- 1Department of Biological and Environmental Science, Konnevesi Research Station, University of Jyväskylä, Jyväskylä, Finland
- 2Wildlife Ecology and Conservation, Environmental Sciences Group, Wageningen University, Wageningen, Netherlands
Prey animals can assess the risks predators present in different ways. For example, direct cues produced by predators can be used, but also signals produced by prey conspecifics that have engaged in non-lethal predator-prey interactions. These non-lethal interactions can thereby affect the physiology, behavior, and survival of prey individuals, and may affect offspring performance through maternal effects. We investigated how timing of exposure to predation-related cues during early development affects offspring behavior after weaning. Females in the laboratory were exposed during pregnancy or lactation to one of three odor treatments: (1) predator odor (PO) originating from their most common predator, the least weasel, (2) odor produced by predator-exposed conspecifics, which we call conspecific alarm cue (CAC), or (3) control odor (C). We monitored postnatal pup growth, and we quantified foraging and exploratory behaviors of 4-week-old pups following exposure of their mothers to each of the three odour treatments. Exposure to odors associated with predation risk during development affected the offspring behavior, but the timing of exposure, i.e., pre- vs. postnatally, had only a weak effect. The two non-control odors led to different behavioral changes: an attraction to CAC and an avoidance of PO. Additionally, pup growth was affected by an interaction between litter size and maternal treatment, again regardless of timing. Pups from the CAC maternal treatment grew faster in larger litters; pups from the PO maternal treatment tended to grow faster in smaller litters. Thus, in rodents, offspring growth and behavior are seemingly influenced differently by the type of predation risk perceived by their mothers.
Introduction
Lethal and non-lethal effects of predators influence the life history of prey (Sih, 1994; Ylönen and Ronkainen, 1994; Werner and Peacor, 2003; Nelson et al., 2004; Ylönen and Brown, 2007; Sheriff et al., 2009). Over the last two decades, increasing attention has been paid to non-lethal effects that relate to fear in the face of high predation risk. Overall, this research suggests that non-lethal effects of predators mediated by fear in prey can have population level effects that are similar in magnitude to the direct effects of predators killing prey (Schmitz et al., 1997; Nelson et al., 2004; Preisser et al., 2005; Pangle et al., 2007; Creel and Christianson, 2008).
Predation risk can be detected by prey in several ways: visually, acoustically, olfactorily, or tactilely. Via olfaction, prey can either detect odors produced directly by a predator (predator odor, PO) or odors produced by conspecifics that engaged in non-lethal interactions with predators (conspecific alarm cue, CAC) (Haapakoski et al., 2018). Conspecific alarm cues are also sometimes referred to as “alarm secretions” (Brand et al., 1989) or “alarm pheromones” (Breed et al., 2004; Gomes et al., 2013). Irrespective of terminology, mechanisms and consequences associated with CAC production and release are well-documented (Gomes et al., 2013), including in anthozoa (Howe and Sheikh, 1975), insects (Bowers et al., 1972; Beale et al., 2006), amphibians (Hempel et al., 2009), and mammals (Boissy et al., 1998; Gutiérrez-García et al., 2007). In several social species, CAC serve as signals to protect at-risk families, colonies, or other groups (Breed et al., 2004; Kiyokawa et al., 2004; Gomes et al., 2013). While the structure of CAC remains unresolved for most mammals, it has been identified in, for example, aphids (Bowers et al., 1972), sea anemones (Howe and Sheikh, 1975), and several insects (Heath and Landolt, 1988; Kuwahara et al., 1989). Work in laboratory rodents has led to analyses of CAC from Wistar rats (Inagaki et al., 2014) and C57BL/6J and OMP-GFP mice (Brechbühl et al., 2013).
After encountering PO or CAC, prey are expected to adjust behaviorally in ways that minimize their chances of being preyed upon. Such changes include increased vigilance (Périquet et al., 2012) and freezing (Wallace and Rosen, 2000; Sundell and Ylönen, 2004), avoidance of risky areas (Kikusui et al., 2001), and altered space use and activity peaks (Jędrzejewska and Jędrzejewski, 1990; Jędrzejewski and Jędrzejewska, 1990; Sundell et al., 2008). In addition to the foraging-related repercussions of the behavioral changes listed above (Brown, 1999), decreased foraging can also be a direct result (Ylönen, 1989; Sundell and Ylönen, 2004; Bleicher et al., 2018). Ultimately, behavioral adjustments that enhance short-term survival of predators may lead to trade-offs with future reproduction (Ylönen and Ronkainen, 1994; Fuelling and Halle, 2004; Love and Williams, 2008; Haapakoski et al., 2012; Sheriff and Love, 2013; Voznessenskaya, 2014).
Stress responses, and in particular corticosterone production, represent another mechanism through which PO or CAC can affect prey reproduction and fitness. Exposure to either type of olfactory cue of predation risk can be transmitted from mother to offspring, influencing offspring growth (Berghänel et al., 2017), development (Hayward and Wingfield, 2004), behavior (St-Cyr et al., 2017; Sievert et al., 2020), and survival (Chin et al., 2009). A mother and fetus are connected prenatally via the placenta and postnatally via lactation; both serve as transmission routes for hormones, and thus information about the environment (Sullivan et al., 2011; Sheriff et al., 2017; Kuijper and Johnstone, 2018). Since maternal care can play a role in programming the hypothalamic-pituitary-adrenal axis (HPA-axis) of offspring, the stress responsiveness of offspring may itself be altered (Liu, 1997). Mothers might also communicate information about predation risk and stress via odors they produce (Koyama et al., 2015).
While predation risk in small mammals can affect the behavior and future reproduction of offspring (Ylönen and Ronkainen, 1994; Ylönen, 2001; Bestion et al., 2014; Sheriff et al., 2015), the role of the timing of exposure to predation risk remains unclear. Prenatal and postnatal exposure, which are governed by different mechanisms, might differ in their consequences. In this study, we exposed mothers to predator odor, CAC, or a control cue during pregnancy or during lactation. We observed the growth rate of their respective offspring and, once weaned, their offspring were tested in an experimental set-up where they encountered these odors again. Exploratory behavior as well as foraging behavior was then observed. We made several specific predictions. First, we predicted that the offspring of mothers exposed prenatally to either PO and CAC would grow more slowly than those of mothers exposed postnatally or to mothers exposed to neither, due to either increased stress metabolites in utero or a reduced maternal investment as an reaction to the perceived increased predation pressure (Bian et al., 2005; Dunn et al., 2010; Coslovsky and Richner, 2011). Second, we predicted that the offspring of mothers exposed postnatally to either PO or CAC would show greater avoidance of the odor to which their mother was exposed compared to offspring exposed prenatally (Dias and Ressler, 2014). This assumes that the odors are carried into the nest in the fur of the mother and the combination of the odor and potential changes in maternal care and/or increased stress hormone levels in the milk will trigger aversive behavior. Third, we predicted that offspring would forage less in the presence of PO and CAC, regardless of their mother’s treatment (Brown, 1988; Sievert et al., 2019). Fourth, we predicted increased latencies to investigate foraging options, reduced time spent in foraging chambers, and fewer foraging chamber visits in chambers with PO or CAC compared to the control chamber (Apfelbach et al., 2015; Sievert and Laska, 2016; Parsons et al., 2018; Sievert et al., 2019). Prediction three and four assume that PO and CAC carries information about an increased risk and therefore these compartments will be largely avoided and not used for foraging (Brown, 1988).
Materials and Methods
Study Species
The bank vole (Myodes glareolus) is a small granivorous rodent that is common in forested areas in boreal and temperate regions from Europe to Siberia. It is often used as a model species that is easy to keep and breed in captivity. The bank vole is killed by various predators, including the least weasel (Mustela nivalis nivalis). Vole populations are known to cycle multi-annually in Scandinavia, and the least weasel and other specialist predators play an important role in driving these cycles (Hanski et al., 2001). In central Finland, bank vole breed from April to September. During this time, female bank voles are strictly territorial, and each male’s territory overlaps with several female territories (Bujalska, 1973). After a gestation of about 20 days, 3–6 pups are born. Pups mature after 30 days.
