- 1School of Earth and Environmental Sciences, The University of Queensland, Brisbane, QLD, Australia
- 2Centre for Biodiversity and Conservation Science, The University of Queensland, Brisbane, QLD, Australia
- 3Institute for Biodiversity and Ecosystem Dynamics (IBED), University of Amsterdam, Amsterdam, Netherlands
- 4Australian Rivers Institute, Griffith University, Nathan, QLD, Australia
- 5Wageningen University and Research, Wageningen, Netherlands
- 6The Peregrine Fund, Boise, ID, United States
- 7Environment, Natural Resources and the Blue Economy Global Practice, The World Bank, Washington, DC, United States
- 8Mohamed Bin Zayed Raptor Conservation Fund, Abu Dhabi, United Arab Emirates
- 9Wildlife Conservation Research Unit, Department of Zoology, Recanati-Kaplan Centre, University of Oxford, Oxford, United Kingdom
- 10Department on Environmental Studies, University of Nairobi, Nairobi, Kenya
- 11Andlinger Center for Energy and the Environment, Princeton University, Princeton, NJ, United States
- 12Dow Centre for Sustainable Engineering Innovation, School of Chemical Engineering, The University of Queensland, Brisbane, QLD, Australia
- 13Department of Geography, University of Cambridge, Cambridge, United Kingdom
Raptors are emblematic of the global biodiversity crisis because one out of five species are threatened with extinction and over half have declining populations due to human threats. Yet our understanding of where these “threats” impact raptor species is limited across terrestrial Earth. This is concerning because raptors, as apex predators, are critically positioned in ecological food webs, and their declining populations can undermine important ecosystem services ranging from pest control to disease regulation. Here, we map the distribution of 15 threats within the known ranges of 172 threatened and near threatened raptor species globally as declared by the International Union for the Conservation of Nature. We analyze the proportion of each raptor range that is exposed to threats, identify global hotspots of impacted raptor richness, and investigate how human impacts on raptors vary based on several intrinsic (species traits) and extrinsic factors. We find that humans are potentially negatively affecting at least one threatened raptor species across three quarters of Earth’s terrestrial area (78%; 113 million km2). Our results also show that raptors have 66% of their range potentially impacted by threats on average (range 2.7–100%). Alarmingly, critically endangered species have 90% of their range impacted by threats on average. We also highlight 57 species (33%) of particular concern that have > 90% of their ranges potentially impacted. Without immediate conservation intervention, these 57 species, including the most heavily impacted Forest Owlet (Athene blewitti), the Madagascar Serpent-eagle (Eutriorchis astur), and the Rufous Fishing-owl (Scotopelia ussheri), will likely face extinction in the near future. Global “hotspots” of impacted raptor richness are ubiquitous, with core areas of threat in parts of the Sahel and East Africa where 92% of the assessed raptors are potentially impacted per grid cell (10 species on average), and in Northern India where nearly 100% of raptors are potentially impacted per grid cell (11 species). Additionally, “coolspots” of unimpacted richness that represent refuges from threats occur in Greenland and Canada, where 98 and 58% of raptors are potentially unimpacted per grid cell, respectively (nearly one species on average), Saharan Africa, where 21% of raptors are potentially unimpacted per grid cell (one species on average), and parts of the Amazon, where 12% of raptors are potentially unimpacted per grid cell (0.6 species on average). The results provide essential information to guide conservation planning and action for the world’s imperiled raptors.
Introduction
Raptors—birds within the orders Accipitriformes, Cathartiformes, Falconiformes, Strigiformes, and Cariamiformes (Iriarte et al., 2019; McClure et al., 2019)—are some of the most iconic species on Earth. They are also some of the most endangered, with 18% of raptor species threatened with extinction, and 52% facing declining populations due to human threats (McClure et al., 2018). This is concerning given the important ecosystem functions and services that predator and scavenger species provide. Indeed, raptors are critically positioned in food webs and their loss can trigger top-down trophic cascades that cause an increase in mesopredators and mesoscavengers, altering ecosystem structure and functioning (Sekercioglu, 2006; Buechley and Şekercioğlu, 2016; O’Bryan et al., 2019). Such trophic cascades can result in burgeoning pest species, increased livestock carcasses and organic waste, and prevalence of reservoir species that host dangerous zoonotic pathogens (Markandya et al., 2008; Sergio et al., 2008; Ogada et al., 2012b; Donázar et al., 2016; O’Bryan et al., 2018). For example, the catastrophic decline of vulture populations across the Indian subcontinent in the 1990’s driven by the veterinary drug diclofenac for cattle (Oaks et al., 2004) coincided with an increase in feral dog populations that consumed livestock carcasses (Markandya et al., 2008). This resulted in a spike in human rabies infections and death, as well as a financial burden for the Indian government in treatment costs (Markandya et al., 2008). Therefore, halting raptor declines and ensuring population persistence is important for ecosystem health, human wellbeing, and associated cultural and inherent value.
Our understanding of raptor distributions is growing (McClure et al., 2021), and globally consistent spatial data on raptor geographic ranges are now openly available (BirdLife, 2021). Similarly, knowledge on the human pressures that threaten raptors is also improving (Carrete et al., 2009b; McClure et al., 2018). Indeed, conversion of habitat for agriculture or aquaculture is the most common threat across all raptor species, followed by logging and wood harvesting, and hunting and trapping (McClure et al., 2018). Poisoning (both direct and indirect), collisions and electrocution with service lines and energy facilities such as wind turbines are also major threats to raptors (Lehman et al., 2007; Carrete et al., 2009a; Ogada et al., 2016; Krone, 2018). However, data on the spatial distribution of threats to biodiversity has historically been lacking (Joppa et al., 2016), especially at fine resolutions necessary for conservation decision making (Venter et al., 2016b). Previous efforts to analyze human impacts on raptors have mapped a small number of their threats, including poisoning, potential wind farm collisions, and general indices of human pressure within raptor ranges (Santangeli et al., 2019a). Past studies have also assessed the overlap between raptor distributions and areas of political instability and violent conflict (Santangeli et al., 2019b), and identified raptor conservation priorities based on extinction risk and scientific attention (Buechley et al., 2019). These studies provide important information for conservation, but they have been constrained by the number of species they assess (e.g., only accipitrid vultures), by their geographical extent (e.g., only the global south), by the spatial resolution at which they analyze threats (e.g., using extinction risk as a range-wide proxy of threat exposure), and involve small subsets of the possible threats to raptors [e.g., four out of a possible 45 identified threats (IUCN, 2022)]. To date, a comprehensive global-scale analysis of threats within the geographic ranges of raptors is lacking, representing a gap in our ability to effectively prioritize conservation actions (Tulloch et al., 2015).
Mapping threats within species ranges is key to support targeted threat management efforts, which will help raptor conservation groups to identify priority sites for conservation action (Wilson et al., 2007; Auerbach et al., 2015). Recent studies have mapped high resolution threat data (e.g., global maps of human pressure; Venter et al., 2016a; Kennedy et al., 2019; Bowler et al., 2020) within the distributions of threatened vertebrate species to identify where potential human impacts are occurring (Allan et al., 2019; Delsen et al., 2020; Gallego-Zamorano et al., 2020; O’Bryan et al., 2020). These studies have shown that the threats that drive species declines can often be extensive within those species’ ranges, or even cover their entire ranges, making their survival dependent on conservation action. The utility of a similar analysis focusing on raptors would help in identifying and prioritizing species and areas that require urgent conservation action and investment.
Here, we aim to determine the human impacts on the world’s imperiled raptors using a recently published database that contains information on the ranges of 172 threatened raptor species (BirdLife, 2021), the distribution of eight major threats and 15 sub-classes of threats to raptors, as identified by the International Union for the Conservation of Nature (IUCN, 2022). These threats include the main drivers of each species’ extinction risk and population decline, and the database contains maps of the distribution of threats within the ranges of species that are specifically sensitive to each threat (Allan et al., 2019). We first identify global hotspots of impacted raptor richness, followed by “coolspots” that act as refuges from threats, and we then explore the impacts of each individual threat. We then analyze the proportion of each raptor range that is exposed to threats and investigate how human impacts on raptors vary based on several conservation-relevant factors, including species extinction risk, habitat preferences (e.g., forest dependence McClure et al., 2018), ecological traits (e.g., generation length, body size, and range size), and migratory status. Our work represents the first global spatial analysis of human impacts on threatened raptors.
Materials and methods
Spatial data on threatened raptor ranges
We obtained extent-of-occurrence maps on the native and reintroduced distributions of all (n = 177) threatened and near threatened raptor species from BirdLife International (January 2021.1 version). We focused on threatened or near threatened raptors because they have been comprehensively assessed by the IUCN and contain information on species-specific threats known to cause their extinction. We only considered the extant and possibly extant parts of each species distribution in our analysis, including the migratory, breeding, and wintering ranges, and excluding ranges of possibly extinct (just Glaucidium mooreorum) or extinct species. We excluded parts of species’ ranges with uncertain or no current records of species presences as assessed by the IUCN, as well as introduced and vagrant species and species with unknown origin. This was done by deleting the polygons within a species range that had these uncertainty characteristics according to the IUCN. We also excluded four species: Otus feae, Otus insularis, Buteo galapagoensis, and Falco araeus whose distributions did not overlap with the extent of the spatial threat data. Although the threat data are global in scope, they do not extend to some of the world’s more remote or smaller islands. This resulted in 172 raptor species for the analyses (Supplementary Table 1).
Spatial data on threats to raptors
We utilized spatial data on the distribution of forest loss from Global Forest Change (Hansen et al., 2013). Forest loss is defined as a stand-replacement disturbance, or a change from a forest to a non-forest state for the years 2001 through 2019 at a 30 m resolution (Hansen et al., 2013).
We obtained the majority of the spatial data on the distribution of threats from the global human footprint maps (Venter et al., 2016a). This includes high resolution (1 km2) globally comparable maps of built environments, human population density, electric infrastructure, crop lands, pasture lands, roads, railways, and navigable waterways for the year 2009 (Venter et al., 2016a). The data have been validated for accuracy and are one of the most up-to-date and comprehensive cumulative threat maps available (McGowan, 2016).
Each underlying layer in the human footprint is scaled between 1 and 10 based on its estimated harm to the environment. Following Allan et al. (2019), we converted each individual threat layer to binary, considering threats as present or absent in any 1 km2 pixel. We did this because there is no standardized data on the relative severity of threats to individual raptor species. For continuous data we set cutoffs beyond which a threat was considered absent (e.g., we consider the threat of roads present up to 3 km on each side) (Table 1). We adopt the same cutoffs as Allan et al. (2019) for consistency as they assessed the impacts of threatened vertebrates, including > 2,000 threatened terrestrial bird species.
