- Groningen Institute for Evolutionary Life Science, University of Groningen, Groningen, Netherlands
Floral pigments are a core component of flower colors, but how much pigment a flower should have to yield a strong visual signal to pollinators is unknown. Using an optical model and taking white, blue, yellow and red flowers as case studies, I investigate how the amount of pigment determines a flower’s color contrast. Modeled reflectance spectra are interpreted using established insect color vision models. Contrast as a function of the amount of pigment shows a pattern of diminishing return. Low pigment amounts yield pale colors, intermediate amounts yield high contrast, and extreme amounts of pigment do not further increase, and sometimes even decrease, a flower’s color contrast. An intermediate amount of floral pigment thus yields the highest visibility, a finding that is corroborated by previous behavioral experiments on bees. The implications for studies on plant-pollinator signaling, intraspecific flower color variation and the costs of flower color are discussed.
Introduction
The coloration of flowers is a major component of plant-pollinator signaling. Flower coloration is due to two optical principles: reflection and scattering of light by the flower’s surface and interior structures, and wavelength-selective absorption of scattered light by floral pigments (Kay et al., 1981; Kevan and Backhaus, 1998; van der Kooi et al., 2016, 2019). The degree of filtering of the reflected light by floral pigments determines a flower’s color contrast to a (green leaf) background. For example, a low amount of pigment yields a pale color, whereas a higher amount of pigment generally results in a more marked color. Whether and how scattering structures and floral pigments are tuned so to yield visually contrasting floral displays to pollinators remains an open question.
To understand if and how different aspects of floral optical properties are tuned, it is important to consider how much the pigmentation and scattering properties vary—both within and between species. Pigmentation is probably more evolutionarily and developmentally labile than are the structural properties of flowers. This difference in variation of pigmentation vs scattering structures can be understood from both a mechanistic and functional point of view. First, the flower pigmentation of numerous species exhibits considerable intraspecific variation [reviewed by Sapir et al. (2021)]. Intraspecific flower color variation can be discrete (Schemske and Bierzychudek, 2007; Streinzer et al., 2019; von Witt et al., 2020; Buide et al., 2021) or continuous, e.g., covering the spectrum of white, pink and purple in Trifolium pratense (Figure 1), orchids (Sletvold et al., 2016; Dormont et al., 2019) and the Iris atropurpurea complex (Roguz et al., 2020). The amount of pigment that is synthesized is a quantitative trait, and therefore small changes in the pigment synthesis pathway can yield appreciable changes in color (Shrestha et al., 2014; van der Kooi et al., 2019). Molecular studies also suggest that the synthesis of floral pigment is an evolutionary labile trait (Koes et al., 1994; Rausher, 2008; Wessinger et al., 2014; Sapir et al., 2021). In contrast, the structural aspects that determine how light is reflected, i.e., the number and type of cells, and flower thickness, are most likely phylogenetically and developmentally constrained (Martin and Gerats, 1993; van der Kooi et al., 2016). Indeed, in related species with differently pigmented flowers, the cellular structures are similar (Martin and Gerats, 1993; Stavenga et al., 2021). Further, whereas pigmentation virtually solely serves for visibility, the thickness and interior structure of flowers are also important for the flower’s mechanical strength. Given the amount of pigment can vary within a species, for a flower with a certain backscattering, how much pigment yields the highest color contrast?
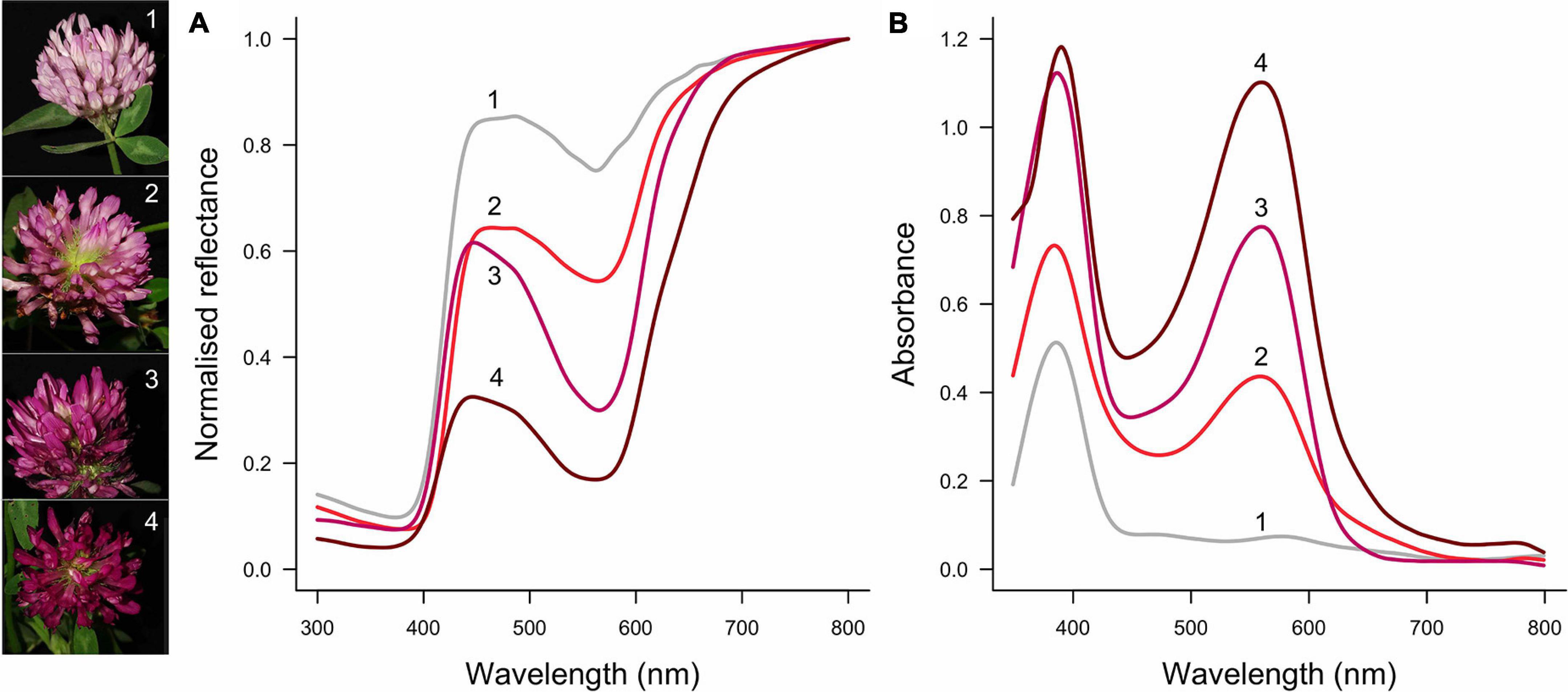
Figure 1. Example of intraspecific flower color variation in Trifolium pratense. Flower color ranges from light pink (1) via pink (2, 3) to dark red (4). From light to dark, modulation of the reflectance spectra increases, particularly between 500 and 600 nm (A), which is due to an increase in the amount of pigment with peak absorption at ∼550 nm (B). Flowers of this species also have a UV-absorbing pigment, the amount of which varies less than the pigment that absorbs in the green wavelength range. The numbered spectra correspond to the left pictures (photo credit: Alina Höwener).
