- 1Department of Biological Sciences, Institute of Environment, Florida International University, North Miami, FL, United States
- 2Department of Biology and Marine Biology, University of North Carolina, Wilmington, Wilmington, NC, United States
- 3Department of Life Sciences, The Natural History Museum, London, United Kingdom
- 4Department of Biology, The University of Texas at Arlington, Arlington, TX, United States
- 5Department of Biology, Tennessee Tech University, Cookeville, TN, United States
Constraints on energy resources and available light in the deep sea should place strong selection pressure on eye size, a fundamental determinant of visual ability. By examining eye size among 16 species (454 individuals) of deep-sea sergestid shrimps, we show significant differences in intraspecific eye growth rates and species eye-size averages that are correlated to different aspects of ecology and result in variable sighting distance thresholds of bioluminescence, one measure of visual performance. We used linear regressions modeling the lowest and highest bounds of phylogenetic signal to test for ecological correlates of relative and absolute eye size, which indicate the allocation of energetic resources toward eyes and an optical basis of visual capability, respectively. Of the ecological variables tested [mean depth, diel vertical migration (DVM) distance, habitat type, and light organ type], light organ type was the only significant correlate of both relative and absolute eye size, suggesting that bioluminescence plays a particularly important role in the evolution of sergestid vision and that these animals may be reliant on bioluminescent signaling. Our findings also suggest that the DVM imposes visual demands distinct from the average depths occupied by a species. While DVM distance correlated with relative eye size, mean depth correlated with absolute eye size, revealing that eye size increases with depth before 1,000 m, then decreases in bathypelagic (aphotic) zone. By applying measured eye sizes to models of visual performance, we estimated that sergestids can detect a bioluminescent point source from ≤3.77 m away, and that these sighting distance thresholds vary between species by a factor of three. In relative terms, however, all sergestids under the test conditions had a common detection threshold at ∼63.5 body lengths, suggesting that bioluminescence sighting distance is proportional among species and may be related to shared behaviors of swarming and copulation. By considering the effects of evolutionary history, light and nutrient availability, and the constraints of body size, our study reveals new patterns of deep-sea eye size evolution and provides new insights into the visual ecology of this diverse and important deep-sea group.
Introduction
The deep sea (>200 m) is one of the most light-limited environments on earth, yet for many of its animals, vision is nevertheless critical for survival. Vision in the deep sea can help animals to gauge depth, find prey, avoid predators, and identify mates, requiring eyes that are adapted to distinct sources of light. In this realm, the visual world consists primarily of scenes illuminated by dim, diffuse downwelling sunlight or of pinpoint flashes of bioluminescence (Warrant and Locket, 2004). In clear water, the intensity of sunlight attenuates exponentially with depth, penetrating no deeper than ∼1,000 m even on the brightest day (Denton, 1990). Until this point, sunlight and bioluminescence are coincident, while in the bathypelagic zone (>1,000 m) only bioluminescence remains. Both sunlight and bioluminescence can have individual or synergistic effects on the evolution of vision, and thus unsurprisingly, midwater animals inhabiting the mesopelagic zone from 200 to 1,000 m have the most variable eye morphologies in the deep sea (Warrant and Locket, 2004). In addition to light limitations, energy limitations also characterize this environment (Rex and Etter, 1998), with reduced nutrient availability predicted to place constraints on the growth and maintenance of eyes (Warrant and Locket, 2004). Together these factors – the critical role of vision, discrete light sources, and energy limitations – place selection pressure on eyes, making the deep sea a natural laboratory for investigating the ecological factors that underlie the evolution of vision.
Visual function is primarily summarized by two features – acuity and sensitivity – which are the resolution of detail and number of photons that eyes can detect, respectively (Land and Nilsson, 2012). There are many neural and optical strategies for improving these features, but one fundamental strategy is increasing eye size (Warrant, 1999, 2000). Larger eyes tend to have larger apertures and longer focal lengths, which can improve light capture and the resolution of vision (Land and Nilsson, 2012). To capitalize on this information, a larger eye typically has more photoreceptor cells to improve resolution or larger photoreceptor cells to improve sensitivity, with the latter being particularly advantageous to animals in light-limited environments like the deep sea. Regardless of photoreceptive strategy, increasing the amount of photoreceptive material in the eye results in a greater demand for ATP (Laughlin et al., 1998; Niven and Laughlin, 2008). Thus, a conflict exists between having eyes large enough for suitable performance yet small enough to support metabolically (Warrant, 2000). In the deep sea, where sources of light and energy are limited (Warrant and Locket, 2004), the tension between performance and cost is exaggerated, likely placing tremendous selection pressure on the rate of eye growth and ultimate eye size.
In general, larger eyes are better at detecting both dim downwelling sunlight and pinpoints of bioluminescence due to improved sensitivity and resolution of vision, respectively (Warrant and Locket, 2004). Therefore, eye size in the deep sea is expected to vary with ecological factors that affect the amount and relative importance of each source of light. For example, we might predict larger eyes in animals that occupy (or migrate to) greater depths where sunlight becomes dimmer and more difficult to detect (Warrant, 2000). Regardless of depth, animals that are active near the nutrient-rich sea floor (benthopelagic animals) generally have larger eyes, which would improve their capacity to detect dim sunlight or pinpoints of bioluminescence, the dominant source of light in deep-sea benthopelagic habitats (Warrant and Locket, 2004). We might also expect larger eye sizes in animals that rely on bioluminescent signaling (Cohen and Morin, 2010), which is used by some animals to find mates, avoid predators, and capture prey.
These eye size trends are predicted in deep-sea ecology regardless of the kind of animal or its type of eye. Whereas fishes have simple camera eyes, deep-sea crustaceans often have superposition compound eyes (Cronin et al., 2014). For both eye types, vision can be improved by increasing eye size, yet to resolve the same bioluminescent source, crustaceans might require larger eyes than fishes because the diffraction limits of compound eye lenses restrict visual resolution (Warrant and McIntyre, 1990). Despite similar predictions for these different eye types, findings on the relationship between eye size and ecology have been mixed. Among families of deep-sea fishes, camera-type eye size is known either to increase with depth (until the bathypelagic; Warrant, 2000), decrease with depth (family Gonostomatidae; Marshall, 1954), or to vary widely with no consistent relationship to depth distribution at all (family Myctophidae; de Busserolles et al., 2013). By contrast, in some crustacean families, relative compound eye size decreases in species occupying greater depths (Hiller-Adams and Case, 1988). In regard to bioluminescence, deep-sea myctophid fishes show no correlation between camera-type eye size and the presence of sexually dimorphic light organs, which are presumably used for sexual signaling (de Busserolles et al., 2013). Among at least two families of deep-sea crustaceans (Oplophoridae and Sergestidae), however, reduced compound eye size is observed in species lacking light organs (Hiller-Adams and Case, 1988), suggesting a possible relationship between eye size and conspecific signaling.
Together, these studies indicate that eye size ecology in the deep sea is complex and requires further study to disentangle the factors that underlie its evolution. Therefore, the goal of this study was to further investigate the ecological basis and functional implications of eye size while considering allometric scaling with body size and phylogenetic history – two intrinsic factors that can constrain eye size. Specifically, eye size depends largely on body size, as a minimum body size is required to support the given eye size of an animal. Thus, our study was conducted in the context of allometry, examining both relative eye size (i.e., corrected for body size) as well as absolute eye size. Whereas relative eye size provides a physiological metric representing the amount of energetic resources allocated to vision, absolute eye size provides an optical metric that represents the absolute geometry of an eye underlying its visual capability. Here, we conducted a comparative study of deep-sea shrimps from the family Sergestidae, as this study system has substantial eye size variation, an established molecular phylogeny (Golightly et al., 2022), and well-documented deep-sea ecology (Vereshchaka, 2000, 2009).
Sergestids occur at diverse depth ranges that span from near the surface to over 2,000 m. Like other deep-sea fauna, sergestids undergo the diel vertical migration (DVM), occupying deep waters (e.g., the mesopelagic zone) during the day, then migrating up toward the surface (e.g., the epipelagic zone, <200 m) at night in order to mate and feed (Flock and Hopkins, 1992). The extent of the DVM varies, with some species exhibiting weak DVM activity and migrating distances of 200 m between day and night, and others traversing distances over 1,000 m. Further, sergestid species occupy different habitats during the day, with some always found in the pelagic realm, and others commonly found in benthopelagic habitats near the seafloor.
Some of these ecological differences occur between the two major groups of sergestids (Vereshchaka, 2000, 2009): the -sergestes group (represented here by Deosergestes, Parasergestes, Allosergestes, Eusergestes, Sergestes, and Neosergestes) and the -sergia group (represented here by Challengerosergia, Gardinerosergia, Robustosergia, and Sergia). Members of the -sergestes group generally occupy shallower waters than members of the -sergia group. In addition, these groups differ in the presence and morphology of bioluminescent light organs. The -sergestes have large luminescent organs stemming from modified regions of hepatopancreas known as “organs of Pesta,” whereas the -sergia have much smaller, and putatively dimmer, dermal photophores that are either lensed or unlensed, or have no photophores at all (Foxton, 1972; Vereshchaka et al., 2014).
The diversity of sergestid light organ sizes and optics suggest that the relative brightness and visual discriminability of these organs vary across species, which might also drive eye size evolution – a prediction that is only valid if these organs function in conspecific signaling. Though conspecific signaling is difficult to study in the deep sea, several lines of evidence suggest that signaling among sergestids is possible. Among some sergestids (and members of the family Oplophoridae), species with light organs have larger eyes than those lacking light organs (Hiller-Adams and Case, 1988). Further, certain -sergia species show sexual dimorphism in light organ patterns (Herring, 2000). Finally, models of maximum sighting distances for bioluminescence suggest that at least some -sergestes have the ability to detect intraspecific bioluminescence over distances relevant for conspecific signaling (Schweikert et al., 2020).
In this study, we set out to explore the ecological basis and functional significance of eye size in sergestids. We examined eye diameters and body lengths of 16 sergestid species (454 adult individuals) to address three central questions: (1) how does eye size scale with body size within and across species?; (2) what ecological factors might place selective pressure on species eye size?; and (3) how does variation in eye size affect visual performance? To that end, we investigated differences in intraspecific adult eye-body allometry (i.e., static allometry) across species. Then, we examined average eye-body allometry across species (i.e., evolutionary allometry) to test how four ecological factors (depth, DVM distance, habitat, and light organ type) correlate to absolute and relative eye size (eye size corrected for body size). Because our results indicated that intraspecific bioluminescence is likely an important correlate of both relative and absolute eye size, we then used computational modeling of best-case maximum visual ranges for the detection of bioluminescent point-source light to see how this varied and scaled across species.
Materials and Methods
Sampling
Animals were obtained from two deep-sea research expeditions and from the Florida International Crustacean Collection (FICC) at Florida International University, with all animals originating from the Gulf of Mexico and Florida Straits. The first expedition occurred in the Florida Straits on May 4–8, 2019 on the R/V Weatherbird II, and the second occurred in the northern Gulf of Mexico on June 9–22, 2019 as part of a NOAA Ocean Research Exploration expedition on the R/V Point Sur. Animals were captured by a 1- or 9-m2 tucker trawl over sampling that occurred both day and night over a total range of depths from 150 to 1,500 m. Animals from the first expedition (n = 49) were measured after fixation in 4% paraformaldehyde in 0.1 M phosphate-buffered saline (PBS; Boston BioProducts, Ashland, MA, United States) for 48-h prior to transfer into 0.1 M PBS, and those from the second expedition (n = 193) were measured fresh prior to fixation. The animals sampled from the FICC (n = 213) were collected during eight additional research cruises in the northern Gulf of Mexico or Florida Straits between 2015 and 2017 and were preserved in ∼70% EtOH prior to measurement. Fixation and storage in ethanol are known to change the size and shape of some soft tissues (Eltoum et al., 2013). As many studies of eye size evolution use museum specimens, sometimes in combination with fresh specimens, fixation method is a likely source of error in eye and body size measurements. Thus in our study, we tested for an effect of preservation method in our data and tried to account for such effects using approaches outlined below.
Measurements of eye diameter and body length (mm, ±0.1 mm resolution) were collected using Mitutoyo CD-8 ASX Digimatic Calipers (Mitutoyo Corporation, Kanagawa, Japan). Sergestids have reflecting superposition eyes that are roughly spherical in shape. Following Hiller-Adams and Case (1988), eye diameter was measured at the maximum distance found perpendicular to the dorsoventral eye axis, and body length was measured from the posterior limit of the eye orbit to the end of the telson. Carapace length (CL) was also measured, taken from the posterior limit of the eye orbit to the end of the carapace. Data were collected from 454 individuals across 16 species, with a median sample size of 29 individuals per species (Table 1). Plotting the data revealed three large outliers among the 454 measurements, which were removed from the dataset prior to subsequent analyses.
Our data represent shrimp in the adult stage of their ontogenetic development as estimated by CL and the observation of mature reproductive structures during species identification. A previous survey of sergestids estimated that adulthood is reached at 4 mm CL for most species and 11 mm CL in at least one species, Robustosergia robusta (Flock and Hopkins, 1992). Of the 454 animals sampled here, only two individuals had a CL < 4 mm (Parasergestes vigilax at 3.8 and 3.9 CL) and all R. robusta sampled had a CL > 11 mm. Thus, our data centers on mature specimens and should not capture allometric variation that might occur between ontogenetic stages. The species means of eye and body size analyzed here, therefore, capture variation that exists across adults of different sizes.