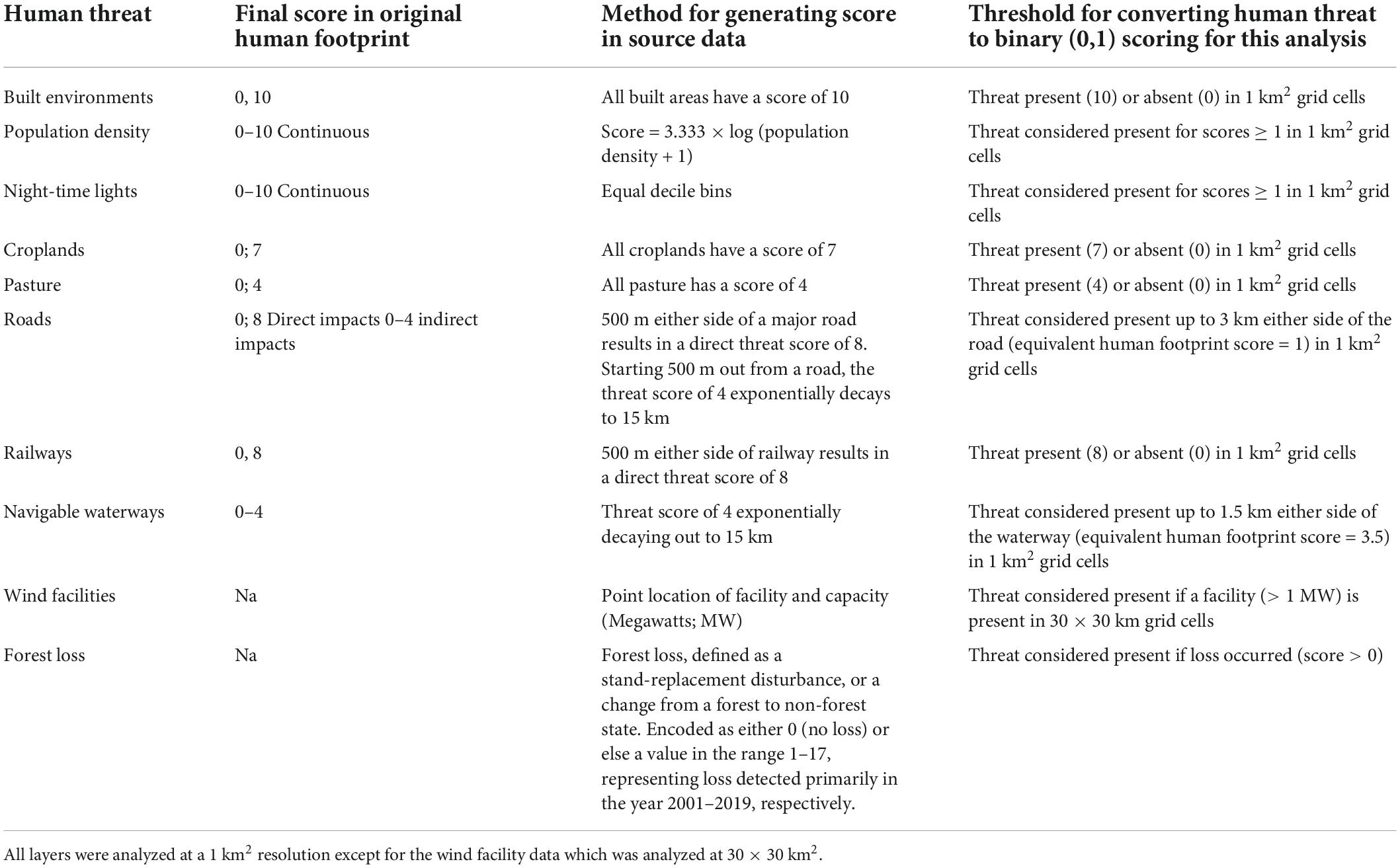
Table 1. Scores assigned to individual threats in the Human Footprint, GlobalData, and Global Forest Change (Hansen et al., 2013; Venter et al., 2016a; GlobalData, 2018), the method by which the human footprint scores were originally designated, and the threshold scheme used to convert the scores into binary (1 = present or 0 = absent) for our impact analyses following (Allan et al., 2019).
In addition to the human footprint data and forest loss data, we also obtained data on the spatial distribution of onshore wind-power facilities from the GlobalData Power Database (GlobalData, 2018). We only included operational facilities that are classified as “active” in the source database. This is one of the most complete global collections of electricity generation facility information available, and has been validated with a high degree of accuracy (Rehbein et al., 2020). Because local perceptions may influence what is considered a “large” facility, we use a 1 MW (MW = MegaWatt) threshold to represent utility-scale turbines (>50 m) (DOE, 2018). Utility-scale facilities are composed of increasingly larger turbines that are on average 75–90 m in height (max 200 m), with an average rotor diameter of 115 m (max 165 m) (DOE, 2018). Modern onshore facilities have one additional turbine for every 1.5–5 MW, and each turbine requires an average area of 0.3 km2 from 0.2 to 1 km2 to operate optimally (Tabassum et al., 2014; Rinne et al., 2018). Therefore, we considered wind turbines present as a threat in a 30 × 30 km grid cell if the facility’s total nominal capacity is > 1 MW. This scale accounts for most facilities having multiple turbines, and for alterations in bird flight patterns and habitat selection in proximity to turbines (May, 2015). See Supplementary Figure 1 for the spatial extent of all threats used in this analysis.
Mapping raptor-specific threats
We identified where spatial data on threats directly or indirectly correspond to biodiversity threats as listed by the IUCN Red List, following Allan et al. (2019). This enabled us to map eight out of 12 major threat classes and 15 out of 45 sub-classes of threats (IUCN, 2022; see Table 2 for a full list of possible threats to species). Although the 15 threats we mapped do not include every possible threat (e.g., direct or indirect poisoning), our list is the most comprehensive global analysis of human impacts on raptors to date and includes many drivers of biodiversity declines globally (Maxwell et al., 2016). For instance, numerous forms of agriculture, urban development, wind energy, and transportation corridors are directly accounted for by our threat data, while biological resource use and overexploitation through hunting, pollution, human disturbance, utility lines, and invasive species are indirectly accounted for by human population density, roads and navigable river networks that act as proxies (Canning, 1998; Trombulak and Frissell, 2000; Laurance et al., 2006; Meunier and Lavoie, 2012; Levin et al., 2015; Benítez-López et al., 2017; Symes et al., 2018; Allan et al., 2019).
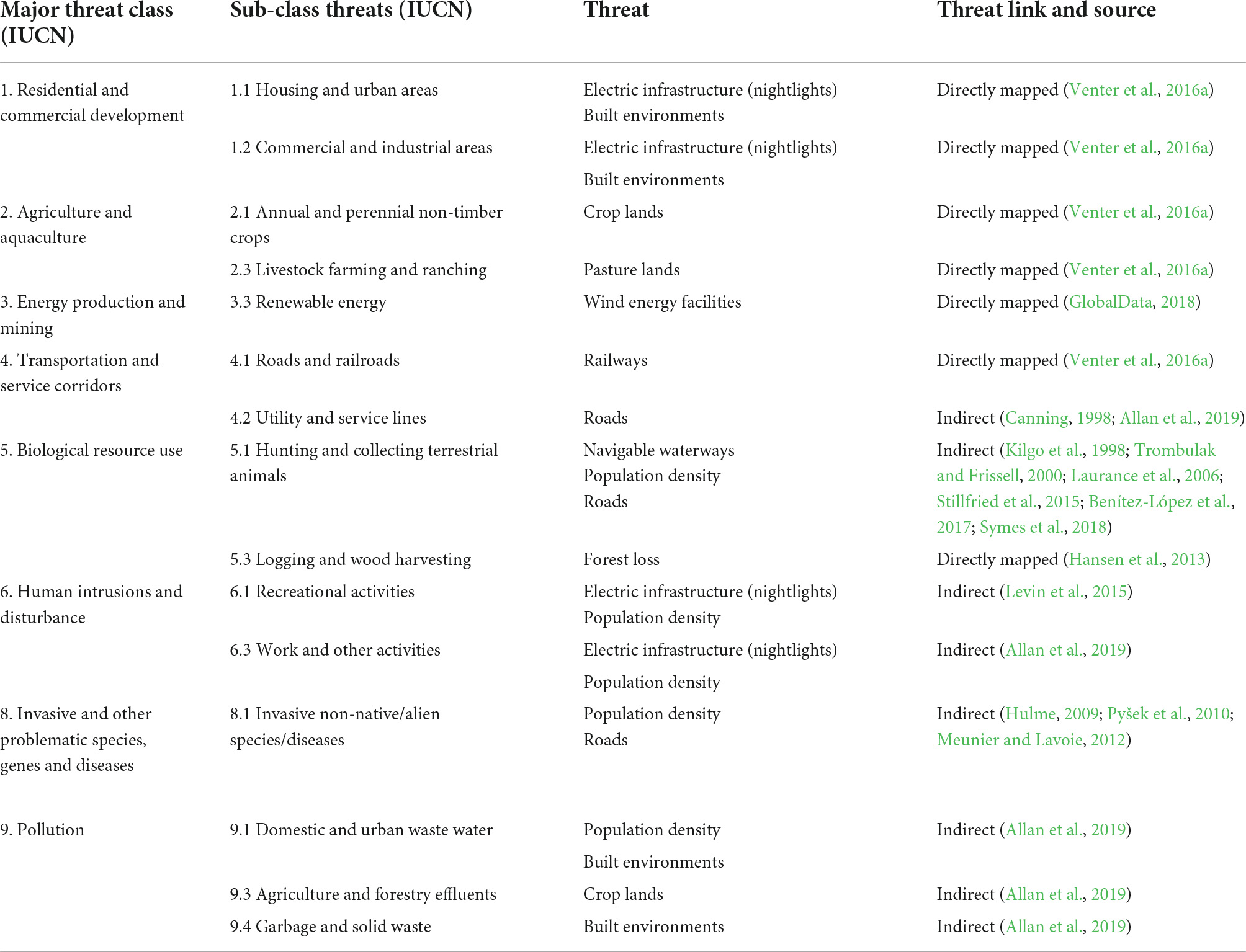
Table 2. Major classes and sub-classes of threats to biodiversity as classified in the IUCN Red List of Threatened Species (IUCN, 2022), and the corresponding spatially explicit threat variable from the updated Human Footprint, GlobalData, and Global Forest Change (Hansen et al., 2013; Venter et al., 2016a; GlobalData, 2018).
