavescens wings. Micros. Res. Tech. 77, 356–362. doi: 10.1002/jemt.22352
Liechti, F., and Bruderer, B. (1998). The Relevance of Wind for Optimal Migration Theory. J. Avian Biol. 29, 561–568. doi: 10.2307/3677176
Liu, X., Hefler, C., Shyy, W., and Qiu, H. (2021). The Importance of flapping kinematic parameters in the facilitation of the different flight modes of dragonflies. J. Bionic Eng. 18, 419–427.
May, M. L. (1984). Energetics of adult Anisoptera, with special reference to feeding and reproductive behaviour. Adv. Odonatol. 2, 95–116.
May, M. L. (1979). Energy metabolism of dragonflies (Odonata: Anisoptera) at rest and during endothermic warm-up. J. Exp. Biol. 83, 79–94.
May, M. L. (1981). Wingstroke frequency of dragonflies (Odonata: Anisoptera) in relation of temperature and body size. J. Compar. Physiol. B 144, 229–240. doi: 10.1007/BF00802761
May, M. L. (1995). Dependence of flight behavior and heat production on air temperature in the green darner dragonfly Anax junius (Odonata: Aeshnidae). J. Exp. Biol. 198, 2385–2392.
May, M. L. (2013). A critical overview of progress in studies of migration of dragonflies (Odonata: Anisoptera), with emphasis on North America. J. Insect Conserv. 17, 1–15. doi: 10.1007/s10841-012-9540-x
May, M. L. (2019). Odonata: Who they are and what they have done for us lately: Classification and ecosystem services of Dragonflies. Insects 2019:10. doi: 10.3390/insects10030062
May, M. L., and Matthews, J. (2008). “Migration in Odonata: a case study of Anax junius,” in Dragonflies and Damselflies: Model Organisms for Ecological and Evolutionary Research (1 st Editi), ed. A. Córdoba-Aguilar (Oxford: Oxford University Press).
McLachlan, R. (1896). Oceanic migration of a nearly cosmopolitan dragon-fly (Pantala flavescens, F.). Entomol. Mag. 32:254.
Meyburg, B., Howey, P., Meyburg, C., and Pretorius, R. (2017). Year-round satellite tracking of Amur Falcon (Falco amurensis) reveals the longest migration of any raptor species across the open sea. British Ornithologists. Union Ann. Conf. 2017:28.
Mitra, T. R. (1974). Another record of migratory flights of the dragonfly Pantala flavescens (Fabricius) (Odonata, Libellulidae) in Calcutta. Entomol. Rec. J. Var. 86, 53–54.
Mitra, T. R. (1994). Observations on the habits and habitats of adult dragonflies of Eastern India with special reference to the fauna of West Bengal. Rec. Zool. Survey India 1994:166.
Moreau, R. E. (1938). Bird-migration over the north-western part of the Indian Ocean, the Red Sea, and the Mediterranean. Proc. Zoolog. Soc. Lon. A 108, 1–26.
Moskowitz, D., Moskowitz, J., Moskowitz, S., and Moskowitz, H. (2001). Notes on a large dragonfly and butterfly migration in New Jersey. Northeast. Natural. 8, 483–490.
Mouritsen, H., and Frost, B. J. (2002). Virtual migration in tethered flying monarch butterflies reveals their orientation mechanisms. Proc. Natl. Acad. Sci. U S A 99, 10162–10166. doi: 10.1073/pnas.152137299
Muthukumaravel, K., Raja, R. B., Amsath, A., Prabakaran, S., and Chezhian, Y. (2015). Seasonal variation of dragonflies diversity in Muthupet Mangrove Forest. Tamil Nadu, India. Internat. J. Pure Appl. Zool. 3, 188–192.
Obata, A., and Shinihara, S. (2009). Flow visualization study of the aerodynamics of modeled dragonfly wings. AIAA J. 47, 3043–3046.
Obata, A., Shinohara, S., Akimoto, K., Suzuki, K., and Seki, M. (2014). Aerodynamic bio-mimetics of gliding dragonflies for ultra-light flying robot. Robotics 3, 163–180. doi: 10.3390/robotics3020163
Porter, R. (2011). Introducing the Dragonflies on Socotra. Tayf – the Soqotra Newsletter, 8:11, Issued by the Friends of Soqotra and Socotra Conservation Fund.