Ecological Data and Phylogeny
Using published literature, we classified each species in our dataset to test four ecological correlates of sergestid eye size: depth, DVM distance, habitat, and light organ type (Table 1). Classifying the ecology of deep-sea fauna can be sensitive to variation in sampling effort. Thus, to reduce error, we only included specimens captured in and around the Gulf of Mexico and, where possible, obtained ecological classifications from Gulf of Mexico studies. To obtain valid depth estimates, we selected three representative metrics: mean daytime depth, mean nighttime depth, and maximum reported depth. For twelve species, we calculated weighted averages for daytime and nighttime depths by factoring in species abundance across depth using a robust study of sergestid depth distributions (Flock and Hopkins, 1992; Table 1) using the program, GetData v0.10.0 (GetData, 2017). For the remaining four species – P. grandis, R. regalis, S. tenuriemis, and C. hansjacobi – abundance data were unavailable, thus reported mean daytime and nighttime depths were taken from Vereshchaka (2000). Maximum reported depths were obtained broadly across the sergestid literature (Table 1). For the three remaining hypotheses, we examined mean DVM distance (calculated as mean daytime minus nighttime depth), primary habitat type (pelagic or benthopelagic), and light organ type (lensed photophores, unlensed photophores, organs of Pesta, or no light organs). For the light organ analyses, we grouped species with unlensed and lensed photophores (n = 6 spp.) together to increase power, separate from those having organs of Pesta (n = 9 spp.), or no light organs at all (n = 1 spp.; Table 1).
For analyses incorporating species evolutionary history, we generated a subtree of sampled species (n = 16) from a recently published molecular phylogeny for Sergestidae (Golightly et al., 2022) using ape v.5.3 in R (Paradis et al., 2004). Though this published molecular phylogeny does not include all species of sergestids (15 major genera and 74 valid species worldwide; Vereshchaka et al., 2016), it does include 13 of the 15 genera and all genera present within the Gulf of Mexico.
Static Allometry: Intraspecific Eye-to-Body Size Scaling
To better understand how eye size scales with body size within and across sergestids, we examined adult eye-body allometry across the 16 species. While literature is divided on the best form of regression for allometry (e.g., Warton et al., 2006; Smith, 2009; Kilmer and Rodriquez, 2017), ordinary least squares (OLS) and standardized major axis (SMA) regression typically give similar results for eye-body allometry (Shrimpton et al., 2021). Following Kilmer and Rodriquez (2017), we fit preliminary standardized major axis (SMA) regressions to log-transformed eye diameter vs. body length for each species using smatr v.3.4.8 (Warton et al., 2012). This allowed us to assess which species had sufficient data for subsequent analyses and revealed intraspecific allometric slopes. Allometric slopes (β) with 95% confidence intervals that were <1, >1, or included 1 indicated either negative, positive, or isometric scaling, respectively (Supplementary Table 1). Negative allometry indicated that eyes grow slower than the body, positive allometry indicated that eyes grow faster than the body, and isometry indicated equal growth rates of the eyes and body. Neosergestes edwardsii (n = 7) was found to have insufficient data to produce a significant correlation between eye and body size and thus, was excluded from two subsequent approaches testing whether sergestid species differed from one another in adult eye-body allometric slope.
To perform pairwise comparisons of allometric slopes, we fit a SMA regression of log-transformed eye diameter vs. body length with species as a covariate for all species except N. edwardsii (n = 15). Pairwise comparisons of allometric slopes between species were adjusted with a Šidák correction, and alpha was set to 0.05 for corrected p-values. However, because this SMA approach treats species as independent and unrelated, we also employed a mixed-model approach to compare adult allometries, as these can account for non-independent data structures (Harrison et al., 2018). Thus, in a second analysis, we used linear mixed models (LMM) in the lme4 v.1.1.25 package (Bates et al., 2015) to fit log eye diameter as the response variable, log body length as a fixed effect, and species identity as a random effect (Table 2). Following Firmat et al. (2014) and Shrimpton et al. (2021), we ran two separate models fitted with restricted maximum likelihood (REML): a variable intercepts model assuming a common allometric slope among species and a variable slopes model allowing different slopes and intercepts among species. Model fits were then evaluated using Akaike’s information criterion (AIC) scores (Burnham et al., 2011).
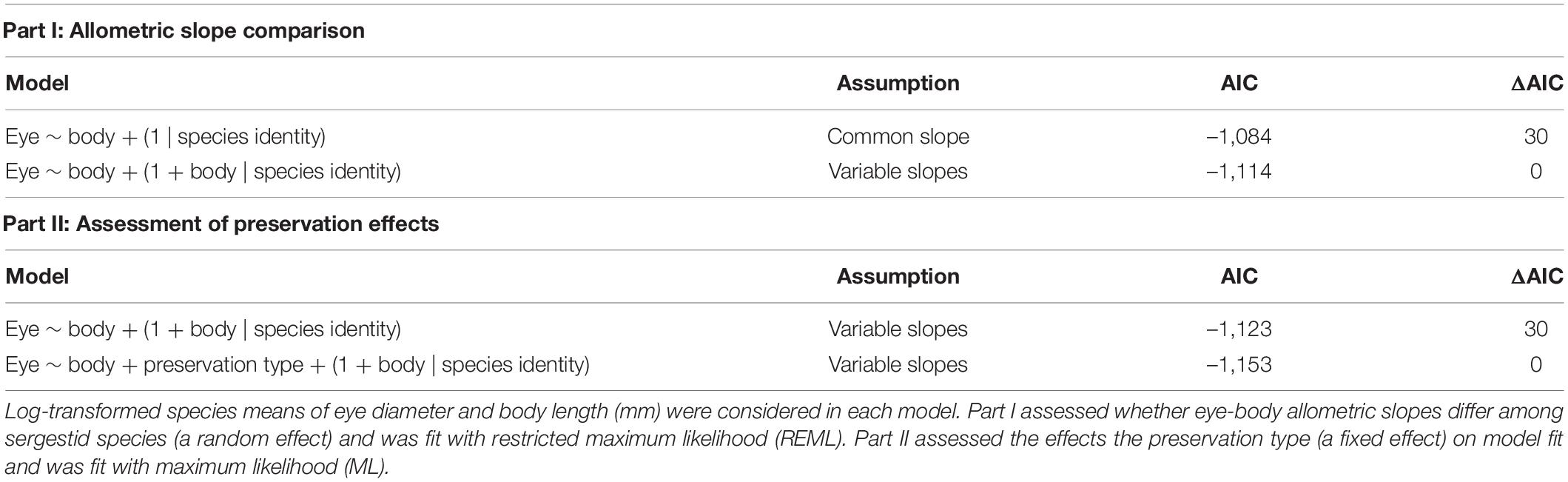
Table 2. Comparison of linear mixed models of sergestid eye-body allometry using Akaike’s information criterion (AIC).
Finally, because some specimens were measured fresh, while others were fixed in paraformaldehyde or stored in EtOH, we also explored whether including preservation type improved the fit of linear mixed models of allometry. The outputs of the previous LMM analyses indicated a better fit of the variable-slopes model than the fixed-slopes model (Table 2). Thus, to test the effects of preservation type on model fit, we used maximum likelihood (ML) to fit two variable-slopes models that either (1) excluded preservation type or (2) included preservation type as a fixed effect and compared model fits using AIC. Preservation type was assigned as a fixed effect because it only had three levels, while random effects should have at least five levels (Harrison et al., 2018). Finally, as preservation type was shown to have a significant effect on model fit, we ran a set of representative analyses to test whether preservation type had significantly impacted the study conclusions. As paraformaldehyde had a greater effect than EtOH on eye-body allometry, we removed paraformaldehyde-fixed specimens from the dataset and reproduced the individual SMA regressions and the LMM comparison of slopes.
The static allometry analyses therefore, were comprised of four sets of linear models: (1) individual SMA regressions for each species (n = 16 species; Supplementary Table 1); (2) a cross-species SMA regression yielding pairwise comparisons of allometric slopes (n = 15; Supplementary Table 2); (3) LMM comparison of slopes treating species as a random effect (n = 15; Table 2); and (4) LMM testing of preservation effects (n = 15; Table 2) and a reproduction of SMA and LMM analyses for data validation (Supplemental Material). The number of models, their composition, and results are summarized in the tables listed above.
Evolutionary Allometry: Ecological Correlates of Relative and Absolute Eye Size
Next, we investigated the evolutionary allometry of sergestid eyes, examining how average eye size scales with body size among all sampled species (n = 16). Despite the differences observed in adult eye-body static allometry, species means of eye and body sizes were used for evolutionary allometry. This approach followed previous studies of eye-body allometry (e.g., Howland et al., 2004), allowing us to capture variation that exists across differently-sized adults. Here, we used phylogenetic comparative methods to account for the non-independence of data from closely related species. We fit a phylogenetic generalized least squares (PGLS) regression to log-transformed species means of eye diameter vs. body length in caper v.1.0.1, which typically uses maximum likelihood to estimate the phylogenetic signal of model residuals (λ; Pagel, 1999). However, λ cannot be reliably estimated for sample sizes of less than 20 species (Freckleton et al., 2002); therefore, we ran two iterations of this PGLS regression to represent bounded extremes of λ: one with λ fixed at 0 (λ0, no phylogenetic signal in residuals and potential independent evolution along branches) and one with λ fixed at 1 (λ1, high phylogenetic signal and covariance as expected under Brownian motion). This approach bookends the true value of λ and is appropriate for small sample sizes (Kamilar and Cooper, 2013). Significant effects maintained at both λ0 and λ1 indicate that patterns are likely robust, regardless of what the true value of λ is. Residuals from the fitted regression (at λ1) were examined and plotted as measure of “eye size investment,” with positive and negative residuals indicating how large or small an eye is for a given body size compared to what is predicted from the regression for evolutionary eye-body allometry.
We then used the same approach to test for ecological correlates of relative eye size. We conducted separate PGLS regressions of log-transformed species means of eye diameter vs. body length with the following covariates: (i) daytime × nighttime depth; (ii) maximum reported depth; (iii) DVM distance; (iv) habitat type; and (v) light organ type. These ecological variables were tested across separate PGLS models rather than building one additive model to preserve statistical power given the high ratio of ecological states to the number of species sampled. Again, each model was fit twice, first at λ0 and then at λ1.
While it is important to consider eye size in the context of body size (i.e., relative eye size), absolute eye size sets the optical aperture for light detection and has direct functional implications for vision. Thus, we also used a PGLS approach in caper to run linear regressions of eye diameter (unlogged and uncorrected for body size) vs. the ecological covariates. Again, these covariates were tested across separate PGLS regressions as outlined above to avoid overparameterization, and each was run in two models with λ fixed at 0 and 1.
Plotting of eye diameter against day and night depths suggested that these relationships may change at transitions from the epipelagic (0–200 m) to the mesopelagic (200–1,000 m) zone and from the mesopelagic to the bathypelagic (>1,000 m) zone. To explore this possibility, we ran three additional sets (λ fixed at 0 and 1) of PGLS models (Supplementary Tables 3–5), examining (i) eye diameter vs. daytime depth × zone (mesopelagic or bathypelagic), (ii) body length vs. daytime depth × zone (mesopelagic or bathypelagic), and (iii) eye diameter vs. nighttime zone (epipelagic or mesopelagic). Nighttime depth was excluded from the third analysis (eye diameter vs. nighttime zone) as the data showed no linear relationship between eye diameter and nighttime depth, but a potential difference in mean eye diameter between the epipelagic and mesopelagic zones.
The evolutionary allometry analyses therefore, were comprised of four sets of linear models each run in two iterations at bounded extremes of λ (λ0 and λ1): (1) an initial PGLS analysis of average eye-body allometry across sergestids (n = 16 species; Table 3), (2) a set of PGLS analyses testing the relationships between each ecological variable and relative eye size (n = 16; Table 3), (3) a set of PGLS analyses testing the relationships between each ecological variable and absolute eye size (n = 16; Table 4) and (4) a set of PGLS analyses testing relationships between eye size, body size, and oceanic zones as outlined above (n = 16; Supplementary Tables 3–5). The number of models, their composition, and results are summarized in the tables listed above.