Analyzing human impacts within individual species distributions
If a spatial threat layer is present within a species’ range, and the species is sensitive to that threat according to the IUCN Red List, then we considered the threat to be potentially impacting the species where they overlap. We calculated this overlap for all species and all their species-specific threats at a 1 km2 resolution globally for all threats except wind turbines, which were calculated at a 30 × 30 km resolution as described above. We also identify where multiple threats overlap. All GIS analyses were conducted in ArcGIS 10.8 (ESRI) in a Mollweide equal area projection.
Mapping hotspots and coolspots of cumulative human impact
We estimated and mapped cumulative human impacts using a 30 × 30 km grid, which is an acceptable resolution for reducing commission errors (i.e., false presences) when working with species range maps (Di Marco et al., 2017). There is much debate concerning the ideal resolution of geographic range maps, with suggestions ranging from 10 km2 (Jenkins et al., 2013) to > 100 km2 (Hurlbert and Jetz, 2007). The 30 × 30 km resolution here represents a middle ground and enables comparisons with similar studies (Allan et al., 2019). We scored a potential impact if a species’ geographic range was present in a grid cell, and at least one threat to which it is sensitive is also present, using a GIS (ESRI ArcGIS v10.8). An overlap was determined if a species or threat had any overlap with a grid cell, which was accomplished by intersecting grid cells with the geographic distributions of threats and raptor ranges. We then summed all impacted species in a grid cell to estimate cumulative human impact (hotspots of impacted species richness). Following similar methods, we considered a cell a refuge if a species was present but no mapped species-specific threats were present. We then summed those species per grid cell to get a cumulative score of unimpacted species richness (coolspots). We also calculated the proportion of species impacted (or unimpacted) per grid cell by taking the number of impacted/unimpacted species for a grid cell and dividing by the richness of threatened raptors in that grid cell. We aggregate and present results at the country and biome scale. Biomes represent distinct bio-geographic units (Olson et al., 2001).
Post hoc analyses: Human impacts and raptor biological traits
We performed an analysis with a random forest algorithm to investigate the extent to which species intrinsic traits (body mass, generation length, forest dependency, raptor group, and nocturnality) and other factors (range size, extinction risk category, and taxonomic order) predict the proportion of a raptors range that is impacted by threats. Species trait data were compiled from multiple sources including the EltonTraits 1.0 database for body size (Wilman et al., 2014), and Bird et al. (2020) for generation length. Information on forest dependency (high, medium, low/non-forest), nocturnality, and if a species is migratory or not (including partial migrants), was extracted from BirdLife (2021). We used a random forest algorithm because it is insensitive to data distribution, does not assume data independence, can take a large number of potentially collinear variables, and handles higher-order interactions (Cutler et al., 2007). Random forest algorithms have also been used previously in research evaluating effects of ecological aspects on extinction risk among phylogenetically related species, including raptors (Buechley et al., 2019). For this analysis, we excluded families with less than 10 species (Sagittariidae, Cathartidae, and Tytonidae families), to avoid issues related to imbalanced datasets that may lead to inflated performance estimates (Evans et al., 2011). We carried out a sensitivity analysis where we included these species but found the patterns of the results did not change while the model explained less variance. Random forests were run using an unbiased tree algorithm because unbiased trees do not artificially favor splits in variables with many categories or continuous variables (Hothorn et al., 2006). The relative importance of predictor variables driving the proportion of range impacted was estimated using a conditional variable importance measure (Strobl et al., 2009). This measure is based on a random permutation of predictor variables and is supposed to be unbiased when predictor variables are correlated. We set the number of trees to 500, whereas the number of classification variables used to calculate the split at each node (mtry = 2) was estimated using the function Tune in R. All analyses were performed using the package “randomForest” in R version 4.0.2 (Liaw and Wiener, 2002).
Results
Global hotspots and coolspots of human impact on raptors
Our results show that humans are potentially negatively impacting at least one threatened raptor species across three quarters of Earth’s terrestrial area (78%; 113 million km2). Hotspots of human impact on threatened raptors occur predominantly in the Sahel region of Africa, parts of East Africa, Northern India, and southeastern South America (Figure 1A). Countries with the highest potential impacts include Ethiopia (16 species potentially impacted per grid cell on average), and Eritrea, Kenya, and Rwanda (14 species potentially impacted per grid cell on average) (Supplementary Table 2). These scores are considerably higher than the global average of 3.9 species impacted per grid cell. The highest impacts within Earth’s Biomes are in montane grasslands and savannas and deserts and xeric shrublands where eight species are impacted in a grid cell on average, followed by flooded grasslands and savannas (six species on average) and tropical and sub-tropical grasslands and savannas where five species are impacted in a grid cell on average (Table 3).
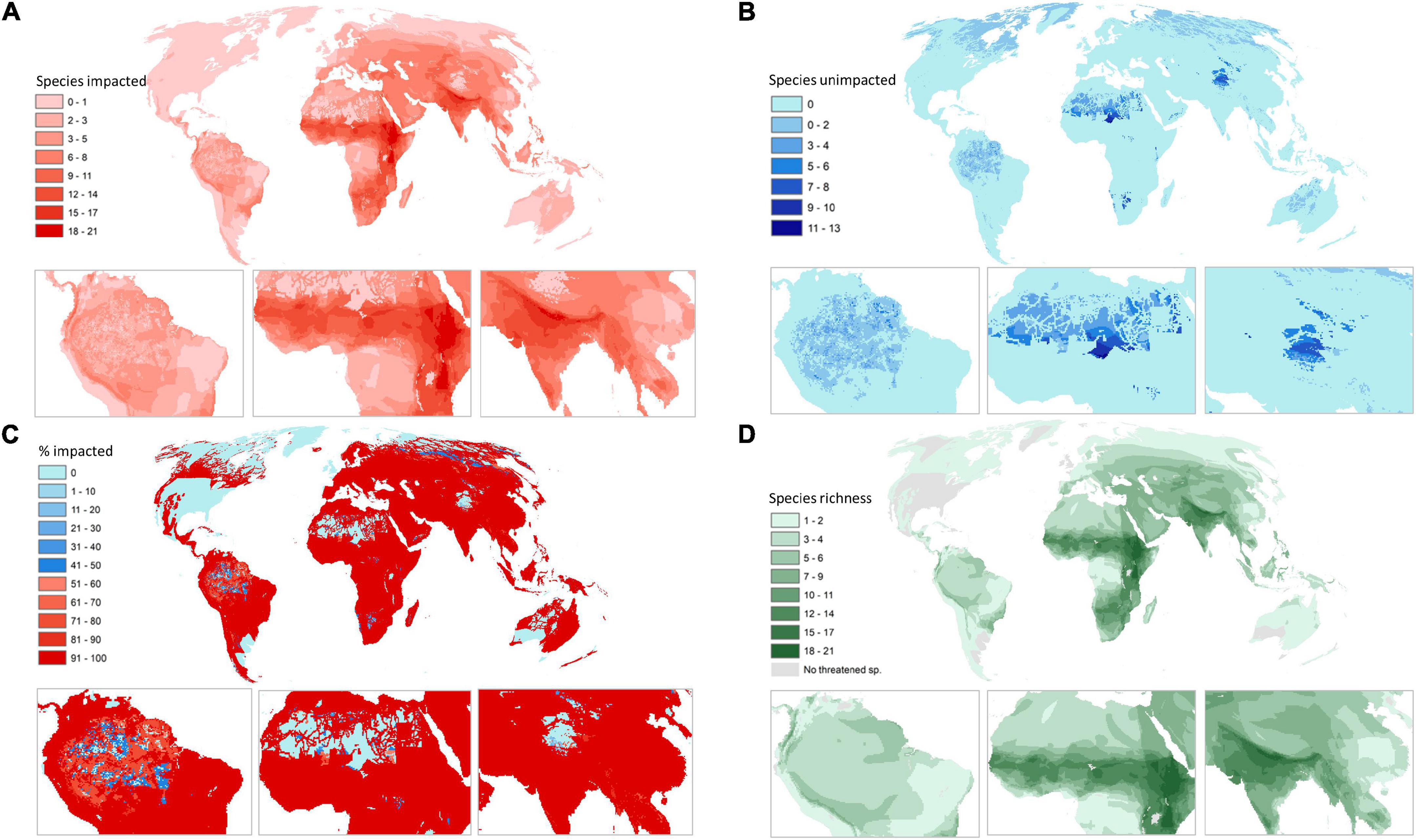
Figure 1. The number of threatened and near threatened raptor species (n = 172) impacted in a grid cell by at least one threat (A). The number of raptor species in a grid cell not impacted by any threats (B). The percentage of raptor species in a grid cell impacted by at least one threat (the inverse of which is the percentage not impacted) (C). And the richness of threatened and near threatened raptors (D). Maps use Mollweide equal area projection and a 30 × 30 km grid.
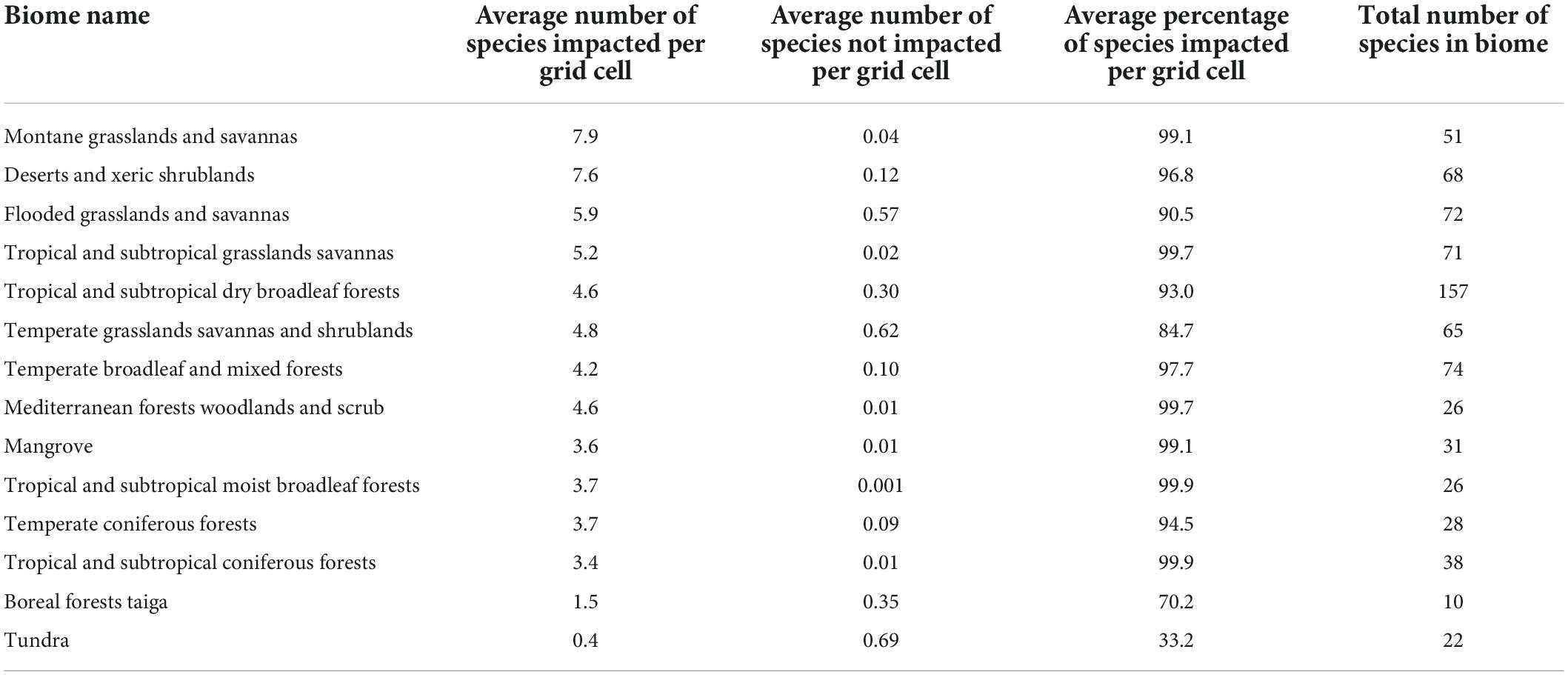
Table 3. The average number and proportion of raptor species impacted or not impacted by threats, including the total number of raptors, in each of Earth’s biomes as defined in Olson et al. (2001).