Ratna, S. B., Cherchi, A., Osborn, T. J., Joshi, M., and Uppara, U. (2021). The Extreme Positive Indian Ocean Dipole of 2019 and associated Indian summer monsoon rainfall response. Geophys. Res. Lett. 48:e2020GL091497. doi: 10.1029/2020GL091497
Reynolds, A. M., Reynolds, D. R., Sane, S. P., Hu, G., and Chapman, J. W. (2016). Orientation in high-flying migrant insects in relation to flows: Mechanisms and strategies. Philosoph. Trans. R. Soc. B: Biol. Sci. 371:392. doi: 10.1098/rstb.2015.0392
Ries, L., Neupane, N., Baum, K. A., and Zipkin, E. F. (2018). Flying through hurricane central: impacts of hurricanes on migrants with a focus on monarch butterflies. Anim. Migr. 5, 94–103. doi: 10.1515/ami-2018-0010
Rowe, R., and Winterbourn, M. (1981). Observations on the body temperature and temperature associated behaviour of three New Zealand dragonflies (Odonata). Mauri Ora 9, 15–23.
Salami, E., Ward, T., Montazer, E., and Ghazali, N. (2019). A review of aerodynamic studies on dragonfly flight. Proc. Instit. Mech. Eng. C J. Mech. Eng. Sci. 233, 6519–6537.
Samways, M. J. (1998). Establishment of resident Odonata populations on the formerly waterless Cousine Island. Seychelles: an Island Biogeography Theory (IBT) perspective. Odonatologica 27, 253–258.
Samways, M. J., and Osborn, R. (1998). Divergence in a transoceanic circumtropical dragonfly on a remote island. J. Biogeogr. 25, 935–946. doi: 10.1046/j.1365-2699.1998.00245.x
Sapir, N., Wikelski, M., McCue, M. D., Pinshow, B., and Nathan, R. (2010). Flight modes in migrating European bee-eaters: heart rate may indicate low metabolic rate during soaring and gliding. PLoS One 5:e13956. doi: 10.1371/journal.pone.0013956
Satterfield, D. A., Sillett, T. S., Chapman, J. W., Altizer, S., and Marra, P. P. (2020). Seasonal insect migrations: massive, influential, and overlooked. Front. Ecol. Env. 18:335–344. doi: 10.1002/fee.2217
Scorer, R. (1954). The nature of convection as revealed by soaring. Q. J. R. Meteorol. Soc. 80, 68–77.
Sillett, T. S., and Holmes, R. T. (2002). Variation in survivorship of a migratory songbird throughout its annual cycle. J. Anim. Ecol. 71, 296–308. doi: 10.1046/j.1365-2656.2002.00599.x
Semmens, D. J., Diffendorfer, J. E., Bagstad, K. J., Wiederholt, R., Oberhauser, K., Ries, L., et al. (2018). Quantifying ecosystem service flows at multiple scales across the range of a long-distance migratory species. Ecosyst. Serv. 2018:002. doi: 10.1016/j.ecoser.2017.12.002
Sformo, T., and Doak, P. (2006). Thermal ecology of Interior Alaska dragonflies (Odonata: Anisoptera). Funct. Ecol. 20, 114–123. doi: 10.1111/j.1365-2435.2006.01064.x
Sharma, G., and Joshi, P. C. (2007). Diversity of Odonata (Insecta) from Dholbaha Dam (Distt. Hoshiarpur) in Punjab Shivalik, India. J. Asia-Pacific Entomol. 10, 177–180. doi: 10.1016/S1226-8615(08)60350-7
Skerrett, A. (2008). The proliferation of records of Amur Falcon Falco amurensis in Seychelles since 1995. Gabar 19, 23–26.
Srygley, R. B. (2003). Wind drift compensation in migrating dragonflies Pantala (Odonata: Libellulidae). J. Insect Behav. 16, 217–232. doi: 10.1023/A:1023915802067
Stefanescu, C., Páramo, F., Åkesson, S., Alarcón, M., Ávila, A., Brereton, T., et al. (2013). Multi-generational long-distance migration of insects: studying the painted lady butterfly in the Western. Palaearctic 36, 474–486. doi: 10.1111/j.1600-0587.2012.07738.x
Stortenbeker, C. W. (1967). Observations on the Population Dynamics of the Red Locust, Nomadacris septemfasciata (Serville), in its Outbreak Areas. Leyden: State University. doi: 10.2307/3057
Su, G., Dudley, R., Pan, T., Zheng, M., Peng, L., and Li, Q. (2020). Maximum aerodynamic force production by the wandering glider dragonfly (Pantala flavescens, Libellulidae). J. Exp. Biol. 223, 1–8. doi: 10.1242/jeb.218552
Suhling, F., and Martens, A. (2007). Dragronflies and Damselflies of Namibia. Windhoek: Gamsberg Macmillan.
Suhling, F., Schenk, K., Padeffke, T., and Martens, A. (2004). A field study of larval development in a dragonfly assemblage in African desert ponds (Odonata). Hydrobiologia 528, 75–85.