The predator-prey interaction between boreal voles and their specialist predators has been well studied (Korpimäki et al., 1991; Norrdahl and Korpimäki, 1995; Ylönen et al., 2019). Least weasels and bank voles share the same habitat. The weasel is able to hunt in tunnels and burrows throughout the year, due to its small size, leaving only a few areas safe for voles (Norrdahl and Korpimäki, 1995, 2000; Sundell et al., 2008). Like all mustelids, the least weasel uses strong odors for intraspecific communication (Brinck et al., 1983), giving the prey a cue to detect the predator’s presence.
Experimental Animals
The experiments were conducted at the Konnevesi Research Station in Central Finland (62°37′ N, 26°20′ E). Bank voles were trapped from the wild and brought into captivity, where they were allowed to acclimatize for 3 weeks before the experiment commenced. All individuals were ear tagged for identification (#1005-1L1, National Band and Tag Company, Newport, Kentucky, United States). In the laboratory, the voles are kept in climate- and light-controlled (16L:8D daily cycle) husbandry rooms. Voles were housed individually in 42 × 26 × 15 cm transparent cages with wire mesh lids and wood shavings and hay as bedding, and they were provided with ad libitum water and food. Males and female voles were maintained in the same room.
Weasels for the odor treatment were housed individually in 60 × 160 × 60 cm cages in an outdoor shelter. Each cage had a nest box and wood shaving and hay as bedding. During the experiment, weasels were fed only with dead bank voles.
Experimental Design
Voles were weighed and divided randomly into three treatments: control (C), CAC and predator odor (PO). These three groups were then divided into prenatal (early) and postnatal (late) treatment groups, so that the mass distribution was similar across treatments (mean ± standard deviation: C early 17.7 g ± 4 g; C late 17.4 ± 3.2 g; CAC early 17.8 g ± 4.1 g; CAC late 17.6 g ± 3.9 g; PO early 17.2 g ± 3.4 g; PO late 16.7 g ± 4.2 g). The prenatal and postnatal control groups each consisted of 40 individuals; the other four treatment groups each consisted of 60 individuals. In all groups, the sex ratio was 50:50. Voles were randomly paired within treatment groups for mating; sibling couples were avoided. To allow for reproduction, the couples were kept in the same cage for 7 days.
During the treatment phase, females were exposed to one of the three odor treatments. The control treatment consisted of clean wood shavings. The CAC treatment consisted of wood shavings used by predator-exposed male voles. Specifically, four males that were not otherwise used in the experiment were placed individually in a weasel cage every weekday (Monday to Friday) for 3 min per day. During this exposure, the weasel was unable to actually attack the vole. The bedding from the cages of the four exposed voles was collected each day after exposure. Males were used for CAC production to avoid possible confounding effects of estrus. The PO treatment consisted of weasel bedding materials, including feces.
Female voles were exposed to the short bursts of the treatments to mimic encountering the odors outside the nest, for example during foraging trips, both pre- and postnatally. Females in the prenatal group were treated from mating until parturition (i.e., for 18–25 days depending on the timing of parturition); females in the postnatal group were treated from parturition until the pups were 21 days old. All the females received the treatment following the same protocol: three times per week (Monday, Wednesday, and Friday) for 3 min per session, females were placed individually in a small cage with a separate compartment holding the treatment materials (Supplementary Figure 1). The pups remained in the home cage during the treatments. The separation from the mothers took place in all six treatment groups to exclude it as a confounding factor.
Eighteen days after initiating mating, females were checked for signs of pregnancy, and the cages were checked twice a day (in the morning and in the evening) for the presence of pups. On the seventh day after birth, pups were toe marked for individual recognition. Pups were weighed three times a week until the 21 days of age. During this same 21 day period, the mothers in the postnatal group received the treatment. In total, 113 experimental pups were produced in 29 litters across the three treatment groups. After 21 days, the pups were separated, ear tagged, and placed in individual cages.
One week after separation, pup behavior was assayed in a foraging choice arena with three arms (Supplementary Figure 2). Four hours before being tested, pups had their food removed to incentivize foraging in the novel environment. The test arena was used to measure giving up density, GUD, as an indicator of foraging effort (Brown, 1988). The test arena was Y-shaped with one central compartment and three surrounding boxes. Each of the surrounding boxes contained one of the treatment materials (control (C) bedding, CAC bedding, or predator odor (PO) bedding). Each of the three boxes also contained a GUD tray with 1 liter sand mixed with 3.85 g of millet seeds. The experiment started by releasing a pup in the central compartment. The orientation of release was consistent over the different trials, but the orientation of the treatment boxes varied to avoid spatial bias. All trials, which were recorded from a birds-eye-view with Go Pro cameras, lasted 3 h. Upon trial completion, the remaining seeds were collected and quantified and GUD was determined for each treatment box. The sand from GUD boxes was sieved to collect the remaining millet. The sand was dried and aired for at least 5 days before it was reused in the experiments in order to minimize odor contaminations. To control for variation in moisture content, the millet was dried for 12 h at 30°C and then equilibrated to ambient indoor humidity for 24 h before weighing.
Data Analyses
A single observer (KB) analyzed all videos by recording (1) the time intervals the voles spent in each GUD tray with the millet seeds, (2) the latency to enter each treatment box, (3) and the latency to enter each GUD tray. A vole was defined as being in a treatment box upon first sight of movement in the box and as being in a GUD tray when wholly in the tray, ignoring its tail. We used the latencies to each GUD tray to determine the first choice (C, CAC or PO GUD tray) of each vole, and we counted the number of visits to each treatment box.
It was not possible to record data blind because the three different treatments (C, CAC, and PO) are visually and olfactorily easily distinguishable.
Statistical analyses were conducted using R version 3.5.1 (R Core Team, 2020). We used linear mixed models (Bates et al., 2015) to assess differences among treatments in terms of giving up density (GUD), foraging speed (grams foraged per second), time spent in boxes, number of box visits, and the two latency variables (Supplementary Appendix 1 provides all models and summary tables). To analyze first choice (i.e., non-numeric: C, CAC, or PO) data, we used the multinomial function (Venables and Ripley, 2002). Offspring body mass data were log-transformed prior to fitting linear mixed models. We used Akaike information criterion (AICc) for purposes of model selection: all models < 2 AICc units away from the best model were averaged by AICc weight to determine model estimates (Barton, 2020). A Tukey HSD post hoc test was carried out for the number of offspring between the treatments. No other post hoc tests were used in the analyses. The fixed variables in the full starting model were maternal treatment, timing of the maternal treatment (i.e., prenatal or postnatal), box treatment, box entry latency, sex, and age on test day. The variables maternal treatment and box treatment were retained in all of the compared models. To account for repeated measures within individuals and for possible effects of the litter, the random effects pup-identity vs. mother-identity over pup-identity were fitted in a full starting model for each response variable and compared by AICc. The better performing random effect was then used in all the other models for a given response variable. In the case of significant interactions between timing and either maternal treatment or box treatment, data were split to further analyze the effect. The same was done with interactions between sex and any other categorical variable.
Results
Birth Mass and Growth Rate
There were no differences in litter size between maternal treatments [n = 35 (nC = 9, nCAC = 16, nPO = 10 and nearly = 14, nlate = 21), early vs. late p = 0.680, C vs. CAC p = 0.664, C vs. PO p = 0.801, Table 1 and Supplementary Table 1]. An additional Tukey HSD post hoc test did not show any significant differences either (p > 0.05 for all comparisons). The treatments did not affect birth mass, but litter size did: each additional pup in a litter decreased the individual pup birth body mass by 0.07 g (n = 141, p < 0.001, weighted average, Supplementary Table 2).
