- 1Denali National Park and Preserve, U.S. National Park Service, Denali Park, AK, United States
- 2Central Alaska Network, U.S. National Park Service, Fairbanks, AK, United States
As climate change accelerates in northern latitudes, there is an increasing need to understand the role of climate in influencing predator-prey systems. We investigated wolf population dynamics and numerical response in Denali National Park and Preserve in Alaska, United States from 1986 to 2016 under a long-term range of varying climatic conditions and in the context of prey vulnerability, abundance, and population structure using an integrated population modeling approach. We found that wolf natality, or the number of wolves added to packs, increased with higher caribou population size, calf:cow ratio, and hare numbers, responding to a 1-year lag. Apparent survival increased in years with higher calf:cow ratios and cumulative snowfall in the prior winter, indicators of a vulnerable prey base. Thus, indices of prey abundance and vulnerability led to responses in wolf demographics, but we did not find that the wolf population responded numerically. During recent caribou and moose population increases wolf natality increased yet wolf population size declined. The decline in wolf population size is attributed to fewer packs in recent years with a few very large packs as opposed to several packs of comparable size. Our results suggest that territoriality can play a vital role in our study area on regulating population growth. These results provide a baseline comparison of wolf responses to climatic and prey variability in an area with relatively low levels of human disturbance, a rare feature in wolf habitat worldwide.
Introduction
Considerable attention has been given to the role of predators, particularly large carnivores, in driving ecosystem dynamics. In a top-down role, predators can limit herbivore abundance and activity, reducing herbivory and subsequently allowing more plant diversity and biomass which in turn supports biodiversity in other biota (Hairston et al., 1960; Terborgh, 1988; Estes, 2005; Schmitz, 2006). Through these top-down forces, predators can be seen as important components of ecological health, providing ecosystem benefits via top-down trophic cascades (Berger et al., 2001; Miller et al., 2001; Ripple and Beschta, 2004; Terborgh et al., 2006). Through opposing bottom-up forces, primary productivity can regulate consumer population abundance and in turn, their predators (Caughley, 1976; Sinclair, 1977; Houston, 1982). Evidence of trophic cascades following predator reintroductions has fostered support for conservation of large carnivores as agents improving biodiversity (Miller et al., 2001; Ripple and Beschta, 2004, 2012; Estes, 2005; Schmitz, 2006; Ripple et al., 2014, 2016). Conversely, predators’ top-down effects on ungulate populations are used as support for controversial predator control activities (Boertje et al., 1996; Titus, 2007).
Perhaps no large carnivore’s role in driving prey populations has fostered more controversy than that of gray wolves (Canis lupus). Across their extensive range, wolf density appears to be linearly related to prey biomass, supporting the theory that wolf populations exist at densities limited by food supply (Fuller, 1989; Fuller and Murray, 1998; but see Vucetich et al., 2002; Fuller et al., 2003). This relationship suggests that at a global scale, wolf populations are limited by bottom–up forces driven by primary productivity and herbivore densities (Oksanen and Oksanen, 2000). However, there is substantial evidence suggesting that wolves exert top-down control of prey populations, as wolves can depress prey abundance over large spatial and temporal scales (Gasaway et al., 1983, 1992; Adams et al., 1995; Boertje et al., 1996, 2010; Crête, 1999; Mech and Peterson, 2006). The influence of top-down controls are difficult to tease apart from bottom up and climatic influences, even in manipulative experiments (Gasaway et al., 1983; Boertje et al., 1996; Vucetich et al., 2005; Keech et al., 2011; Valkenburg et al., 2016; Allen et al., 2017).
There is ample evidence that the role of top-down control of ungulates by wolves is nuanced, and factors such as weather conditions and prey age structure alter vulnerability of prey (McRoberts et al., 1995; Mech et al., 1998; Vucetich et al., 2005; Valkenburg et al., 2016). Ultimately, it is vulnerability to predation that determines which and how much prey is availability to wolves. Climatic factors play an important role in prey availability, as wolves tend to be more successful when winters are severe in terms of heavy snowfall and cold temperatures (Peterson and Allen, 1974; Peterson and Page, 1988; Mech et al., 1998). Snowpack affects the vulnerability of prey directly by covering possible food sources and restricting prey movement. Indeed, snow depth has been considered the most important landscape attribute affecting ungulate movement and mobility (Wallmo and Gill, 1971; Hugie, 1973; Telfer, 1978). Snowpack can also affect prey indirectly; for example, in years following severe winters, caribou are more susceptible to predation, in part because of poor nutrition during the natal period affecting the susceptibility of yearling caribou (Peterson, 1977; Mech, 1991). Ungulate availability can also fluctuate from a variety of factors in addition to snowpack such as disease, other climatic variables, available forage, and ungulate age structure (Klein, 1991; Valkenburg et al., 2016). When ungulates are in poor condition or exhibiting density dependent limitations, wolves may be the proximate but not necessarily the ultimate cause of ungulate mortality (Murie, 1944; Vucetich et al., 2005; Mech and Peterson, 2006). Therefore, the role that wolves play in exerting top-down controls and limiting ungulate populations is context dependent or may be compensatory.