Based on evidence from studies on the optical properties of flowers and insect vision, I hypothesize that intermediate amounts of floral pigment yield the most conspicuous colors. Floral pigment absorption spectra generally feature a broadband unimodal distribution (see Figure 3 in van der Kooi et al., 2016). A low amount of pigment will yield a pale color, but because of the broadband absorption, extremely high amounts of floral pigment often yield dark, dull colors. For example, different amounts of a highly similar floral pigment create different shades of red in Papaver flowers and yellow/orange Meconopsis cambrica flowers (see Figure 6 in van der Kooi and Stavenga, 2019). Papiorek et al. (2013), investigating how quickly honeybees and bumblebees detect artificial stimuli with different concentrations of blue or orange pigment, found that bees most easily detect stimuli with intermediate concentrations of floral pigment.
Here, I provide a framework that enables exploration of how the amount of pigment determines a flower’s visibility to pollinators, with the aim to test whether there are optima in the amount of pigment. Using our previously developed optical model (Stavenga and van der Kooi, 2016), I investigate how systematic changes in the amount of pigment change the reflectance spectrum of white, blue, yellow and red flowers. The resulting reflectance spectra are interpreted with a “pollinator-subjective view” using established models for animal color vision (Chittka, 1992; Vorobyev and Osorio, 1998). It is thus found that the highest color contrast is often obtained by moderate amounts of pigment. Finally, I discuss how this approach could help to further our understanding of the optical properties and costs of floral displays.
Materials and Methods
Model Species
Four species with different flower colors were chosen: the white campion Silene latifolia (ssp. alba) Poir. (Caryophyllaceae), the Chilean bellflower Nolana paradoxa Lindl. (Solanaceae), the Missouri evening primrose Oenothera macrocarpa Nutt. (also known as O. missouriensis, Onagraceae) and the common poppy Papaver rhoeas L. (Papaveraceae) (Figure 2). Together these species span the spectrum of flower colors that are common in nature. S. latifolia and O. macrocarpa are pollinated by nocturnal moths (e.g., Young, 2002; Krakos and Austin, 2020), and P. rhoeas in Europe is pollinated by bees (van der Kooi and Stavenga, 2019). For N. paradoxa I could not find reliable data on the pollinating species. For N. paradoxa and P. rhoeas, we previously applied the optical model to study the optical properties (particularly the backscattering) of these flowers (Stavenga and van der Kooi, 2016; van der Kooi and Stavenga, 2019).
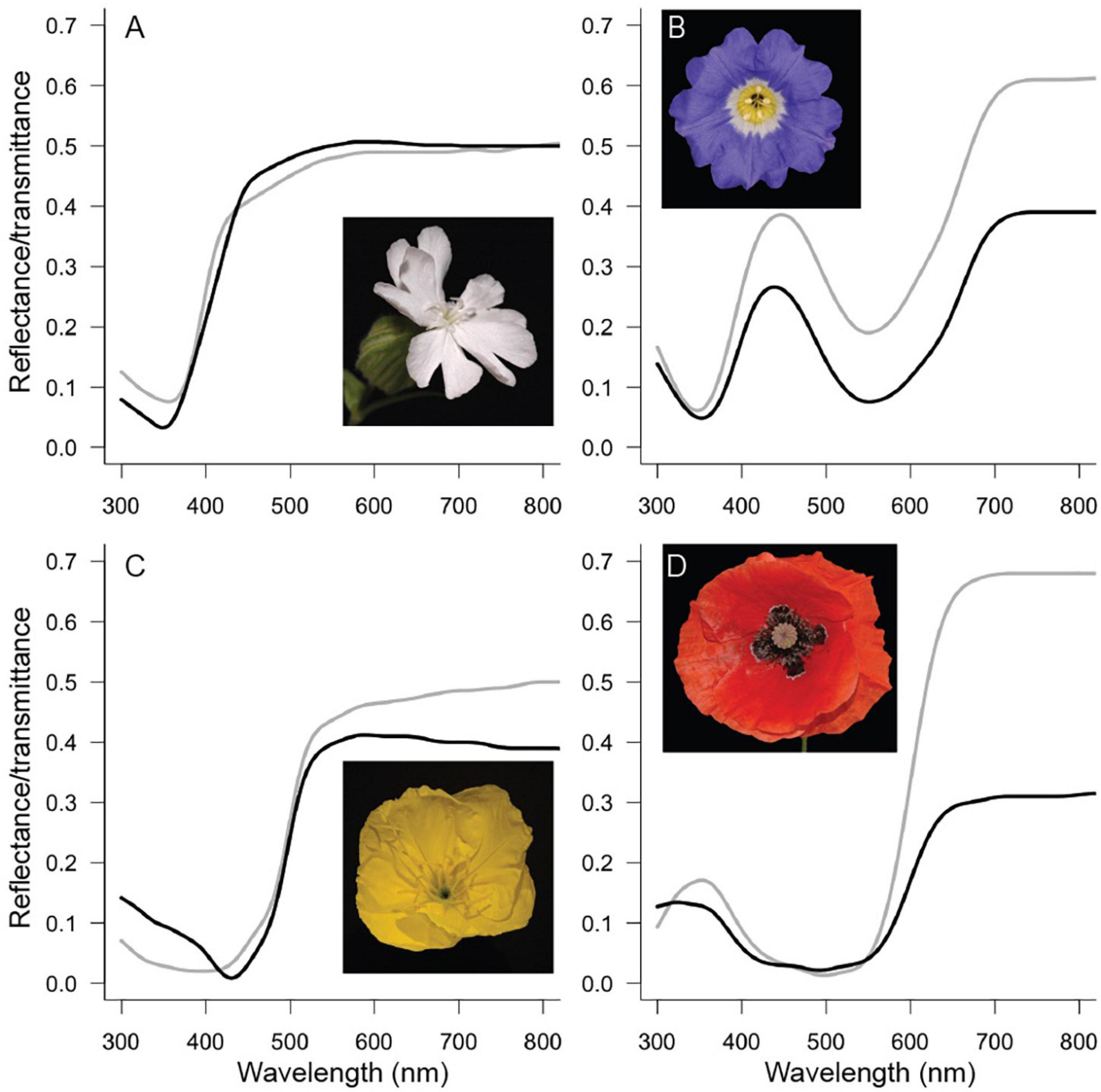
Figure 2. Flowers investigated in this study. Measured reflectance (black curves) and transmittance (gray curves) spectra for Silene latifolia (A), Nolana paradoxa (B), Oenothera macrocarpa (C), and Papaver rhoeas (D).