Pup body mass was influenced by a three-way interaction between age in days, litter size, and maternal treatment, while the timing of the treatments (pre- or postnatal) had no significant effect on its own nor showed significant interactions with the treatments (n = 35 litters and n = 1316 observations, day*litter size*maternal CAC p = 0.023, day*litter size*maternal PO p = 0.00048, Figure 1, Table 2, Supplementary Table 3). A subset analysis showed that pups from the maternal C treatment from larger litters started at a disadvantage compared to smaller litters from this treatment, but this was compensated for over the first 21 days of growth (log values: day = 0.08, litter size = –0.03, day*litter size = 0.001, Figure 1). In contrast, pups from the maternal CAC treatment started heavier and showed slightly faster growth in larger litters (log values: day = 0.08, litter size = 0.04, day*litter size = 0.0005, Figure 1). In the maternal PO treatment, pups from larger litters were smaller at birth and grew more slowly than pups from smaller litters (log values: day = 0.08, litter size = –0.03, day*litter size = –0.002, Figure 1).
First Choice of the Treatment Box and the Giving Up Density Tray
Pups from CAC-treated mothers were more likely to enter first into the CAC-treated box compared to the control box (54% vs. 14%; n = 113, p = 0.005, weighted average, Supplementary Table 4). Similarly, pups from CAC-treated mothers were more likely to enter first into the CAC GUD tray compared to the control GUD tray (50% vs. 22%; n = 113, p = 0.0194, weighted average, Supplementary Table 5). No other treatment showed significant preferences. Treatment timing and sex did not have a measurable effect in either case.
Visits to the Treatment Boxes
Overall, pups visited the PO treatment box less frequently than the C treatment box (ca. 7 fewer visits, n = 113, p < 0.001, weighted average, Figure 2 and Supplementary Table 6). Additionally, pups from CAC-treated mothers made fewer visits to any treatment box compared to pups from control mothers (ca. 10 fewer visits, n = 113, p = 0.002, weighted average, Figure 2 and Supplementary Table 6). The number of visits to any treatment box decreased by 0.43 per day of age (n = 113, p = 0.026, weighted average, Supplementary Table 6). Female pups made more visits to any treatment box compared to males (ca. 7.8 visits more, n = 113, p = 0.014, weighted average, Supplementary Table 6). Visit frequency was unaffected by the timing of the maternal treatments.
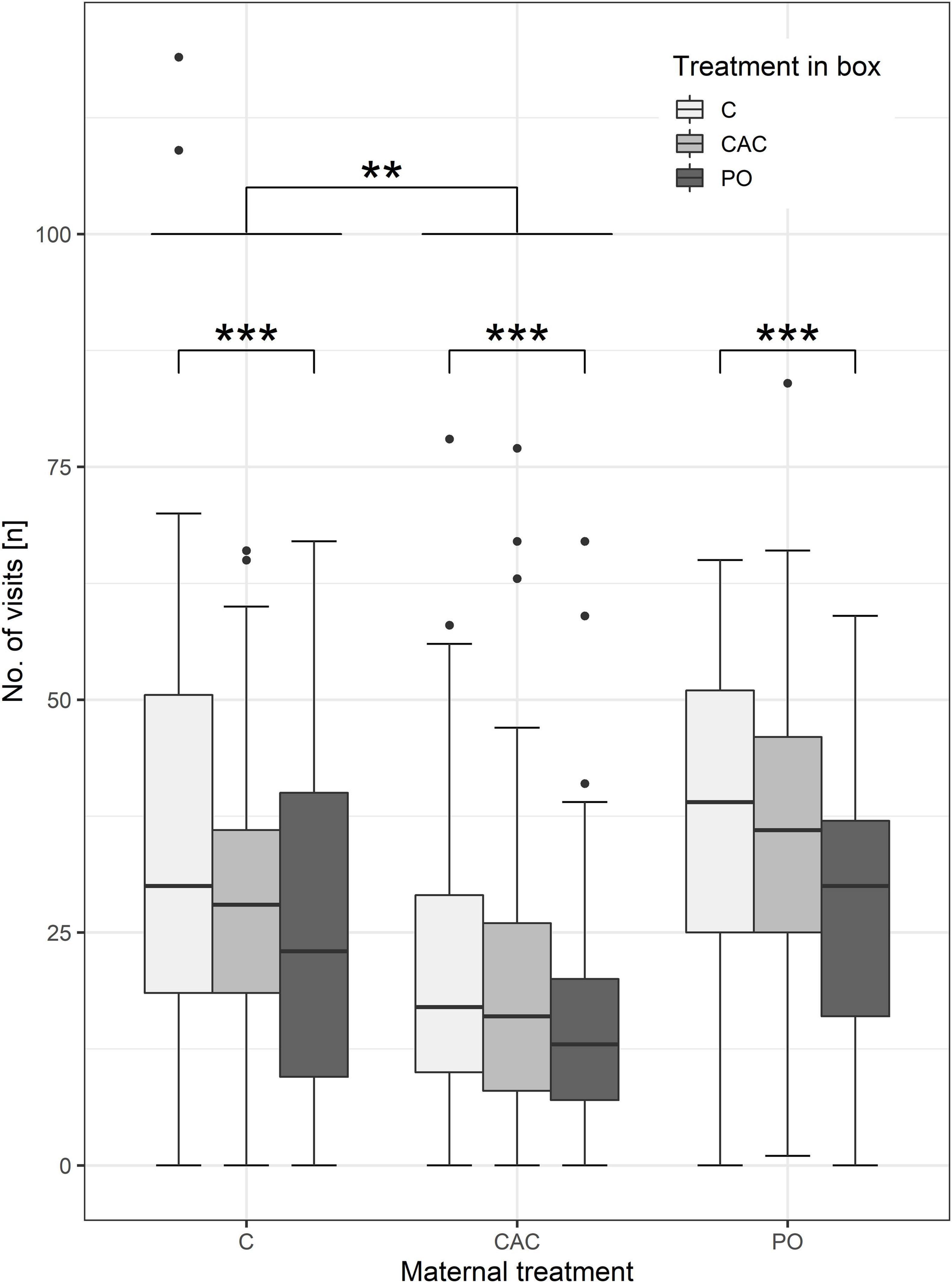
Figure 2. Number of visits in the treatment boxes, split by maternal treatments. Asterisks indicate a significant difference from C at p < 0.01 (**) and p < 0.001 (***).
Latency to Enter the Treatment Box and the Giving Up Density Tray, and the Time Spent in the Giving up Density Tray
The results for the differences in latency to enter the treatment box are inconclusive as all models produce singular fit errors. There were no significant differences in latency times between PO, CAC, or control GUD trays (Supplementary Table 7). Overall, pups spent less time in the PO GUD tray compared to the control tray (ca. 492 s less, n = 113, p < 0.001, Figure 3 and Supplementary Table 8). Pups from PO-treated mothers spent more time in any GUD tray compared to pups from control mothers (ca. 310 s, n = 113, p = 0.039, Figure 3 and Supplementary Table 8). Time spent in any GUD tray decreased by 18 s per day of age (n = 113, p = 0.004, Supplementary Table 8). Treatment timing and sex did not have statistically significant effects on time spent in GUD trays.