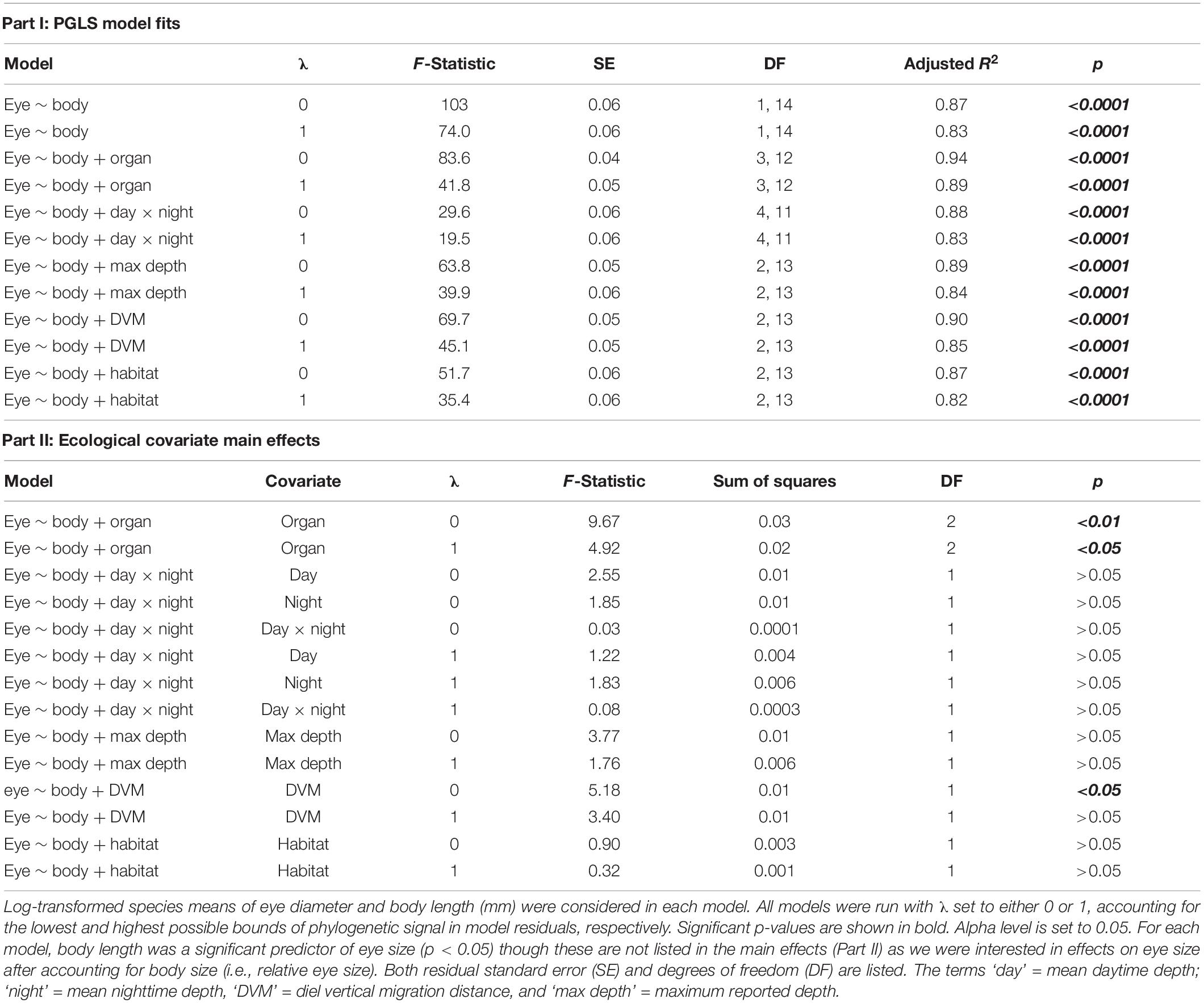
Table 3. Summary of phylogenetic generalized least squares (PGLS) models of relative eye size in sergestid shrimps.
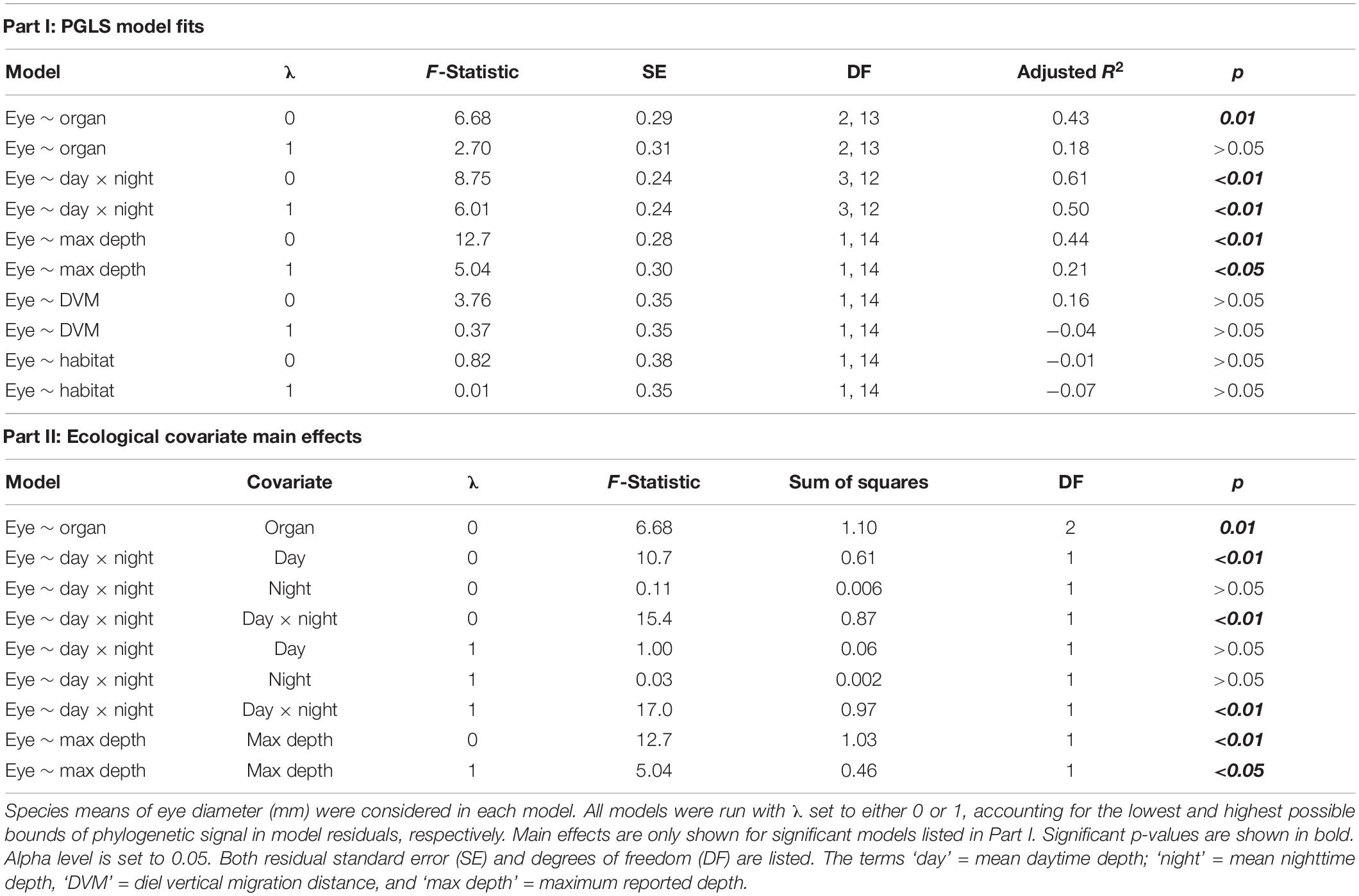
Table 4. Summary of phylogenetic generalized least squares (PGLS) models of absolute eye size in sergestid shrimps.
Sighting Distance Modeling
Finally, to examine the implications for visual function, we modeled the maximum distance at which each species might detect a bioluminescent point source of light based on species means for absolute eye size. A point source is defined as the light detected from a singular point in space because the angular size of the stimulus is below the resolution limit of a given animal’s vision (Johnsen, 2012). Following Schweikert et al. (2020), bioluminescence sighting distances were modeled given a hypothetical situation of each species observing a bioluminescent point source of light under the same environmental conditions. The actual conditions under which sergestids might visualize one another’s bioluminescence remains unknown. However, as the sergestids tested here are sympatric and overlap in depth distributions (Felder et al., 2009), it is not unreasonable that different species might encounter a similar visual scenario in situ. Holding all parameters constant except absolute eye size allowed us to assess the functional implications of eye size for this group.
We used an approach originally developed by Nilsson et al. (2014), and then modified by Ruxton and Johnsen (2016), that uses information on eye aperture, retinal physiology (i.e., integration rate), environmental light, and the optical properties of a visual target to estimate the maximum distance at which sergestids might detect a bioluminescent point source. In each model, we set ambient light conditions to absolute darkness in order to approximate conditions in the bathypelagic zone or shallower water during a new moon phase. This approach yields a best-case scenario (and thus, maximum distance) that sergestids might visualize a point source of light. Previous work has suggested that sergestid vision is monochromatic and spectrally sensitive to the blue-light emissions of their own bioluminescence (Frank and Widder, 1999; Lindsay et al., 1999). Thus, for ecological relevance, we set the bioluminescent point source as an emission from a sergestid found sympatrically to the species examined here (Vereshchaka et al., 2014). Information on the emission intensities of sergestid bioluminescence is limited, but at least one report by Lindsay et al. (1999) estimated the maximum emission intensity of Sergestes similis as ∼19 × 1010 photons/s, which is a reasonable estimate contained within the range of emissions intensities previously reported across deep-sea fauna (108–1011 photons/s; Mensinger and Case, 1990; Herring, 2000).
The sighting distance model for a bioluminescent point source is shown below (for full derivation see Schweikert et al., 2020):
Sighting distance (r) is a function of the beam attenuation coefficient of water at 480 nm (c), aperture diameter (A), photoreceptor diameter (d), emittance of the bioluminescent point source (E), integration time of the eye (Δt), and the photons absorbed per second for a one-meter-wide aperture in the sighting direction of interest (N0), which is set to zero because we are considering water without ambient light. The Lambert-W function (the inverse function of y = xex) is represented by W. All parameter values for the model were derived from the published literature and were held constant across species, except for aperture diameter (A) representing original data from this study. The photons absorbed per second from the background light was set to zero. The beam attenuation coefficient was assumed to be constant for depths > 200 m and was taken from Ruxton and Johnsen (2016). Photoreceptor diameter was set to 3 μm (Land and Nilsson, 2012), and because aperture diameter is roughly equivalent to eye diameter in sergestid superposition eyes (Hiller-Adams and Case, 1988), aperture diameter was set to the mean eye diameter for each species. Integration time for all species was set to 0.05 s, which was calculated using the average of known critical flicker fusion rate of shrimps in the family Sergestidae (Frank, 2000).
Outputs of the sighting distance models (i.e., absolute sighting distances) are reported in meters. To better understand the significance of these sighting distances to each species, we converted absolute sighting distance (m) to a relative measure of sighting distance in number of mean body lengths for a given species. Finally, we fit an OLS regression of relative sighting distance vs. body length to better understand how sighting distance scales with species size. As the output of this OLS regression was nonsignificant, showing no support for a change in relative sighting distance with species size, we then averaged bioluminescence sighting distance to better understand how this aspect of visual performance is shared among sergestids.
Data Analysis and Reproducibility
Data were analyzed with R 4.0.2 (R Core Team, 2020) in RStudio 1.3.1093 (RStudio Team, 2020). Morphological data are available in the Supplemental Excel File 1, and code to reproduce all analyses and diagnostic checks of regressions are annotated in RMarkdown and available on GitHub.1 Examination of model diagnostics indicated slight bimodality of the residuals in some PGLS regressions, but no obvious outliers of these residuals were found.
Results
Static Allometry: Intraspecific Eye-to-Body Size Scaling
Standardized major axis regressions showed significant eye-body allometry for all species except N. edwardsii (Supplementary Table 1). Across species, allometric slope estimates ranged substantially from 0.8 in Sergia tenuiremis to 2.38 in P. vigilax (Figure 1). Species from the -sergia group generally had slopes less than 1 (eyes that grow slower than the body), whereas those from the -sergestes group generally had slopes greater than 1 (eyes that grow faster than the body; Figure 1 and Supplementary Table 1). However, only four (-sergestes) species had significantly non-isometric eye-body allometry where 95% confidence intervals of β excluded 1: P. vigilax, Sergestes atlanticus, Allosergestes sargassi, and Allosergestes pectinatus. The slopes of these four species were all greater than 1, ranging from 1.84 to 2.38, indicating a significantly faster growth of the eye relative to the body for these -sergestes species.
