Neontological and paleontological congruence in the evolution of Podocarpaceae (coniferales) reproductive morphology
- 1Department of Geological Sciences, Stanford University, Stanford, CA, United States
- 2Department of Earth Sciences, University of Oxford, Oxford, United Kingdom
Introduction: Podocarpaceae are a diverse, primarily tropical conifer family that commonly produce large leaves and highly reduced, fleshy seed cones bearing large seeds. These features may result from relatively recent adaptation to closed-canopy angiosperm forests and bird-mediated seed dispersal, although determining precisely when shifts in leaf and seed cone morphology occurred is difficult due to a sparse fossil record and relatively few surviving deep lineages.
Methods: We compare the fossil record of Podocarpaceae with results from ancestral state reconstruction methods and correlated character models using neontological data and a previously published molecular time-tree.
Results: Ancestral state reconstructions suggest that small leaves, small seeds, and multi-seeded cones are ancestral in crown Podocarpaceae, with reduced cones bearing few seeds appearing in the Early Cretaceous and the correlated evolution of large leaves and large seeds occurring from the Late Cretaceous onwards. The exact timing of these shifts based on neontological data alone are poorly constrained, however, and estimates of leaf and seed size are imprecise.
Discussion: The fossil record is largely congruent with results based on the molecular time-tree, but provide important constraints on the range of leaf and seed sizes that were present in Cretaceous Podocarpaceae and the time by which changes in cone morphology and seed size likely occurred. We suggest in particular that reduced seed cones appeared in the Early Cretaceous and are linked to the contemporaneous diversification of small bodied avialans (birds), with shifts to larger seed sizes occurring after the Cretaceous in association with the spread of closed-canopy angiosperm forests.
Introduction
Molecular phylogenies are crucial tools for studying major patterns in plant evolution, thanks to the tremendous growth in sequence data over the past several decades and increasingly powerful ways to analyze it. Although molecular phylogenies necessarily include only living (or subfossil) plants, they are nevertheless widely used to understand much deeper evolutionary processes through statistical methods that estimate divergence ages among extant clades (Sanderson, 2003; Drummond and Rambaut, 2007), reconstruct ancestral states (Cunningham et al., 1998; Pagel et al., 2004; Revell, 2014), and detect changes in diversification rate through time (Morlon, 2014; Rabosky et al., 2014; Condamine et al., 2019). Fossils are a crucial component of these analyses, as they provide the means to calibrate divergence times (see Parham et al., 2012) and can improve the accuracy of ancestral state reconstructions (Slater et al., 2012). The importance of fossil data has driven the development of increasingly sophisticated methods for incorporating them into molecular analyses (e.g., Slater et al., 2012; Heath et al., 2014; Zhang et al., 2016; O’Reilly and Donoghue, 2020). Nevertheless, the ages of deep divergences inferred from molecular clock analyses often differ from those expected based on the fossil record (Smith et al., 2010; Wilf and Escapa, 2015; Herendeen et al., 2017; Morris et al., 2018; Sauquet et al., 2022) and molecular phylogenies can give rise to unexpected or ambiguous ancestral state estimates (e.g., Harris et al., 2020). Recent studies also suggest that the ability of molecular phylogenies to unambiguously record past diversification histories may be inherently limited (Louca and Pennell, 2020). Reconciling results based on molecular phylogenies with the fossil record therefore remains an ongoing challenge for evolutionary biology.
Conifers, the most species-rich group of extant non-flowering seed plants, are a useful system in which to evaluate congruence between the fossil record and molecular analyses because they combine a well-resolved extant phylogeny with a temporally extensive fossil record (Leslie et al., 2018). Although most extant conifer species likely diverged relatively recently (Leslie et al., 2012), the earliest probable stem conifers date back ∼310 million years (Scott and Chaloner, 1983) and crown conifer clades have been present since at least the Early Jurassic (∼200 million years ago; Axsmith et al., 2008; Contreras et al., 2019). Because crown conifer clades were a dominant component of many Mesozoic terrestrial ecosystems, the rich fossil record of their leaves, cones, and seeds provides many opportunities to evaluate divergence ages, ancestral state reconstructions, and diversification shifts inferred from extant phylogenies. In this study, we focus on one major clade of living conifers, the Podocarpaceae, and explore congruence between the fossil record and reconstructions of trait evolution based on a time-calibrated molecular phylogeny.
The Podocarpaceae are the second most speciose major clade among extant conifers, with ∼180 species (Farjon, 2010). The clade has a deep evolutionary history; widely acknowledged fossil representatives of the group occur in the Jurassic and Cretaceous (Harris, 1979; Hill and Brodribb, 1999; Taylor et al., 2009) with possible stem members as far back as the Late Permian (Blomenkemper et al., 2018). Among living conifers, the clade is distinctive for its primarily tropical distribution (Cernusak et al., 2011), the occurrence of large, flattened needle leaves in many taxa (Biffin et al., 2012), and small fleshy reproductive structures specialized for seed dispersal by animals (Contreras et al., 2017; Leslie et al., 2017), particularly birds (Geldenhuys, 1993; Willson et al., 1996; Klaus and Matzke, 2020). Despite the age of the clade, these distinctive leaf and reproductive traits may reflect adaptations to relatively recent ecological conditions associated with the Cenozoic: large, broad leaves for more efficient light reception in closed-canopy, angiosperm-dominated forests (Brodribb and Feild, 2008; Biffin et al., 2012) and reduced, fleshy seed cones that link seed dispersal with crown bird groups radiating over the last 65 million years (Leslie et al., 2017; Klaus and Matzke, 2020). Several Podocarpaceae lineages have also evolved notably large seeds (Leslie et al., 2017), a further possible adaptation to angiosperm-dominated forests where closed canopies favor substantial nutritional reserves for seedlings growing in low light (Tiffney, 1984; Eriksson et al., 2000; Eriksson, 2016). These hypotheses remain incompletely tested, however, and the exact evolutionary history of leaf and reproductive traits in Podocarpaceae is uncertain due to long phylogenetic branches and a limited fossil record.
Podocarpaceae have been the focus of many molecular phylogenetic studies (e.g., Kelch, 1998; Biffin et al., 2012; Knopf et al., 2012; Quiroga et al., 2016; Chen et al., 2022) which have generally split the group into a large clade of species-rich, primarily tropical taxa (e.g., Dacrydium, Dacrycarpus, Podocarpus) sister to a more depauperate, primarily temperate “prumnopityoid” clade (Chen et al., 2022; Figure 2A), and both clades contain long-branched lineages often regarded as relictual (e.g., Lagarostrobus, Microstrobus, and Saxegothaea; Khan and Hill, 2021). However, most extant Podocarpaceae richness is inferred by dated phylogenies to be relatively recent, with species divergences concentrated in the Neogene (Leslie et al., 2012, 2018). The number of lineages that span the deeper Jurassic and Cretaceous history of the group is therefore limited, although previous studies have nevertheless been able to use dated phylogenies to link the evolution of large leaves with the appearance of closed-canopy tropical angiosperm forests (Biffin et al., 2012). Using neontological data and molecular phylogenies to understand the deep evolution of reproductive traits is challenging, however; Podocarpaceae appear to have repeatedly evolved simplified, fleshy reproductive structures whose specific morphology and anatomy differ in detail (Contreras et al., 2017; Khan and Hill, 2021, Khan et al., 2022). With such disparate morphologies on long phylogenetic branches, the exact sequence of evolutionary events leading to Podocarpaceae reproductive diversity is unclear.
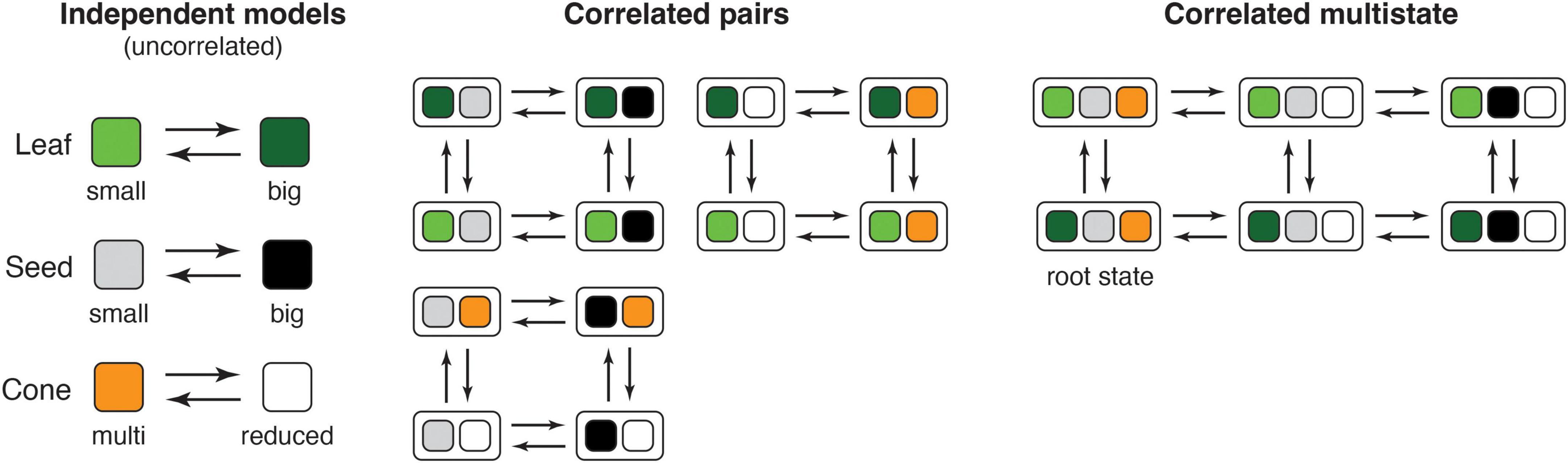
Figure 1. Character models used in this study. Independent models treat each of the three characters (leaf size, seed size, and cone type) separately and fit transition rates to and from character states (arrows). We assessed correlated evolution of character pairs by fitting models that combine states from two characters (leaf size + seed size, leaf size + cone type, and seed size + cone type), and among all three characters (“correlated multistate”) by fitting models that encompassed all known state combinations (eight are possible but only the six shown occur in living taxa). Model fit and support were assessed by summing likelihoods from the independent models and comparing those to the relevant correlated models.