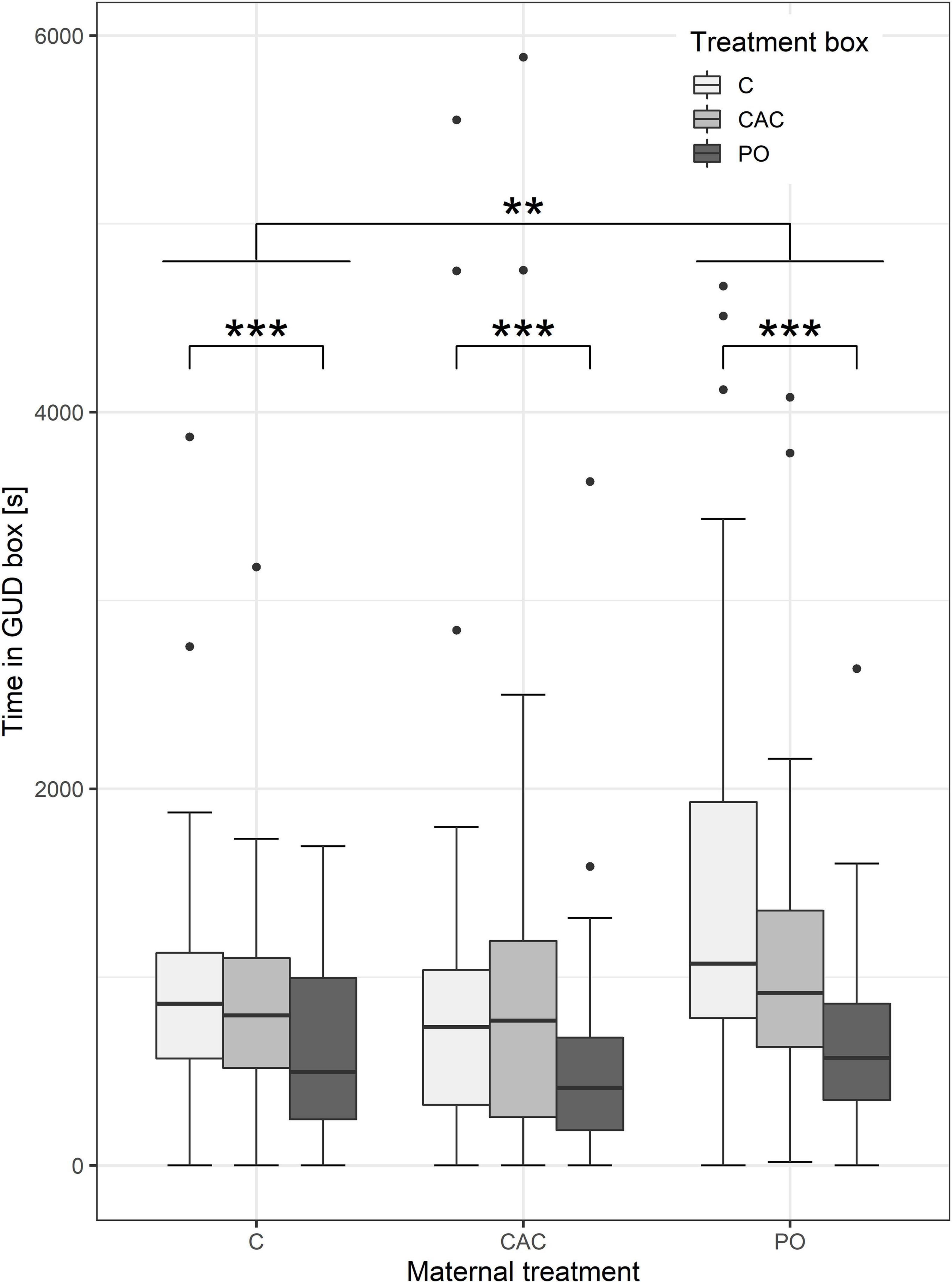
Figure 3. Time spent in the GUD trays, split by maternal treatments. Asterisks indicate a significant difference from C at p < 0.01 (**) and p < 0.001 (***).
Giving-Up-Densities and Foraging Speed
The interaction between maternal treatment and treatment timing significantly influenced GUD in the CAC and PO treatment, although not in the controls (Figure 4). Pups from prenatal control mothers left 3.65 g of millet per liter of sand. Compared to pups from mothers exposed prenatally to the same treatment, pups from mothers treated postnatally with CAC foraged 0.11 g more, and pups from mothers treated postnatally with PO foraged 0.10 g more (i.e., lower GUD following post-natal exposure, Figure 4, n = 113, p = 0.006 and p = 0.029, respectively, weighted model average, Supplementary Table 9). Among pups from postnatally-treated mothers, pups from CAC-treated mothers foraged significantly more (by ca. 0.09 g) than pups from control mothers, which left ca. 3.69 g of millet per liter of sand (n = 67, p = 0.021, weighted model average, Figure 4 and Supplementary Table 10) while no difference was observed for pups from PO-treated mothers (n = 67, p = 0.021, weighted model average, Figure 4 and Supplementary Table 10). Among pups from prenatally-treated mothers, no significant differences in GUD were observed between the maternal treatments (n = 46, p > 0.05, Supplementary Table 11). Overall, foraging box treatment did not significantly influence GUD. The foraging speed, grams per second, does not differ significantly between any treatments (n = 64, p > 0.05 for all comparisons, Supplementary Table 12).
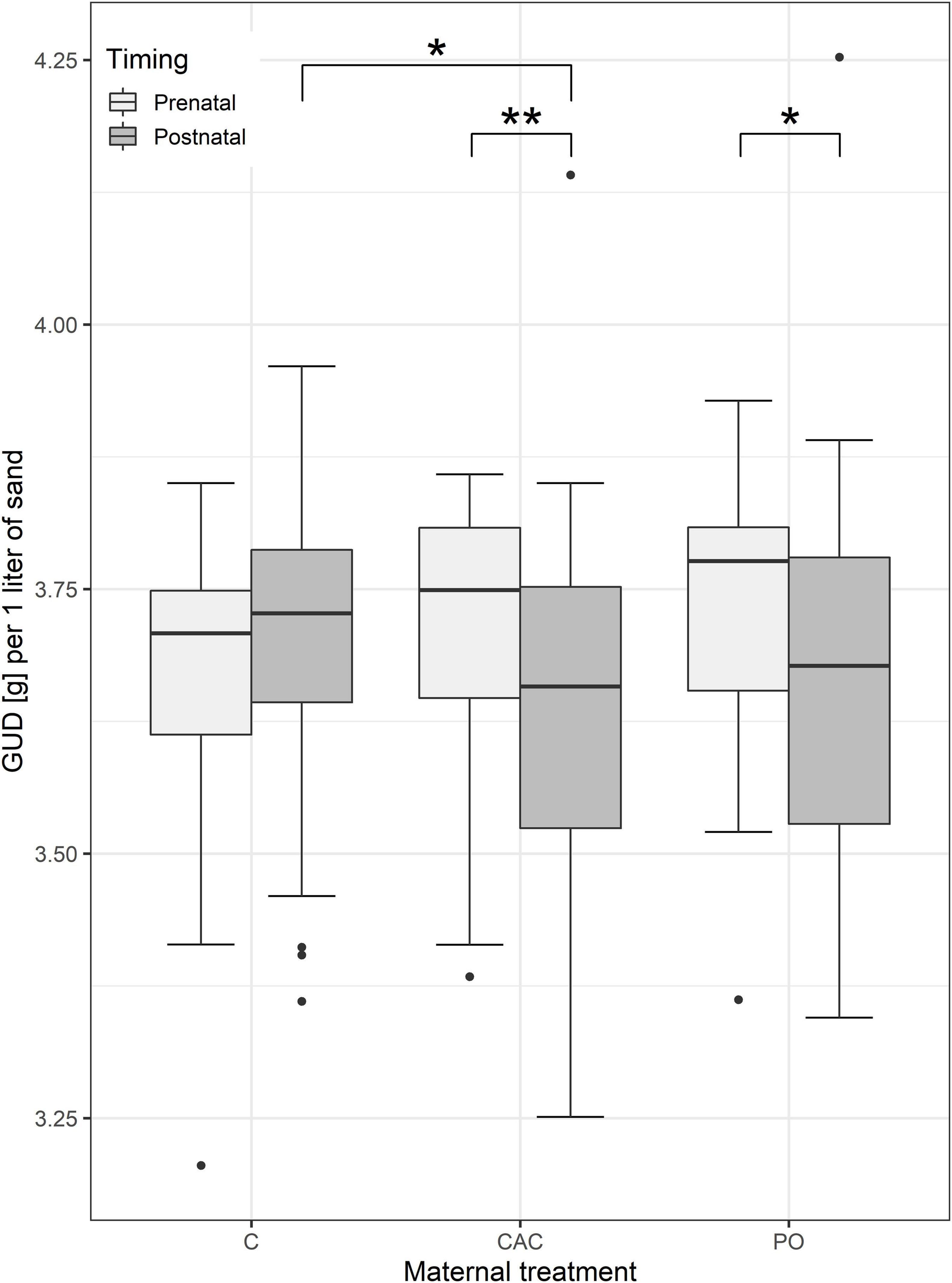
Figure 4. Boxplots showing the GUD in gram split by the different maternal treatments and treatment timing (pre- and postnatal). Asterisks indicate a significant difference at p < 0.05 (*) and p < 0.01 (**).