Unimpacted species occur across 22% of Earth’s terrestrial surface with potentially impacted and unimpacted species co-occurring across just four percent (31.5 and 6 million km2, respectively; Figure 1B). Coolspots of unimpacted raptor richness are found throughout the Amazon in South America, Sarahan Africa, and the Himalayas in central Asia. The highest number of unimpacted species in a single grid cell is 13, located in Niger and Namibia. Other coolspots of note include Botswana, Mauritania, and Suriname (two unimpacted species per grid cell on average). Although the number of species unimpacted is low across all biomes, the biome with the most unimpacted species is the tundra, with nearly one species not impacted per grid cell on average. This is followed by temperate grasslands savannas and shrublands, and flooded grasslands and savannas where just 0.6 species are not impacted on average (Table 3).
The proportion of impacted vs. unimpacted species in a grid cell is an important metric to correct for species richness (Figures 1C,D), which is one of the key drivers of the global patterns of hotspots and coolspots. Areas with high proportions of impacted species per grid cell are ubiquitous across South America, Africa, Asia, and Oceania. On average, far more raptors are impacted in a grid cell than not (4 vs. 0.3), with 77% of species impacted in a grid cell on average. The majority of countries have > 90% relative impacts per grid cell on average (153 countries). Some countries with the lowest relative impacts include Greenland (2% of species impacted on average), followed by Canada (41% of species impacted on average) and Niger (53% of species impacted on average). Tropical and subtropical moist broadleaf forests and tropical and subtropical coniferous forests had the highest proportional impacts of all Biomes (99.9% of species impacted on average; Table 3).
Of the 15 IUCN threats we mapped (directly or indirectly), all are potentially negatively impacting raptors within their ranges (Figure 2). The threat potentially impacting the most raptors is “logging and wood harvesting” (IUCN sub-class 5.3, mapped using forest loss data), with 125 raptors (73%) potentially impacted, followed by “cropping” (IUCN sub-class 2.1, mapped using crop lands data; n = 122; 71%), and “hunting” (IUCN sub-class 5.1, mapped using navigable waterways, population density, and roads data; n = 84; 49%) (Table 4). The IUCN sub-class threats of “logging and wood harvesting” as well as “cropping” also impact the most species per grid cell on average (4.9 species) followed by “agriculture and forestry effluents” (IUCN sub-class 9.3, mapped using crop lands data; 4.5 species) and “utility and service lines” (IUCN sub-class 4.2, mapped using roads data; 3.7 species), suggesting that these threat categories are particularly harmful when they co-occur with raptor species (Table 4). For further information on the proxy threat layers used to map each IUCN sub-class threats see Table 2.
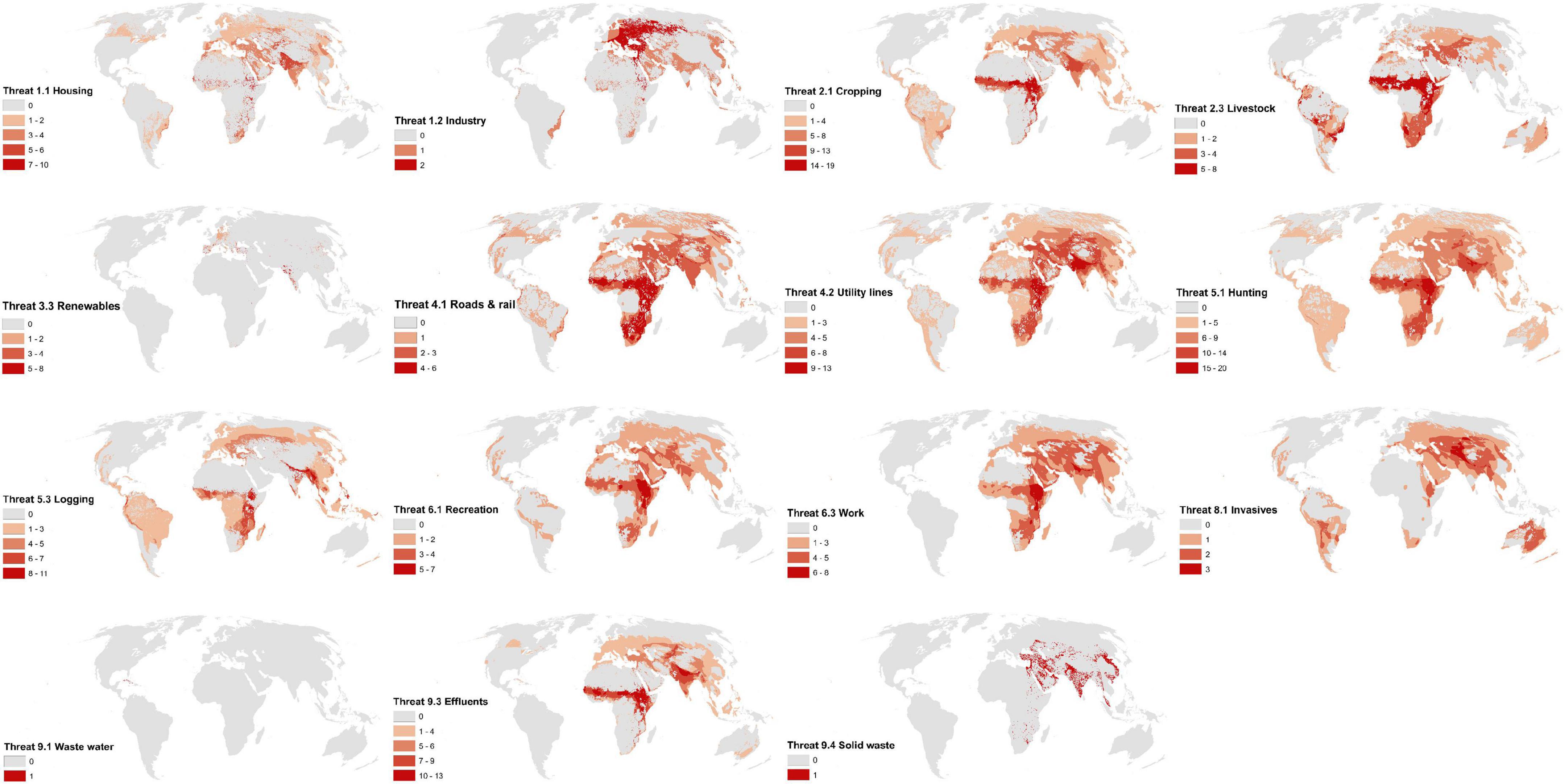
Figure 2. Hotspots of where individual threats as listed by the International Union for Conservation of Nature (IUCN) are potentially impacting threatened raptors (n = 172). Scales represent the number of species impacted by the threat in a grid cell. Hotspots of potential impact are in dark red. Maps use a 30 × 30 km grid and a Mollweide equal area projection. Note that some IUCN sub-class threats are mapped using the same proxy layers (e.g., IUCN sub-class threat 1.1 and 1.2 are mapped using nightlights and built environments, see Table 2) and that the differences in distribution for these are driven by the distribution of impacted raptors.
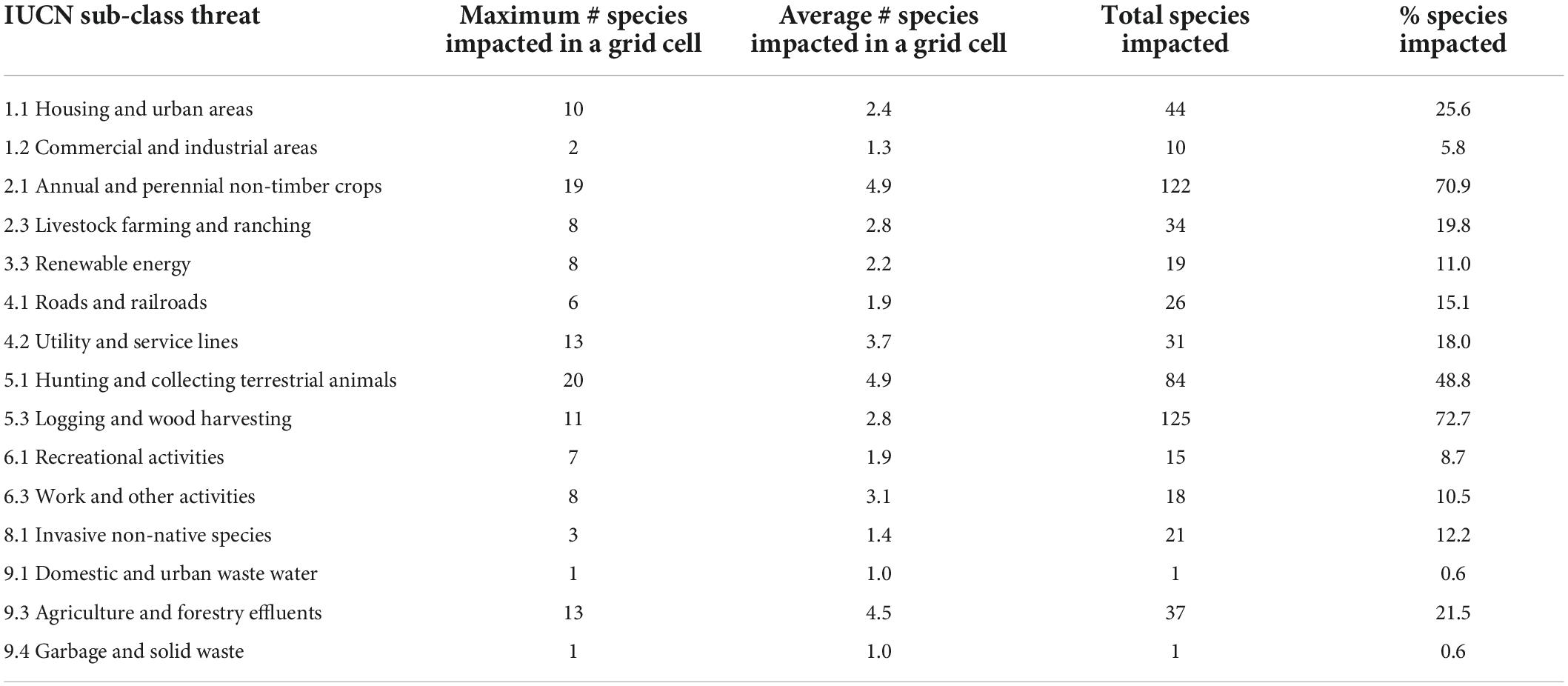
Table 4. Threats listed by the International Union for Nature Conservation (IUCN) and the maximum and average number of species they impact per grid cell, and the total number and percentage of species they impact.