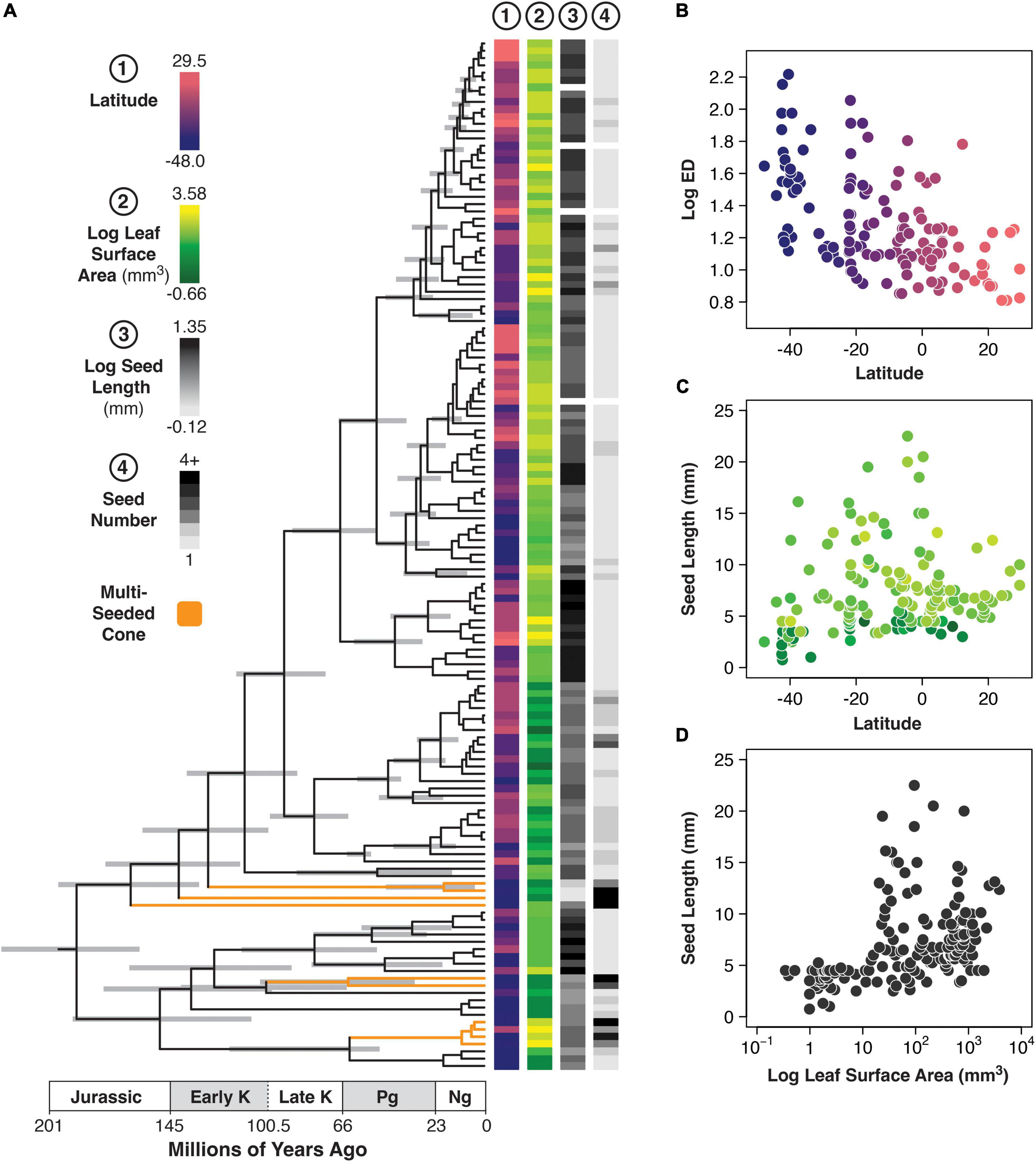
Figure 2. Phylogenetic distribution and relationships of selected Podocarpaceae traits. (A) Time-calibrated molecular phylogeny of extant Podocarpaceae plotted with midpoint species latitude (1), log10-transformed estimated leaf area (2), log10-transformed seed length (3), and seed number per reproductive structure (4). Taxa lacking information for seed length and number are shown as white breaks in the data column. Phylogeny is taken from Leslie et al. (2018) and errors on estimated divergence ages [95% height posterior distributions (HPD)] are shown as gray bars. (B) Relationship between species latitude and log10-transformed evolutionary distinctiveness (ED); points are color coded by latitude as in panel (A) to facilitate comparison with the phylogeny. (C) Relationship between species latitude and seed length; points are color coded by leaf area as in panel (A). (D) Relationship between log10-transformed leaf area and seed length.
Podocarpaceae fossils provide a direct view of past leaf and reproductive morphologies, but their record is sparse compared to other species-rich conifer clades like Cupressaceae and Pinaceae (Hill and Brodribb, 1999; Leslie et al., 2018). Widely acknowledged Mesozoic Podocarpaceae with preserved reproductive structures are restricted to a few representatives from the Jurassic (Harris, 1979; Reymanowna, 1987) and a handful of taxa from the Early Cretaceous of Antarctica (Cantrill and Falcon-Lang, 2001), Argentina (Archangelsky, 1966; Archangelsky and Del Fueyo, 1989), and India (Vishnu-Mittre, 1959; Banerji and Ghosh, 2006). None of these taxa have been directly linked to modern Podocarpaceae clades, either qualitatively or through an explicit phylogenetic analysis, and fossils unambiguously belonging to extant genera appear only in the early Cenozoic (Greenwood, 1987; Hill and Brodribb, 1999; Wilf, 2012; Andruchow-Colombo et al., 2019a,b) or the latest Cretaceous (Wilf et al., 2017). Podocarpaceae leaves are more common in the fossil record than reproductive structures, but older examples are difficult to unambiguously interpret. For example, large multi-veined fossil leaves are often assigned to the Podocarpaceae because some living members of the group (e.g., Nageia) produce them, but detailed whole plant reconstructions of fossil taxa suggest that at least some of them belong to unrelated conifer clades (e.g., the Triassic conifer leaf Heidiphyllum and seed cone Telemachus; Escapa et al., 2010; Bomfleur et al., 2013). Overall, both the Podocarpaceae fossil record and inferences from molecular trees suggest a deep Mesozoic history for the group, but neither source of information provides a detailed understanding of leaf and seed cone evolution and thus the extent to which changes in these traits relate to ecological shifts in the Cretaceous and Cenozoic.
Here we compare the fossil record of Podocarpaceae reproductive structures and their associated leaves to ancestral state reconstructions from a molecular phylogeny to ask if combining these two sources of evolutionary information generates a clearer picture of trait evolution in the group. Although Podocarpaceae fossils and reconstructions based on the molecular phylogeny are both sparse sources of data, we find that they are nonetheless consistent and complementary: both suggest that shifts to highly reduced seed cones bearing few seeds began in the Early Cretaceous and pre-date the ecological dominance of angiosperms, while the evolution of large leaves and seeds is correlated and begins in the Late Cretaceous through the early Cenozoic. The suite of traits that makes Podocarpaceae distinctive among living conifers (large leaves, reduced seed cones, and large seeds) therefore appears to have evolved in a stepwise manner over a prolonged period of the Cretaceous. We use vertebrate fossil data to further suggest that these changes began due to interactions with small-bodied stem avialans (birds), which also underwent an important diversification event in the Early Cretaceous (O’Connor et al., 2011; Benson and Choiniere, 2013; Yu et al., 2021).
Materials and methods
We compiled leaf size measurements from 177 extant Podocarpaceae species and seed length measurements from 163 extant species based on data from Farjon (2010). Where ranges in leaf length and width were provided, we used midpoint values. In taxa with strongly dimorphic foliage (e.g., some Dacrycarpus species), we used measurements from the largest leaves. For Phyllocladus, we treated the phylloclades as “leaves”, given that they are the photosynthetic units of the plant. For all taxa, we estimated leaf (or phylloclade) area as an ellipse with major and minor axes corresponding to provided length and width measurements, respectively. Seeds are difficult to measure in some Podocarpaceae genera [e.g., Podocarpus, Prumnopitys sensu lato (Pectinopitys, Prumnopitys, Sundacarpus)] because they are enveloped by an organ called the epimatium that creates a combined diaspore larger than the seed itself; seed size is only directly accessible if the diaspore is broken or sectioned. To be consistent across all Podocarpaceae, including taxa that lack an enclosing epimatium, we analyzed the true length of the seed. For taxa where only total diaspore length was provided, we estimated seed length using a scaling factor based on comparing diaspore to seed length in the subset of taxa for which both measurements were given in Farjon (2010). This subset includes 27 species from Afrocarpus, Podocarpus, Retrophyllum, and Sundacarpus. On average, seed length in these taxa was 75% of total diaspore length (median 75%, mean 75.1%, interquartile range 70.4–80.9%). For taxa with only diaspore length given (93 species in total), we therefore estimated seed length as 75% of diaspore length.
In addition to continuous leaf and seed size characters, we analyzed several discrete or binned characters. We binned leaf size into “small” and “large” states based on a threshold value of 10 mm2; this represents a natural break in the distribution of estimated leaf areas for extant taxa (see Figure 3A) and generally corresponds to a distinction between taxa with imbricated scale leaves and those with larger needles. We also binned seed length, although here there was no clear break in the distribution of sizes. We used three schemes to assess the potential effects of binning: we divided seed length into “small” and “large” states based on whether length was greater or less than the median value (50th percentile) of extant taxa (6 mm; see Figure 3B), the lower 25th percentile (4.5 mm), or the lower 10th percentile values (3.375 mm; Supplementary Figure 2). Lastly, we scored the typical number of fertile (potentially seed-producing) units produced in Podocarpaceae reproductive structures, using the average or midpoint value when a range was given (Molloy, 1995; Farjon, 2010). We then used these data to score whether Podocarpaceae taxa produce “multi-seeded cones” or “reduced” cones; multi-seeded cones are defined here as having three or more fertile scales (or sterile scales that are morphologically equivalent to fertile scales) arranged in a packed or imbricated configuration. In contrast, many extant Podocarpaceae (e.g., Afrocarpus, Podocarpus, and Prumnopitys sensu lato) produce ovulate structures consisting of just one or two seeds enclosed or subtended by fleshy organs (Contreras et al., 2017; Leslie et al., 2017). Taxa with multi-seeded cones here include Lagarostrobus, Manoao, Microcachrys, Pherosphaera, Phyllocladus, and Saxegothaea. Manoao cones often have only two mature seeds, but they generally produce more fertile scales initially and are known to produce three or more seeds occasionally (Molloy, 1995).