Discussion
The current study contributes to a growing understanding about how olfactory cues related to predation risk can have transgenerational impacts on behavior and physiology (Sievert et al., 2019, 2020). Our main focus here was to investigate to what extent pre- vs. postnatal exposure to predator odor and CAC affects offspring differently. While we found only limited evidence for such differential effects in bank voles, our study suggests that odor cue origin (i.e., from predators vs. from conspecifics) matters in ways that interact with other factors that are unrelated to exposure timing. For example, pups from predator-odor-treated mothers grew faster in smaller litters, while the opposite (faster growth in bigger litters) was found for pups from mothers treated with CAC. Additionally, we found that vole pups showed an innate avoidance of predator odor regardless of maternal exposure, but pups from mothers treated with CAC showed signs of preference for that cue. This apparent mismatch shows that both physiological and behavioral adaptations need to be considered together as only looking at one aspect might skew the interpretations.
We found limited differences between in anti-predator behavior in relation to the timing of predation odor cue exposure of mothers. In fact, only foraging was affected by treatment timing. However, the increased consumption by pups from postnatally exposed mothers compared to those from prenatally treated mothers, ca. 19 millet seeds (ca. 0.0052 g per seed), likely represents an ecologically meaningful change in the foraging behavior. Interestingly we did not see a similar pattern when it comes to the time spent in the GUD trays. There pups from PO-treated mothers spent about 310 s more time in them, compared to pups from control-treated mothers. We further found that foraging speed is independent of any of our treatments. Previous studies have explored different aspects of early life exposure to a potential stressor, highlighting possible mechanisms such as maternal care (Bauer et al., 2015) and differences in the milk composition (Brummelte et al., 2010), or the adaptive aspects of the resulting changes in offspring physiology and behavior (Patacchioli et al., 2002; Dias and Ressler, 2014). However, the timing during which the early life stressor has to occur to trigger these changes remains largely obscure.
Pups in bigger litters from mothers treated with CAC and pups in smaller litters from mothers treated with predator odor both gained mass faster compared to the control. These drastically opposite result hint that different reproductive strategies are associated with different perceived risks that are communicated by different odor cues, as previously suggested (Sievert et al., 2019). CAC exposure might trigger a form of terminal investment with a focus on larger litters; predator odor might trigger investment in a few high quality offspring. Specifically, we saw that offspring in the largest litters from the maternal CAC treatment weighted on average 14 g, while individuals from the smallest litters were only about 11 g (Figure 1). This difference was even more pronounced in the maternal predator odor treatment where now individuals from the smallest litters were about 15.5 g and pups in the biggest litters weighted only 9 g. Only male voles were used to generate the CAC, and this sex specificity may underlie the cue’s mechanism of action. For example, exposure might communicate more about the unfamiliar conspecific male (Eccard et al., 2017) than about the predator that male encountered. While this possibility cannot be ruled out in the current study, previous work in bank voles revealed different reactions to the CAC produced by predator exposed male voles and the “normal” odors produced by unexposed male voles (Haapakoski et al., 2018; Sievert et al., 2019). Furthermore, we have recently analyzed possible molecular candidates for alarm substances in the bank vole (Sievert, 2020) showing that differences in chemical odor composition are only caused by the treatments (i.e., no handling, handling, and weasel exposure) and show no significant differences based on the sex of the individual.
A strong tendency to avoid boxes treated with predator odor compared to boxes treated with the CAC was found for pups from both maternal treatments (i.e., PO and CAC) at both time points (i.e., pre- and postnatally). This avoidance took the form of fewer visits to and less time spent in boxes treated with predator odor. Because this effect was independent of the maternal treatments, voles seemed to innately avoid areas that were possibly dangerous. Other species exhibit similar avoidance responses when predator-naïve individuals are confronted with predator odors, e.g., fathead minnows (Pimephales promelas) (Mathis and Smith, 2008), European rabbits (Oryctolagus cuniculus) (Monclús et al., 2005), mice (Sievert and Laska, 2016), and Microtus voles (Calder and Gorman, 1991; Bolbroe et al., 2000; Borowski, 2011). However, these studies and our current results contradict the conclusions that areas with predator odor only are not avoided by foraging individuals because odor alone might not be a sufficiently reliable cue (Orrock et al., 2004; Powell and Banks, 2004). Furthermore, some studies suggest that predator odor cues are actually attractive and trigger intensive investigative behavior (Garvey et al., 2016; Parsons et al., 2018). These contradictory outcomes might result from mismatched predator odors and prey species, as not all predator odors might evoke the same reaction in prey species, or from differences in individual sensitivity to the odor (Apfelbach et al., 2005); however, in general odors are important and reliable chemosensory cues for assessing predation risk as predator odors not only reveal predator presence but can also signal predator activity patterns and diet (Kats and Dill, 1998). However, for this study a mismatch between predator and prey can be excluded.
Opposite to avoidance of predator odor, pups from mothers treated with CAC tended to go first into CAC-treated box. Early exposure to odors can alter development of the olfactory bulb and induce preferences for biologically important odors (Todrank et al., 2011). For example, mice exhibit enhanced sensitivity to olfactory cues experienced by their parents (Dias and Ressler, 2014), and a variety of young mammals (e.g., mice, rats, and humans) show preferences for individually distinctive odors associated with their mothers and their mother’s diet (Todrank et al., 2011). While these studies did not consider odors related to predation, they identify potential mechanisms linking maternal olfactory experience with offspring perception and response. Our study hints that these mechanisms might show a degree of odor specificity when it comes to risk cues, thereby highlighting differences between odors produced directly by predators and those odors produced by conspecifics having experienced predator threat (i.e., “second-hand” cues; Bian et al., 2005). While the predator-produced odor may carry a direct signal of danger, odors produced by conspecifics may need to be inspected more thoroughly, perhaps due to the complexity of their information content composition. Despite these differences, exposure to either odor may well influence the fitness of cue recipients (Bestion et al., 2014; Koyama et al., 2015).
Our study documents the relative strength of postnatal cues; this strength may result from the fact these cues can work via several mechanisms that may be additive. For example, if a mother is exposed to cues indicating predator presence (i.e., either predator odor or CAC) while outside of her nest (e.g., while foraging), she communicates that risk to her pups through changes in her body odors, her behavior, her milk, or some combination of these. Both the direct predator odor cue and the indirect CAC seem to shape the behavior of offspring, but the timing of exposure, pre- vs. postnatal, played only a minor role. While our results are mixed, our work highlights the need for more fine-tuned approaches to investigate how and when cross-generational effects are triggered in wild animals and in what ways these effects influence fitness. Moreover, the conditions under which the CAC becomes attractive, rather than repellant, warrants further study.
Data Availability Statement
The datasets presented in this study can be found in online repositories. The names of the repository/repositories and accession number(s) can be found below: 10.6084/m9.figshare.11185868 and 10.6084/m9.figshare.11186600.
Ethics Statement
The animal study was reviewed and approved by the Etelä-Suomen aluehallintovirasto (ESAVI/6370/04.10.07/2014).
Author Contributions
TS, MH, and HY designed and planned the study. TS and KB collected the data and analyzed it. TS, KB, MH, KM, and HY wrote the manuscript. All authors contributed to the article and approved the submitted version.
Funding
This study was supported by the Finnish Academy Research grant for HY (Grant No. 288990, 11.5.2015).
Conflict of Interest
The authors declare that the research was conducted in the absence of any commercial or financial relationships that could be construed as a potential conflict of interest.