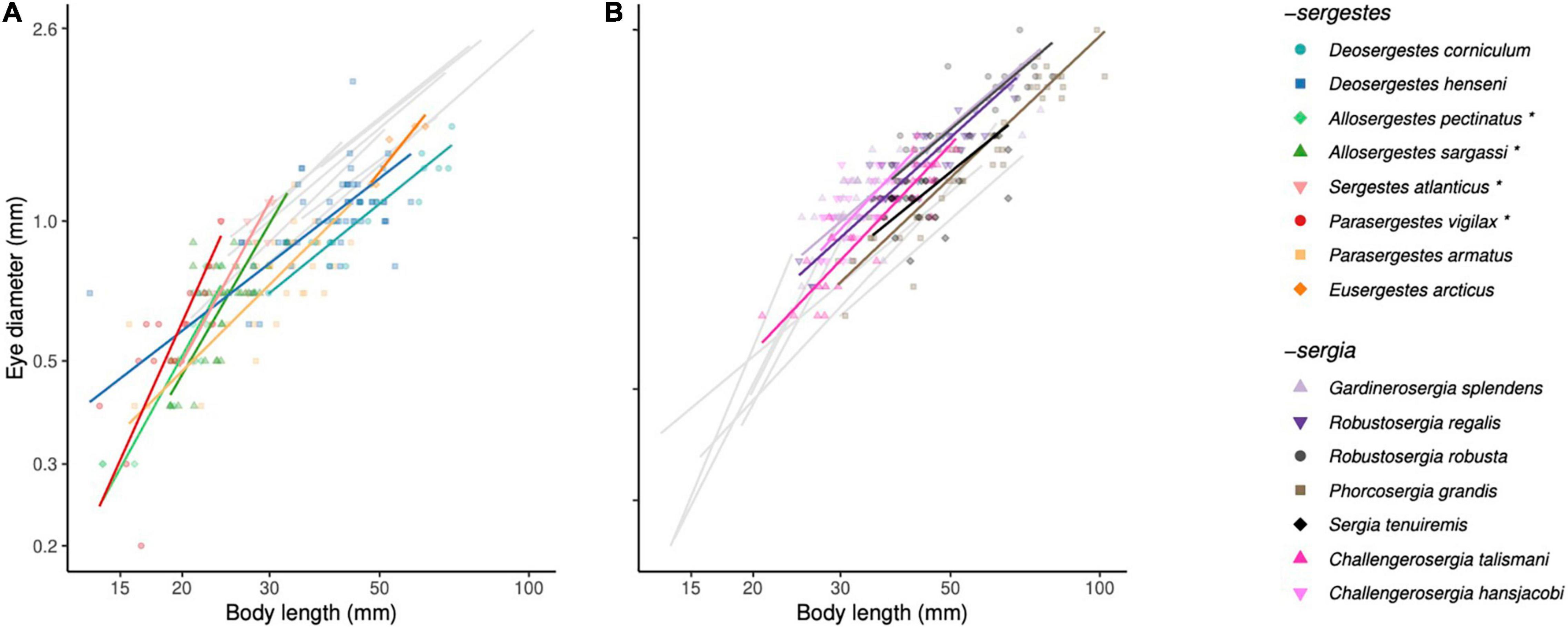
Figure 1. Static allometry. Adult eye-body size allometry is shown for 15 species of sergestid shrimps. Neosergestes edwardsii was not included as a regression could not be fitted due to low sample size. Eye-body allometry for the (A) -sergestes and (B) -sergia groups have been separated, with the regression lines of the opposing groups shown in grey. Data for each species are indicated by combinations of symbol and coloration. Asterisks indicate species with significantly non-isometric eye-body allometry. Lines indicate standardized major axis (SMA) regressions of log10 eye diameter vs. log10 body length.
Comparing the allometric slopes across species, we found that linear mixed models allowing for variable slopes fit the data better than those assuming a common slope across species (ΔAIC = 30; Table 2). This is consistent with the finding of the SMA regression with species as a covariate, which also indicated that eye-body allometry varies between sergestids (likelihood ratio statistic = 78.4; df = 14; p < 0.0001). Of the 105 Šidák-corrected pairwise comparisons of allometric slopes across species, 32 significant differences (p < 0.05) were detected (Supplementary Table 2). These differences were largely explained by the four species with positive allometry mentioned above (P. vigilax, S. atlanticus, A. sargassi, and A. pectinatus). Their slopes did not differ from one another, but did differ from the slopes of all other species. Inspection of the regression intercepts (α) indicated that -sergia species generally had larger eyes for a given body size than -sergestes species (Figure 1). These data also show that species with the highest allometric slopes had the smallest absolute eye and body sizes, suggesting a relationship between relative growth rate of the eye and overall size (Figure 1).
Finally, we examined the effects of preservation type on eye-body allometry between species by adding preservation type as a covariate, which we found to improve model fit (ΔAIC = 30; Table 2). Comparison of model intercepts indicated that preservation type had a significant, but small effect on eye-body allometry. Specifically, specimens preserved in EtOH had a slightly lower intercept (α = −1.37) than fresh specimens (α = −1.35), and those fixed in paraformaldehyde had a slightly higher intercept (α = −1.31), yielding relatively smaller and larger eye sizes compared to the body after preservation, respectively (Supplementary Figure 1). Because preservation type is represented unevenly across species, we could neither determine the role of preservation type separate from species effects nor apply a correction for preservation type to subsequent analyses. However, the analyses evaluating the effect of preservation type on the study conclusions indicated that removing the paraformaldehyde fixed specimens did not change the outcomes of the SMA and LMM analyses of static allometry. Specifically, the four -sergestes species having the only significant non-isometric eye-body allometry were maintained and the allometric slopes remained significantly different across sergestids (see all results in previously cited Github repository). Though the preservation methods did not appear to affect our study conclusions, their slight but differential effects call for an emphasis on fresh-specimen sampling where possible in future studies of crustacean allometry.
Evolutionary Allometry: Ecological Correlates of Relative and Absolute Eye Size
Initial examination of eye-body allometry across species means indicated negative allometric scaling in the uncorrected and corrected PGLS regressions (λ0: β = 0.93 and λ1: β = 0.82; Figure 2 and Table 3), indicating that eye size becomes relatively smaller across sergestid species as body size increases. Examining the ecological correlates of this relationship with subsequent PGLS regressions, light organ type and DVM distance were the only two variables to significantly correlate to relative eye size. Whereas light organ type significantly correlated to relative eye size both before and after phylogenetic correction (λ0: p < 0.01 and λ1: p < 0.05), DVM distance was only a correlated in the uncorrected model representing independent evolution (λ0: p < 0.05 and λ1: p > 0.05; Figure 3 and Table 3).
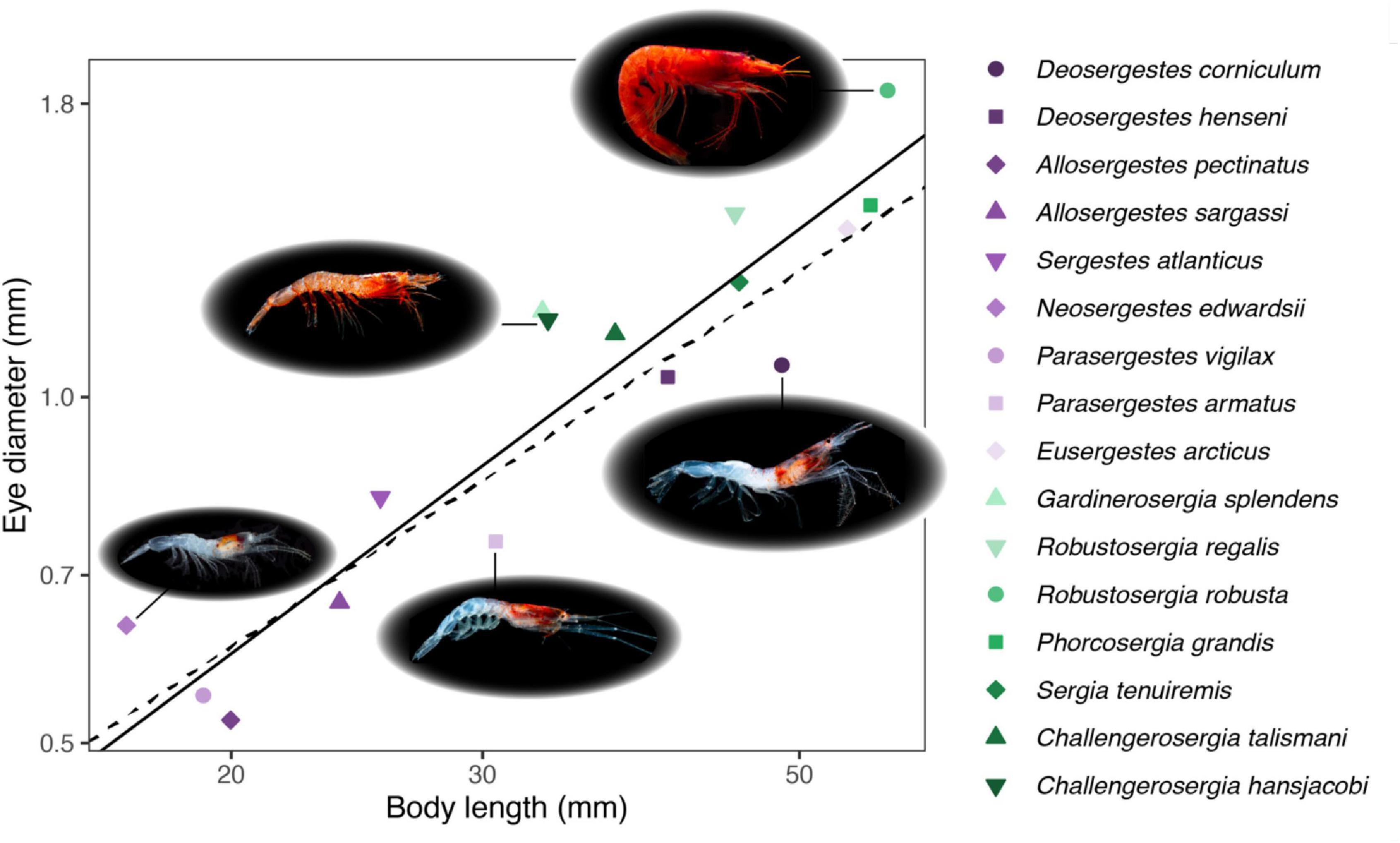
Figure 2. Evolutionary allometry. Mean eye-body size allometry is shown for -sergia (green) and -sergestes (purple) groups of sergestid shrimps. Lines indicate phylogenetic generalized least squares (PGLS) regressions of log10 eye diameter vs. log10 body length. Models were run with λ set to either 0 (solid line) or 1 (dashed line), accounting for the lowest and highest bounds of phylogenetic signal in model residuals, respectively. Images represent a subset of sergestid shrimps and were used with permission from the photographer, ©DantéFenolio.
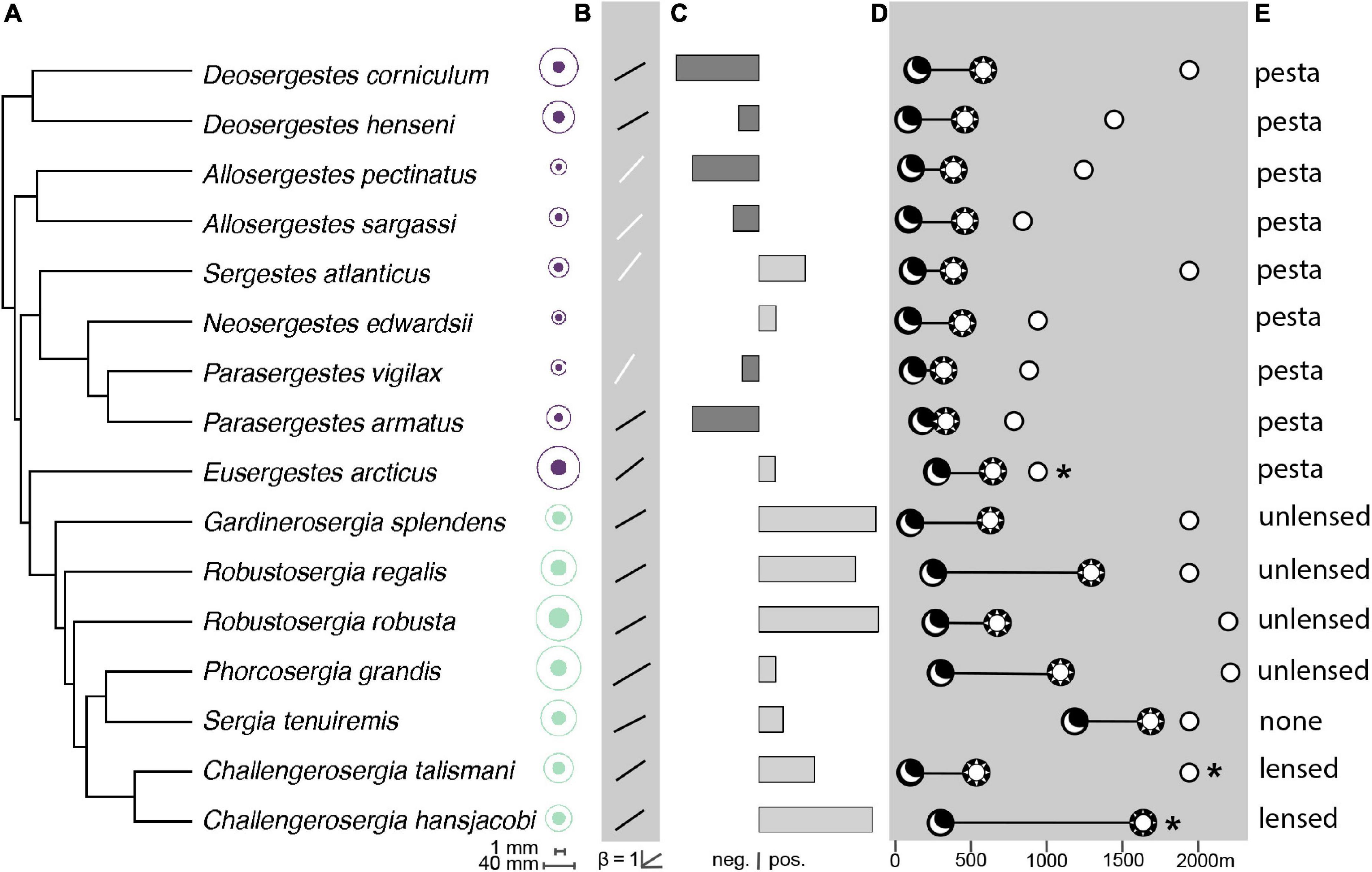
Figure 3. A summary of sergestid eye-size ecology. Data are presented for -sergia (green) and -sergestes (purple) groups of sergestid shrimps across a phylogeny adapted from Golightly et al. (2022). (A) Mean eye size (filled circle) and body size (open circle). The legend indicates how the eye diameter (top) and body length (bottom) scale in absolute terms. (B) Allometric slopes (β) from adult eye-body size standardized major axis (SMA) regressions of log10 eye diameter vs. log10 body length. White lines indicate allometric slopes that are significantly different from 1 (non-isometric). (C) Residuals from the PGLS regression of sergestid evolutionary eye-body allometry (at λ1) indicates a measure of eye investment, with negative and positive residuals indicating how small or large an eye is for a given sergestid body size compared to what is predicted by the model. (D) Mean depth distributions and (E) light organ types. The moon symbol indicates mean nighttime depth, the sun symbol indicates mean daytime depth, the diel vertical migration (DVM) distance is shown by the black line, and the open circle indicates maximum reported depth. The asterisks indicate species that occupy a benthopelagic habitat. Depth estimates were taken from published literature, see Table 1 for citations.