Spectroscopy
Reflectance spectra of the four species were measured with an integrating sphere (AvaSphere-50-Refl) and a deuterium-halogen lamp [AvaLight-D(H)-S] and the spectrometer an AvaSpec-2048 (Avantes, Netherlands). A piece of flower was directionally and about perpendicularly illuminated from within the sphere at an area with diameter ∼5 mm. A white diffuse tile (Avantes WS-2) was used as a reference. For transmittance measurements, the sample was illuminated from outside the sphere with a fiber, with an illumination spot size of ∼1 mm. To model the spectra, it is necessary to have the (absolute) amounts of transmittance and reflectance, which can be obtained with an integrating sphere and not with a reflection probe. For T. pratense (Figure 1A) the reflectance spectra were obtained with a bifurcated reflection probe, because the flowers were too small to be measured with the sphere. Petal absorbance spectra (Figure 1B) were obtained using a microspectrophotometer equipped with a xenon arc light source (for details, see Stavenga and van der Kooi, 2016).
Modeling
To obtain reflectance spectra of similar shape but with different degrees of modulation, I applied our previously developed optical model (Stavenga and van der Kooi, 2016). That model combines the Kubelka-Munk theory for scattering and absorbing media (Kubelka and Munk, 1931; Allen et al., 1969) with a layer-stack model (Stavenga et al., 2006). Two key aspects of the model are the scattering and absorption parameters, S∗ and K∗, which can be derived from the measured reflectance (R) and transmittance (T) (Yamada and Fujimura, 1991; Stavenga and van der Kooi, 2016):
with
S∗ and K∗ are proportional to the amount of scattering and absorption, respectively. After the scattering and absorption parameters of a flower have been calculated using measured spectra (Figures 3A,B), modeled reflectance spectra can be obtained using a series of matrix calculations (see the appendix of Stavenga and van der Kooi, 2016; also see the R script). Spectra of similar shape but with different modulation were obtained by systematically varying K∗ but leaving S∗ unchanged, because K∗ is the main factor that determines the modulation. The contribution of K∗ in the model was set to be 0.2, 0.4, 0.6 … 4 (Figure 3C). The chosen parameter range yields spectra that are similar to those that can be found in real flowers and enables modeling cases of extreme amounts of pigment. What follows is a series of reflectance spectra, which are similar to those of flowers with different amounts of the same pigment (Figure 3D). S∗ was kept identical to the value obtained for real flowers, so the modulation of the reflectance spectrum varied independently from the total amount of reflectance (see the convergence in the long wavelength range, Figure 3D).