Talavera, G., Bataille, C., Benyamini, D., Gascoigne-Pees, M., and Vila, R. (2018). Round-trip across the Sahara: Afrotropical Painted Lady butterflies recolonize the Mediterranean in early spring. Biol. Lett. 14:274. doi: 10.1098/rsbl.2018.0274
The Amur Falcon Partnership (2016). Satellite Tracking of Amur Falcons. Nagaland: The Amur Falcon Partnership.
Tigreros, N., and Davidowitz, G. (2019). Flight-fecundity tradeoffs in wing-monomorphic insects. Adv. Insect Physiol. 56, 1–41. doi: 10.1016/bs.aiip.2019.02.001
Van Damme, K., and Dumont, H. J. (1999). A drought-resistant larva of Pantala flavescens (Fabricius, 1798) (Odonata: Libellulidae) in the Lençois Maranhenses, ne-Brazil. Internat. J. Odonatol. 2, 69–76. doi: 10.1080/13887890.1999.9748113
Vargas, A., Mittal, R., and Dong, H. (2008). A computational study of the aerodynamic performance of a dragonfly wing section in gliding flight. Bioinspirat. Biomimet. 3:26004. doi: 10.1088/1748-3182/3/2/026004
Wakeling, J., and Ellington, P. (1997). Dragonfly flight: I. Gliding flight and steady-state aerodynamic forces. J. Exp. Biol. 200, 543–556. doi: 10.1063/1.3001877
Wang, Y. P., Wu, M. F., Lin, P. J., Wang, Y., Ai Dong, C., Yu-Ying, J., et al. (2020). Plagues of Desert Locusts: Very Low Invasion Risk to China. Insects 11, 1–8. doi: 10.3390/insects11090628
Waterston, A., and Pittaway, A. (1991). The Odonata or Dragonflies of Oman and neighbouring territories. J. Oman Stud. 10, 131–168.
Weber, J. M. (2009). The physiology of long-distance migration: Extending the limits of endurance metabolism. J. Exp. Biol. 212, 593–597. doi: 10.1242/jeb.015024
Weimerskirch, H., Bishop, C., Jeanniard-du-Dot, T., Prudor, A., and Sachs, G. (2016). Frigate birds track atmospheric conditions over months-long transoceanic flights. Science 353, 74–78. doi: 10.1126/science.aaf4374
Wikelski, M., Moskowitz, D., Adelman, J. S., Cochran, J., Wilcove, D. S., and May, M. L. (2006). Simple rules guide dragonfly migration. Biol. Lett. 2, 325–329. doi: 10.1098/rsbl.2006.0487
Wilson, K. (1995). “Insect migration in heterogeneous environments,” in Insect Migration. Tracking Resources through Space and Time, eds V. A. Drake and A. G. Gatehouse (Cambridge. MA: Cambridge University Press), 243–264. doi: 10.1017/cbo9780511470875.012
Wotton, K. R., Gao, B., Menz, M. H. M., Morris, R. K. A., Ball, S. G., Lim, K. S., et al. (2019). Mass Seasonal Migrations of Hoverflies Provide Extensive Pollination and Crop Protection Services. Curr. Biol. 29, 2167–2173. doi: 10.1016/j.cub.2019.05.036
Wu, Q. L., Hu, G., Westbrook, J. K., Sword, G. A., and Zhai, B. P. (2018). An advanced numerical trajectory model tracks a corn earworm moth migration event in Texas. Insects 9:115. doi: 10.3390/insects9030115
Keywords: insect migration, migratory physiology, wind trajectory analysis, dragonfly migration, Pantala flavescens
Citation: Hedlund JSU, Lv H, Lehmann P, Hu G, Anderson RC and Chapman JW (2021) Unraveling the World’s Longest Non-stop Migration: The Indian Ocean Crossing of the Globe Skimmer Dragonfly. Front. Ecol. Evol. 9:698128. doi: 10.3389/fevo.2021.698128
Received: 20 April 2021; Accepted: 19 July 2021;
Published: 19 August 2021.
Edited by:
Matthew Jason Noakes, Nicolaus Copernicus University in Toruń, PolandReviewed by:
Erfan Salami, University of Malaya, MalaysiaKeith Alan Hobson, Western University, Canada
Michael May, Rutgers, The State University of New Jersey, United States
Copyright © 2021 Hedlund, Lv, Lehmann, Hu, Anderson and Chapman. This is an open-access article distributed under the terms of the Creative Commons Attribution License (CC BY). The use, distribution or reproduction in other forums is permitted, provided the original author(s) and the copyright owner(s) are credited and that the original publication in this journal is cited, in accordance with accepted academic practice. No use, distribution or reproduction is permitted which does not comply with these terms.
*Correspondence: Johanna S. U. Hedlund, johanna.hedlund@biol.lu.se, j.hedlund@exeter.ac.uk
†These authors have contributed equally to this work and share first authorship