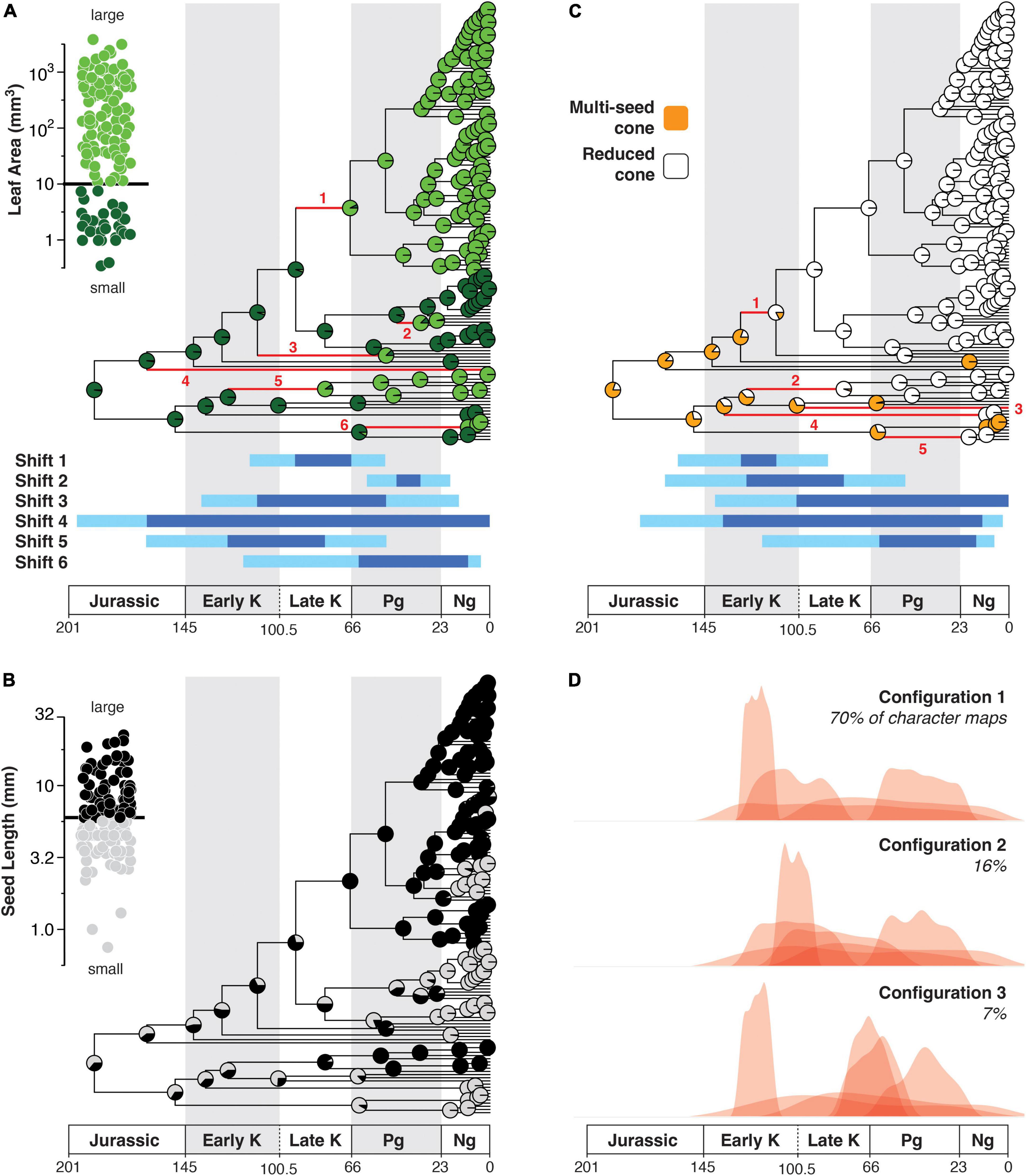
Figure 3. Ancestral state reconstructions of discrete Podocarpaceae characters using a molecular phylogeny. (A) Reconstruction of leaf area as a binary trait, binned into “small” and “large” leaves based on a threshold estimated value of 10 mm2. The full distribution of extant leaf area estimates with the threshold value is shown in the stripchart to the left of the tree. Branches where shifts to large leaf sizes are inferred to have occurred are numbered and highlighted in red. Age ranges for these five shifts are shown under the phylogeny; dark blue indicates the range in median estimated ages for the two nodes between which the shift is inferred to have occurred while light blue indicates the total possible age range (maximum HPD age of oldest node and minimum HPD age of youngest node). (B) Reconstruction of seed length as a binary trait, with the full distribution of seed lengths and the threshold value shown in the stripchart to the left of the tree. Here we show a cutoff value between “small” and “large” at the median seed length (50th percentile; 6 mm). Inferred shifts are ambiguous at deep nodes and are not shown in detail (see main text for discussion). (C) Reconstruction of multi-seeded cones, with inferred shifts to reduced structures numbered and highlighted in red; age ranges for these shifts are shown below the phylogeny as in panel (A). (D) Scaled frequency distributions for the ages of inferred shifts from multi-seeded cones to reduced cones in the three most common character histories (“configurations”) from stochastic character mapping. Configurations and associated frequency distributions are based on 1000 simulated character histories.
To reconstruct the evolution of leaf size, seed size, and the presence of multi-seeded cones based on extant taxa alone, we used phylogenetic analyses of both continuous and discrete/binned characters. We used a time-calibrated phylogeny of extant Podocarpaceae taken from a larger, previously published conifer phylogeny (Leslie et al., 2018) based on three loci (rbcL, matK, 18S). This phylogeny was dated using the Bayesian software BEAST (Drummond and Rambaut, 2007) with 26 conifer calibration fossils, including five from the Podocarpaceae (dating splits of crown Dacrycarpus, stem Dacrydium, stem Phyllocladus, crown Podocarpus, and stem Retrophyllum; see Leslie et al., 2018 for details). The phylogeny includes 141 taxa, from which leaf measurements were available for all and seed measurements from 137. To reconstruct ancestral states for continuous characters (using log10-transformed leaf area and seed length), we used the maximum likelihood-based “fastAnc” function in the R package “phytools” (Revell, 2012); this method provides estimates of values with associated confidence intervals for internal nodes of the tree. To reconstruct ancestral states for discrete/binned characters, we used two likelihood-based approaches that estimate transition rates among character states (e.g., between “small” and “large” leaves) given tree topology and branch lengths, and then used these parameters to estimate character state probabilities for internal nodes within the tree. One approach implemented in the R package “corHMM” (version 2.8; Beaulieu et al., 2021) calculates the marginal likelihood of character states at internal nodes. The other, stochastic character mapping (Huelsenbeck et al., 2003), uses transition rates to simulate character histories. For each character we ran 1000 simulations using the R package “phytools” (Revell, 2012), which were then summed in order to calculate state probabilities at internal nodes and the most likely locations of state shifts along branches. To explore the potential effect of outgroups on reconstructions of deep nodes, we also ran analyses in “corHMM” with a phylogeny that includes three members of the Podocarpaceae sister lineage (the Araucariaceae, including Agathis australis, Araucaria bidwillii, and Wollemia nobilis). These taxa were all scored as having large leaves, large seeds, and multi-seeded cones.
We also used likelihood-based transition rate models implemented in “corHMM” to test whether leaf size, seed size, and cone reduction evolved in a correlated manner (see Pagel, 1994). To test whether characters evolved independently, we fit separate models for binned leaf size (“small” versus “large”), binned seed size (“small” versus “large” using the 50th and 25th percentile cutoffs), and cone type (“multi-seeded” versus “reduced”). For each character, we fit two types of models whose parameters were transition rates to and from each character state: one in which transition rates were constrained to be the same (equal rates; ER) and one in which they were allowed to vary (all rates different; ARD). We then summed the likelihoods of these models and calculated an aggregate AIC score for the ER and ARD models, respectively; this score can be thought of as representing a single evolutionary model with three completely independent (uncorrelated) traits (Figure 1). We then compared these AIC scores to those of models where characters were scored as combined states, implying that they evolve in a correlated fashion and cannot be analyzed separately (Pagel, 1994). We first tested whether pairs of characters evolve in a correlated manner by fitting transition rate models with up to eight parameters (for an ARD model) between possible character state combinations (Figure 1). We then fit full character state models consisting of either five or six combinations of leaf size, seed size, and the presence of multi-seeded cones; five combinations occur in extant Podocarpaceae using the 50% percentile binning scheme and six in the 25% scheme, resulting in either 10 or 14 possible transition rate parameters among the combined states (Figures 1, 4). We also tested versions of these models where the root (ancestral state) was fixed as having small leaves, small seeds, and multi-seeded cones. For all these analyses, we included only taxa that were sampled in the phylogeny and for which leaf size, seed size, and cone morphology were known (137 taxa). All statistical analyses were performed using R version 4.1.3 (R Core Team, 2022).
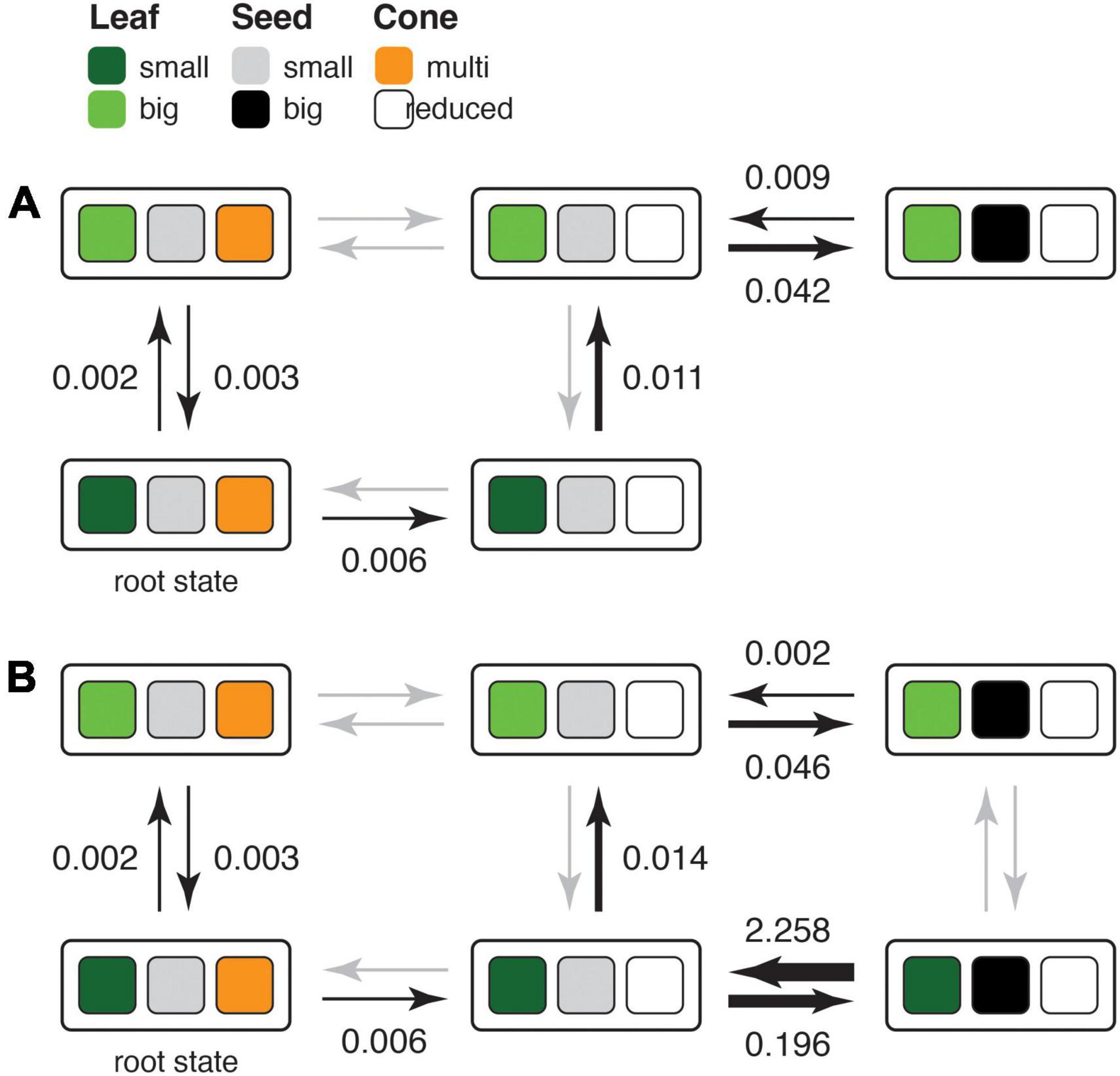
Figure 4. Illustration of the best supported models of character evolution for leaf area, seed length, and multi-seeded cones. (A) Best supported model using median (50th percentile) seed length as the cutoff for “large” seeds. (B) Best supported model using 25th percentile as the cutoff. Rectangles represent state combinations for the three characters, with arrows between them representing possible transition rates. Gray arrows indicate transition rates are inferred to be zero; arrow thickness is proportional to rate order of magnitude. The best supported models (see Table 1) had a fixed root state with small leaves, small seeds, and multi-seeded cones.