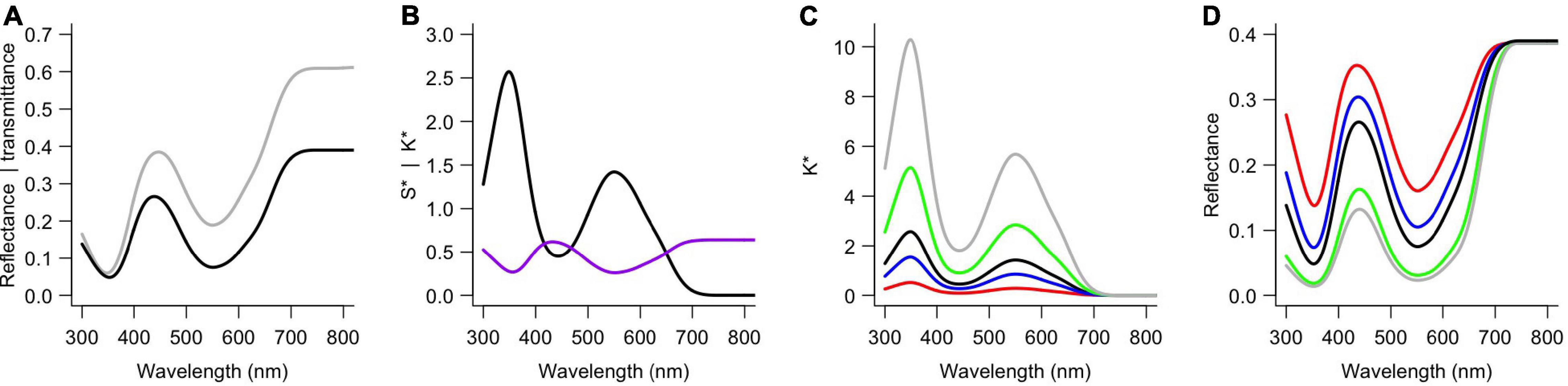
Figure 3. Example spectra and calculations for the blue flowers of Nolana paradoxa. (A) Measured reflectance (black) and transmittance (gray) spectra (as in Figure 2B). (B) Absorption (K*, black) and scattering parameter (S*, purple) calculated using the measured spectra from panel (A). (C) Different absorption parameters used for the modeling; 0.2K* (red), 0.6K* (blue), K* [black, as in panel (B)], 2K* (green) and 4K* (gray). (D) Reflectance spectra obtained using the absorption parameters as in panel (C) (with corresponding colors).
Different optical processes determine the modulation of the reflected light and the applied optical model can be used to quantitatively investigate that, but the approach used here is not to study these factors. The aim of this study is to investigate the consequences of variation in absorption by pigments, but not via which anatomical ways this is achieved, because that requires detailed species-specific anatomical information. For example, increases in modulation of the reflected light can be obtained by increasing the total amount of pigment as well as by concentrating a smaller amount of pigment in the outer layer at the side of viewing (see Figure 5 in van der Kooi et al., 2016). To standardize the degree of modulation among the four different species, I used fractions and multiples of the empirical K∗ (Figure 3). The different cases are classified by a “pigment index,” with pigment index = 5 being identical to the measured spectra (Figure 3) and lower and higher indices being the more weakly and strongly modulated cases, respectively.
It is important to point out that scattering and absorption, and so S∗ and K∗, are not strictly separated, because presence of pigments (slightly) influences the refractive index of a medium, and thereby the medium’s scattering. Nevertheless, the obtained spectra are highly similar to those found in nature, so the chosen approach is sufficient to study the effect of modulation for visibility to pollinators.
To interpret the different reflectance spectra with a pollinator subjective view, I deployed two commonly used insect vision models, i.e., the hexagon and the receptor noise-limited model (Chittka, 1992; Vorobyev and Osorio, 1998), using honeybee (Apis mellifera) and hawk moth (Deilephila elpenor) spectral sensitivities (van der Kooi et al., 2021a) and an average green leaf background under D65 ambient illumination. Both models yield a color contrast value, which is broadly considered a good proxy for visibility to a wide range of animals (Giurfa et al., 1996; Spaethe et al., 2001; Kelber et al., 2003; Dyer and Chittka, 2004).
Results
Color Contrast Follows a Pattern of Diminishing Return
When color contrast is modeled as a function of the amount of pigment, for all four cases there is a non-linear relationship. At low levels (i.e., for pale flowers), an increase in the amount of pigment enhances the flower’s contrast to the background. However, at a certain (species-specific) point, the color contrast curve plateaus, and further increases in the amount of pigment will not increase or even decrease color contrast (Figure 4)—indicating a pattern of diminishing return. The relationship of the color contrast and the amount of pigment depends on the type of pigment, but at least for the modeled white, blue and yellow colors the overall effect is similar. For the (ultraviolet reflecting) red P. rhoeas flowers the amount of pigment does not drastically change the flower’s contrast, meaning that within the current set of parameters changing the amount of pigment has a comparatively small effect on the visibility. Nevertheless, also for this species, the overall observation of diminishing returns in contrast with the amount of pigment is supported.