Impacts within individual raptor ranges
We found that on average, 65.9% of a raptor species’ range is impacted by the threats that are known to directly drive raptor population declines and extinctions (range 2.7–100%; Supplementary Table 1). Of the 172 raptor species analyzed, one third (33%, n = 57) have > 90% of their range impacted by threats, including species such as the Forest Owlet (Athene blewitti), the Madagascar Serpent-eagle (Eutriorchis astur), and the Rufous Fishing-owl (Scotopelia ussheri) that have their entire range impacted. Disconcertingly, 16 species (10.8%) have > 99% of their range potentially impacted (Supplementary Table 1). Only 11 species have > 90% of their ranges free from potential impacts according to our analysis.
Raptor species that are highly threatened with extinction are disproportionately impacted by threats within their ranges compared to less threatened species. Specifically, raptors listed as Critically Endangered species have on average 90% (±SD 19%) of their range potentially impacted by threats (n = 15), followed by 80% (±SD 22%) for Endangered species (n = 34) and 64% (± SD 27%) for Vulnerable raptors (n = 59), while Near-threatened species ranges are on average of 54% (±SD 32%) impacted (n = 64). The extent of human impacts within raptor ranges varied considerably across orders, with Falconiformes and Cathartiformes most impacted with 79% (±SD 23%) and 78% (±SD 0.6%) of their ranges exposed to threats on average, respectively. Migratory raptors (including full migrants but excluding altitudinal migrants because those migrations are small relative to the spatial extent of this analysis) have larger proportions of their range impacted by threats (75.5% on average; ± SD 22.6%) than non-migratory raptors (64.1% on average; ± SD 30.7%). However, nomadic raptors that move in response to seasonal resource availability (n = 4; Elanus scriptus, Sagittarius serpentarius, Falco hypoleucos, Circaetus beaudouini) have the largest proportion of their range impacted by threats (79.3% on average; ± SD 18.6%).
The random forest algorithm shows that body size and generation length are the strongest predictors of the proportion of a species range potentially impacted by threats (27% of the variance explained). Species with longer generations and higher body mass tend to be slightly more impacted than shorter lived and smaller species (Figure 3). Although range size shows a high variable importance compared to other variables (Figure 3A), our model does not show a clear relationship between range size and the proportion of the range impacted (Figure 3D). Other variables included in the model (IUCN threat status, raptor group, forest dependency, nocturnality, and raptor family) showed very little predictive power.
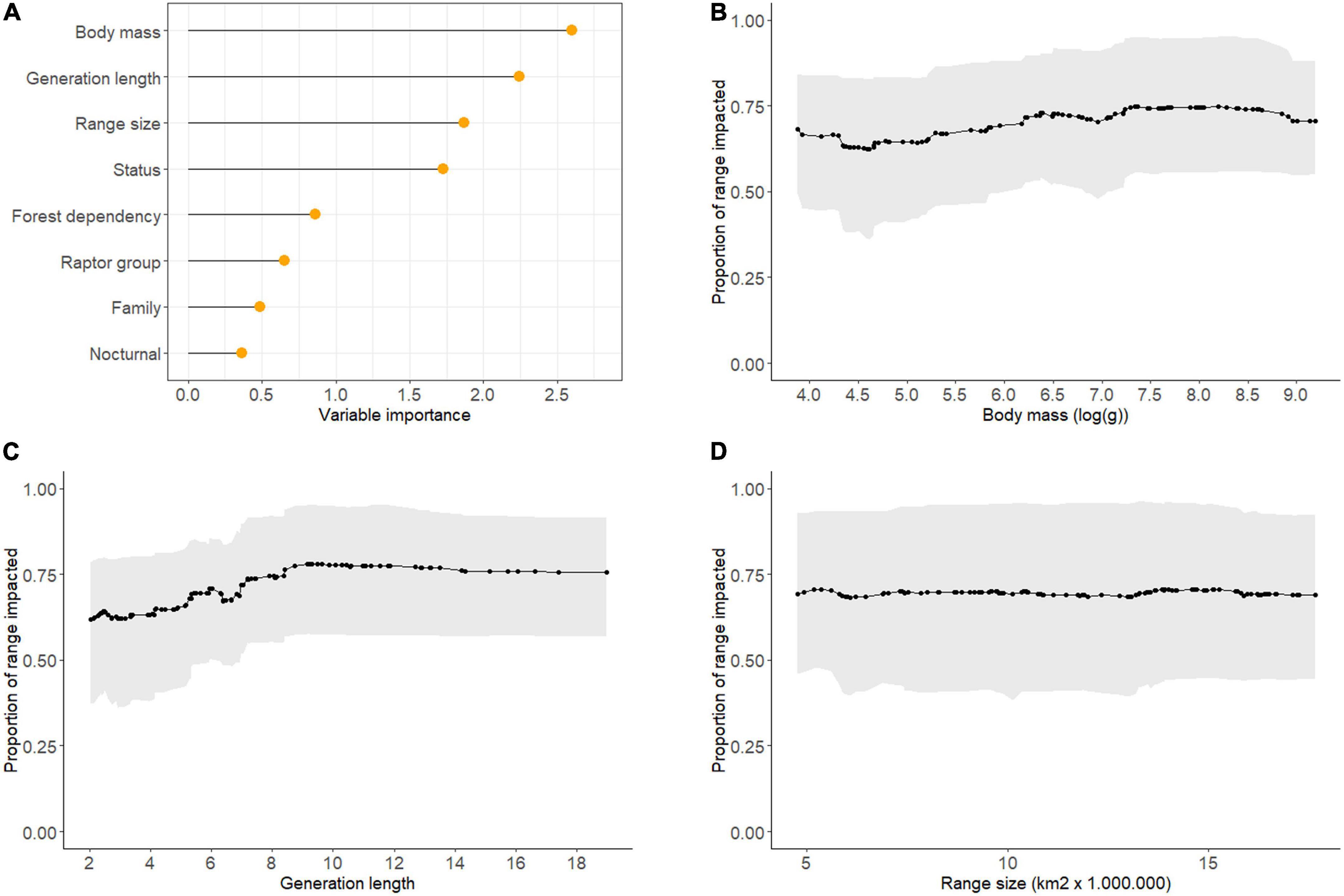
Figure 3. The relative importance of each variable in the random forest model at predicting the proportion of a species range impacted by threats (A), and partial dependence plots of the strongest predictors including; body mass (B), generation length (C), and range size (D). The gray shaded areas in (B–D) represent 95% confidence intervals.
Discussion
This is the first global spatial analysis of threats within the ranges of threatened and near threatened raptor species. We only mapped threats within a raptor range if that species is known to be endangered by that specific threat. In doing so, we identified places where humans are potentially negatively impacting the world’s raptors. We found that on average two-thirds of a threatened or near threatened raptor range is potentially impacted by threats (27% of the variance explained). This is higher than the global average for threatened or near threatened vertebrates (38%) and birds (37%) (Allan et al., 2019; O’Bryan et al., 2020). Our result is consistent with studies suggesting raptors are one of the most disproportionately threatened groups of species worldwide (Şekercioğlu et al., 2004; Buechley and Şekercioğlu, 2016; Buechley et al., 2019; McClure and Rolek, 2020), but demonstrates this with a new metric: the extent of a species range exposed to threats.
We found that the IUCN sub-class threats potentially impacting the most raptors included “logging and wood harvesting” along with “cropping” and “hunting and trapping.” Deforestation due to agricultural expansion and logging has been found to be a leading extinction threat for raptors globally (Thiollay and Meyburg, 1988; McClure et al., 2018), with species losing reproductive viability and habitat suitability as deforestation increases (e.g., with Harpy Eagles in Brazil, Miranda et al., 2021). Our findings for the threat of “hunting and trapping” may be partly a result of using human population density and accessibility via roads and waterways as a proxy for hunting threats because global spatial layers that quantify hunting are absent. Similarly, “utility and service lines” were mapped using these proxies and are widespread and can have devastating impacts on raptors. For example, a study in Mongolia found 490 electrocuted Saker Falcons (Falco cherrug) along a single power line in 2013–2014 (Dixon et al., 2020), and a study in Sudan suggests that persistent mortality along power lines is a key driver of Egyptian Vulture (Neophron percnopterus) declines (Angelov et al., 2013).
The global hotspots of human impacts on raptors that we have identified are mostly consistent with studies that have mapped raptor conservation priorities (Buechley et al., 2019; Santangeli et al., 2019a). East Africa and parts of Latin America and the Indian sub-Continent, especially the Himalayas, often contain hotspots of richness, threat, human impact, and conservation priority regardless of the methods or metrics used. An interesting difference between our study and others is that Southeast Asia does not emerge as a top global hotspot of human impacts on raptors, while others suggest Southeast Asia, especially Indonesia, as the highest global priority for raptor conservation and research (McClure et al., 2018, 2020; Buechley et al., 2019). A plausible reason for this difference could be spatial resolution—our analysis is grid-based while previous studies aggregated information at the country or ecoregion level and thus do not account for the extent of species ranges within those countries and may estimate high national level priority in places with many small-ranged species (McClure et al., 2018). For example, Indonesia is tied for the second-greatest (n = 13) species richness of threatened raptors in the world (McClure et al., 2018). Yet, any particular grid cell within the country is unlikely to contain many species because the threatened raptors within Indonesia have small ranges that are unlikely to overlap.