To place extant leaf and seed sizes in a broader macroecological and macroevolutionary context, we compiled selected data from living Podocarpaceae species generated by several previous studies of conifers (Sundaram et al., 2019; Sundaram and Leslie, 2021). Specifically, these data include midpoint species latitude and several climatic variables that could potentially influence the evolution of leaf and seed size: mean annual temperature, temperature seasonality, annual precipitation, and precipitation seasonality. Climatic variables were derived from WorldClim bioclimatic variables (BIO1,4,12, and15, respectively; Fick and Hijmans, 2017) and calculated for a given taxon as the average value for each variable across all 100 × 100 km grid cells in which the taxon occurs. We also analyzed evolutionary distinctiveness (ED) for each extant taxon; this is based on the “fair proportion” metric where ED is the sum of branch lengths from root to tip weighted by the inverse of the number of taxa sharing each portion of branch length (Isaac et al., 2007; Jetz et al., 2014). Values used here are based on the full podocarp phylogeny of 141 taxa and taken from Sundaram et al. (2019). We used phylogenetic least squares (PGLS) regression to analyze potential relationships among latitude, climatic variables, ED, and leaf/reproductive characters; our analyses were conducted with scripts described in Leslie et al. (2014) that incorporate phylogenetic covariance into regression calculations.
To compare ancestral state reconstructions based on the extant Podocarpaceae phylogeny with fossil data, we compiled leaf and seed size measurements from published studies of fossil taxa (see Supplementary Appendix I for sources). We included leaf measurements from shoots that were either in organic connection with fossil reproductive structures or were interpreted to belong to the same plant based on strong and consistent association; we did not compile a general database of possible fossil Podocarpaceae leaves due to uncertainty about whether all such leaves belong to the group. We focused on Mesozoic seed and leaf sizes for this study, as Cenozoic Podocarpaceae reproductive structures are rare and similar to extant genera where known (Hill and Carpenter, 1991; Wilf, 2012). We also compared the timing of shifts in Podocarpaceae reproductive structures to the avian fossil record using a compilation of avian body sizes spanning the Late Jurassic through to the recent. This compilation was assembled by combining body mass estimates for Mesozoic birds from Benson et al. (2014) and Benson et al. (2018) with measurements of Cenozoic and extant birds from Mitchell (2015). Body masses of Cenozoic birds were estimated using a linear model resulting from regression of log10 body mass on log10 femoral length in extant birds, using phylogenetic regression (Grafen, 1989) implemented in the R packages nlme 3.1–159 (Pinheiro et al., 2022) and ape version 5.6.2 (Paradis and Schliep, 2019). We note that most data on extinct birds comes from a handful of exceptional fossil-bearing deposits (e.g., Mayr, 2009; Zhou, 2014), and so does not represent a continuously-sampled time series. Nevertheless, these data allowed us to constrain the timing of the appearance and diversification of small-bodied stem or crown birds (Avialae). Small-bodied birds are involved in podocarp seed dispersal today (Geldenhuys, 1993; Willson et al., 1996) and have the potential to have done so since their early evolutionary history (Tiffney, 2004; Hu et al., 2022).
Results
Leaf area, seed length, and cone morphology are variable across extant Podocarpaceae (Figure 2A). Deep-branching, species-poor lineages (i.e., those with high ED) tend to occur at higher southern latitudes (Figure 2B; β = –0.001, R2 = 0.04, p = 0.01 using PGLS with a Brownian motion model of phylogenetic trait covariance) and often produce multi-seeded cones (Figure 2A). Temperate taxa superficially appear to produce smaller seeds and leaves compared to tropical ones (Figure 2C), but these relationships are not statistically significant using PGLS (β = –0.012, R2 = 0.005, p = 0.40 and β = –2.23, R2 = 0.003, p = 0.49 for seed length and leaf area regressed on latitude, respectively). Small seeds are associated with small leaves in extant Podocarpaceae (Figure 2D; β = 1.74, R2 = 0.12, p = < 0.001 using PGLS regression of seed length on log10-transformed leaf area; also significant if using untransformed leaf area) although the relationship is not linear; large seeds occur only in taxa with relatively large leaves while small seeds occur in large-leaved taxa as well. Of the climate variables that we included for analysis, only precipitation seasonality (BIO15) was significantly correlated with seed length using a PGLS regression (p < 0.001), where precipitation seasonality was positively associated with larger seeds, albeit weakly (β = 0.042, R2 = 0.10).
Ancestral state reconstructions based on neontological data suggest that small leaves, small seeds, and multi-seeded cones were ancestral in crown Podocarpaceae with varying degrees of uncertainty (Figure 3; Supplementary Figures 1, 2). Including an outgroup in the analysis does not appreciably change ancestral state reconstructions for deep nodes in crown Podocarpaceae (Supplementary Figure 1). Reconstructions of binned leaf area strongly support small leaves as ancestral (Figure 3A; Supplementary Figure 1A) with six shifts to larger leaves: on the lineage leading to the Podocarpus+Retrophyllum+Afrocarpus+Nageia clade (1), along stem Falcatifolium (2), along stem Acmopyle (3), along the lineage leading to Saxegothaea (4), along stem Prumnopitys sensu lato (the Pectinopitys+Prumnopitys+Sundacarpus; 5), and along stem Phyllocladus (6). Dating these shifts is imprecise due to wide age uncertainties [given as 95% height posterior density (HPD)] on nodes in the dated phylogeny; we show the range in median estimated ages for the two nodes along which each shift in leaf size was inferred to have occurred and the full age uncertainty associated with these pairs of nodes (i.e., the maximum HPD age for the older node and the minimum HPD age for the younger node; Figure 3A). Some of these estimated dates are uninformative; for example, the shift to larger leaves in extant monotypic Saxegothaea could have occurred at any point between the Jurassic and the present (Figure 3A; shift 4). But potential age distributions for more informative shifts are centered in the Late Cretaceous and Cenozoic.
In contrast to leaf size, the ancestral state for binned seed size is uncertain, with nearly equal probabilities inferred for most deep nodes in the Early Cretaceous if the “small” and “large” distinction is made at the 50th percentile seed length (Figure 3B). Adding to this uncertainty, different binning schemes produce different results; “small”seeds are reconstructed as the ancestral condition with higher probability if the 25th length percentile (4.5 mm) is used as the cutoff (Supplementary Figure 2B). In contrast, a very strict “small” seed cutoff at the 10th percentile (3.375 mm) results in strong support for “large” seeds as ancestral (Supplementary Figure 2C), although many of the “large” seeds here are objectively quite small. Given this ambiguity, we did not attempt to quantify or summarize possible ages for state shifts, although the distribution of “small” and “large” seeds at both the 50th or 25th percentiles across the Podocarpaceae tree does qualitatively mirror shifts inferred for leaf size (Figures 3A,B; Supplementary Figures 2A,B).
Ancestral state reconstruction provides a more robust estimate for multi-seeded cones as ancestral (Figure 3C), with five likely shifts to reduced (1–2 seeded) structures in crown Podocarpaceae: along the lineage leading to the last common ancestor of Acmopyle and the Podocarpus+Dacrydium clade (1), along stem Prumnopitys sensu lato (2), along the lineage leading to Parasitaxus (3), along stem Halocarpus (4), and along stem Lepidothamnus (5). The inferred dates for these shifts are again highly imprecise, but several of them are older than inferred shifts in leaf morphology with age distributions centered around the Early Cretaceous (Figure 3C). Similar results are found by stochastic character mapping, with five shifts to reduced seed cones in the most common inferred pathway (Figure 3D; “Configuration 1”: 70% of simulated histories). A smaller fraction of simulated histories reconstruct six shifts, either separate shifts in Acmopyle and the Podocarpus+Dacrydium clade (“Configuration 2”) or separate shifts in stem Prumnopitys sensu lato (“Configuration 3”). In all common character histories there is a high probability of shifts to reduced seed cones first occurring in the Early Cretaceous, with additional shifts in the Cenozoic (Figure 3D).
Leaf size, seed size, and cone type also appear to evolve in concert, as combined character state models of leaf area, seed length, and cone type were better supported than independent models (Table 1; Supplementary Table 1). Combined models of character pairs (leaf size + seed size, leaf size + cone type, seed size + cone type) are all better supported than independent models for the evolution of these character pairs, regardless of whether a 50th or 25th percentile length cutoff was used to define “small” and “large” seeds (Supplementary Table 1). Among the three-character combined states, an all rates different (ARD) model where small leaves, small seeds, and multi-seeded cones was fixed as the root or ancestral condition was the best supported, regardless of seed size binning (Table 1 and Figure 4). These models suggest the ancestral condition evolved along separate pathways, one path reversibly leading to large-leaved taxa with small, multi-seeded cones (e.g., Saxegothaea) and the other unidirectional path leading to small-leaved taxa with small-seeded, reduced cones (e.g., Dacrydium). This latter state is then inferred to unidirectionally shift to larger leaves, which in turn ultimately gives rise to the combination of large leaves, large seeds, and reduced cones (Figure 4A) that is most abundant among extant Podocarpaceae (e.g., Podocarpus). Results are generally similar for a 25th percentile seed size cutoff, although here shifts to and from an additional combined state occur (Figure 4B).
For continuous measurements of leaf area and seed length, ancestral state reconstruction is largely uninformative for nodes with Mesozoic estimated divergence times (Figure 5). For these nodes, leaf area is reconstructed as intermediate between that of extant “small” and “large” leaves, with median values close to our binning threshold size of 10 mm2 (Figure 5A). These estimates are associated with extremely wide confidence intervals, however, spanning nearly the entire range of extant leaf sizes. Leaves from Mesozoic fossil Podocarpaceae are considerably smaller than reconstructed median values and similar in size to extant taxa that produce small, imbricated scale leaves (e.g., Dacrydium, Halocarpus, and Lepidothamnus), apart from one taxon (Mataia) whose Podocarpaceae affinities are not definitive given its seed cone (Figure 5A). Confidence intervals on estimated seed lengths for Mesozoic nodes are likewise wide (Figure 5B), and known fossil seeds are considerably smaller than reconstructed median values for contemporaneous nodes in the molecular tree (Figure 5B). The total number of fertile scales (for fossils this was assessed as the total number of seeds) produced per reproductive structure changes through time (Figure 5B), with highly reduced cones producing 1–3 seeds appearing by around 120 Ma. Although the Cenozoic record of Podocarpaceae reproductive structures is extremely sparse, available representatives (Dacrydium puertae, Podocarpus witherdenensis) are nearly identical in morphology to extant taxa and fall within typical modern seed sizes (Figure 4B).