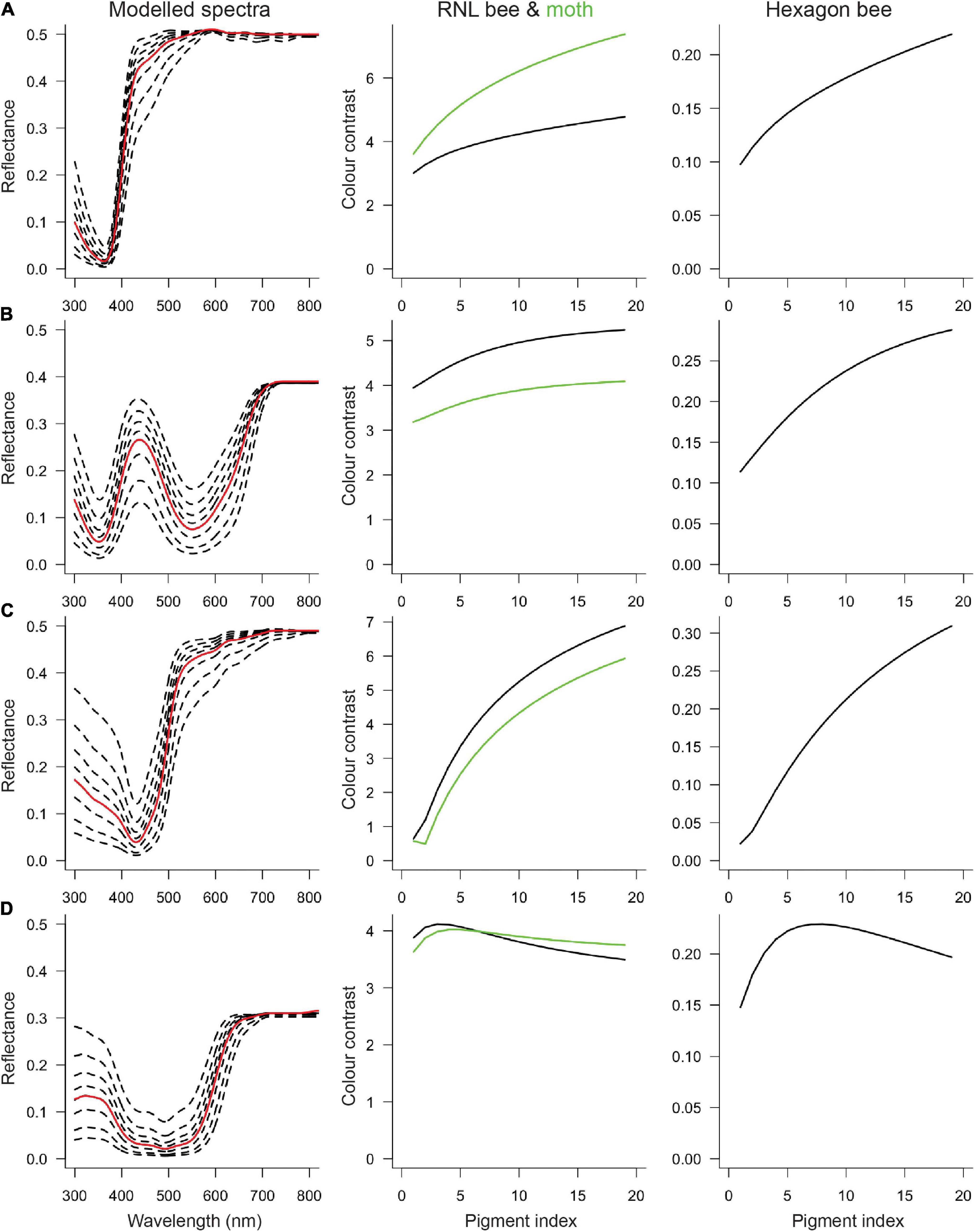
Figure 4. Color contrast for reflectance spectra with different degrees of modulation. The first column shows the measured reflectance spectra (red curve) and a selection of the modeled cases (dashed curves), including the most weakly and strongly modulated spectra. The measured reflectance is identical to the fifth modeled spectrum, so the empirical color contrast values are at pigment index = 5. The second column shows bee (black) and moth (green) color contrast calculated with the receptor noise-limited (RNL) model. The third column shows bee color contrast calculated with the hexagon model. (A) Silene latifolia, (B) Nolana paradoxa, (C) Oenothera macrocarpa, and (D) Papaver rhoeas.
The results are largely vision model-independent, that is, within one color category, color contrast as a function of the amount of pigment is similar regardless of whether the hexagon or the receptor noise-limited model was applied (compare columns 2 and 3 in Figure 4). The effect of the amount of pigment on color contrast is also similar for bees and hawk moths (compare black and green in column 2, Figure 4), though there is one interesting exception. For moth-pollinated S. latifolia, color contrast increases more for moths than for bees (Figure 4A), suggesting that increasing the amount of pigment would disproportionally improve visibility to pollinators. However, in the other moth-pollinated species, O. macrocarpa, the effect may be opposite with (slightly) larger increases for bees than for moths.
Intriguingly, for almost all species, empirical contrast values (pigment index = 5) are not the highest that are theoretically possible. For the (ultraviolet reflecting) red poppy flower, the empirical color contrast is very close to the theoretical optimum, but for white, blue and yellow flowers, more wavelength-specific absorption by pigment would increase visibility. On the other hand, the empirical color contrast values are (well) above the detection threshold for bees, which are ∼0.10 for the hexagon model (Dyer and Chittka, 2004) and ∼2 for the RNL model (Vorobyev et al., 2001).
Discussion
Floral pigments are a crucial component of flower coloration. Whereas numerous studies showed that the type of pigment determines flower color and visibility to pollinators (e.g., Rausher, 2008; Lunau et al., 2011; Shrestha et al., 2013), it is virtually unknown how much pigment is needed to produce a conspicuous flower. The amount of pigment can, however, vary within and between species (Introduction), meaning that the amount of absorption of light by floral pigments can be tuned to the scattering structures.
Using a previously developed optical model, I systematically varied the amount of pigment for four differently colored flowers. Interpretation with established bee and moth vision models revealed that more pigment does not necessarily improve, and may even reduce, a flower’s visibility. This means that pale flowers have a relatively low contrast to the background and flowers with an intermediate amount of pigment a high contrast. Above a certain amount of pigment, the reflected light is sufficiently modulated to be clearly visible to pollinators and more pigment does not increase the flower’s visibility to pollinators. Owing to the broadband absorption range that is typical for floral pigments (van der Kooi et al., 2016), a very high amount of pigment may render the flower dull and little contrasting with the green background. The modeling results dovetail those of Papiorek et al. (2013) who found that honeybees and bumblebees most easily detect artificial stimuli with intermediate amounts of blue and orange pigments.
The observation that for three of the four cases, the flowers do not exhibit the amount of pigment that would yield the highest contrast that is theoretically possible suggests that there is a trade-off between visibility and investment in floral pigments. Although more research is needed to infer whether this is a general phenomenon, it is tempting to speculate why flowers would not exhibit maximum contrast. Increases in pigmentation may be not worth the energy investment once the threshold at which pollinators readily detect the flower has been reached. Color contrast does not scale linearly with detectability by animals, but generally shows a sigmoidal relationship (Olsson et al., 2015; Garcia et al., 2017, 2020; Santiago et al., 2020). Above the detection threshold, which is rapidly reached in the cases considered here, further increases in color contrast may not increase the likelihood of detection by pollinators. Alternatively, for plant species that share pollinators and have the same floral pigment, differences in the amount of pigment may help to obtain more dissimilar flower colors and so enhance character displacement.