Interestingly, migratory raptors had larger proportions of their ranges exposed to threats than non-migratory raptors. This suggests that the breeding and non-breeding sites as well as the area raptors traverse between breeding and non-breeding sites contains substantial human pressures that threaten raptors with extinction, pointing to the need for minimizing threats across all portions of a species’ range. A Memorandum of Understanding on the conservation of migratory birds of prey has been signed by 61 range states—nations that contain ranges of raptors—under the Convention on Migratory Species (CMS, 2014). The aim is for nations to take coordinated measures to reduce raptor declines and return them to a favorable conservation status. A key step is for nations to prepare national action plans; however, only a handful of countries have done so to date. This is concerning given that migratory raptors are strongly exposed to threats. Our analyses could potentially help to inform raptor action plans. By mapping species and their specific threats, our analytical framework provides the basis for analyses that prioritize species and threat-specific management actions globally (Tulloch et al., 2015). This would ensure that conservation interventions provide the greatest biodiversity return on investment (Cattarino et al., 2015). Encouraging nations to prepare action plans and meet their CMS commitments is crucial for successful raptor conservation across international boundaries.
Our analysis of the traits that predict the proportion of a species, range impacted by threats shows that large-bodied species with slow life histories are more extensively impacted. These traits are also among the key predictors of raptor extinction risk (Buechley et al., 2019). It is important to note that the confidence intervals in our analysis are large, and variables including extinction risk, habitat preference, nocturnality, and forest dependency showed low predictive power.
Our analysis also has other caveats worthy of discussion. We highlight potential impact, but there may be cases where impacts are not occurring or have not been yet realized in the population. And many of the threats are mapped using proxy layers, for example the use of nightlights for the IUCN threat of recreational activities (a relationship shown for protected areas but not for other regions; Levin et al., 2015). Further, although the data used in our analysis may become more accurate in the coming decades, our analysis is limited by available coarse data on species distributions, threat assessments, and human pressures, with some potential for multicollinearity across threat layers. Although local decision-makers can use our findings as a guide, they should also harness more nuanced and locally accurate maps of threats and species’ distributions (Efrat et al., 2020). We are also limited by the time-frame of our threat data, and recent development and planned development for the future (e.g., wind farm and power line expansion in the eastern African-Eurasian flyway) will result in even larger proportions of the species ranges being impacted, particularly for migratory species. Moreover, our estimates of impacts on raptors are likely conservative because we are limited to species’ extent of occurrence and not the area of occupancy. This likely produces underestimates for threats that are mapped at fine scales (e.g., forest loss). Our results are also conservative because we could not account for all possible threats. For example, we did not include invasive species, human overharvesting, poisoning, climate change, and pollution, all of which can imperil raptors (Speziale and Lambertucci, 2013; Sarasola et al., 2018). Poisoning is an especially important threat for old world vultures and future studies would benefit from its inclusion. Santangeli et al. (2019a) mapped poisoning risk to old world vultures using human-carnivore conflict as a proxy. Nevertheless, scaling this up to a globally consistent and comparable map of poisoning is challenging given the global diversity of poisoning ranging from lead used in hunter bullets in the United States to cyanide poisoning of carnivores in Africa where scavenging raptors are indirectly killed, and carbofuran poisoning of Andean condors (Vultur gryphus) in Argentina (Alarcón and Lambertucci, 2018). Poisoning is also a leading cause of mortality for many species in Europe (e.g., Ogada et al., 2012a). Additionally, many of the threats we assess vary spatially and according to cultural and socio-economic conditions. For example, for the IUCN sub-threat 9.3 “Agriculture and forestry effluents,” the types and regimes of pesticide use are likely to be very different in Europe compared to the Sahel of Africa. Lastly, we found that owls (Strigiformes) were one of the least impacted raptor groups. However, this may be due to biases in research, knowledge, and threat data availability favoring diurnal raptors, since owls are the least studied raptor group globally (Brambilla et al., 2015; McClure et al., 2018; Buechley et al., 2019). As such, future research can focus specifically on threats to nocturnal raptors (Buechley et al., 2019).
There is room for hope because many if not all of the threats we mapped can be mitigated through in situ conservation action or smart policy to regulate threats. For example, one of the rarest raptors in the world, the Spanish imperial eagle (Aquila adalberti) suffered high mortality due to electrocutions on power lines, yet after the Spanish government established mandatory rules for power line design to minimize electrocution risk in the 1990’s the number of electrocuted birds has decreased substantially (López-López et al., 2011). This policy change was coupled with supplementary feeding efforts to improve the fledging rate, and together mean the Spanish imperial eagle population has increased (González et al., 2006). Another good example is the Ridgeway’s Hawk (Buteo ridgwayi) in the Dominican Republic that faces multiple threats including hunting, habitat loss, invasive species, and utility lines. This cocktail of threats was so intense that assisted dispersal was required to move young hawks to safer territory and expand the species’ range, while simultaneously retrofitting powerlines to reduce electrocution risk (McClure et al., 2017). These conservation efforts have been successful and there are now > 400 hawks in three distinct populations (up from < 300 in one population) (Watson, 2018).
Conservation of the world’s raptors requires understanding the individual and combined threats within their geographic distributions (McClure et al., 2018). Although our study is the first to map the threats that are known to drive declines of the world’s raptors, there is opportunity for enhancing our knowledge on the vast number of human threats that imperil these species globally. Our results point to many raptors being exposed to both active and passive threats, meaning that conservation practitioners must employ a diverse set of actions to ameliorate risk of further population declines. However, our results also point to gaps in our knowledge on threats to raptors, with nocturnal species such as owls potentially lacking critical information on what could cause their extinction. It is our hope that our work stems more nuanced investigation on human-derived threats to raptors, with a focus on mapping active threats such as retaliatory killings, poaching pressures, habitat fragmentation, and climate change. Future work can also investigate the areas where raptors have gone extinct or lost historic range and assess prevalence of threats to inform future restoration efforts.
Raptors are critical elements in ecosystem food webs that have direct and indirect contributions to human health and wellbeing (O’Bryan et al., 2018). Multiple studies have shown that raptors are declining at an unprecedented rate, potentially with many impending extinctions (McClure et al., 2018; Buechley et al., 2019). This will undoubtedly have adverse effects on ecosystem structure and function and affect human society. The socio-ecological cascades resulting from raptor declines range from burgeoning disease risk due to increased organic waste (Gangoso et al., 2013; Plaza et al., 2020) and increased financial burden due to control of crop pests and associated losses (Kross et al., 2012, 2016). Therefore, protecting the world’s raptors is a global imperative for biodiversity conservation, and for human society.
Data availability statement
The original contributions presented in this study are included in the article/Supplementary material, further inquiries can be directed to the corresponding author.
Author contributions
JA initially led the study. CO’B led the study at a later stage. JA, WDK, MV, RB, and CM conceived the study. JA, CO’B, AS-C, DD, PT, PB, and JR carried out the analyses. CG contributed to the data. JA, WDK, and CO’B wrote the initial manuscript. All authors edited and reviewed the manuscript.
Funding
WDK acknowledges support from the Faculty Research Cluster “Global Ecology” of the University of Amsterdam and from a University of Amsterdam starting grant.
Acknowledgments
We would like to acknowledge Oscar Venter, Hugh Possingham, and Moreno Di Marco for discussions on this topic.
Conflict of interest
The authors declare that the research was conducted in the absence of any commercial or financial relationships that could be construed as a potential conflict of interest.
The handling editor declared a past co-authorship with several authors, RB, CM, WDK, and MV.
Publisher’s note
All claims expressed in this article are solely those of the authors and do not necessarily represent those of their affiliated organizations, or those of the publisher, the editors and the reviewers. Any product that may be evaluated in this article, or claim that may be made by its manufacturer, is not guaranteed or endorsed by the publisher.
Supplementary material
The Supplementary Material for this article can be found online at: https://www.frontiersin.org/articles/10.3389/fevo.2022.624896/full#supplementary-material
References
Alarcón, P. A. E., and Lambertucci, S. A. (2018). Pesticides thwart condor conservation. Science 360, 612–612. doi: 10.1126/science.aat6039
Allan, J. R., Watson, J. E. M., Di Marco, M., O’Bryan, C. J., Possingham, H. P., Atkinson, S. C., et al. (2019). Hotspots of human impact on threatened terrestrial vertebrates. PLoS Biol. 17:e3000158. doi: 10.1371/journal.pbio.3000158
Angelov, I., Hashim, I., and Oppel, S. (2013). Persistent electrocution mortality of Egyptian Vultures Neophron percnopterus over 28 years in East Africa. Bird Conserv. Int. 23, 1–6. doi: 10.1017/S0959270912000123
Auerbach, N. A., Wilson, K. A., Tulloch, A. I. T., Rhodes, J. R., Hanson, J. O., and Possingham, H. P. (2015). Effects of threat management interactions on conservation priorities. Conserv. Biol. 29, 1626–1635. doi: 10.1111/cobi.12551
Benítez-López, A., Alkemade, R., Schipper, A. M., Ingram, D. J., Verweij, P. A., Eikelboom, J. A. J., et al. (2017). The impact of hunting on tropical mammal and bird populations. Science 356, 180–183. doi: 10.1126/science.aaj1891
Bird, J. P., Martin, R., Akçakaya, H. R., Gilroy, J., Burfield, I. J., Garnett, S. T., et al. (2020). Generation lengths of the world’s birds and their implications for extinction risk. Conserv. Biol. 34, 1252–1261. doi: 10.1111/cobi.13486
BirdLife (2021). Birdlife International and Handbook of the Birds of the World: Bird species distribution maps of the world. Version 2021.1. Gland: IUCN.