Publisher’s Note
All claims expressed in this article are solely those of the authors and do not necessarily represent those of their affiliated organizations, or those of the publisher, the editors and the reviewers. Any product that may be evaluated in this article, or claim that may be made by its manufacturer, is not guaranteed or endorsed by the publisher.
Acknowledgments
We would like to thank the staff of the Konnevesi Research Station for their technical help, and Olga Ylönen and Teemu Käpylä for assistance in the laboratory.
Supplementary Material
The Supplementary Material for this article can be found online at: https://www.frontiersin.org/articles/10.3389/fevo.2021.709207/full#supplementary-material
References
Apfelbach, R., Blanchard, C. D., Blanchard, R. J., Hayes, R. A., and McGregor, I. S. (2005). The effects of predator odors in mammalian prey species: a review of field and laboratory studies. Neurosci. Biobehav. Rev. 29, 1123–1144. doi: 10.1016/j.neubiorev.2005.05.005
Apfelbach, R., Parsons, M. H., Soini, H. A., and Novotny, M. V. (2015). Are single odorous components of a predator sufficient to elicit defensive behaviors in prey species? Front. Neurosci. 9:263. doi: 10.3389/fnins.2015.00263
Bates, D., Mächler, M., Bolker, B., and Walker, S. (2015). Fitting linear mixed-effects models using lme4. J. Stat. Softw. 67, 1–48. doi: 10.18637/jss.v067.i01
Bauer, C. M., Hayes, L. D., Ebensperger, L. A., Ramírez-Estrada, J., León, C., Davis, G. T., et al. (2015). Maternal stress and plural breeding with communal care affect development of the endocrine stress response in a wild rodent. Horm. Behav. 75, 18–24. doi: 10.1016/j.yhbeh.2015.07.021
Beale, M. H., Birkett, M. A., Bruce, T. J. A., Chamberlain, K., Field, L. M., Huttly, A. K., et al. (2006). Aphid alarm pheromone produced by transgenic plants affects aphid and parasitoid behavior. Proc. Natl. Acad. Sci. U.S.A. 103, 10509–10513. doi: 10.1073/pnas.0603998103
Berghänel, A., Heistermann, M., Schülke, O., and Ostner, J. (2017). Prenatal stress accelerates offspring growth to compensate for reduced maternal investment across mammals. Proc. Natl. Acad. Sci. U.S.A. 114, E10658–E10666. doi: 10.1073/pnas.1707152114
Bestion, E., Teyssier, A., Aubret, F., Clobert, J., and Cote, J. (2014). Maternal exposure to predator scents: offspring phenotypic adjustment and dispersal. Proc. R. Soc. B 281:20140701. doi: 10.1098/rspb.2014.0701
Bian, J., Wu, Y., and Liu, J. (2005). Effect of predator-induced maternal stress during gestation on growth in root voles Microtus oeconomus. Acta Theriol. 50, 473–482. doi: 10.1007/BF03192640
Bleicher, S. S., Ylönen, H., Käpylä, T., and Haapakoski, M. (2018). Olfactory cues and the value of information: voles interpret cues based on recent predator encounters. Behav. Ecol. Sociobiol. 72, 187–199. doi: 10.1007/s00265-018-2600-9
Boissy, A., Terlouw, C., and Le Neindre, P. (1998). Presence of cues from stressed conspecifics increases reactivity to aversive events in cattle: evidence for the existence of alarm substances in urine. Physiol. Behav. 63, 489–495. doi: 10.1016/S0031-9384(97)00466-6
Bolbroe, T., Jeppesen, L. L., and Leirs, H. (2000). Behavioural response of field voles under mustelid predation risk in the laboratory: more than neophobia. Ann. Zool. Fennici 37, 169–178.
Borowski, Z. (2011). Influence of predator odour on the feeding behaviour of the root vole Microtus oeconomus (Pallas, 1776). Can. J. Zool. 76, 1791–1794. doi: 10.1139/z98-094
Bowers, W. S., Nault, L. R., Webb, R. E., and Dutky, S. R. (1972). Aphid alarm pheromone: isolation, identification, synthesis. Science 177, 1121–1122. doi: 10.1126/science.177.4054.1121
Brand, J. M., Page, H. M., Lindner, W. A., and Markovetz, A. J. (1989). Are ant alarm-defense secretions only for alarm defense? Naturwissenschaften 76:277. doi: 10.1007/BF00368641
Brechbühl, J., Moine, F., Klaey, M., Nenniger-Tosato, M., Hurni, N., Sporkert, F., et al. (2013). Mouse alarm pheromone shares structural similarity with predator scents. Proc. Natl. Acad. Sci. U.S.A. 110, 4762–4767. doi: 10.1073/pnas.1214249110
Breed, M. D., Guzmán-Novoa, E., and Hunt, G. J. (2004). Defensive behavior of honey bees: organization, genetics, and comparisons with other bees. Annu. Rev. Entomol. 49, 271–298. doi: 10.1146/annurev.ento.49.061802.123155
Brinck, C., Erlinge, S., and Sandell, M. (1983). Anal sac secretion in mustelids a comparison. J. Chem. Ecol. 9, 727–745. doi: 10.1007/BF00988779
Brown, J. S. (1988). Patch use as an indicator of habitat preference, predation risk, and competition. Behav. Ecol. Sociobiol. 22, 37–47. doi: 10.1007/BF00395696
Brown, J. S. (1999). Vigilance, patch use and habitat selection: foraging under predation risk. Evol. Ecol. Res. 1, 49–71.
Brummelte, S., Schmidt, K. L., Taves, M. D., Soma, K. K., and Galea, L. A. M. (2010). Elevated corticosterone levels in stomach milk, serum, and brain of male and female offspring after maternal corticosterone treatment in the rat. Dev. Neurobiol. 70, 714–725. doi: 10.1002/dneu.20805
Bujalska, G. (1973). The role of spacing behavior among females in the regulation of reproduction in the bank vole. J. Reprod. Fertil. Suppl. 19, 465–474.