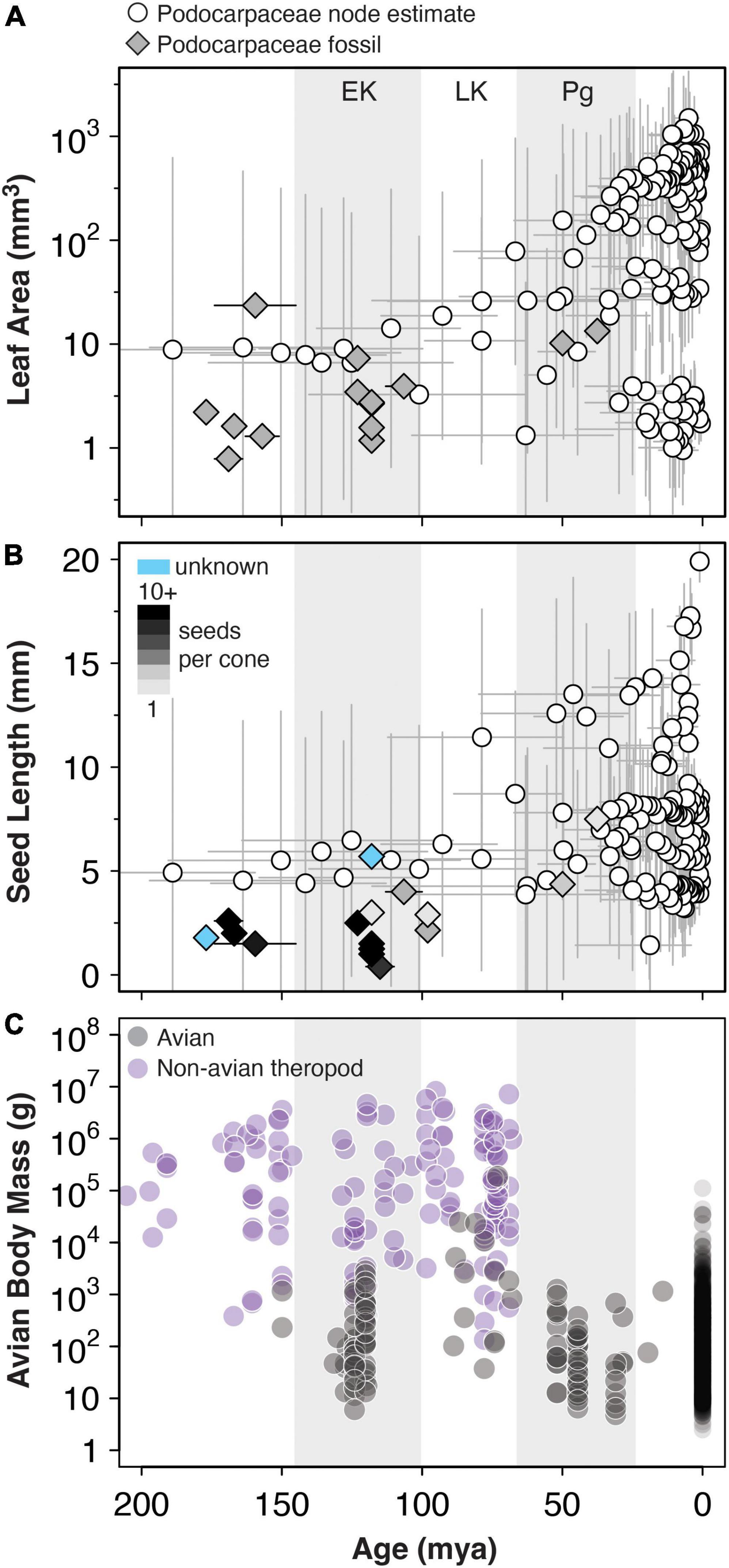
Figure 5. Ancestral state reconstructions and fossil evidence of continuous traits through time. (A) Estimates of leaf area from fossils (diamonds) and ancestral state reconstructions of internal nodes in a molecular phylogeny based on neontological data (white circles). Node age is shown as the median estimated divergence age. (B) Estimates of seed length from fossil (diamonds) and ancestral state reconstructions using the molecular phylogeny (white circles). Fossil icons are colored according to average number of seeds per reproductive structure; fragmentary structures where total seed number is unknown are indicated by blue. For (A,B), 95% confidence intervals on node estimates are shown by gray bars. (C) Avian (gray circles) and non-avian theropod (purple circles) body sizes from the Late Jurassic to the recent; data from Benson et al. (2014, 2018).
Body mass data show that small-bodied birds weighing less than 100 grams, reflecting the modal body size of extant birds (∼30 grams; e.g. Maurer, 1998), first appeared by the mid Early Cretaceous, approximately 125 million years ago (Figure 5C). These body masses are much smaller than any other theropod dinosaurs (which have minimum adult body masses of several hundred grams) and are much more similar to body masses of extant and early Cenozoic birds (Figure 5C). It is also noteworthy that the initial appearance of extremely small birds closely matches the initial appearance of reduced Podocarpaceae seed cones (Figure 5B).
Discussion
Congruence between molecular analyses and the fossil record
Both the phylogenetic structure and the fossil record of Podocarpaceae provide information about the evolutionary history of the group. Both have important limitations, however: ancestral state reconstructions of deep nodes and estimated ages of character transitions based on molecular phylogenies are imprecise, while the fossil record is poorly sampled and the phylogenetic affinities of most fossils are unknown. Nevertheless, both data sources tell a congruent story for reproductive evolution in the group: the molecular phylogeny suggests the ancestral state was multi-seeded cones bearing small seeds, consistent with the morphology of Jurassic and many Cretaceous fossil taxa (Vishnu-Mittre, 1959; Harris, 1979). Ancestral state reconstructions and transition rate models suggest that Podocarpaceae clades first evolved reduced cones with one or two seeds in the Early Cretaceous, and that seeds in these lineages remained relatively small. This inference is consistent with the fossil record, where reduced podocarp cones with one or two seeds first appeared as fossils during the middle of the Early Cretaceous, around 120 Ma (Figure 5B). Although confidence intervals are extremely wide for reconstructions of specific leaf and seed sizes, mean estimates for Jurassic and Early Cretaceous nodes are not radically different from fossil data, particularly for seeds (Figures 5A,B). Comparing the Late Cretaceous and Cenozoic history of the group is more challenging given the lack of reproductive fossils (Figure 5B), but the few that do exist are consistent with patterns inferred from the molecular tree; the largest known podocarp seed in the fossil record comes from the Late Eocene and was tentatively placed in crown Podocarpus (Hill and Carpenter, 1991), which is inferred by these molecular analyses to have been diversifying around this time.
Whether the temporal congruence between our inferences from molecular phylogenies and the fossil record is coincidental is difficult to assess; a single new Jurassic Podocarpaceae fossil with a reduced seed cone would complicate the concordance between molecular reconstructions and the fossil record with regards to seed number, as would molecular trees with different estimated divergence ages. Indeed, inferred Podocarpaceae divergence dates do vary considerably among studies; several (Quiroga et al., 2016; Chen et al., 2022) estimate older ages than our analysis while others estimate younger dates (Biffin et al., 2012; Klaus and Matzke, 2020). In these studies, the earliest shifts to reduced cones (corresponding to shifts 1 and 2 in Figure 3C) would have most likely occurred either between the Early to Late Jurassic (older inferred ages) or the middle to Late Cretaceous (younger ages). Taken at face value, the fossil record suggests that Jurassic splits between crown genera (e.g., Quiroga et al., 2016; Chen et al., 2022) are too old; with the exception of an undescribed possible Lepidothamnus from the mid-Cretaceous (Khan et al., 2022), the first fossils unambiguously assigned to extant genera appear only in the latest Cretaceous to Paleogene (Hill and Brodribb, 1999; Wilf, 2012; Wilf et al., 2017). On the other hand, the phylogenetic position of Jurassic and Cretaceous Podocarpaceae (e.g., Vishnu-Mittre, 1959; Archangelsky and Del Fueyo, 1989) is unresolved; they could in theory represent stem members of extant genera and it could then be argued that the Mesozoic fossil record is simply uninformative as to when deep divergences among extant clades and genera occurred. Determining the timing of major splits within crown Podocarpaceae therefore remains an ongoing challenge in understanding their evolution.
But beyond challenges with dating, the fossil record is congruent with reconstructions based on the molecular phylogeny regarding the sequence of character state transitions in Podocarpaceae cone evolution, from initially multi-seeded cones with small seeds to reduced cones with large seeds. From the molecular perspective, this sequence of events is based primarily on topology, which varies less among the phylogenetic studies. Small multi-seeded cones are also the most likely ancestral state combination from a fossil perspective, as all Mesozoic fossil taxa produce small seeds and multi-seeded cones are undoubtedly more common among early taxa. A general taphonomic bias against the preservation of small, reduced seed cones is likely because they are fragile, but such structures nonetheless do occur in the fossil record and there is no reason to expect that this bias would suddenly change halfway through the Early Cretaceous to favor the preservation of reduced cones, which are the only type known in the subsequent fossil record (Figure 5B). The fossil record then suggests that extant Podocarpaceae clades do accurately record aspects of the Cretaceous reproductive evolution of the group, with deep divergences among living lineages sampling major reproductive character state changes occurring in the Mesozoic, especially among currently depauperate lineages that appear to show long-term stasis in reproductive morphology.
Seed cones, birds, and forests in Podocarpaceae reproductive evolution
If the fossil record and our molecular reconstructions tell a congruent story of Cretaceous reproductive evolution in Podocarpaceae, what factors might have favored a shift toward reduced cones, and later, larger seeds? Our findings raise the possibility that the diversification of stem-birds acted as an initial driver of reproductive changes in the Early Cretaceous. Birds are regarded as the primary seed dispersal agents for Podocarpaceae today (e.g., Geldenhuys, 1993; Willson et al., 1996; Klaus and Matzke, 2020) and there is a general correlation between the size of plant reproductive structures and the size of vertebrates that consume them (Wheelwright, 1985; Burns, 2013; Chen and Moles, 2015). The diversification of a new group of small vertebrates with the potential for frugivory should then be highly relevant for Podocarpaceae cone size evolution. We suggest in particular that early birds favored the evolution of reduced cones, consisting of a few seeds and adhering fleshy tissues, that could function as small, easily ingestible disseminules. The reduction in seed number may have been favored in order to maintain relatively small disseminule sizes regardless of seed size (Leslie et al., 2017). For example, multi-seeded cones that can be ingested whole by small animals such as birds will necessarily contain very small seeds (e.g., extant Microcachrys). Evolving larger seeds (even if they were still “small” based on the thresholds used in this analysis) while minimizing total disseminule size could be accommodated by reducing the number of seeds per cone, a trade-off that appears across gymnosperms (Leslie et al., 2017). The shift toward fewer seeds per cone in Early Cretaceous Podocarpaceae may then reflect a new selective regime dominated by small-bodied animal dispersal agents like birds.