The Amount of Pigment and Perspectives for Future Studies on Flower Color
The observation that, in addition to the type of pigment, the amount of pigment is important for flower visibility is relatively overlooked in studies on flower color. These results open perspectives for future research in various directions.
Intraspecific trait variation (provided that it is heritable) is the cornerstones of evolution, and studies on the mechanistic underpinnings and consequences of such variation are paramount in understanding trait evolution (van der Kooi et al., 2021b). Generally, yellow colors are generated by carotenoids, white flowers by flavonols, and blue, purple, and red colors by anthocyanin pigments. Of these types of pigments, anthocyanins are most commonly associated with flower color polymorphisms (Sapir et al., 2021). Indeed, many species for which flower color variation was reported (Frey, 2004; Rakosy et al., 2012; Renoult et al., 2013; Sletvold et al., 2016; Dormont et al., 2019; Paine et al., 2019; Streinzer et al., 2019; Gómez et al., 2020; Whitney et al., 2020) have blue, pink or purple colors that are commonly due to anthocyanin pigments. It would be interesting to know, for these species, how standing variation in pigmentation relates to the flower’s conspicuousness to their pollinators.
The four cases considered here revealed (subtle) differences between types of pigment, as well as between bee and moth visual systems in how close the empirical amount of pigment is to the theoretical optimum and the variation around that optimum. Comparative studies covering species that are serviced by pollinators with different visual systems can elucidate the role of the amount of pigment in tuning flower color to pollinator vision. Something to consider is that the perceived flower color variation probably differs across pollinators. Whitney et al. (2020), for example, recently showed that for the same set of flowers, birds perceive more intraspecific variation than bees. It is known that different pollinators select for different flower colors (hues) owing to variation in spectral sensitivity and/or color preferences (Lunau et al., 2011; Shrestha et al., 2013; van der Kooi et al., 2019), but pollinators may also impose different selective pressures on the degree of variation around a certain hue. In other words, the degree of stabilizing selection on flower color probably varies with the type of pollinator, which would translate to different optima ranges in the amount of pigment.
To conclude, the amount of pigment is an important though often overlooked aspect of flower coloration. The amount of pigment shows a pattern of diminishing return and, corroborated by behavioral experiments with bees (Papiorek et al., 2013), I conclude that intermediate amounts often yield sufficient, if not maximal, visibility to pollinators. Future studies on how much floral pigment is needed to create conspicuous flowers will further our understanding on (the maintenance of) flower color variation and the costs of floral displays (Obeso, 2002; Roddy et al., 2020).
Data Availability Statement
The original contributions presented in the study are included in the article/Supplementary Material, further inquiries can be directed to the corresponding author/s.
Author Contributions
The author confirms being the sole contributor of this work and has approved it for publication.
Funding
The author was funded by the Dutch Science Foundation (016.Veni.181.025) and by AFOSR/EOARD (FA9550-19-1-7005).
Conflict of Interest
The author declares that the research was conducted in the absence of any commercial or financial relationships that could be construed as a potential conflict of interest.
Publisher’s Note
All claims expressed in this article are solely those of the authors and do not necessarily represent those of their affiliated organizations, or those of the publisher, the editors and the reviewers. Any product that may be evaluated in this article, or claim that may be made by its manufacturer, is not guaranteed or endorsed by the publisher.
Acknowledgments
The author would like to thank Doekele Stavenga and Johannes Spaethe for interesting discussions and comments on an early version of this manuscript. Two reviewers provided suggestions that greatly improved the manuscript. The author would also like to thank Alina Höwener for providing the pictures of T. pratense used in Figure 1.
Supplementary Material
The Supplementary Material for this article can be found online at: https://www.frontiersin.org/articles/10.3389/fevo.2021.731626/full#supplementary-material
References
Allen, W. A., Gausman, H. W., Richardson, A. J., and Thomas, J. R. (1969). Interaction of isotropic light with a compact plant leaf. J. Opt. Soc. Am. 59, 1376–1379. doi: 10.1364/josa.59.001376
Buide, M. L., Del Valle, J. C., Prado-Comesaña, A., and Narbona, E. (2021). The effects of pollination, herbivory and autonomous selfing on the maintenance of flower colour variation in Silene littorea. Plant Biol. 23, 275–284. doi: 10.1111/plb.13209
Chittka, L. (1992). The colour hexagon: a chromaticity diagram based on photoreceptor excitations as a generalized representation of colour opponency. J. Comp. Physiol. A 170, 533–543.
Dormont, L., Joffard, N., and Schatz, B. (2019). Intraspecific variation in floral color and odor in orchids. Int. J. Plant Sci. 180, 1036–1058. doi: 10.1086/705589
Dyer, A. G., and Chittka, L. (2004). Biological significance of distinguishing between similar colours in spectrally variable illumination: bumblebees (Bombus terrestris) as a case study. J. Comp. Physiol. A 190, 105–114. doi: 10.1007/s00359-003-0475-2
Frey, F. M. (2004). Opposing natural selection from herbivores and pathogens may maintain floral color variation in Claytonia virginica (Portulacaceae). Evolution 58, 2426–2437. doi: 10.1111/j.0014-3820.2004.tb00872.x
Garcia, J. E., Phillips, R. D., Peter, C. I., and Dyer, A. G. (2020). Changing how biologists view flowers-colour as a perception not a trait. Front. Plant Sci. 11:1775.