For light organ type, species with organs of Pesta (the -sergestes group) consistently had smaller relative eye sizes (λ0: α = −1.24, SE = 0.02, t = 4.39, p < 0.001; λ1: α = −1.19, SE = 0.03, t = 3.06, p < 0.01) than those in the photophore-bearing (-sergia) group (λ0: α = −1.13; λ1: α = −1.08), with eye-to-body size ratios of these groups at 0.028 (±0.005 sd) and 0.032 (±0.003 sd), respectively (Figures 3, 4). For DVM distance, relative eye size increased slightly with increasing DVM distance at λ0 (β = 0.0001, SE = 0.00005, t = 2.28, p < 0.05). Neither the depth variables (mean daytime depth, mean nighttime depth, maximum reported depth) nor habitat type correlated significantly with relative eye size (Table 3).
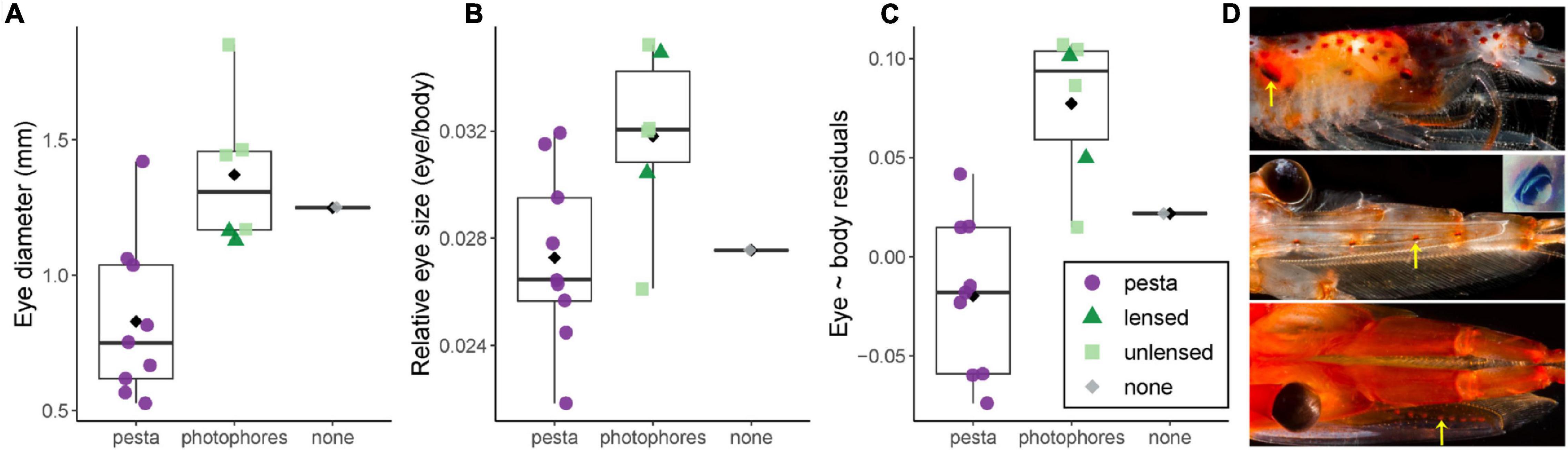
Figure 4. Eye size variation by light organ type. Data are presented for -sergia (green) and -sergestes (purple) groups of sergestid shrimps across types of light organ: organs of Pesta (pesta; circle), lensed dermal photophores (photophores; triangle), unlensed dermal photophores (photophores; square), or those lacking light organs (diamond; none). For all plots, black diamonds indicate means, black bars indicate medians, boxes indicate interquartile ranges, and whiskers indicate minimum and maximum values. The Y-axes of each plot indicate (A) mean eye diameter, (B) mean eye diameter divided by mean body length, (C) residuals from the PGLS regression of sergestid eye-body evolutionary allometry at λ1, with negative and positive residuals indicating how small or large an eye is for a given sergestid compared to what is predicted by the model. (D) Representative images of sergestid light organs (yellow arrows), used with permission from the photographer, ©DantéFenolio. Top: organs of Pesta of Desosergestes henseni; Center: lensed dermal photophores of Challengerosergia talismani (inset photo credit L. S.); Bottom: unlensed dermal photophores of Gardinerosergia splendens.
In contrast to relative eye size, the ecological correlates of absolute eye size were light organ type, mean daytime depth, and maximum reported depth (Figure 3 and Table 4). Light organ type significantly correlated to absolute eye size before correction (λ0: p < 0.01) but not after (λ1: p = 0.1; Figures 3, 4). The same was true for mean daytime depth (λ0: p < 0.01 and λ1: p = 0.3); however, the interaction of mean daytime and nighttime depths, and maximum reported depth, were significant correlates both before and after phylogenetic correction (λ0: p < 0.01 and λ1: p < 0.01).
As with relative eye size, absolute eye size was smaller in species with organs of Pesta (the -sergestes group; λ0: μ = 0.83, SE = 0.10) than in photophore-bearing (-sergia) species (λ0: μ = 1.37, SE = 0.15, t = 3.59, p < 0.01; Figure 4). As for the depth metrics, absolute eye size increased with increasing depth, though for mean daytime depth, that trend only held to 1,000 m depth – the transition from the mesopelagic zone to the bathypelagic zone (Figure 5). Subsequent PGLS regressions indicated that the interaction of zone and daytime depth significantly correlated to absolute eye size at both values of λ (λ0: p < 0.0001 and λ1: p < 0.0001; Figure 5 and Supplementary Table 3). This pattern was also reflected in sergestid body size at both values of λ (λ0: p < 0.001 and λ1: p < 0.01; Figure 5 and Supplementary Table 4). No relationship was found between eye size and nighttime depth zone at either value of λ (λ0: p = 0.62 and λ1: p = 0.77; Supplementary Table 5). Nighttime depth, DVM distance, and habitat type were not correlated with absolute eye size in either the corrected or uncorrected PGLS regressions (Table 4).
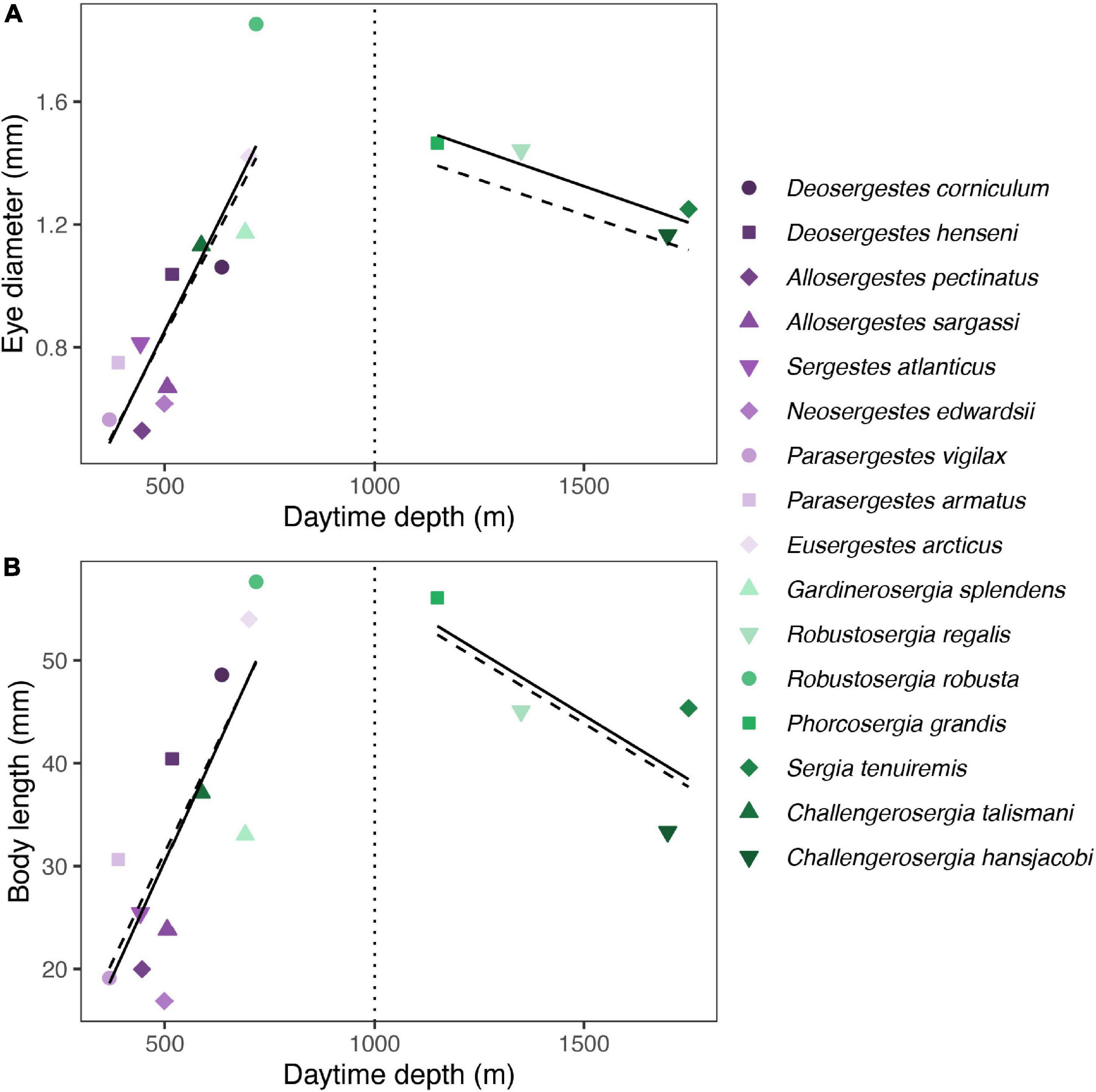
Figure 5. Eye and body size variation with depth. Plots shows mean eye diameter (A) and mean body length (B) vs. daytime depth for -sergia (green) and -sergestes (purple) groups of sergestid shrimps. Regression lines indicate phylogenetic generalized least squares (PGLS) regressions. Models were run with λ set to either 0 (solid line) or 1 (dashed line), accounting for the lowest and highest bounds of phylogenetic signal in model residuals, respectively. The dotted vertical lines in plots indicate the transition from the mesopelagic to the bathypelagic zone.
Sighting Distance Modeling
The sighting distance models indicated that based on species means for absolute eye size, the maximum distance for detecting a standardized bioluminescent point source on a black background differs among sergestids by a factor of three (Figure 6 and Supplementary Table 6). The shortest sighting distance threshold was 1.11 m for A. pectinatus and the maximum was 3.77 m for R. robusta, scaling positively with increased eye size and body size as would be expected (Figure 6). Considering these sighting distance thresholds in relative terms (and perhaps, in a more ecological context), we examined sighting distance as the number of body lengths for a given species. Fitting a regression to these data indicated that relative sighting distance does not differ across sergestids of different body sizes (F(1,14) = 3.0, R2 = 0.12, p = 0.11). Taking the mean of these relative distances, we found that sergestids can detect a bright bioluminescence point source against a black background around 63.5 (±9.6 sd) body lengths away.