The timing of this change in cone morphology is consistent with the avian fossil record. The bird crown-group evolved slightly more than 70 million years ago, during the latest Cretaceous (Prum et al., 2015; Field et al., 2020), but was preceded by the origins of highly bird-like stem lineages during the Early Cretaceous (e.g., O’Connor et al., 2011; Zhou, 2014; Benson, 2018). These taxa, belonging to the group Pygostylia and its subclade Ornithoraces (which includes the diverse Mesozoic group Enantiornithes) had high species richness from shortly after their origin (Benson, 2018). They also possessed many bird-like flight-related and dietary features (O’Connor et al., 2011; O’Connor, 2019; Miller and Pittman, 2021) as well as small body sizes similar to those of extant birds (Figure 5C; Benson et al., 2018). Pygostylians were therefore equivalent to extant birds in at least some important aspects of their ecology and diversity, and unlike any previous groups of non-avian dinosaurs. Moreover, several Early Cretaceous stem-birds include seeds in their fossilized gut contents (Zhou and Zhang, 2002; Zheng et al., 2011, 2018). The affinity of these seeds is unknown, but there is strong evidence in at least one taxon (Hu et al., 2022; see also Ksepka et al., 2019; Mayr et al., 2020) that their presence reflects frugivory and mutualism rather than seed predation. Together, the observations above are consistent with the potential for bird-plant mutalisms as an important driver of plant evolution from the Early Cretaceous onwards (and see Tiffney, 2004).
Klaus and Matzke (2020) likewise suggested that bird diversification in the Cretaceous drove the evolution of seed cone morphology in Podocarpaceae, although they focused on fleshiness rather than seed number. We found that the evolution of fleshiness was more difficult to reconstruct and interpret than seed number. If treated as a single trait, fleshiness was the likely ancestral state for crown Podocarpaceae (results not shown; see also Herting et al., 2020; Khan et al., 2022). But specific fleshy tissues across Podocarpaceae are variable (including epimatia, arils, and different bracts; see Contreras et al., 2017; Khan et al., 2022), suggesting either that the ability to produce fleshy cone tissues evolved once and has been expressed in many organs around the cone or that fleshiness evolved independently many times. Podocarpaceae cones that were apparently fleshy do occur throughout the fossil record, including Jurassic Scarburgia and Harrisocarpus (Harris, 1979; Reymanowna, 1987) and Cretaceous Trisaccocladus and Bellingshausium (Archangelsky, 1966; Cantrill and Falcon-Lang, 2001), but these taxa have not been placed phylogenetically and therefore shed little light on possible ancestral states. Nevertheless, these fossils suggest that fleshiness was a feature of podocarp reproductive structures prior to the reduction in seed number. To the extent that fleshiness is correlated with biotic seed dispersal (but see Fountain et al., 1989 for an alternative explanation), Podocarpaceae may have utilized animals prior to the diversification of stem birds, but the rapid radiation of a new lineage of small-bodied arboreal taxa would likely have expanded opportunities for animal seed dispersal syndromes and the range of successful dispersal morphologies, and with these the potential for long-distance seed dispersal in the group. The nearly synchronous appearance of small-bodied birds and reduced Podocarpaceae cones (Figures 5B,C) is suggestive that this is the case.
Following the evolution of reduced seed cones, the fossil record is too sparse to pinpoint when a shift toward larger seed sizes occurred. However, a general Paleogene increase in average seed size among plant communities has long been noted (Tiffney, 1984) and would be consistent with molecular-based reconstructions for Podocarpaceae. This increase has been attributed to the spread of closed-canopy angiosperm forests after the K-Pg extinction, which are thought to have favored increased investment in seeds as nutrient reserves for seedlings growing under low light (Eriksson et al., 2000; Eriksson, 2008) and/or promoted the diversification of frugivorous seed dispersers (Wing and Tiffney, 1987). As noted in the Introduction, the spread of Cenozoic angiosperm forests has been proposed as a driver of leaf evolution in Podocarpaceae (Biffin et al., 2012) and may explain the correlation between large leaves and large seeds (Figures 2D, 4); low-light ecosystems that favor large leaves would presumably also favor investment in large seeds. Although such a mechanism is intuitive, extant species do not provide much support for it; Podocarpaceae seed size shows no significant relationship with tropical latitudes (Figure 2C) and is correlated only with high precipitation seasonality, a feature not typically associated with warm and wet early Cenozoic forests. The data used in this study may not be appropriate to tease apart these patterns, however; latitude does not necessarily indicate whether a species lives in a closed-canopy forest and our climatic data is of low resolution in general (species-wide averages of 100 × 100 km grid cells). More finely resolved Podocarpaceae data sets would be needed in order to assess current climatic and ecological correlates of seed size more fully. Furthermore, current species climatic tolerances and dispersal strategies may be far removed from conditions early in the history of modern genera; a focus on reproductive material, seed size, and paleoclimate from Paleogene Podocarpaceae (e.g., Hill and Carpenter, 1991; Wilf, 2012) may therefore provide a more accurate ecological context for understanding their early Cenozoic evolution.
Conclusion
Although molecular and fossil data regarding the deep evolution of Podocarpaceae reproductive structures are sparse, they are complementary. Both suggest that cones, seeds, and leaves evolved as a suite of traits in a stepwise manner in response to changing ecological conditions from the Early Cretaceous through the early Cenozoic. First, cones evolved a reduced number of seeds in conjunction with a major radiation of stem birds in the middle Early Cretaceous. Next, large seeds then evolved in lineages with large leaves, most likely as forest ecosystems were reorganized in the wake of angiosperm dominance from the Late Cretaceous through the early Cenozoic. Given the poorly sampled Podocarpaceae fossil record and nature of inferences made from molecular phylogenies of extant taxa, this apparent congruence in evolutionary history should be taken more as a hypothesis than a complete picture of Podocarpaceae evolution. Further testing this scenario requires better phylogenetic integration of fossil and living Podocarpaceae; incorporating more fossil taxa into total evidence analyses (e.g., fossilized birth-death; Heath et al., 2014) may help to refine estimated divergence ages, while focused phylogenetic studies of fossil Podocarpaceae should help to unravel the polarity of reproductive character shifts within the group.
Data availability statement
The original contributions presented in this study are included in the article/Supplementary material, further inquiries can be directed to the corresponding authors.
Author contributions
AL conceived of the study. AL and RB designed and conducted the analyses, contributed to wrote the manuscript. Both the authors contributed to the article and approved the submitted version.
Acknowledgments
We thank three reviewers for their helpful comments. We also would like to thank Dori Contreras, Julian Herting, and Santiago Ramirez-Barahona for their helpful reviews.
Conflict of interest
The authors declare that the research was conducted in the absence of any commercial or financial relationships that could be construed as a potential conflict of interest.
Publisher’s note
All claims expressed in this article are solely those of the authors and do not necessarily represent those of their affiliated organizations, or those of the publisher, the editors and the reviewers. Any product that may be evaluated in this article, or claim that may be made by its manufacturer, is not guaranteed or endorsed by the publisher.
Supplementary material
The Supplementary Material for this article can be found online at: https://www.frontiersin.org/articles/10.3389/fevo.2022.1058746/full#supplementary-material
References
Andruchow-Colombo, A., Wilf, P., and Escapa, I. H. (2019a). A South American fossil relative of Phyllocladus: huncocladus laubenfelsii gen. et sp. nov. (Podocarpaceae), from the early eocene of Laguna del Hunco, Patagonia, Argentina. Aust. Syst. Bot. 32, 290–309. doi: 10.1071/SB18043
Andruchow-Colombo, A., Escapa, I. H., Carpenter, R. J., Hill, R. S., Iglesias, A., Abarzua, A. M., et al. (2019b). Oldest record of the scale-leaved clade of podocarpaceae, early paleocene of Patagonia, Argentina. Alcheringa 43, 127–145. doi: 10.1080/03115518.2018.1517222
Archangelsky, S. (1966). New gymnosperms from the tico flora, Santa Cruz province, Argentina.-bulletin of the British museum (natural history). Geology 13, 259–295. doi: 10.5962/p.313841
Archangelsky, S., and Del Fueyo, G. (1989). Squamastrobus gen. n., a fertile podocarp from the early cretaceous of Patagonia, Argentina. Rev. Palaeobot. Palynol. 59, 109–126. doi: 10.1016/0034-6667(89)90010-9
Axsmith, B. J., Escapa, I. H., and Huber, P. (2008). An araucarian conifer bract-scale complex from the lower jurassic of Massachusetts: Implications for estimating phylogenetic and stratigraphic congruence in the araucariaceae. Palaeontol. Electron. 11:9.
Banerji, J., and Ghosh, A. K. (2006). Podospermum gen. et sp. nov., an acmopyle-like dispersed silicified ovule/seed from lower cretaceous intertrappean beds of the rajmahal basin, India. Cretac. Res. 27, 707–711. doi: 10.1016/j.cretres.2006.03.003
Beaulieu, J., O’Meara, B., Oliver, J., and Boyko, J. (2021). CorHMM: Hidden markov models of character evolution. R package version 2.7. Available online at: https://CRAN.R-project.org/package=nlme (accessed January 9, 2022).