Garcia, J. E., Spaethe, J., and Dyer, A. G. (2017). The path to colour discrimination is S-shaped: behaviour determines the interpretation of colour models. J. Comp. Physiol. A 203, 983–997. doi: 10.1007/s00359-017-1208-2
Giurfa, M., Vorobyev, M., Kevan, P., and Menzel, R. (1996). Detection of coloured stimuli by honeybees: minimum visual angles and receptor specific contrasts. J. Comp. Physiol. A 178, 699–709.
Gómez, J. M., Perfectti, F., Armas, C., Narbona, E., González-Megías, A., Navarro, L., et al. (2020). Within-individual phenotypic plasticity in flowers fosters pollination niche shift. Nat. Commun. 11:4019. doi: 10.1038/s41467-020-17875-1
Kay, Q. O. N., Daoud, H. S., and Stirton, C. H. (1981). Pigment distribution, light reflection and cell structure in petals. Bot. J. Linn. Soc. 83, 57–83. doi: 10.1111/j.1095-8339.1981.tb00129.x
Kelber, A., Vorobyev, M., and Osorio, D. (2003). Animal colour vision–behavioural tests and physiological concepts. Biol. Rev. 78, 81–118. doi: 10.1017/s1464793102005985
Kevan, P. G., and Backhaus, W. G. K. (1998). “Color vision: ecology and evolution in making the best of the photic environment,” in Color Vision: Perspectives from Different Disciplines, eds W. G. K. Backhaus, R. Kliegl, and J. S. Werner (Berlin: De Gruyter).
Koes, R. E., Quattrocchio, F., and Mol, J. N. M. (1994). The flavonoid biosynthetic pathway in plants: function and evolution. BioEssays 16, 123–132. doi: 10.1002/bies.950160209
Krakos, K. N., and Austin, M. W. (2020). Testing pollination syndromes in oenothera (Onagraceae). J. Pollinat. Ecol. 26, 52–66.
Kubelka, P., and Munk, F. (1931). Ein beitrag zur optik der farbanstriche. Zeitschrift für Tech. Phys. 12, 593–601.
Lunau, K., Papiorek, S., Eltz, T., and Sazima, M. (2011). Avoidance of achromatic colours by bees provides a private niche for hummingbirds. J. Exp. Biol. 214, 1607–1612. doi: 10.1242/jeb.052688
Martin, C., and Gerats, T. (1993). Control of pigment biosynthesis genes during petal development. Plant Cell 5:1253. doi: 10.1105/tpc.5.10.1253
Obeso, J. R. (2002). The costs of reproduction in plants. New Phytol. 155, 321–348. doi: 10.1046/j.1469-8137.2002.00477.x
Olsson, P., Lind, O., and Kelber, A. (2015). Bird colour vision: behavioural thresholds reveal receptor noise. J. Exp. Biol. 218, 184–193. doi: 10.1242/jeb.111187
Paine, K. C., White, T. E., and Whitney, K. D. (2019). Intraspecific floral color variation as perceived by pollinators and non-pollinators: evidence for pollinator-imposed constraints? Evol. Ecol. 33, 461–479. doi: 10.1007/s10682-019-09991-2
Papiorek, S., Rohde, K., and Lunau, K. (2013). Bees’ subtle colour preferences: how bees respond to small changes in pigment concentration. Naturwissenschaften 100, 633–643. doi: 10.1007/s00114-013-1060-3
Rakosy, D., Streinzer, M., Paulus, H. F., and Spaethe, J. (2012). Floral visual signal increases reproductive success in a sexually deceptive orchid. Arthropod. Plant. Interact. 6, 671–681. doi: 10.1007/s11829-012-9217-0
Rausher, M. D. (2008). Evolutionary transitions in floral color. Int. J. Plant Sci. 169, 7–21. doi: 10.1086/523358
Renoult, J. P., Thomann, M., Schaefer, H. M., and Cheptou, P. O. (2013). Selection on quantitative colour variation in Centaurea cyanus: the role of the pollinator’s visual system. J. Evol. Biol. 26, 2415–2427. doi: 10.1111/jeb.12234
Roddy, A. B., Martínez-Perez, C., Teixido, A. L., Cornelissen, T. G., Olson, M. E., Oliveira, R. S., et al. (2020). Towards the flower economics spectrum. New Phytol. 229, 665–672. doi: 10.1111/nph.16823
Roguz, K., Gallagher, M. K., Senden, E., Bar-Lev, Y., Lebel, M., Heliczer, R., et al. (2020). All the colors of the rainbow: diversification of flower color and intraspecific color variation in the genus Iris. Front. Plant Sci. 11:1519.