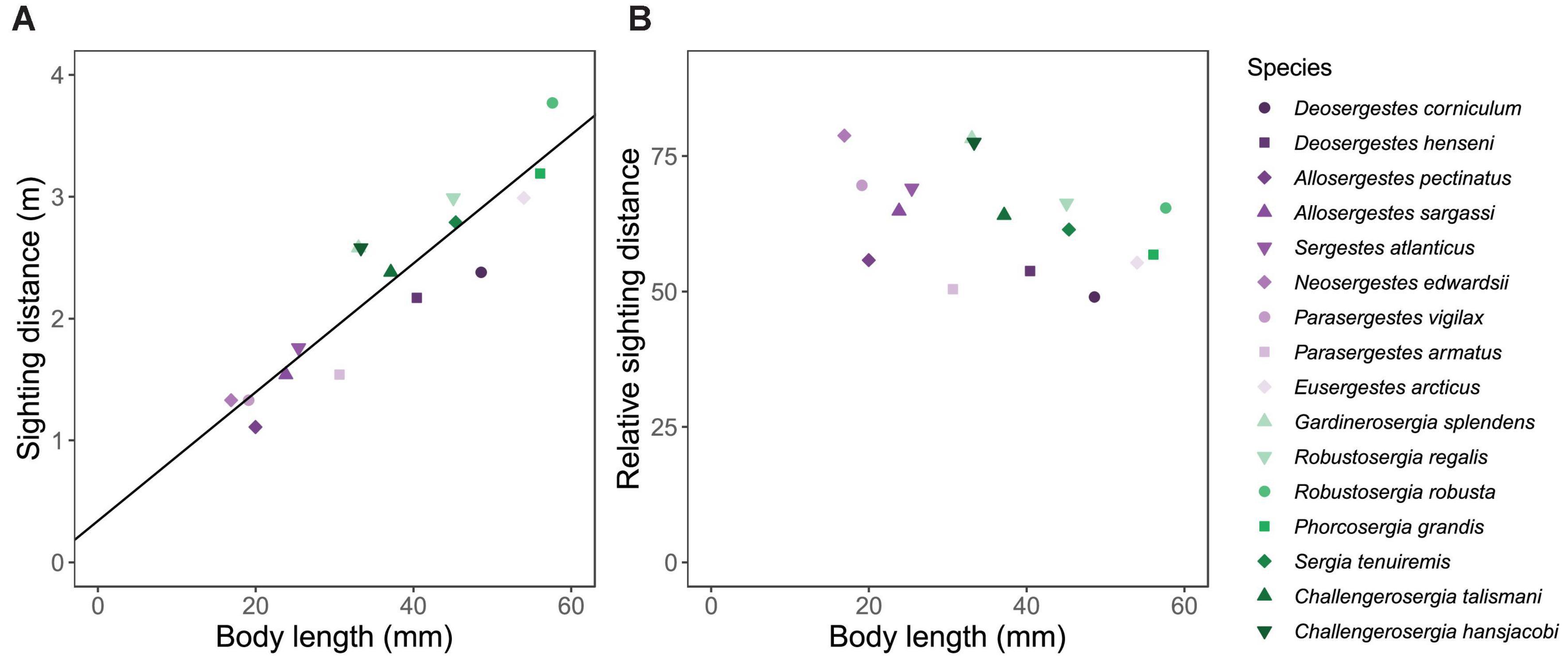
Figure 6. Maximum thresholds of bioluminescence sighting distance. Plots show (A) estimated sighting distance and (B) relative sighting distance (as a factor of body lengths for a given species) vs. mean body length for -sergia (green) and -sergestes (purple) groups of sergestid shrimps. Sighting distances are based on species means for absolute eye size, indicating the maximum distance a sergestid might detect a bioluminescent point source on a black background using an approach originally developed by Nilsson et al. (2014) and then modified by Ruxton and Johnsen (2016). Solid line indicates a significant ordinary least square (OLS) regression (adjusted R2 = 0.83, p < 0.0001).
Discussion
Constraints on energy resources and available light in the deep sea should place strong selection pressure on eye size, which is a fundamental determinant of visual ability (Warrant and Locket, 2004). Investigating eye size among sergestids, our results show significant differences in eye growth rate and average eye size that are correlated to different aspects of sergestid ecology and result in variable thresholds of bioluminescence sighting distance. As it is not possible to accurately estimate phylogenetic signal (λ) across 16 species (Freckleton et al., 2002), we duplicated each analysis with and without a maximum-possible phylogenetic correction. We found different ecological predictors of relative and absolute eye size, with depth metrics only correlating to absolute size, DVM distance only correlating to relative eye size, and light organ type the only significant predictor of both. Neither relative nor absolute eye size significantly correlated to habitat type (pelagic vs. benthopelagic), indicating that occupation of the benthopelagic (punctuated by DVMs to the pelagic; Flock and Hopkins, 1992) has no observable relationship to eye size among these sergestids.
Considering the relationship between eye size and light organ type, as well as the role of absolute eye size in visual performance, we examined sighting distance thresholds of bioluminescence, finding that thresholds fall within ≤3.77 m and vary between sergestids by a factor of three. In relative terms, we found that all sergestids under the test conditions share a common bioluminescence detection threshold at ∼64 body lengths. This suggests the maintenance of relative visual ability, at least for maximum sighting distance of a standardized bioluminescent point source, that scales evenly with species size. Together, our study reveals patterns of eye growth rate and average eye size in sergestids, identifying different ecological factors that may underlie the relative energetic investment in eyes (i.e., relative eye size) and the optical basis of visual capability (i.e., absolute eye size). These findings suggest an important relationship between vision and intraspecific bioluminescence in particular, and the ability to detect bioluminescence over an ecologically relevant range, providing new insights into the visual ecology and eye size evolution of this diverse deep-sea group.
Intraspecific Eye Size by Phylogenetic Group, Ecology, and Body Size
Our results show that adult static eye-body allometry – an indicator of eye growth rate – differs substantially across sergestids. The -sergestes group contained the only four species with significantly non-isometric allometry: P. vigilax, S. atlanticus, A. sargassi, and A. pectinatus, which had the four highest allometric slopes and thus, the fastest growing eyes relative to the body of all sergestids sampled. One explanation for rapid eye growth in the -sergestes group might be to meet the visual demands of relatively shallow waters. Specifically, -sergestes species occupy shallower waters than -sergia species both throughout ontogeny (Flock and Hopkins, 1992) and at adulthood (see Golightly et al., 2022), with those four species (with the fastest eye growth rates) occupying on average 150 m nighttime and 440 m daytime depths (Table 1). At these relatively shallow depths, animals are exposed to higher ambient light levels and stronger predation risk (Jerlov, 1976; Hamner, 1996); thus, rapidly increasing eye size may help these species to accomplish tasks such as detecting prey and avoiding predators in these brighter light conditions. Previous work supports a relationship between eye size and predation risk, with one study indicating larger eye sizes associated with increased predator abundance in amphipod crustaceans (Glazier and Deptola, 2011) and another indicating predator-induced pupil enlargement in teleost fish (Vinterstare et al., 2020).
Despite rapid growth rates, these four -sergestes species ultimately had the smallest average absolute eye size compared to the remaining sampled species (Figure 1). Although this trend appears related to several ecological factors (addressed later by evolutionary allometry), a further explanation may relate to a law of diminishing returns for how visual performance (i.e., sighting distance) scales with eye size in pelagic habitats (Nilsson et al., 2012). Increasing the size of smaller eyes provides greater returns in visual performance than increasing larger eyes by the same magnitude (Nilsson et al., 2012, 2014). Thus, higher growth rates may be particularly advantageous for animals with smaller eyes. This claim is further supported by our finding that the two species with the lowest eye growth rates (P. grandis; β = 0.94 and R. robusta: β = 0.84) also had the largest absolute eye sizes (Figure 1 and Supplementary Table 1).
This connection between eye growth rate and absolute size brings up an additional consideration about nutrient availability in the deep sea. An early survey of deep-sea crustaceans indicated that body size increases until the start of the bathypelagic zone (1,000 m) then begins to taper off (Mauchline, 1972). Increasing body size with depth is predicted to provide metabolic advantages, such as resource allocation for producing fewer, but larger offspring than those produced by smaller species found in shallower waters (Mauchline, 1972; Rex and Etter, 1998). Large animals (and offspring) can feed upon a wider range of material and have greater mobility for traveling long distances in search of food and mates (Mauchline, 1972; Rex and Etter, 1998). Our data supported this trend, with sergestid body size increasing until 1,000 m and decreasing thereafter (Figure 5). Some of the largest-bodied and deepest-dwelling species also had some of the lowest eye growth rates (with eyes growing slower than body) found among sergestids. This allocation of resources toward the rate of body growth over eye growth suggests that the metabolic advantage of increasing body size may be greater than visual advantage of fast eye growth at greater depths. Despite these low eye growth rates, deeper-dwelling species end up with the largest absolute eye sizes – a trend that we later relate to body size and visual performance.
Of all species, S. tenuiremis had the lowest eye growth rate relative to body size by a factor of three. Correspondingly, S. tenuiremis had the deepest daytime depth average (1,750 m) and was the only sergestid studied here to lack bioluminescent light organs entirely (Vereshchaka, 2000). This observation may provide additional support for a relationship between eye growth and the capacity for conspecific bioluminescent signaling, but is presented with caution, as eye-body allometry in S. tenuiremis did not differ from isometry and only represents a single non-bioluminescent species. Thus, among sergestids – environmental light level, predation risk, gains in visual performance, nutrient availability, and the capacity for bioluminescent signaling present several potential explanations for patterns of adult eye-body allometry found here.
Eye Size and Bioluminescence
Of the ecological correlates tested, light organ type was the only significant correlate of both relative and absolute eye size, suggesting that sergestid eye size evolution has been guided, at least in part, by visual demands of bioluminescent signaling. Specifically, larger relative and absolute eye sizes were found in species with dermal photophores than in those with larger organs of Pesta (Figure 4), suggesting that species invest more resources in their eyes for improved visual ability when they have smaller (and putatively dimmer) bioluminescent light organs. As sergestid light organs, such as organs of Pesta (Herring, 1981), are comprised of individual luminescent units (photocytes), it stands to reason that larger organs are comprised of either more or thicker-diameter photocytes that are capable of emitting a greater intensity of light. Looking to the largest-bodied species for an example, we see a ten-fold difference in the diameter of the largest dermal photophore (on the antennal scale) of R. robusta at ∼0.2 mm (Vereshchaka, 2000) and the largest organ of Pesta in E. arcticus at ∼2 mm (Foxton, 1972). Therefore, as sergestid dermal photophores are much smaller than organs of Pesta, they might produce weaker signals that are more difficult to visually detect or spatially resolve.
For putative bioluminescent signaling, it remains unknown whether these shrimps resolve individual light organs, light organ patterns, or if they rely on temporal emissions (flashes) from some or all light organs combined (Schweikert et al., 2020). Either way, one might expect the much smaller size of dermal photophores to produce dimmer emissions that require larger eyes for detection—a prediction that was supported by our findings. Though we were unable to consider unlensed vs. lensed photophores as separate states due to low sample size (and thus low power), examination of the data suggests that animals with unlensed photophores may have the largest absolute eye sizes (Figure 4). As lenses are thought to concentrate and guide light emissions (Denton et al., 1970; Cavallaro et al., 2004), it is possible that unlensed photophores emit more diffuse light than lensed photophores, ultimately requiring larger eyes.
Our findings add to a growing body of work that indicates an important relationship between bioluminescence and vision in deep-sea crustaceans. A previous survey of deep-sea shrimps (including three sergestid species) found that spectral sensitivity was better tuned to bioluminescence emission spectra found commonly in the deep sea than to downwelling light (Frank and Widder, 1999). In another survey of deep-sea crustaceans, larger relative eye sizes were found in species (including sergestids) with light organs than in those lacking the trait, which was suggested by the authors to help the animals form aggregations and identify mates through conspecific signaling (Hiller-Adams and Case, 1988). This putative relationship between eye size and conspecific signaling has also been supported by previous work on ostracods, indicating that the presence and size of eyes in males (compared to females) is related to a need for bioluminescent signaling (e.g., Morin, 1986; Oakley, 2005). In line with these findings, our results show that sergestid eye sizes are correlated with the morphology (and putative brightness) of bioluminescent organs, suggesting a role of sergestid bioluminescence in conspecific signaling.
Currently, sergestid bioluminescence has not been documented to function in conspecific signaling and is primarily thought to serve in counterillumination camouflage, in which ventral bioluminescence replaces sunlight blocked by the body, reducing predation risk from viewers below (Warner et al., 1979; McFall-Ngai, 1990). Growing evidence suggests that conspecific signaling may be predicted in some -sergia species, after findings of sexual dimorphism in photophore pattern (Herring, 2000, 2007). Therefore, our finding that animals with photophores (i.e., -sergia spp.) have larger eyes than species with organs of Pesta (-sergestes spp.) suggests that both groups might rely on conspecific signaling or, alternatively, that only photophore-bearing species (-sergia spp.) rely on conspecific signaling, requiring comparatively larger eyes.
Implications for Visual Performance
With our results highlighting the importance of bioluminescence as a correlate of eye size, we aimed to better understand the relationship between these factors by modeling maximum sighting distances of bioluminescence under best-case environmental conditions (i.e., darkness) based on the mean eye size for each species. The arrangement and emission intensities of bioluminescent light organs among sergestids have yet to be fully characterized, preventing us from modeling visibility differences related to each light organ type. Instead, we modeled maximum sighting distances of a standardized bioluminescent source, revealing the best-case scenario of sighting distance based on eye size differences alone. We estimated that sergestid species can visually detect a point-source of bioluminescence from distances of 1.11–3.77 m (Figure 6), falling in the range of a previous study of sergestid sighting distances (≤7.57 m) that considered greater possible variation in bioluminescence emission intensities (Schweikert et al., 2020). These sighting distances are ecologically relevant for possible conspecific signaling, as the species sampled here are known to occur in multi-species swarms (Bracken-Grissom, pers. observation; Vereshchaka, 2009), with some species found at abundances of up to 20 individuals per m3 (Vereshchaka, 2009). With diverse genitalia and clasping organs on males, sergestids are thought to reproduce by copulation (Genthe, 1969; Vereshchaka, 2009). Therefore, the detection of bioluminescence even at short ranges may support a need to find and identify conspecifics for aggregation or copulation (Foxton, 1972; Vereshchaka, 2009).
Knowing that larger eyes permit greater sighting distances, we then asked whether larger-eyed species have relatively greater sighting distances than smaller-eyed species, and for the given test conditions, the answer appears to be no. By standardizing sighting distances by body length, we found that relative sighting distance of a given bioluminescent point source does not differ among species, averaging 63.5 body lengths. As eye size scales with body size with only slight negative allometry (Figure 2 and Table 3), one would expect this finding, that relative sighting distance remains constant with increasing body size across all species under standardized external conditions. This finding helps place the allometry results in an ecological context, suggesting that although absolute visual performance varies with eye size, relative visual performance may hold constant to body size in sergestids. Here, that reveals a shared detection distance for bioluminescence (63.5 body lengths), which may or may not relate to shared behaviors of these species requiring close proximity of conspecifics, such as swarming and copulation (Vereshchaka, 2009).