Bowler, D. E., Bjorkman, A. D., Dornelas, M., Myers-Smith, I. H., Navarro, L. M., Niamir, A., et al. (2020). Mapping human pressures on biodiversity across the planet uncovers anthropogenic threat complexes. People Nat. 2, 380–394. doi: 10.1002/pan3.10071
Brambilla, M., Bergero, V., Bassi, E., and Falco, R. (2015). Current and future effectiveness of Natura 2000 network in the central Alps for the conservation of mountain forest owl species in a warming climate. Eur. J. Wildlife Res. 61, 35–44. doi: 10.1007/s10344-014-0864-6
Buechley, E. R., Santangeli, A., Girardello, M., Neate-Clegg, M. H. C., Oleyar, D., McClure, C. J. W., et al. (2019). Global raptor research and conservation priorities: Tropical raptors fall prey to knowledge gaps. Divers. Distrib. 25, 856–869. doi: 10.1111/ddi.12901
Buechley, E. R., and Şekercioğlu, ÇH. (2016). The avian scavenger crisis: looming extinctions, trophic cascades, and loss of critical ecosystem functions. Biol. Conserv. 198, 220–228. doi: 10.1016/j.biocon.2016.04.001
Canning, D. (1998). A Database of World Infrastructure Stocks, 1950-95. Washington, DC: World Bank. doi: 10.1596/1813-9450-1929
Carrete, M., Sánchez-Zapata, J. A., Benítez, J. R., Lobón, M., and Donázar, J. A. (2009a). Large scale risk-assessment of wind-farms on population viability of a globally endangered long-lived raptor. Biol. Conserv. 142, 2954–2961. doi: 10.1016/j.biocon.2009.07.027
Carrete, M., Tella, J. L., Blanco, G., and Bertellotti, M. (2009b). Effects of habitat degradation on the abundance, richness and diversity of raptors across Neotropical biomes. Biol. Conserv. 142, 2002–2011. doi: 10.1016/j.biocon.2009.02.012
Cattarino, L., Hermoso, V., Carwardine, J., Kennard, M. J., and Linke, S. (2015). Multi-action planning for threat management: a novel approach for the spatial prioritization of conservation actions. PLoS One 10:e0128027. doi: 10.1371/journal.pone.0128027
CMS (2014). Memorandum of Understanding on the Conservation of Migratory Birds of Prey in Africa and Eurasia. Baltimore, MA: Content management system.
Cutler, D. R., Edwards, T. C. Jr., Beard, K. H., Cutler, A., Hess, K. T., Gibson, J., et al. (2007). Random forests for classification in ecology. Ecology 88, 2783–2792. doi: 10.1890/07-0539.1
Delsen, D. M., Kissling, W. D., and Allan, J. R. (2020). Threatened terrestrial vertebrates are exposed to human pressures across 94% of Europe’s protected land. bioRxiv [Preprint]. doi: 10.1101/2020.08.25.266379
Di Marco, M., Watson, J. E. M., Possingham, H. P., and Venter, O. (2017). Limitations and trade-offs in the use of species distribution maps for protected area planning. J. Appl. Ecol. 54, 402–411. doi: 10.1111/1365-2664.12771
Dixon, A., Batbayar, N., Bold, B., Davaasuren, B., Erdenechimeg, T., Galtbalt, B., et al. (2020). Variation in electrocution rate and demographic composition of saker falcons electrocuted at power lines in mongolia. J. Raptor Res. 54:111. doi: 10.3356/0892-1016-54.2.136
DOE (2018). United States Department of Energy 2018 Wind Technologies Market Report. Office of Energy Efficiency and Renewable Energy. Washington, DC: United States Department of Energy.
Donázar, J. A., Cortés-Avizanda, A., Fargallo, J. A., Margalida, A., Moleón, M., Morales-Reyes, Z., et al. (2016). Roles of raptors in a changing world: from flagships to providers of key ecosystem services. Ardeola 63:154. doi: 10.13157/arla.63.1.2016.rp8
Efrat, R., Hatzofe, O., and Berger-Tal, O. (2020). Translating large-scale prioritization models for vultures to local-scale decision-making: response to Santangeli et al. 2019. Conserv. Biol. 34, 1305–1307. doi: 10.1111/cobi.13557
Evans, J. S., Murphy, M. A., Holden, Z. A., and Cushman, S. A. (2011). “Modeling Species Distribution and Change Using Random Forest,” in Predictive Species and Habitat Modeling in Landscape Ecology, eds C. Drew, Y. Wiersma, and F. Huettmann (New York, NY: Springer), doi: 10.1007/978-1-4419-7390-0_8
Gallego-Zamorano, J., Benítez-López, A., Santini, L., Hilbers, J. P., Huijbregts, M. A. J., and Schipper, A. M. (2020). Combined effects of land use and hunting on distributions of tropical mammals. Conserv. Biol. 34, 1271–1280. doi: 10.1111/cobi.13459
Gangoso, L., Agudo, R., Anadón, J. D., de la Riva, M., Suleyman, A. S., Porter, R., et al. (2013). Reinventing mutualism between humans and wild fauna: insights from vultures as ecosystem services providers. Conserv. Lett. 6, 172–179. doi: 10.1111/j.1755-263X.2012.00289.x
González, L. M., Margalida, A., Sánchez, R., and Oria, J. (2006). Supplementary feeding as an effective tool for improving breeding success in the Spanish imperial eagle (Aquila adalberti). Biol. Conserv. 129, 477–486. doi: 10.1016/j.biocon.2005.11.014
Hansen, M. C., Potapov, P. V., Moore, R., Hancher, M., Turubanova, S. A., Tyukavina, A., et al. (2013). High-resolution global maps of 21st-Century forest cover change. Science 342, 850–853. doi: 10.1126/science.1244693
Hothorn, T., Hornik, K., and Zeileis, A. (2006). Unbiased recursive partitioning: a conditional inference framework. J. Comput. Graph. Stat. 15, 651–674. doi: 10.1198/106186006X133933
Hulme, P. E. (2009). Trade, transport and trouble: managing invasive species pathways in an era of globalization. J. Appl. Ecol. 46, 10–18. doi: 10.1111/j.1365-2664.2008.01600.x
Hurlbert, A. H., and Jetz, W. (2007). Species richness, hotspots, and the scale dependence of range maps in ecology and conservation. Proc. Nat. Acad. Sci. U.S.A. 104, 13384–13389. doi: 10.1073/pnas.0704469104
Iriarte, J. A., Rivas-Fuenzalida, T., and Jaksic, F. (2019). Las Aves Rapaces de Chile. Santiago: Ocho Libros.
IUCN (2022). The IUCN red list of threatened species. Version 2022-1. Available online at: https://www.iucnredlist.org
Jenkins, C. N., Pimm, S. L., and Joppa, L. N. (2013). Global patterns of terrestrial vertebrate diversity and conservation. Proc. Nat. Acad. Sci. U.S.A. 110, E2602–E2610. doi: 10.1073/pnas.1302251110
Joppa, L. N., O’Connor, B., Visconti, P., Smith, C., Geldmann, J., Hoffmann, M., et al. (2016). Filling in biodiversity threat gaps. Science 352, 416–418. doi: 10.1126/science.aaf3565
Kennedy, C. M., Oakleaf, J. R., Theobald, D. M., Baruch-Mordo, S., and Kiesecker, J. (2019). Managing the middle: A shift in conservation priorities based on the global human modification gradient. Global Change Biol. 25, 811–826. doi: 10.1111/gcb.14549
Kilgo, J. C., Labisky, R. F., and Fritzen, D. E. (1998). Influences of hunting on the behavior of white-tailed deer: implications for conservation of the florida panther. Conserv. Biol. 12, 1359–1364. doi: 10.1111/j.1523-1739.1998.97223.x
Krone, O. (2018). “Lead poisoning in birds of prey,” in Birds of Prey, eds J. Sarasola, J. Grande, and J. Negro (Cham: Springer). doi: 10.1007/978-3-319-73745-4_11
Kross, S. M., Bourbour, R. P., and Martinico, B. L. (2016). Agricultural land use, barn owl diet, and vertebrate pest control implications. Agric. Ecosyst. Environ. 223, 167–174. doi: 10.1016/j.agee.2016.03.002
Kross, S. M., Tylianakis, J. M., and Nelson, X. J. (2012). Effects of introducing threatened falcons into vineyards on abundance of passeriformes and bird damage to grapes. Conserv. Biol. 26, 142–149. doi: 10.1111/j.1523-1739.2011.01756.x
Laurance, W. F., Croes, B. M., Tchignoumba, L., Lahm, S. A., Alonso, A., Lee, M. E., et al. (2006). Impacts of Roads and Hunting on Central African Rainforest Mammals. Conserv. Biol. 20, 1251–1261. doi: 10.1111/j.1523-1739.2006.00420.x
Lehman, R. N., Kennedy, P. L., and Savidge, J. A. (2007). The state of the art in raptor electrocution research: A global review. Biol. Conserv. 136, 159–174. doi: 10.1016/j.biocon.2006.09.015
Levin, N., Kark, S., and Crandall, D. (2015). Where have all the people gone? Enhancing global conservation using night lights and social media. Ecol. Applic. 25, 2153–2167. doi: 10.1890/15-0113.1
López-López, P., Ferrer, M., Madero, A., Casado, E., and McGrady, M. (2011). Solving man-induced large-scale conservation problems: the spanish imperial eagle and power lines. PLoS One 6:e17196. doi: 10.1371/journal.pone.0017196
Markandya, A., Taylor, T., Longo, A., Murty, M. N., Murty, S., and Dhavala, K. (2008). Counting the cost of vulture decline—An appraisal of the human health and other benefits of vultures in India. Ecol. Econ. 67, 194–204. doi: 10.1016/j.ecolecon.2008.04.020
May, R. F. (2015). A unifying framework for the underlying mechanisms of avian avoidance of wind turbines. Biol. Conserv. 190, 179–187. doi: 10.1016/j.biocon.2015.06.004
Maxwell, S. L., Fuller, R. F., Brooks, T. M., and Watson, J. E. M. (2016). Biodiversity: The ravages of guns nets and bulldozers. Nature 536, 143–145. doi: 10.1038/536143a
McClure, C. J. W., Anderson, D. L., Belthoff, J. R., Botha, A., Buechley, E. R., Buij, R., et al. (2021). Commentary: the past, present, and future of the global raptor impact network. J. Raptor Res. 55, 605–618. doi: 10.3356/JRR-21-13
McClure, C. J. W., Lepage, D., Dunn, L., Anderson, D. L., Schulwitz, S. E., Camacho, L., et al. (2020). Towards reconciliation of the four world bird lists: hotspots of disagreement in taxonomy of raptors. Proc. R. Soc. B Biol. Sci. 287:20200683. doi: 10.1098/rspb.2020.0683
McClure, C. J. W., Rolek, B. W., Hayes, T. I., Hayes, C. D., Thorstrom, R., Curti, M., et al. (2017). Successful enhancement of Ridgway’s Hawk populations through recruitment of translocated birds. Condor 119, 855–864. doi: 10.1650/CONDOR-17-77.1