Benson, R. B. (2018). Dinosaur macroevolution and macroecology. Ann. Rev. Ecol. Evol. Syst. 49, 379–408. doi: 10.1146/annurev-ecolsys-110617-062231
Benson, R. B. J., and Choiniere, J. N. (2013). Rates of dinosaur limb evolution provide evidence for exceptional radiation in Mesozoic birds. Proc. R. Soc. B 280:20131780. doi: 10.1098/rspb.2013.1780
Benson, R. B. J., Campione, N. E., Carrano, M. T., Mannion, P. D., Sullivan, C., Upchurch, P., et al. (2014). Rates of dinosaur body mass evolution indicate 170 million years of sustained ecological innovation on the avian stem lineage. PLoS Biol. 12:e1001853. doi: 10.1371/journal.pbio.1001853
Benson, R. B. J., Hunt, G., Carrano, M. T., and Campione, N. (2018). Copes’ rule and the adaptive landscape of dinosaur body size evolution. Palaeontology 61, 13–48. doi: 10.1111/pala.12329
Biffin, E., Brodribb, T. J., Hill, R. S., Thomas, P., and Lowe, A. J. (2012). Leaf evolution in Southern Hemisphere conifers tracks the angiosperm ecological radiation. Proc. R. Soc. B 279, 341–348. doi: 10.1098/rspb.2011.0559
Blomenkemper, P., Kerp, H., Abu Hamad, A., DiMichele, W. A., and Bomfleur, B. (2018). A hidden cradle of plant evolution in permian tropical lowlands. Science 362, 1414–1416. doi: 10.1126/science.aau4061
Bomfleur, B., Decombeix, A. L., Escapa, I. H., Schwendemann, A. B., and Axsmith, B. (2013). Whole-plant concept and environment reconstruction of a Telemachus conifer (voltziales) from the triassic of Antarctica. Int. J. Plant Sci. 174, 425–444. doi: 10.1086/668686
Brodribb, T. J., and Feild, T. S. (2008). Evolutionary significance of a flat-leaved Pinus in Vietnamese rainforest. New Phytol. 178, 201–209. doi: 10.1111/j.1469-8137.2007.02338.x
Burns, K. C. (2013). What causes size coupling in fruit–frugivore interaction webs? Ecology 94, 295–300. doi: 10.1890/12-1161.1
Cantrill, D. J., and Falcon-Lang, H. J. (2001). Cretaceous (late albian) coniferales of Alexander Island, Antarctica. 2. Leaves, reproductive structures and roots. Rev. Palaeobot. Palynol. 115, 119–145. doi: 10.1016/S0034-6667(01)00053-7
Cernusak, L. A., Adie, H., Bellingham, P. J., Biffin, E., Brodribb, T. J., Coomes, D. A., et al. (2011). Podocarpaceae in tropical forests: A synthesis. Smithson. Contrib. Bot. 95, 189–202. doi: 10.5479/si.0081024X.95.189
Chen, L., Jin, W. T., Liu, X. Q., and Wang, X. Q. (2022). New insights into the phylogeny and evolution of podocarpaceae inferred from transcriptomic data. Mol. Phylogenet. Evol. 166:107341. doi: 10.1016/j.ympev.2021.107341
Chen, S. C., and Moles, A. T. (2015). A mammoth mouthful? A test of the idea that larger animals ingest larger seeds. Glob. Ecol. Biogeogr. 24, 1269–1280. doi: 10.1111/geb.12346
Condamine, F. L., Rolland, J., and Morlon, H. (2019). Assessing the causes of diversification slowdowns: Temperature-dependent and diversity-dependent models receive equivalent support. Ecol. Lett. 22, 1900–1912. doi: 10.1111/ele.13382
Contreras, D. L., Duijnstee, I. A. P., Ranks, S., Marshall, C. R., and Looy, C. V. (2017). Evolution of dispersal strategies in conifers: Functional divergence and convergence in the morphology of diaspores. Perspect. Plant Ecol. Evol. 24, 93–117. doi: 10.1016/j.ppees.2016.11.002
Contreras, D. L., Escapa, I. H., Iribarren, R. C., and Cúneo, N. R. (2019). Reconstructing the early evolution of the cupressaceae: A whole-plant description of a new Austrohamia species from the Cañadón asfalto formation (early Jurassic), Argentina. Int. J. Plant Sci. 180, 834–868. doi: 10.1086/704831
Cunningham, C. W., Omland, K. E., and Oakley, T. H. (1998). Reconstructing ancestral character states: A critical reappraisal. Trends Ecol. Evol. 13, 361–366. doi: 10.1016/S0169-5347(98)01382-2
Drummond, A. J., and Rambaut, A. (2007). BEAST: Bayesian evolutionary analysis by sampling trees. BMC Evol. Biol. 7:214. doi: 10.1186/1471-2148-7-214
Eriksson, O. (2008). Evolution of seed size and biotic seed dispersal in angiosperms: Paleoecological and neoecological evidence. Int. J. Plant Sci. 169, 863–870. doi: 10.1086/589888
Eriksson, O. (2016). Evolution of angiosperm seed disperser mutualisms: The timing of origins and their consequences for coevolutionary interactions between angiosperms and frugivores. Biol. Rev. 91, 168–186. doi: 10.1111/brv.12164
Eriksson, O., Friis, E. M., and Löfgren, P. (2000). Seed size, fruit size, and dispersal systems in angiosperms from the early cretaceous to the late tertiary. Am. Nat. 156, 47–58. doi: 10.1086/303367
Escapa, I. H., Decombeix, A. L., Taylor, E. L., and Taylor, T. N. (2010). Evolution and relationships of the conifer seed cone telemachus: Evidence from the triassic of Antarctica. Int. J. Plant Sci. 171, 560–573. doi: 10.1086/651948
Fick, S. E., and Hijmans, R. J. (2017). WorldClim 2: New 1km spatial resolution climate surfaces for global land areas. Int. J. Climatol. 37, 4302–4315. doi: 10.1002/joc.5086
Field, D. J., Benito, J., Chen, A., Jagt, J. W. M., and Ksepka, D. T. (2020). Late cretaceous neornithine from Europe illuminates the origins of crown birds. Nature 579, 397–401. doi: 10.1038/s41586-020-2096-0
Fountain, D. W., Holdsworth, J. M., and Outred, H. A. (1989). The dispersal unit of Dacrycarpus dacrydioides (A. Rich.) de laubenfels (podocarpaceae) and the significance of the fleshy receptacle. Bot. J. Linn. Soc. 99, 197–207. doi: 10.1111/j.1095-8339.1989.tb00399.x
Geldenhuys, C. J. (1993). Reproductive biology and population structures of Podocarpus falcatus and P. latifolius in southern cape forests. Bot. J. Linn. Soc. 112, 59–74. doi: 10.1006/bojl.1993.1041
Grafen, A. (1989). The phylogenetic regression. Phil. Trans. R. Soc. B 326, 119–157. doi: 10.1098/rstb.1989.0106
Greenwood, D. R. (1987). Early tertiary podocarpaceae megafossils from the eocene Anglesea locality, Victoria, Australia. Aust. J. Bot. 35, 111–133. doi: 10.1071/BT9870111
Harris, B. J., Harrison, C. J., Hetherington, A. M., and Williams, T. A. (2020). Phylogenomic evidence for the monophyly of bryophytes and the reductive evolution of stomata. Curr. Biol. 11, 2001–2012. doi: 10.1016/j.cub.2020.03.048
Harris, T. M. (1979). The Yorkshire jurassic flora, V. Coniferales. London: British Museum (Natural History).
Heath, T. A., Huelsenbeck, J. P., and Stadler, T. (2014). The fossilized birth–death process for coherent calibration of divergence-time estimates. Proc. Natl. Acad. Sci. U.S.A. 111, E2957–E2966. doi: 10.1073/pnas.1319091111
Herendeen, P. S., Friis, E. M., Pedersen, K. R., and Crane, P. R. (2017). Palaeobotanical redux: Revisiting the age of the angiosperms. Nat. Plants 3, 1–8. doi: 10.1038/nplants.2017.15
Herting, J., Stützel, T., and Klaus, K. V. (2020). The ancestral conifer cone: What did it look like? A modern trait-evolution approach. Int. J. Plant Sci. 181, 871–886. doi: 10.1086/710489
Hill, R. S., and Brodribb, T. J. (1999). Southern conifers in time and space. Aust. J. Bot. 47, 639–696. doi: 10.1071/BT98093
Hill, R. S., and Carpenter, R. J. (1991). Evolution of Acmopyle and Dacrycarpus (podocarpaceae) foliage as inferred from macrofossils in south-eastern Australia. Aust. Syst. Bot. 4, 449–479. doi: 10.1071/SB9910449
Hu, H., Wang, Y., McDonald, P. G., Wroe, S., O’Connor, J. K., Bjarnason, A., et al. (2022). Earliest evidence for frugivory and seed dispersal by birds. Elife 11:e74751. doi: 10.7554/eLife.74751
Huelsenbeck, J. P., Nielsen, R., and Bollback, J. P. (2003). Stochastic mapping of morphological characters. Syst. Biol. 52, 131–158. doi: 10.1080/10635150390192780
Isaac, N. J., Turvey, S. T., Collen, B., Waterman, C., and Baillie, J. E. (2007). Mammals on the EDGE: Conservation priorities based on threat and phylogeny. PLoS One 2:e296. doi: 10.1371/journal.pone.0000296
Jetz, W., Thomas, G. H., Joy, J. B., Redding, D. W., Hartmann, K., and Mooers, A. O. (2014). Global distribution and conservation of evolutionary distinctness in birds. Curr. Biol. 24, 919–930. doi: 10.1016/j.cub.2014.03.011
Kelch, D. G. (1998). Phylogeny of podocarpaceae: Comparison of evidence from morphology and 18S rDNA. Am. J. Bot. 85, 986–996. doi: 10.2307/2446365
Khan, R., and Hill, R. S. (2021). Morpho-anatomical affinities and evolutionary relationships of three paleoendemic podocarp genera based on seed cone traits. Ann. Bot. 128, 887–902. doi: 10.1093/aob/mcab113
Khan, R., Hill, R. S., Dörken, V. M., and Biffin, E. (2022). Detailed seed cone morpho-anatomy of the Prumnopityoid clade: An insight into the origin and evolution of podocarpaceae seed cones. Ann. Bot. 30:mcac097. doi: 10.1093/aob/mcac097
Klaus, K. V., and Matzke, N. J. (2020). Statistical comparison of trait-dependent biogeographical models indicates that podocarpaceae dispersal is influenced by both seed cone traits and geographical distance. Syst. Biol. 69, 61–75. doi: 10.1093/sysbio/syz034
Knopf, P., Schulz, C., Little, D. P., Stützel, T., and Stevenson, D. W. (2012). Relationships within podocarpaceae based on DNA sequence, anatomical, morphological, and biogeographical data. Cladistics 28, 271–299. doi: 10.1111/j.1096-0031.2011.00381.x
Ksepka, D. T., Grande, L., and Mayr, G. (2019). Oldest finch-beaked birds reveal parallel ecological radiations in the earliest evolution of passerines. Curr. Biol. 29, 657–663. doi: 10.1016/j.cub.2018.12.040
Leslie, A. B., Beaulieu, J. M., Crane, P. R., and Donoghue, M. J. (2014). Cone size is related to branching architecture in conifers. New Phytol. 203, 1119–1127. doi: 10.1111/nph.12864
Leslie, A. B., Beaulieu, J. M., Holman, G., Campbell, C. S., Mei, W., Raubeson, L. R., et al. (2018). An overview of extant conifer evolution from the perspective of the fossil record. Am. J. Bot. 105, 1531–1544. doi: 10.1002/ajb2.1143
Leslie, A. B., Beaulieu, J. M., and Mathews, S. (2017). Variation in seed size is structured by dispersal syndrome and cone morphology in conifers and other nonflowering seed plants. New Phytol. 216, 429–437. doi: 10.1111/nph.14456
Leslie, A. B., Beaulieu, J. M., Rai, H. S., Crane, P. R., Donoghue, M. J., and Mathews, S. (2012). Hemisphere-scale differences in conifer evolutionary dynamics. Proc. Natl. Acad. Sci. U.S.A. 40, 16217–16221. doi: 10.1073/pnas.1213621109
Louca, S., and Pennell, M. W. (2020). Extant timetrees are consistent with a myriad of diversification histories. Nature 580, 502–505. doi: 10.1038/s41586-020-2176-1
Maurer, B. A. (1998). The evolution of body size in birds. II. The role of reproductive power. Evol. Ecol. 12, 935–944. doi: 10.1023/A:1006564105504
Mayr, G., Kaye, T. G., Pittman, M., Saitta, E. T., and Pott, C. (2020). Reanalysis of putative ovarian follicles suggests that early cretaceous birds were feeding not breeding. Sci. Rep. 10:19035. doi: 10.1038/s41598-020-76078-2
Miller, C. V., and Pittman, M. (2021). The diet of early birds based on modern and fossil evidence and a new framework for its reconstruction. Biol. Rev. 96, 2058–2112. doi: 10.1111/brv.12743
Mitchell, J. S. (2015). Extant-only comparative methods fail to recover the disparity preserved in the bird fossil record. Evolution 69, 2414–2424. doi: 10.1111/evo.12738
Molloy, B. P. (1995). Manoao (podocarpaceae), a new monotypic conifer genus endemic to New Zealand. N. Z. J. Bot. 33, 183–201. doi: 10.1080/0028825X.1995.10410483
Morlon, H. (2014). Phylogenetic approaches for studying diversification. Ecol. Lett. 17, 508–525. doi: 10.1111/ele.12251
Morris, J. L., Puttick, M. N., Clark, J. W., Edwards, D., Kenrick, P., Pressel, S., et al. (2018). The timescale of early land plant evolution. Proc. Natl. Acad. Sci. U.S.A. 115, E2274–E2283. doi: 10.1073/pnas.1719588115
O’Connor, J. (2019). The trophic habits of early birds. Palaeogeogr. Palaeoclimatol. Palaeoecol. 513, 178–195. doi: 10.1016/j.palaeo.2018.03.006
O’Connor, J. K., Chiappe, L. M., and Bell, A. (2011). “Pre-modern birds: Avian divergences in the mesozoic,” in Living dinosaurs: The evolutionary history of modern birds, eds G. Dyke and G. Kaiser (Oxford: Wiley), 39–114. doi: 10.1002/9781119990475.ch3
O’Reilly, J. E., and Donoghue, P. C. (2020). The effect of fossil sampling on the estimation of divergence times with the fossilized birth–death process. Syst. Biol. 69, 124–138. doi: 10.1093/sysbio/syz037
Pagel, M. (1994). Detecting correlated evolution on phylogenies: A general method for the comparative analysis of discrete characters. Proc. R. Soc. B 255, 37–45. doi: 10.1098/rspb.1994.0006
Pagel, M., Meade, A., and Barker, D. (2004). Bayesian estimation of ancestral character states on phylogenies. Syst. Biol. 53, 673–684. doi: 10.1080/10635150490522232
Paradis, E., and Schliep, K. (2019). Ape 5.0: An environment for modern phylogenetics and evolutionary analyses in R. Bioinformatics 35, 526–528. doi: 10.1093/bioinformatics/bty633
Parham, J. F., Donoghue, P. C., Bell, C. J., Calway, T. D., Head, J. J., Holroyd, P. A., et al. (2012). Best practices for justifying fossil calibrations. Syst. Biol. 61, 346–359. doi: 10.1093/sysbio/syr107
Pinheiro, J., Bates, D., and R Core Team (2022). Nlme: Linear and nonlinear mixed effects models. R package version 3.1-159. New York, NY: Springer.