Santiago, C., Green, N. F., Hamilton, N., Endler, J. A., Osorio, D. C., Marshall, N. J., et al. (2020). Does conspicuousness scale linearly with colour distance? A test using reef fish. Proc. R. Soc. B 287:20201456. doi: 10.1098/rspb.2020.1456
Sapir, Y., Gallagher, M. K., and Senden, E. (2021). What maintains flower colour variation within populations? Trends Ecol. Evol. 36, 507–519. doi: 10.1016/j.tree.2021.01.011
Schemske, D. W., and Bierzychudek, P. (2007). Spatial differentiation for flower color in the desert annual Linanthus parryae: was Wright right? Evolution 61, 2528–2543. doi: 10.1111/j.1558-5646.2007.00219.x
Shrestha, M., Dyer, A. G., Bhattarai, P., and Burd, M. (2014). Flower colour and phylogeny along an altitudinal gradient in the Himalayas of Nepal. J. Ecol. 102, 126–135. doi: 10.1111/1365-2745.12185
Shrestha, M., Dyer, A. G., Boyd-Gerny, S., Wong, B. B. M., and Burd, M. (2013). Shades of red: bird-pollinated flowers target the specific colour discrimination abilities of avian vision. New Phytol. 198, 301–310. doi: 10.1111/nph.12135
Sletvold, N., Trunschke, J., Smit, M., Verbeek, J., and Ågren, J. (2016). Strong pollinator-mediated selection for increased flower brightness and contrast in a deceptive orchid. Evolution 70, 716–724. doi: 10.1111/evo.12881
Spaethe, J., Tautz, J., and Chittka, L. (2001). Visual constraints in foraging bumblebees: flower size and color affect search time and flight behavior. Proc. Natl. Acad. Sci. U.S.A. 98, 3898–3903. doi: 10.1073/pnas.071053098
Stavenga, D. G., Giraldo, M. A., and Hoenders, B. J. (2006). Reflectance and transmittance of light scattering scales stacked on the wings of pierid butterflies. Opt. Express 14, 4880–4890. doi: 10.1364/oe.14.004880
Stavenga, D. G., Leertouwer, H. L., Dudek, B., and van der Kooi, C. J. (2021). Colouration of flowers by flavonoids and consequences of pH dependent absorption. Front. Plant Sci. 11:2148. doi: 10.3389/fpls.2020.600124
Stavenga, D. G., and van der Kooi, C. J. (2016). Coloration of the Chilean bellflower, Nolana Paradoxa, interpreted with a scattering and absorbing layer stack model. Planta 243, 171–181. doi: 10.1007/s00425-015-2395-0
Streinzer, M., Roth, N., Paulus, H. F., and Spaethe, J. (2019). Color preference and spatial distribution of glaphyrid beetles suggest a key role in the maintenance of the color polymorphism in the peacock anemone (Anemone pavonina, Ranunculaceae) in Northern Greece. J. Comp. Physiol. A 205, 735–743. doi: 10.1007/s00359-019-01360-2
van der Kooi, C. J., Dyer, A. G., Kevan, P. G., and Lunau, K. (2019). Functional significance of the optical properties of flowers for visual signalling. Ann. Bot. 123, 263–276. doi: 10.1093/aob/mcy119
van der Kooi, C. J., Elzenga, J. T. M., Staal, M., and Stavenga, D. G. (2016). How to colour a flower: on the optical principles of flower coloration. Proc. R. Soc. B 283:20160429. doi: 10.1098/rspb.2016.0429
van der Kooi, C. J., and Stavenga, D. G. (2019). Vividly coloured poppy flowers due to dense pigmentation and strong scattering in thin petals. J. Comp. Physiol. A 205, 363–372. doi: 10.1007/s00359-018-01313-1
van der Kooi, C. J., Stavenga, D. G., Belusic, G., Arikawa, K., and Kelber, A. (2021a). Evolution of insect color vision: from spectral sensitivity to visual ecology. Annu. Rev. Entomol. 66, 435–462. doi: 10.1146/annurev-ento-061720-071644
van der Kooi, C. J., Vallejo-Marín, M., and Leonhardt, S. D. (2021b). Mutualisms and (a)symmetry in plant-pollinator interactions. Curr. Biol. 31, R91–R99. doi: 10.1016/j.cub.2020.11.020
von Witt, C. G., Anderson, B., Durbach, I. N., and Johnson, S. D. (2020). Breeding systems of floral colour forms in the Drosera cistiflora species complex. Plant Biol. 22, 992–1001. doi: 10.1111/plb.13159
Vorobyev, M., Brandt, R., Peitsch, D., Laughlin, S. B., and Menzel, R. (2001). Colour thresholds and receptor noise: behaviour and physiology compared. Vision Res. 41, 639–653. doi: 10.1016/s0042-6989(00)00288-1
Vorobyev, M., and Osorio, D. (1998). Receptor noise as a determinant of colour thresholds. Proc. R. Soc. London B 265, 351–358. doi: 10.1098/rspb.1998.0302
Wessinger, C. A., Hileman, L. C., and Rausher, M. D. (2014). Identification of major quantitative trait loci underlying floral pollination syndrome divergence in Penstemon. Philos. Trans. R. Soc. B Biol. Sci. 369:20130349. doi: 10.1098/rstb.2013.0349
Whitney, K. D., Smith, A. K., Williams, C., and White, T. E. (2020). Birds perceive more intraspecific color variation in bird-pollinated than bee-pollinated flowers. Front. Plant Sci. 11:1806.
Yamada, N., and Fujimura, S. (1991). Nondestructive measurement of chlorophyll pigment content in plant leaves from three-color reflectance and transmittance. Appl. Opt. 30, 3964–3973. doi: 10.1364/ao.30.003964
Keywords: pigmentation, color vision, pollination, reflection, absorbance, contrast, diminishing return
Citation: van der Kooi CJ (2021) How Much Pigment Should Flowers Have? Flowers With Moderate Pigmentation Have Highest Color Contrast. Front. Ecol. Evol. 9:731626. doi: 10.3389/fevo.2021.731626
Received: 27 June 2021; Accepted: 24 August 2021;
Published: 23 September 2021.
Edited by:
Isabel Marques, University of Lisbon, PortugalReviewed by:
Yuval Sapir, Tel Aviv University, IsraelSteven Johnson, University of KwaZulu-Natal, South Africa
Copyright © 2021 van der Kooi. This is an open-access article distributed under the terms of the Creative Commons Attribution License (CC BY). The use, distribution or reproduction in other forums is permitted, provided the original author(s) and the copyright owner(s) are credited and that the original publication in this journal is cited, in accordance with accepted academic practice. No use, distribution or reproduction is permitted which does not comply with these terms.
*Correspondence: Casper J. van der Kooi, Qy5KLnZhbi5kZXIuS29vaUBydWcubmw=; orcid.org/0000-0003-0613-7633