McClure, C. J. W., and Rolek, B. W. (2020). Relative conservation status of bird orders with special attention to raptors. Front. Ecol. Evol. 8:593941. doi: 10.3389/fevo.2020.593941
McClure, C. J. W., Schulwitz, S. E., Anderson, D. L., Robinson, B. W., Mojica, E. K., Therrien, J.-F., et al. (2019). Commentary: defining raptors and birds of prey. J. Raptor Res. 53:412. doi: 10.3356/0892-1016-53.4.419
McClure, C. J. W., Westrip, J. R. S., Johnson, J. A., Schulwitz, S. E., Virani, M. Z., Davies, R., et al. (2018). State of the world’s raptors: distributions, threats, and conservation recommendations. Biol. Conserv. 227, 390–402. doi: 10.1016/j.biocon.2018.08.012
McGowan, P. J. K. (2016). Conservation: Mapping the terrestrial human footprint. Nature 537, 172–173. doi: 10.1038/537172a
Meunier, G., and Lavoie, C. (2012). Roads as corridors for invasive plant species: New evidence from smooth bedstraw (Galium mollugo). Invasive Plant Sci. Manag. 5, 92–100. doi: 10.1614/IPSM-D-11-00049.1
Miranda, E. B. P., Peres, C. A., Carvalho-Rocha, V., Miguel, B. V., Lormand, N., Huizinga, N., et al. (2021). Tropical deforestation induces thresholds of reproductive viability and habitat suitability in Earth’s largest eagles. Sci. Rep. 11:13048. doi: 10.1038/s41598-021-92372-z
O’Bryan, C. J., Holden, M. H., and Watson, J. E. M. (2019). The mesoscavenger release hypothesis and implications for ecosystem and human well-being. Ecol. Lett. 22, 1340–1348. doi: 10.1111/ele.13288
O’Bryan, C. J., Allan, J. R., Holden, M., Sanderson, C., Venter, O., Di Marco, M., et al. (2020). Intense human pressure is widespread across terrestrial vertebrate ranges. Glob. Ecol. Conserv. 21:e00882. doi: 10.1016/j.gecco.2019.e00882
O’Bryan, C. J., Braczkowski, A. R., Beyer, H. L., Carter, N. H., Watson, J. E. M., and McDonald-Madden, E. (2018). The contribution of predators and scavengers to human well-being. Nat. Ecol. Evol. 2, 229–236. doi: 10.1038/s41559-017-0421-2
Oaks, J. L., Gilbert, M., Virani, M. Z., Watson, R. T., Meteyer, C. U., Rideout, B. A., et al. (2004). Diclofenac residues as the cause of vulture population decline in Pakistan. Nature 427, 630–633. doi: 10.1038/nature02317
Ogada, D., Shaw, P., Beyers, R. L., Buij, R., Murn, C., Thiollay, J. M., et al. (2016). Another continental vulture crisis: africa’s vultures collapsing toward extinction. Conserv. Lett. 9, 89–97. doi: 10.1111/conl.12182
Ogada, D. L., Keesing, F., and Virani, M. Z. (2012a). Dropping dead: causes and consequences of vulture population declines worldwide. Ann. N. Y. Acad. Sci. 1249, 57–71. doi: 10.1111/j.1749-6632.2011.06293.x
Ogada, D. L., Torchin, M. E., Kinnaird, M. F., and Ezenwa, V. O. (2012b). Effects of vulture declines on facultative scavengers and potential implications for mammalian disease transmission. Conserv. Biol. 26, 453–460. doi: 10.1111/j.1523-1739.2012.01827.x
Olson, D. N., Dinerstein, E., Wikramanayake, E. D., Burgess, N., Powell, G. V., Underwood, E. C., et al. (2001). Terrestrial ecoregions of the world: a new map of life on Earth. Bioscience 51, 933–938. doi: 10.1641/0006-3568(2001)051[0933:TEOTWA]2.0.CO;2
Plaza, I. P., Blanco, G., and Lambertucci, S. A. (2020). Implications of bacterial, viral and mycotic microorganisms in vultures for wildlife conservation, ecosystem services and public health. IBIS 162, 1109–1124. doi: 10.1111/ibi.12865
Pyšek, P., Jarošík, V., Hulme, P. E., Kühn, I., Wild, J., Arianoutsou, M., et al. (2010). Disentangling the role of environmental and human pressures on biological invasions across Europe. Proc. Natl. Acad. Sci. U.S.A. 107, 12157–12162. doi: 10.1073/pnas.1002314107
Rehbein, J. A., Watson, J. E. M., Lane, J. L., Sonter, L. J., Venter, O., Atkinson, S. C., et al. (2020). Renewable energy development threatens many globally important biodiversity areas. Global Change Biol. 26, 3040–3051. doi: 10.1111/gcb.15067
Rinne, E., Holttinen, H., Kiviluoma, J., and Rissanen, S. (2018). Effects of turbine technology and land use on wind power resource potential. Nat. Energy 3, 494–500. doi: 10.1038/s41560-018-0137-9
Santangeli, A., Girardello, M., Buechley, E., Botha, A., Minin, E. D., and Moilanen, A. (2019a). Priority areas for conservation of old world vultures. Conserv. Biol. 33, 1056–1065. doi: 10.1111/cobi.13282
Santangeli, A., Girardello, M., Buechley, E. R., Eklund, J., and Phipps, W. L. (2019b). Navigating spaces for implementing raptor research and conservation under varying levels of violence and governance in the Global South. Biol. Conserv. 239:108212. doi: 10.1016/j.biocon.2019.108212
Sarasola, J., Grande, J., and Negro, J. (2018). Birds of Prey: Biology and conservation in the XXI century. Berlin: Springer. doi: 10.1007/978-3-319-73745-4
Sekercioglu, C. H. (2006). Increasing awareness of avian ecological function. Trends Ecol. Evol. 21, 464–471. doi: 10.1016/j.tree.2006.05.007
Şekercioğlu, ÇH., Daily, G. C., and Ehrlich, P. R. (2004). Ecosystem consequences of bird declines. Proc. Natl. Acad. Sci. U.S.A. 101, 18042–18047. doi: 10.1073/pnas.0408049101
Sergio, F., Caro, T., Brown, D., Clucas, B., Hunter, J., Ketchum, J., et al. (2008). Top predators as conservation tools: ecological rationale, assumptions, and efficacy. Annu. Rev. Ecol., Evol. Syst. 39, 1–19. doi: 10.1146/annurev.ecolsys.39.110707.173545
Speziale, K. L., and Lambertucci, S. A. (2013). The effect of introduced species on raptors. J. Raptor Res. 47:112. doi: 10.3356/JRR-12-00003.1
Stillfried, M., Belant, J. L., Svoboda, N. J., Beyer, D. E., and Kramer-Schadt, S. (2015). When top predators become prey: Black bears alter movement behaviour in response to hunting pressure. Behav. Process. 120, 30–39. doi: 10.1016/j.beproc.2015.08.003
Strobl, C., Hothorn, T., and Zeileis, A. (2009). Party on! a new, conditional variable importance measure for random forests available in the party package. R J. 2, 14–17. doi: 10.32614/RJ-2009-013
Symes, W. S., Edwards, D. P., Miettinen, J., Rheindt, F. E., and Carrasco, R. (2018). Combined impacts of deforestation and wildlife trade on tropical biodiversity are severely underestimated. Nat. Commun. 9:4052. doi: 10.1038/s41467-018-06579-2
Tabassum, A., Premalatha, M., Abbasi, T., and Abbasi, S. A. (2014). Wind energy: Increasing deployment, rising environmental concerns. Renew. Sustain. Energy Rev. 31, 270–288. doi: 10.1016/j.rser.2013.11.019
Thiollay, J.-M., and Meyburg, B. U. (1988). Forest fragmentation and the conservation of raptors: a survey on the island of Java. Biol. Conserv. 44, 229–250. doi: 10.1016/0006-3207(88)90018-3
Trombulak, S. C., and Frissell, C. A. (2000). Review of ecological effects of roads on terrestrial and aquatic communities. Conserv. Biol. 14, 18–30. doi: 10.1046/j.1523-1739.2000.99084.x
Tulloch, V. J. D., Tulloch, A. I. T., Visconti, P., Halpern, B. S., Watson, J. E. M., Evans, M. C., et al. (2015). Why do we map threats? Linking threat mapping with actions to make better conservation decisions. Front. Ecol. Environ. 13:140022. doi: 10.1890/140022
Venter, O., Sanderson, E. W., Magrach, A., Allan, J. R., Beher, J., Jones, K. R., et al. (2016b). Sixteen years of change in the global terrestrial human footprint and implications for biodiversity conservation. Nat. Commun. 7:12558. doi: 10.1038/ncomms12558
Venter, O., Sanderson, E. W., Magrach, A., Allan, J. R., Beher, J., Jones, K. R., et al. (2016a). Global terrestrial Human Footprint maps for 1993 and 2009. Sci. Data 3:160067. doi: 10.1038/sdata.2016.67
Watson, R. T. (2018). “Raptor Conservation in Practice,” in Birds of Prey, eds J. Sarasola, J. Grande, and J. Negro (Berlin: Springer). doi: 10.1007/978-3-319-73745-4_20
Wilman, H., Belmaker, J., Simpson, J., de la Rosa, C., Rivadeneira, M. M., and Jetz, W. (2014). EltonTraits 1.0: species-level foraging attributes of the world’s birds and mammals. Ecology 95, 2027–2027. doi: 10.1890/13-1917.1
Keywords: biodiversity conservation, conservation planning, extinction risk, human footprint, human pressure, macroecology, species distribution, threat mapping
Citation: O’Bryan CJ, Allan JR, Suarez-Castro AF, Delsen DM, Buij R, McClure CJW, Rehbein JA, Virani MZ, McCabe JD, Tyrrell P, Negret PJ, Greig C, Brehony P and Kissling WD (2022) Human impacts on the world’s raptors. Front. Ecol. Evol. 10:624896. doi: 10.3389/fevo.2022.624896
Received: 01 November 2020; Accepted: 29 July 2022;
Published: 13 September 2022.
Edited by:
Çagan H. Sekercioglu, The University of Utah, United StatesReviewed by:
Neil Paprocki, University of Idaho, United StatesLouis Phipps, Independent Researcher, Northamptonshire, United Kingdom
Copyright © 2022 O’Bryan, Allan, Suarez-Castro, Delsen, Buij, McClure, Rehbein, Virani, McCabe, Tyrrell, Negret, Greig, Brehony and Kissling. This is an open-access article distributed under the terms of the Creative Commons Attribution License (CC BY). The use, distribution or reproduction in other forums is permitted, provided the original author(s) and the copyright owner(s) are credited and that the original publication in this journal is cited, in accordance with accepted academic practice. No use, distribution or reproduction is permitted which does not comply with these terms.
*Correspondence: Christopher J. O’Bryan, Yy5vYnJ5YW5AdXEuZWR1LmF1