Eye Size, Depth, and the Diel Vertical Migration
In addition to light organ type, mean daytime depth, maximum reported depth, and DVM distance were significantly correlated with sergestid eye size (Figure 3). Unexpectedly, the relationships between these ecological variables and eye size were mutually exclusive; mean daytime and maximum reported depth correlated only with absolute eye size and DVM distance correlated only with relative eye size. This finding, that larger relative eye sizes correlate to more extensive DVMs, but not to deeper depths, suggests that migration distance imposes visual demands distinct from the average depths occupied by sergestids.
For many deep-sea animals, including sergestids, diel changes in the light field are thought to trigger DVM behavior (Widder and Frank, 2001), with a leading hypothesis (i.e., preferendum hypothesis) suggesting that animals migrate to stay within a preferred illumination level, known as an isolume (Ewald, 1910; Rose, 1925; Russell, 1926; Cohen and Forward, 2016). Animals with greater DVM distances may have lower intensity isolumes as they migrate to greater depths in order to maintain constant illumination during the day. Thus, farther migration distances may coincide with greater eye investment to allow a low intensity isolume to be detected.
Absolute eye size (representing the optical capability of vision) was correlated to depth, with three significant predictors found across depth variables: mean daytime depth, its interaction with nighttime depth, and maximum reported depth. Across these models, we found that absolute eye size increased with depth, suggesting that sergestids require progressively larger eyes (for enhanced sensitivity, acuity, or both) as sunlight dims and the scene becomes dominated by bioluminescent light. Further examination of daytime depth revealed that the transition between the photic and aphotic oceanic zones present an interesting division in sergestid eye size evolution. Across daytime depths, eye size (and body size) steadily increases across the epipelagic and the mesopelagic zones, but then decreases in the bathypelagic zone (Figure 5). This trend is also found among certain deep-sea fishes (Munk, 1965; Warrant, 2000), suggesting that larger eyes and enhanced visual ability may not be necessary (or attainable) with the absence of sunlight and limited nutrients in bathypelagic waters.
Our finding that absolute eye size correlated to depth, but relative eye investment did not, was driven by the fact that eye size and body size co-vary with changes in depth (Figure 5). This tight correlation between eye and body size makes it difficult to determine whether changes in eye size over depth are driven by selection on vision or are simply a byproduct of selection on body size (described above; Mauchline, 1972; Rex and Etter, 1998). It is well established through allometric studies that larger animals tend to have larger eyes (Hughes, 1977; Land and Nilsson, 2012), and we also found strong eye-body allometry in sergestids. Thus, a coincidental relationship is possible, with eye size passively scaling with changes in body size over depth.
An alternative interpretation, however, is that in addition to the metabolic and reproductive benefits of increasing body size, body size may increase with depth in order to support the growth of larger eyes, as has been suggested in giant squids (Nilsson et al., 2012). Increasing eye size is energetically costly (Niven and Laughlin, 2008), and the deep sea is energy limited (Warrant and Locket, 2004), suggesting that deep-sea eye size may be under strong selection that could drive changes in body size. The factors selecting for eye size and body size are difficult to disentangle and require further study across animal groups, eye types, and ecologies found in the deep sea.
Study Limitations
Perhaps due to the relative inaccessibility of the deep sea, only a handful of comparative studies of eye size exist on the animals inhabiting this realm (Marshall, 1971; Locket, 1977; Hiller-Adams and Case, 1988; Warrant, 2000; de Busserolles et al., 2013). Well-resolved molecular phylogenies are less common among deep-sea taxa than shallow or terrestrial groups, but are necessary to account for evolutionary relationships among species that may influence the distribution of eye size and other traits. Here, we studied a diverse group of shrimps (family Sergestidae) with an available molecular phylogeny and were still met with several study limitations. The first concerns how the ecological variables were distributed across our subtree. For example, all species with an organ of Pesta were closely related (representing the -sergestes group), with a single evolutionary transition to photophores (representing the -sergia group). Future studies that incorporate a greater diversity of species – with more independent transitions between light organ types – will provide more convincing evidence for possible functional relationships and coevolution between bioluminescence and eye size. A second limitation was our inability to apply a corrective factor to our analyses to account for preservation effects on eye-body allometry across the species sampled. Subsequent comparison of analytical outcomes with and without the inclusion of paraformaldehyde-fixed specimens however, helped validate the conclusions presented here. A third limitation of our dataset was that we were unable to estimate phylogenetic signal (λ), as our sample size was <20 species (Freckleton et al., 2002). In response, we assumed the highest possible phylogenetic signal (λ1) in our data and examined our results with and without applying a corresponding correction, revealing the ecological correlates to eye size most robust to the possible effects of shared descent. Overall, these findings provide some support that a correlation exists between eye size and these ecological variables, with our confidence in these relationships depending on the true value of λ (phylogenetic signal).
Study Conclusion
The deep sea is light limited, and increasing eye size can enhance light detection. In turn, large eyes are metabolically expensive and energy constraints in this habitat would suggest that eye size evolves under strong selection, making the deep sea a valuable system for investigating the factors that influence eye size. Overall, we found that higher eye growth rates are found in sergestids with smaller absolute eye sizes, a relationship that might be related to ecology and/or to the cost of diminishing returns to vision by increasing already large eye sizes. The ecological correlates of absolute eye size and relative eye size differed, perhaps owing to patterns of body size that either yield metabolic benefits at greater depths, support the growth of large eyes, or both (Mauchline, 1972; Rex and Etter, 1998); however, both absolute and relative eye size correlated to light organ type. These findings suggest that bioluminescence plays a particularly important role in the evolution of sergestid vision and that these animals may be reliant on bioluminescent signaling. Models of maximum sighting distance thresholds for bioluminescence estimated absolute and relative detection ranges that appear relevant to sergestid reproductive ecology, also suggesting a possible function of conspecific visual signaling that – taken with the findings of other sergestid studies – remains an attractive and important area for future deep-sea crustacean research.
Data Availability Statement
Morphological data are available in the Supplemental Excel File 1, and code to reproduce all analyses and diagnostic checks of regressions are annotated in RMarkdown and available on GitHub (https://github.com/knthomas/sergestid-eyes).
Author Contributions
LS, KT, and HB-G conceptualized and supervised the study, analyzed, and interpreted the data. LS, VM, AC, and CG collected and curated the data. LS and KT generated the figures and wrote initial drafts of the manuscript. All authors contributed to final manuscript and approved the final submission.
Funding
This work was supported by Florida International University’s College of Arts, Sciences, and Education Distinguished Scholar Award (awarded to LS), the Gulf of Mexico Research Initiative (GOMRI, DEEPEND Consortium awarded to HB-G), the National Science Foundation (NSF) Division of Environmental Biology Grant (#1556059 awarded to HB-G), the National Oceanic and Atmospheric Administration’s (NOAA) RESTORE Science Program (#NA19NOS4510193 to Florida International University and Nova Southeastern University), the NOAA Ocean Exploration Research (NOAA-OER) Grant (#NA170AR0110208; awarded to S. Johnsen) and the Florida Institute of Oceanography Shiptime Funding (awarded to HB-G). Data are publicly available through the Gulf of Mexico Research Initiative Information and Data Cooperative (GRIIDC) at https://data.gulfresearchinitiative.org (10.7266/N7XP7385, 10.7266/N7902234, 10.7266/n7-ac8e-0240, 10.7266/N70P0X3T).
Conflict of Interest
The authors declare that the research was conducted in the absence of any commercial or financial relationships that could be construed as a potential conflict of interest.
The reviewer KF declared a past co-authorship with several of the authors LS and HB-G, to the handling editor.
Publisher’s Note
All claims expressed in this article are solely those of the authors and do not necessarily represent those of their affiliated organizations, or those of the publisher, the editors and the reviewers. Any product that may be evaluated in this article, or claim that may be made by its manufacturer, is not guaranteed or endorsed by the publisher.
Acknowledgments
We would like to thank the captain, crew, and science parties of two deep-sea research expeditions that occurred on May 4–8, 2019 on the R/V Weatherbird II and on June 9–22, 2019 on the R/V Point Sur. We thank the Florida International Crustacean Collection (FICC) at Florida International University for providing specimens for this study. We also thank Natalie Cooper for help with the comparative methods, as well as associate editor DA and JT-D, KF, and Alexandra Kingston for helpful comments on an earlier draft of this manuscript. This is contribution #1408 from the Coastlines and Oceans Division of the Institute of Environment at Florida International University.
Supplementary Material
The Supplementary Material for this article can be found online at: https://www.frontiersin.org/articles/10.3389/fevo.2022.787315/full#supplementary-material
Footnotes
References
Barnard, K. H. (1946). Descriptions of new species of South African decapod Crustacea, with notes on synonymy and new records. Ann. Mag. Nat. Hist. 13, 361–392.
Bates, D., Mächler, M., Bolker, B., and Walker, S. (2015). Fitting linear mixed-effects models using lme4. J. Stat. Soft. 67, 1–48.
Burnham, K. P., Anderson, D. R., and Huyvaert, K. P. (2011). AIC model selection and multimodel inference in behavioral ecology: some background, observations and comparisons. Behav. Ecol. Sociobiol. 65, 23–35.
Cavallaro, M., Mammola, C. L., and Verdiglione, R. (2004). Structural and ultrastructural comparison of photophores of two species of deep-sea fishes: Argyropelecus hemigymnus and Maurolicus muelleri. J. Fish Biol. 64, 1552–1567. doi: 10.1111/j.0022-1112.2004.00410.x
Cohen, A., and Morin, J. (2010). Two new bioluminescent ostracode genera, Enewton and Photeros (Myodocopida: Cypridinidae), with three new species from Jamaica. J. Crust. Biol. 30, 1–55. doi: 10.1651/08-3075.1
Cohen, J. H., and Forward, R. B. Jr. (2016). “Zooplankton diel vertical migration—a review of proximate control,” in Oceanography and Marine Biology, eds R. N. Gibson, R. J. Atkinson, and J. M. Gordon (Boca Raton: CRC Press), 89–122. doi: 10.1201/9781420094220-5
Cronin, T. W., Johnsen, S., Marshall, N. J., and Warrant, E. J. (2014). Visual Ecology. Princeton: University Press.
de Busserolles, F., Fitzpatrick, J. L., Paxton, J. R., Marshall, N. J., and Collin, S. P. (2013). Eye-size variability in deep-sea lanternfishes (Myctophidae): an ecological and phylogenetic study. PLoS One 8:e58519. doi: 10.1371/journal.pone.0058519
Denton, E. J. (1990). “Light and vision at depths greater than 200 metres,” in Light and Life in the Sea, eds P. J. Herring, A. K. Campbell, M. Whitfield, and L. Maddock (Cambridge: Cambridge University Press), 127–148.
Denton, E. J., Gilpin-Brown, J. B., and Wright, P. G. (1970). On the ‘filters’ in the photophores of mesopelagic fish and on a fish emitting red light and especially sensitive to red light. J. Physiol. 208, 728–738.
Eltoum, I., Fredenburgh, J., Myers, R. B., and Grizzle, W. E. (2013). Introduction to the theory and practice of fixation of tissues. J. Histotechnol. 24, 173–190. doi: 10.1179/his.2001.24.3.173
Ewald, W. F. (1910). Über Orientierung, Lokomotion und Lichtreaktionen einiger Cladoceren und deren Bedeutung für die Theorie der Tropismen. Biol. Zentbl. 30, 1–399.
Felder, D. L., Álvarez, F., Goy, J. W., and Lemaitre, R. (2009). “Decapoda (Crustacea) of the Gulf of Mexico, with comments on the Amphionidacea,” in Gulf of Mexico: Origin, Waters, and Biota, eds D. L. Felder and D. K. Camp (College Station, TX: Texas A&M Press), 1019–1104.
Firmat, C., Lozano-Fernández, I., Agustí, J., Bolstad, G. H., Cuenca-Bescós, G., Hansen, T. F., et al. (2014). Walk the line: 600000 years of molar evolution constrained by allometry in the fossil rodent Mimomya savini. Philos. Trans. R. Soc. B. 369:20140057. doi: 10.1098/rstb.2014.0057
Flock, M. E., and Hopkins, T. L. (1992). Species composition, vertical distribution, and food habits of the sergestid shrimp assemblage in the eastern Gulf of Mexico. J. Crustacean Biol. 12, 210–223. doi: 10.2307/1549076
Foxton, P. (1972). Further evidence of the taxonomic importance of the organs of Pesta in the genus Sergestes (Natantia, Penaeidea). Crustaceana 22, 181–189.
Frank, T. M. (2000). Temporal resolution in mesopelagic crustaceans. Philos T. R. Soc. B. 355, 1195–1198.
Frank, T. M., and Widder, E. A. (1999). Comparative study of the spectral sensitivities of mesopelagic crustaceans. J. Comp. Physiol. 185, 255–265.
Freckleton, R. P., Harvey, P. H., and Pagel, M. (2002). Phylogenetic analysis and comparative data: a test and review of evidence. Am. Nat. 160, 712–726. doi: 10.1086/343873
Genthe, H. C. (1969). The reproductive biology of Sergestes similis (Decapoda, Natantia). Mar. Biol. 2, 203–217.
GetData (2017). The Getdata Project. Available online at: http://getdata-graph-digitizer.com/. (accessed September 14, 2020).