Calder, C. J., and Gorman, M. L. (1991). The effects of red fox Vulpes vulpes faecal odours on the feeding behaviour of Orkney voles Microtus arvalis. J. Zool. 224, 599–606. doi: 10.1111/j.1469-7998.1991.tb03788.x
Chin, E. H., Love, O. P., Verspoor, J. J., Williams, T. D., Rowley, K., and Burness, G. (2009). Juveniles exposed to embryonic corticosterone have enhanced flight performance. Proc. R. Soc. B 276, 499–505. doi: 10.1098/rspb.2008.1294
Coslovsky, M., and Richner, H. (2011). Predation risk affects offspring growth via maternal effects. Funct. Ecol. 25, 878–888. doi: 10.1111/j.1365-2435.2011.01834.x
Creel, S., and Christianson, D. (2008). Relationships between direct predation and risk effects. Trends Ecol. Evol. 23, 194–201. doi: 10.1016/j.tree.2007.12.004
Dias, B. G., and Ressler, K. J. (2014). Parental olfactory experience influences behavior and neural structure in subsequent generations. Nat. Neurosci. 17, 89–96. doi: 10.1038/nn.3594
Dunn, J. C., Hamer, K. C., and Benton, T. G. (2010). Fear for the family has negative consequences: indirect effects of nest predators on chick growth in a farmland bird. J. Appl. Ecol. 47, 994–1002. doi: 10.1111/j.1365-2664.2010.01856.x
Eccard, J. A., Dammhahn, M., and Ylönen, H. (2017). The Bruce effect revisited: is pregnancy termination in female rodents an adaptation to ensure breeding success after male turnover in low densities? Oecologia 185, 81–94. doi: 10.1007/s00442-017-3904-6
Fuelling, O., and Halle, S. (2004). Breeding suppression in free-ranging grey-sided voles under the influence of predator odour. Oecologia 138, 151–159. doi: 10.1007/s00442-003-1417-y
Garvey, P. M., Glen, A. S., and Pech, R. P. (2016). Dominant predator odour triggers caution and eavesdropping behaviour in a mammalian mesopredator. Behav. Ecol. Sociobiol. 70, 481–492. doi: 10.1007/s00265-016-2063-9
Gomes, L. A. P., Salgado, P. M. P., Barata, E. N., and Mira, A. P. P. (2013). Alarm scent-marking during predatory attempts in the Cabrera vole (Microtus cabrerae Thomas, 1906). Ecol. Res. 28, 335–343. doi: 10.1007/s11284-012-1023-8
Gutiérrez-García, A. G., Contreras, C. M., Mendoza-López, M. R., García-Barradas, O., and Cruz-Sánchez, J. S. (2007). Urine from stressed rats increases immobility in receptor rats forced to swim: Role of 2-heptanone. Physiol. Behav. 91, 166–172. doi: 10.1016/j.physbeh.2007.02.006
Haapakoski, M., Hardenbol, A. A., and Matson, K. D. (2018). Exposure to chemical cues from predator-exposed conspecifics increases reproduction in a wild rodent. Sci. Rep. 8:17214. doi: 10.1038/s41598-018-35568-0
Haapakoski, M., Sundell, J., and Ylönen, H. (2012). Predation risk and food: opposite effects on overwintering survival and onset of breeding in a boreal rodent: predation risk, food and overwintering. J. Anim. Ecol. 81, 1183–1192. doi: 10.1111/j.1365-2656.2012.02005.x
Hanski, I., Henttonen, H., Korpimaki, E., Oksanen, L., and Turchin, P. (2001). Small-rodent dynamics and predation. Ecology 82:1505. doi: 10.2307/2679796
Hayward, L. S., and Wingfield, J. C. (2004). Maternal corticosterone is transferred to avian yolk and may alter offspring growth and adult phenotype. Gen. Comp. Endocrinol. 135, 365–371. doi: 10.1016/j.ygcen.2003.11.002
Heath, R. R., and Landolt, P. J. (1988). The isolation, identification and synthesis of the alarm pheromone of Vespula squamosa (Drury) (Hymenoptera: Vespidae) and associated behavior. Experientia 44, 82–83. doi: 10.1007/BF01960257
Hempel, J., Fraker, M. E., Relyea, R. A., Hu, F., Cuddapah, V., McCollum, S. A., et al. (2009). Characterization of an alarm pheromone secreted by amphibian tadpoles that induces behavioral inhibition and suppression of the neuroendocrine stress axis. Horm. Behav. 55, 520–529. doi: 10.1016/j.yhbeh.2009.01.007
Howe, N., and Sheikh, Y. (1975). Anthopleurine: a sea anemone alarm pheromone. Science 189, 386–388. doi: 10.1126/science.238286
Inagaki, H., Kiyokawa, Y., Tamogami, S., Watanabe, H., Takeuchi, Y., and Mori, Y. (2014). Identification of a pheromone that increases anxiety in rats. Proc. Natl. Acad. Sci. U.S.A. 111, 18751–18756. doi: 10.1073/pnas.1414710112
Jędrzejewska, B., and Jędrzejewski, W. (1990). Antipredatory behaviour of bank voles and prey choice of weasels – enclosure experiments. Ann. Zool. Fennici 27, 321–328.
Jędrzejewski, W., and Jędrzejewska, B. (1990). Effect of a predator’s visit on the spatial distribution of bank voles: experiments with weasels. Can. J. Zool. 68, 660–666. doi: 10.1139/z90-096
Kats, L. B., and Dill, L. M. (1998). The scent of death: chemosensory assessment of predation risk by prey animals. Écoscience 5, 361–394. doi: 10.1080/11956860.1998.11682468
Kikusui, T., Takigami, S., Takeuchi, Y., and Mori, Y. (2001). Alarm pheromone enhances stress-induced hyperthermia in rats. Physiol. Behav. 72, 45–50. doi: 10.1016/S0031-9384(00)00370-X
Kiyokawa, Y., Kikusui, T., Takeuchi, Y., and Mori, Y. (2004). Partner’s stress status influences social buffering effects in rats. Behav. Neurosci. 118, 798–804. doi: 10.1037/0735-7044.118.4.798
Korpimäki, E., Norrdahl, K., and Rinta-Jaskari, T. (1991). Responses of stoats and least weasels to fluctuating food abundances: is the low phase of the vole cycle due to mustelid predation? Oecologia 88, 552–561. doi: 10.1007/BF00317719
Koyama, S., Soini, H. A., Wager-Miller, J., Alley, W. R., Pizzo, M. J., Rodda, C., et al. (2015). Cross-generational impact of a male murine pheromone 2-sec-butyl-4,5-dihydrothiazole in female mice. Proc. Biol. Sci. 282:20151074. doi: 10.1098/rspb.2015.1074
Kuijper, B., and Johnstone, R. A. (2018). Maternal effects and parent–offspring conflict. Evolution 72, 220–233. doi: 10.1111/evo.13403
Kuwahara, Y., Leal, W. S., Nakano, Y., Kaneko, Y., Nakao, H., and Suzuki, T. (1989). Phermone study on astigmait mites?: XXIII. Identification of the alarm pheromone on the acarid mite, Tyrophagus neiswanderi and species specificities of alarm pheromones among four species of the same genus. Appl. Entomol. Zool. 24, 424–429. doi: 10.1303/aez.24.424
Liu, D. (1997). Maternal care, hippocampal glucocorticoid receptors, and hypothalamic-pituitary-adrenal responses to stress. Science 277, 1659–1662. doi: 10.1126/science.277.5332.1659
Love, O. P., and Williams, T. D. (2008). The adaptive value of stress-induced phenotypes: effects of maternally derived corticosterone on sex-biased investment, cost of reproduction, and maternal fitness. Am. Nat. 172, E135–E149. doi: 10.1086/590959
Mathis, A., and Smith, R. J. F. (2008). Avoidance of areas marked with a chemical alarm substance by fathead minnows (Pimephales promelas) in a natural habitat. Can. J. Zool. 70, 1473–1476. doi: 10.1139/z92-203
Monclús, R., Rödel, H. G., Von Holst, D., and De Miguel, J. (2005). Behavioural and physiological responses of naïve European rabbits to predator odour. Anim. Behav. 70, 753–761. doi: 10.1016/j.anbehav.2004.12.019
Nelson, E. H., Matthews, C. E., and Rosenheim, J. A. (2004). Predators reduce prey population growth by inducing changes in prey behavior. Ecology 85, 1853–1858. doi: 10.1890/03-3109
Norrdahl, K., and Korpimäki, E. (1995). Mortality factors in a cyclic vole population. Proc. R. Soc. B 261, 49–53. doi: 10.1098/rspb.1995.0116
Norrdahl, K., and Korpimäki, E. (2000). The impact of predation risk from small mustelids on prey populations. Mamm. Rev. 30, 147–156. doi: 10.1046/j.1365-2907.2000.00064.x
Orrock, J. L., Danielson, B. J., and Brinkerhoff, R. J. (2004). Rodent foraging is affected by indirect, but not by direct, cues of predation risk. Behav. Ecol. 15, 433–437. doi: 10.1093/beheco/arh031
Pangle, K. L., Peacor, S. D., and Johannsson, O. E. (2007). Large nonlethal effects of an invasive invertebrate predator on zooplankton population growth rate. Ecology 88, 402–412. doi: 10.1890/06-0768
Parsons, M. H., Apfelbach, R., Banks, P. B., Cameron, E. Z., Dickman, C. R., Frank, A. S. K., et al. (2018). Biologically meaningful scents: a framework for understanding predator-prey research across disciplines. Biol. Rev. 93, 98–114. doi: 10.1111/brv.12334
Patacchioli, F. R., Catalani, A., Scaccianoce, S., Angelucci, L., Spinozzi, P., and Casolini, P. (2002). Maternal corticosterone during lactation permanently affects brain corticosteroid receptors, stress response and behaviour in rat progeny. Neuroscience 100, 319–325. doi: 10.1016/s0306-4522(00)00277-3
Périquet, S., Todd-Jones, L., Valeix, M., Stapelkamp, B., Elliot, N., Wijers, M., et al. (2012). Influence of immediate predation risk by lions on the vigilance of prey of different body size. Behav. Ecol. 23, 970–976. doi: 10.1093/beheco/ars060
Powell, F., and Banks, P. B. (2004). Do house mice modify their foraging behaviour in response to predator odours and habitat? Anim. Behav. 67, 753–759. doi: 10.1016/j.anbehav.2003.08.016
Preisser, E. L., Bolnick, D. I., and Benard, M. F. (2005). Scared to death? The effects of intimidation and consumption in predator-prey interactions. Ecology 86, 501–509. doi: 10.1890/04-0719
Schmitz, O. J., Beckerman, A. P., and O’Brien, K. M. (1997). Behaviorally mediated trophic cascades: effects of predation risk on food web interactions. Ecology 78:1388. doi: 10.2307/2266134
Sheriff, M. J., and Love, O. P. (2013). Determining the adaptive potential of maternal stress. Ecol. Lett. 16, 271–280. doi: 10.1111/ele.12042
Sheriff, M. J., Bell, A., Boonstra, R., Dantzer, B., Lavergne, S. G., McGhee, K. E., et al. (2017). Integrating ecological and evolutionary context in the study of maternal stress. Integr. Comp. Biol. 57, 437–449. doi: 10.1093/icb/icx105
Sheriff, M. J., Krebs, C. J., and Boonstra, R. (2009). The sensitive hare: sublethal effects of predator stress on reproduction in snowshoe hares. J. Anim. Ecol. 78, 1249–1258. doi: 10.1111/j.1365-2656.2009.01552.x
Sheriff, M. J., McMahon, E. K., Krebs, C. J., and Boonstra, R. (2015). Predator-induced maternal stress and population demography in snowshoe hares: the more severe the risk, the longer the generational effect. J. Zool. 296, 305–310. doi: 10.1111/jzo.12249
Sievert, T. (2020). Indirect and Transgenerational Effects of Predation Risk: Predator Odour and Alarm Pheromones in the Bank Vole. Doctoral dissertations. Jyväskylän: University of Jyväskylä.