Prum, R. O., Berv, J. S., Dornburg, A., Field, D. J., Townsend, J. P., Lemmon, E. M., et al. (2015). A comprehensive phylogeny of birds (Aves) using targeted next-generation DNA sequencing. Nature 526, 569–573. doi: 10.1038/nature15697
Quiroga, M. P., Mathiasen, P., Iglesias, A., Mill, R. R., and Premoli, A. C. (2016). Molecular and fossil evidence disentangle the biogeographical history of Podocarpus, a key genus in plant geography. J. Biogeogr. 43, 372–383. doi: 10.1111/jbi.12630
Rabosky, D. L., Grundler, M., Anderson, C., Title, P., Shi, J. J., Brown, J. W., et al. (2014). BAMM tools: An R package for the analysis of evolutionary dynamics on phylogenetic trees. Methods Ecol. Evol. 5, 701–707. doi: 10.1111/2041-210X.12199
Revell, L. J. (2012). Phytools: An R package for phylogenetic comparative biology (and other things). Methods Ecol. Evol. 3, 217–223. doi: 10.1111/j.2041-210X.2011.00169.x
Revell, L. J. (2014). Ancestral character estimation under the threshold model from quantitative genetics. Evolution 68, 743–759. doi: 10.1111/evo.12300
Reymanowna, M. (1987). A Jurassic podocarp from Poland. Rev. Palaeobot. Palyno. 51, 133–143. doi: 10.1016/0034-6667(87)90026-1
Sanderson, M. J. (2003). R8s: Inferring absolute rates of molecular evolution and divergence times in the absence of a molecular clock. Bioinformatics 19, 301–302. doi: 10.1093/bioinformatics/19.2.301
Sauquet, H., Ramírez-Barahona, S., and Magallón, S. (2022). What is the age of flowering plants? J. Exp. Bot. 73, 3840–3853. doi: 10.1093/jxb/erac130
Scott, A. C., and Chaloner, W. G. (1983). The earliest fossil conifer from the westphalian B of Yorkshire. Proc. R. Soc. B 220, 163–182. doi: 10.1098/rspb.1983.0094
Slater, G. J., Harmon, L. J., and Alfaro, M. E. (2012). Integrating fossils with molecular phylogenies improves inference of trait evolution. Evolution 66, 3931–3944. doi: 10.1111/j.1558-5646.2012.01723.x
Smith, S. A., Beaulieu, J. M., and Donoghue, M. J. (2010). An uncorrelated relaxed-clock analysis suggests an earlier origin for flowering plants. Proc. Natl. Acad. Sci. U.S.A. 107, 5897–5902. doi: 10.1073/pnas.1001225107
Sundaram, M., and Leslie, A. B. (2021). The influence of climate and palaeoclimate on distributions of global conifer clades depends on geographical range size. J. Biogeogr. 48, 2286–2297. doi: 10.1111/jbi.14152
Sundaram, M., Donoghue, M. J., Farjon, A., Filer, D., Mathews, S., Jetz, W., et al. (2019). Accumulation over evolutionary time as a major cause of biodiversity hotspots in conifers. Proc. R. Soc. B 286:20191887. doi: 10.1098/rspb.2019.1887
Taylor, E. L., Taylor, T. N., and Krings, M. (2009). Paleobotany: The biology and evolution of fossil plants. Burlington, MA: Academic Press, Elsevier.
Tiffney, B. H. (1984). Seed size, dispersal syndromes, and the rise of the angiosperms: Evidence and hypothesis. Ann. Mo. Bot. Gard. 71, 551–576. doi: 10.2307/2399037
Tiffney, B. H. (2004). Vertebrate dispersal of seed plants through time. Ann. Rev. Ecol. Evol. Syst. 35, 1–29. doi: 10.1146/annurev.ecolsys.34.011802.132535
Vishnu-Mittre. (1959). Studies on the fossil flora of nipania (rajmahal series), bihar-coniferales. Palaeobotanist 6, 82–112. doi: 10.54991/jop.1957.560
Wheelwright, N. T. (1985). Fruit-size, gape width, and the diets of fruit-eating birds. Ecology 66, 808–818. doi: 10.2307/1940542
Wilf, P. (2012). Rainforest conifers of eocene Patagonia: Attached cones and foliage of the extant Southeast Asian and Australasian genus Dacrycarpus (podocarpaceae). Am. J. Bot. 99, 562–584. doi: 10.3732/ajb.1100367
Wilf, P., and Escapa, I. H. (2015). Green web or megabiased clock? Plant fossils from gondwanan Patagonia speak on evolutionary radiations. New Phytol. 207, 283–290. doi: 10.1111/nph.13114
Wilf, P., Donovan, M. P., Cúneo, N. R., and Gandolfo, M. A. (2017). The fossil flip-leaves (Retrophyllum, podocarpaceae) of southern South America. Am. J. Bot. 104, 1344–1369. doi: 10.3732/ajb.1700158
Willson, M. F., Sabag, C., Figueroa, J., and Armesto, J. J. (1996). Frugivory and seed dispersal of Podocarpus nubigenus in Chiloe, Chile. Rev. Chil. De Hist. Nat. 69, 343–349.
Wing, S. L., and Tiffney, B. H. (1987). The reciprocal interaction of angiosperm evolution and tetrapod herbivory. Rev. Palaeobot. Palynol. 50, 179–210. doi: 10.1016/0034-6667(87)90045-5
Yu, Y., Zhan, C., and Xu, X. (2021). Deep time diversity and the early radiations of birds. Proc. Natl. Acad. Sci. U.S.A. 118:e2019865118. doi: 10.1073/pnas.2019865118
Zhang, C., Stadler, T., Klopfstein, S., Heath, T. A., and Ronquist, F. (2016). Total-evidence dating under the fossilized birth–death process. Syst. Biol. 65, 228–249. doi: 10.1093/sysbio/syv080
Zheng, X., Martin, L. D., Zhou, Z., Burnham, D. A., Zhang, F., and Miao, D. (2011). Fossil evidence of avian crops from the early cretaceous of China. Proc. Natl. Acad. Sci. U.S.A. 108, 15904–15907. doi: 10.1073/pnas.1112694108
Zheng, X., O’Connor, J. K., Wang, X., Wang, Y., and Zhou, Z. (2018). Reinterpretation of a previously described jehol bird clarifies early trophic evolution in the ornithuromorpha. Proc. R. Soc. B 285:20172494. doi: 10.1098/rspb.2017.2494
Zhou, Z. (2014). The jehol biota, an early cretaceous terrestrial lagerstätte: New discoveries and implications. Natl. Sci. Rev. 1, 543–559. doi: 10.1093/nsr/nwu055
Keywords: conifers, seed cone, birds, plant-animal interactions, Early Cretaceous
Citation: Leslie AB and Benson RBJ (2022) Neontological and paleontological congruence in the evolution of Podocarpaceae (coniferales) reproductive morphology. Front. Ecol. Evol. 10:1058746. doi: 10.3389/fevo.2022.1058746
Received: 30 September 2022; Accepted: 11 November 2022;
Published: 25 November 2022.
Edited by:
Mario Coiro, University of Vienna, AustriaReviewed by:
Santiago Ramírez-Barahona, National Autonomous University of Mexico, MexicoDori Contreras, Perot Museum of Nature and Science, United States
Julian Herting, Royal Botanic Gardens and Domain Trust, Australia
Copyright © 2022 Leslie and Benson. This is an open-access article distributed under the terms of the Creative Commons Attribution License (CC BY). The use, distribution or reproduction in other forums is permitted, provided the original author(s) and the copyright owner(s) are credited and that the original publication in this journal is cited, in accordance with accepted academic practice. No use, distribution or reproduction is permitted which does not comply with these terms.
*Correspondence: Andrew B. Leslie, aleslieb@stanford.edu; Roger B. J. Benson, roger.benson@earth.ox.ac.uk