Glazier, D. S., and Deptola, T. J. (2011). The amphipod Gammarus minus has larger eyes in freshwater springs with numerous fish predators. Invertebr. Biol. 130, 60–67.
Golightly, C., DeLeo, D., Perez, N., Chan, T. Y., Landeira, J., and Bracken-Grissom, H. (2022). Tracing the evolution of bioluminescent light organs across the deep-sea shrimp family Sergestidae using a genomic skimming and phylogenetic approach. Invertbr. Syst. 36, 22–25. doi: 10.1071/IS21013
Gordon, I. (1939). A new species of Sergestes (Crustacea, Decapoda) from the south Atlantic. Ann. Mag. Nat. Hist. 4, 498–509.
Hamner, W. M. (1996). “Predation, cover, and convergent evolution in epipelagic oceans,” in Zooplankton: Sensory Ecology and Physiology, eds P. Lenz, D. Hartline, J. Purcell, and D. Macmillan (Amsterdam: Overseas Publishers Association), 17–35. doi: 10.1201/9780203733615-2
Harrison, X. A., Donaldson, L., Correa-Cano, M. E., Evans, J., Fisher, D. N., Goodwin, C. E. D., et al. (2018). A brief introduction to mixed effects modelling and multi-model inference in ecology. PeerJ 6:e4794. doi: 10.7717/peerj.4794
Herring, P. J. (1981). The comparative morphology of hepatic photophores in decapod Crustacea. J. Mar. Biol. Assoc. U.K. 61, 723–737.
Herring, P. J. (2000). Species abundance, sexual encounter and bioluminescent signalling in the deep sea. Philos T. R. Soc. B. 355, 1273–1276. doi: 10.1098/rstb.2000.0682
Herring, P. J. (2007). Sex with the lights on? A review of bioluminescent sexual dimorphism in the sea. J. Mar. Biol. Assoc. U.K. 87, 829–842.
Hiller-Adams, P., and Case, J. F. (1988). Eye size of pelagic crustaceans as a function of habitat depth and possession of photophores. Vision Res. 28, 667–680. doi: 10.1016/0042-6989(88)90047-8
Howland, H. C., Merola, S., and Basarab, J. R. (2004). The allometry and scaling of the size of vertebrate eyes. Vision Res. 44, 2043–2065. doi: 10.1016/j.visres.2004.03.023
Hughes, A. (1977). “The topography of vision in mammals of contrasting life style: comparative optics and retinal organization,” in The Visual System in Vertebrates, ed. F. Crescitelli (Berlin, Heidelberg: Springer), 613–756.
Judkins, D. C., and Kensley, B. (2008). New genera in the family Sergestidae (Crustacea: Decapoda: Penaeidae). Phil. Biol. Soc. Wash. 121, 72–84. doi: 10.2988/06-26.1
Kamilar, J. M., and Cooper, N. (2013). Phylogenetic signal in primate behaviour, ecology and life history. Phil. Trans. R. Soc. B. 368:20120314. doi: 10.1098/rstb.2012.0341
Kilmer, J. T., and Rodriquez, R. L. (2017). Ordinary least squares regression is indicated for studies of allomtery. J. Evol. Biol. 30, 4–12.
Krøyer, H. (1855). Bidrag til kundskab om kraebsdyrslaegten Sergestes edw. Oversigt Over det Kongelige Danske Videnskabernes Selskabs Forhandlinger. 1855, 22–34.
Laughlin, S. B., van Steveninck, R. D., and Anderson, J. C. (1998). The metabolic cost of neural information. Nat. Neurosci. 1, 36–41. doi: 10.1038/236
Lindsay, S. M., Frank, T. M., Kent, J., Partridge, J. C., and Latz, M. I. (1999). Spectral sensitivity of vision and bioluminescence in the midwater shrimp Sergestes similis. Biol. Bull. 197, 348–360. doi: 10.2307/1542789
Locket, N. A. (1977). “Adaptations to the deep-sea environment,” in Handbook of Sensory Physiology, ed. F. Crescitelli (Berlin, Heidelberg: Springer), 67–192.
Marshall, N. B. (1971). Explorations in the Life of Fishes. Cambridge, MA: Harvard University Press.
Mauchline, J. (1972). The biology of bathypelagic organisms, especially Crustacea. Deep Sea Res. 19, 753–780.
McFall-Ngai, M. J. (1990). Crypsis in the pelagic environment. Am. Zool. 30, 175–188. doi: 10.1093/icb/30.1.175
Mensinger, A. F., and Case, J. F. (1990). Luminescent properties of deep sea fish. J. Exp. Mar. Biol. Ecol. 144, 1–15.
Milne Edwards, H. (1830). Description des genres Glaucothoe, Sicyonie, Sergeste et Acete, de l’ordre des Crustacés décapodes. Ann. Des Sci. Natur. 19, 333–352.
Morin, J. G. (1986). Firefleas of the sea: luminescent signaling in marine ostracode crustaceans. Florida Entomol. 69, 105–121.
Nilsson, D. E., Warrant, E., and Johnsen, S. (2014). Computational visual ecology in the pelagic realm. Philos T. R. Soc. B. 369:20130038. doi: 10.1098/rstb.2013.0038
Nilsson, D. E., Warrant, E. J., Johnsen, S., Hanlon, R., and Shashar, N. (2012). A unique advantage for giant eyes in giant squid. Curr. Biol. 22, 683–688. doi: 10.1016/j.cub.2012.02.031
Niven, J. E., and Laughlin, S. B. (2008). Energy limitation as a selective pressure on the evolution of sensory systems. J. Exp. Biol. 211, 1792–1804. doi: 10.1242/jeb.017574
Oakley, T. H. (2005). Myodocopa (Crustacea: Ostracoda) as models for evolutionary studies of light and vision: multiple origins of bioluminescence and extreme sexual dimorphism. Hydrobiologia 538, 179–192.
Ortmann, A. E. (1893). “Decapoden und Schizopoden,” in Der Ergebnisse Der Plankton-Expedition Der Humboldt-stiftung, ed. V. Hensen (Kiel and Leipzig: Lipsius und Tischer), 1–120.
Pagel, M. (1999). Inferring the historical patterns of biological evolution. Nature 401, 877–884. doi: 10.1038/44766
Paradis, E., Claude, J., and Strimmer, K. (2004). APE: analyses of phylogenetics and evolution in R language. Bioinformatics 20, 289–290. doi: 10.1093/bioinformatics/btg412
R Core Team (2020). R: A Language and Environment for Statistical Computing. Vienna, Austria: R Foundation for Statistical Computing.
Rex, M. A., and Etter, R. J. (1998). Bathymetric patterns of body size: implications for deep-sea biodiversity. Deep Sea Res. Part II Top. Stud. Oceanogr. 45, 103–127. doi: 10.1016/s0967-0645(97)00082-9
Rose, M. (1925). Contribution à l’étude de la biologie du plankton: le problème des migrations verticales journalières. Arch. Zool. Exp. Gen. 64, 387–542.
Russell, F. S. (1926). The vertical distribution of marine macroplankton IV. The apparent importance of light intensity as a controlling factor in the behaviour of certain species in the Plymouth area. J. Mar. Biol. Assoc. U.K. 14, 415–440. doi: 10.1017/s0025315400007918
Ruxton, G. D., and Johnsen, S. (2016). The effect of aggregation on visibility in open water. Roy. Soc. B. Biol. Sci. 283:20161463. doi: 10.1098/rspb.2016.1463
Schweikert, L. E., Davis, A. L., Johnsen, S., and Bracken-Grissom, H. D. (2020). Visual perception of light organ patterns in deep-sea shrimps and implications for conspecific recognition. Ecol. Evol. 10, 9503–9513. doi: 10.1002/ece3.6643
Shrimpton, S. J., Streicher, J. W., Gower, D. J., Bell, R. C., Fujita, M. K., Schott, R. K., et al. (2021). Eye-body allometry across biphasic ontogeny in anuran amphibians. Evol. Ecol. 35, 337–359. doi: 10.1007/s10682-021-10102-3
Smith, R. J. (2009). Use and misuse of the reduced major axis for line-fitting. Am. J. Phys. Anthes. 140, 476–486. doi: 10.1002/ajpa.21090
Smith, S. I. (1882). Reports on the results of dredging under the supervision of Alexander Agassiz, on the East Coast of the United States during the summer of 1880, by the U.S. Coast Survey steamer “Blake”, Commander J. R. Bartlett, U. S. N., commanding. Bull. Museum Comparat. Zool. Harvard College. 10, 1–108.
Stimpson, W. (1858). Prodromus descriptionis animalium evertebratorum, quae in Expeditione ad Oceanum Pacificum Septentrionalem, a Republic Federata missa, Cadwaladore Ringgold et Johanne Rodgers Ducibus, observavit et descripsit. Pars VIII, Crustacea Macrura. Proc. Acad. Nat. Sci. Phil. 1858, 22–47.
Sund, O. (1920). Peneides and stenopides. Rep. Sci. Res. Michael Sars North Atlantic Deep Sea Exp. 3, 1–36.
Vereshchaka, A. L. (1994). North Atlantic and Caribbean species of Sergia (Crustacea, Decapoda, Sergestidae) and their horizontal and vertical distribution. Steenstrupia 20, 73–95.
Vereshchaka, A. L. (2000). Revision of the genus Sergia (Decapoda: Dendrobranchiata: Sergestidae): taxonomy and distribution. Galathea Rep. 18, 69–207.
Vereshchaka, A. L. (2009). Revision of the genus Sergestes (Decapoda: Dendrobranchiata: Sergestidae): taxonomy and distribution. Galathea Rep. 22, 7–104.
Vereshchaka, A. L., Lunina, A. A., and Olesen, J. (2016). Phylogeny and classification of the shrimp genera Acetes, Peisos, and Sicyonella (Sergestidae: Crustacea: Decapoda). Zool. J. Linn. Soc. 177, 353–377.
Vereshchaka, A. L., Olesen, J., and Lunina, A. A. (2014). Global diversity and phylogeny of pelagic shrimps of the former genera Sergestes and Sergia (Crustacea, Dendrobranchiata, Sergestidae), with definition of eight new genera. PLoS One 9:e112057. doi: 10.1371/journal.pone.0112057
Vinterstare, J., Hulthén, K., Nilsson, D. E., Nilsson, P. A., and Brönmark, C. (2020). More than meets the eye: predator-induced pupil size plasticity in a teleost fish. J. Anim. Ecol. 89, 2258–2267. doi: 10.1111/1365-2656.13303
Warner, J. A., Latz, M. I., and Case, J. F. (1979). Cryptic bioluminescence in a midwater shrimp. Science 203, 1109–1110. doi: 10.1126/science.203.4385.1109
Warrant, E. (2000). The eyes of deep–sea fishes and the changing nature of visual scenes with depth. Philos T. R. Soc. B. 355, 1155–1159. doi: 10.1098/rstb.2000.0658
Warrant, E. J. (1999). Seeing better at night: life style, eye design and the optimum strategy of spatial and temporal summation. Vision Res. 39, 1611–1630. doi: 10.1016/s0042-6989(98)00262-4
Warrant, E. J., and McIntyre, P. D. (1990). Limitations to resolution in superposition eyes. J. Comput. Physiol. A. 167, 785–803.
Warton, D. I., Duursma, R. A., Falster, D. S., and Taskinen, S. (2012). smatr 3: an R package for estimation and inference about allometric lines. Meth. Ecol. Evol. 3, 257–259. doi: 10.1111/j.2041-210x.2011.00153.x
Warton, D. I., Wright, I. J., Falster, D. S., and Westoby, M. (2006). Bivariate line-fitting methods for allometry. Biol. Rev. 81, 259–291. doi: 10.1017/S1464793106007007
Welsh, J. H., and Chace, F. A. (1938). Eyes of deep-sea crustaceans: ii. Sergestidae. Biol. Bull. 74, 364–375. doi: 10.2307/1537810
Widder, E. A., and Frank, T. M. (2001). The speed of an isolume: a shrimp’s eye view. Mar. Biol. 138, 669–677.
Keywords: vision, bioluminescence, sighting distance, signaling, allometry, phylogenetic, Sergestidae
Citation: Schweikert LE, Thomas KN, Moreno VM, Casaubon A, Golightly C and Bracken-Grissom HD (2022) Ecological Predictors and Functional Implications of Eye Size in Deep-Sea Shrimps. Front. Ecol. Evol. 10:787315. doi: 10.3389/fevo.2022.787315
Received: 30 September 2021; Accepted: 02 February 2022;
Published: 24 March 2022.
Edited by:
Derya Akkaynak, Florida Atlantic University, United StatesReviewed by:
Julián Torres-Dowdall, University of Konstanz, GermanyKate Feller, Union College, United States
Copyright © 2022 Schweikert, Thomas, Moreno, Casaubon, Golightly and Bracken-Grissom. This is an open-access article distributed under the terms of the Creative Commons Attribution License (CC BY). The use, distribution or reproduction in other forums is permitted, provided the original author(s) and the copyright owner(s) are credited and that the original publication in this journal is cited, in accordance with accepted academic practice. No use, distribution or reproduction is permitted which does not comply with these terms.
*Correspondence: Lorian E. Schweikert, bG9yaWFuLnNjaHdlaWtlcnRAZ21haWwuY29t; Kate N. Thomas, a2F0ZS5uaWNvbGUudGhvbWFzQGdtYWlsLmNvbQ==
†These authors have contributed equally to this work and share first authorship