Sievert, T., and Laska, M. (2016). Behavioral responses of CD-1 Mice to six predator odor components. Chem. Senses 41, 399–406. doi: 10.1093/chemse/bjw015
Sievert, T., Haapakoski, M., Palme, R., Voipio, H., and Ylönen, H. (2019). Secondhand horror: effects of direct and indirect predator cues on behavior and reproduction of the bank vole. Ecosphere 10:e02765. doi: 10.1002/ecs2.2765
Sievert, T., Kerkhoven, A., Haapakoski, M., Matson, K. D., Ylönen, O., and Ylönen, H. (2020). In utero behavioral imprinting to predation risk in pups of the bank vole. Behav. Ecol. Sociobiol. 74:13. doi: 10.1007/s00265-019-2791-8
Sih, A. (1994). Predation risk and the evolutionary ecology of reproductive behaviour. J. Fish Biol. 45, 111–130. doi: 10.1111/j.1095-8649.1994.tb01087.x
St-Cyr, S., Abuaish, S., Sivanathan, S., and McGowan, P. O. (2017). Maternal programming of sex-specific responses to predator odor stress in adult rats. Horm. Behav. 94, 1–12. doi: 10.1016/j.yhbeh.2017.06.005
Sullivan, E. C., Hinde, K., Mendoza, S. P., and Capitanio, J. P. (2011). Cortisol concentrations in the milk of rhesus monkey mothers are associated with confident temperament in sons, but not daughters. Dev. Psychobiol. 53, 96–104. doi: 10.1002/dev.20483
Sundell, J., and Ylönen, H. (2004). Behaviour and choice of refuge by voles under predation risk. Behav. Ecol. Sociobiol. 56, 263–269. doi: 10.1007/s00265-004-0777-6
Sundell, J., Trebatická, L., Oksanen, T., Ovaskainen, O., Haapakoski, M., and Ylönen, H. (2008). Predation on two vole species by a shared predator: antipredatory response and prey preference. Popul. Ecol. 50, 257–266. doi: 10.1007/s10144-008-0086-4
Todrank, J., Heth, G., and Restrepo, D. (2011). Effects of in utero odorant exposure on neuroanatomical development of the olfactory bulb and odour preferences. Proc. R. Soc. B 278, 1949–1955. doi: 10.1098/rspb.2010.2314
Venables, W. N., and Ripley, B. D. (2002). Modern Applied Statistics with S, 4th Edn. New York, NY: Springer. doi: 10.1007/978-0-387-21706-2
Voznessenskaya, V. V. (2014). “Influence of cat odor on reproductive behavior and physiology in the house mouse: (Mus Musculus),” in Neurobiology of Chemical Communication, ed. C. Mucignat-Caretta (Boca Raton, FL: CRC Press/Taylor & Francis), 389–406. doi: 10.1201/b16511-15
Wallace, K. J., and Rosen, J. B. (2000). Predator odor as an unconditioned fear stimulus in rats: elicitation of freezing by trimethylthiazoline, a component of fox feces. Behav. Neurosci. 114, 912–922. doi: 10.1037/0735-7044.114.5.912
Werner, E. E., and Peacor, S. D. (2003). A review of trait-mediated indirect interactions in ecological communities. Ecology 84, 1083–1100.
Ylönen, H. (1989). Weasels Mustela Nivalis suppress reproduction in cyclic bank voles Clethrionomys Glareolus. Oikos 55:138. doi: 10.2307/3565886
Ylönen, H. (2001). “Scent of death – the effect of weasel odor on vole reproduction,” in New Mammals Encyclopedia, ed. D. W. Macdonald (Oxford: Oxford University Press), 634–635.
Ylönen, H., and Brown, J. S. (2007). “Fear and the foraging, breeding, and sociality of rodents,” in Rodent Societies: An Ecological & Evolutionary Perspective, eds J. O. Wolff and P. W. Sherman (Chicago, IL: University of Chicago Press), 610.
Ylönen, H., and Ronkainen, H. (1994). Breeding suppression in the bank vole as antipredatory adaptation in a predictable environment. Evol. Ecol. 8, 658–666. doi: 10.1007/BF01237848
Keywords: alarm pheromone, conspecific alarm cue, predation risk, odor cues, cross-generational effects, rodents
Citation: Sievert T, Bouma K, Haapakoski M, Matson KD and Ylönen H (2021) Pre- and Postnatal Predator Cues Shape Offspring Anti-predatory Behavior Similarly in the Bank Vole. Front. Ecol. Evol. 9:709207. doi: 10.3389/fevo.2021.709207
Received: 13 May 2021; Accepted: 24 November 2021;
Published: 14 December 2021.
Edited by:
Peter Banks, The University of Sydney, AustraliaReviewed by:
Burt P. Kotler, Ben-Gurion University of the Negev, IsraelGabriel Francescoli, Universidad de la República, Uruguay
Copyright © 2021 Sievert, Bouma, Haapakoski, Matson and Ylönen. This is an open-access article distributed under the terms of the Creative Commons Attribution License (CC BY). The use, distribution or reproduction in other forums is permitted, provided the original author(s) and the copyright owner(s) are credited and that the original publication in this journal is cited, in accordance with accepted academic practice. No use, distribution or reproduction is permitted which does not comply with these terms.
*Correspondence: Thorbjörn Sievert, dGhvcmJqb2Vybi5zaWV2ZXJ0QGdteC5uZXQ=
†ORCID: Thorbjörn Sievert orcid.org/0000-0002-4242-3779; Kerstin Bouma orcid.org/0000-0002-3366-1003; Marko Haapakoski orcid.org/0000-0003-0901-6319; Kevin D. Matson orcid.org/0000-0002-4373-5926; Hannu Ylönen orcid.org/0000-0001-6935-1464
‡These authors have contributed equally to this work