Although wolves are considered obligate consumers of ungulates (Peterson and Ciucci, 2006), they are opportunistic predators and subsidies from alternate prey may play a role in wolf density and wolf-prey dynamics (Adams et al., 2010; Gable et al., 2018). Where available, hares (Lepus spp.) may be a particularly important prey for wolves during summer months (Mech, 2004; Haber and Holleman, 2013; Newsome et al., 2016). While pups are too young to travel with the pack in the summer, adult pack member range away from homesites (dens and rendezvous) to hunt for food and return to feed pups. Pack cohesion is lower in the summer (Benson and Patterson, 2015), perhaps because traveling separately increases efficiency in hunting smaller prey that is available in summer (Mech et al., 1998). In the High Arctic, wolves rely heavily on hares where the only other prey are muskox (Mech, 2004) and near 100% of pup survival variation is due to availability of small prey (Mech, 1995). Other studies have postulated about the role of hares in providing nutritional subsidy for wolves in a sub-arctic population, but the effect of hare abundance on wolf demographics has not been quantified (Murie, 1944; Mech et al., 1998; Haber and Holleman, 2013).
Much of what we know about wolf population dynamics and relationships between wolves and prey comes from long term studies (Mech, 1966, 1986; Mech et al., 1998; Smith and Bangs, 2009; Nelson et al., 2011). Long term studies are particularly important because the conclusions regarding predator-prey dynamics can drastically change based on the time-period studied (Nelson et al., 2011). Large terrestrial mammals can be difficult and expensive to study especially when they exist at low densities and are wide ranging (Estes, 1996) and these long-term studies represent a significant investment and body of work. The wolf population in and around Denali National Park and Preserve (hereafter, Denali) has a long history of research and relative protection from harvest (Murie, 1944; Mech et al., 1998). This makes the Denali wolf population unique worldwide and valuable as a conservation baseline (Borg and Burch, 2014; Borg et al., 2015).
We explored wolf population dynamics in Denali from 1986 to 2016 in the context of prey vulnerability and population structure using the powerful integrated population modeling approach developed by Schmidt et al. (2015, 2017). The overall goal of this study was to identify prey population characteristics associated with variation in wolf vital rates to better understand the relative roles of wolves and their prey as system drivers. A prior analysis of wolves in Denali documented a period with above average snowfall, high wolf populations and major changes in wolf and caribou numbers (Mech et al., 1998). In contrast, subsequent decades have been characterized by mild winters, a decreasing wolf population and increasing prey base. Here, we examine 30 years of variation in weather and prey populations to identify underlying decadal trends in these factors and their impacts on wolf demographics.
Our primary objectives were to: (1) estimate wolf vital rates for the study population, (2) quantify the effects of prey population size, productivity, and indices of vulnerability (i.e., winter weather) on wolf demographic rates, and (3) quantify the potential role of secondary non-ungulate prey resources (i.e., snowshoe hares) on wolf vital rates. We hypothesized that prey productivity and weather-driven prey vulnerability, rather than raw abundance, were the ultimate drivers of wolf population dynamics in the Denali ecosystem. Finally, we expected that snowshoe hares might play an important role in subsidizing wolf populations during cyclic population highs due to the large increase in available biomass during cyclic peaks. We expected that the overall numbers of hares and caribou in the current year would be representative of prey abundance, while snow depth and caribou fall calf:cow ratio in the previous year, would serve as indices of prey vulnerability. We based these assumptions on findings that young ungulates often comprise a large proportion of wolf diets (Murie, 1944) and that heavy snows increase the vulnerability of ungulates to predation by wolves (Mech et al., 1998, 2001).
Materials and Methods
Study Area
The study area encompassed approximately 17,270 km2 of wolf habitat primarily north and west of the Alaska Range in and adjacent to Denali National Park and Preserve (Figure 1) and ranged in elevation from 150 to 3,000 m. The eastern region of Denali contains habitat patches of boreal forest, high alpine, open gravel river bars, and willow-lined creeks. The western region of the park is more homogenous, dominated by relatively flat, lowland black spruce (Picea mariana) forest and long meandering rivers and wetlands.
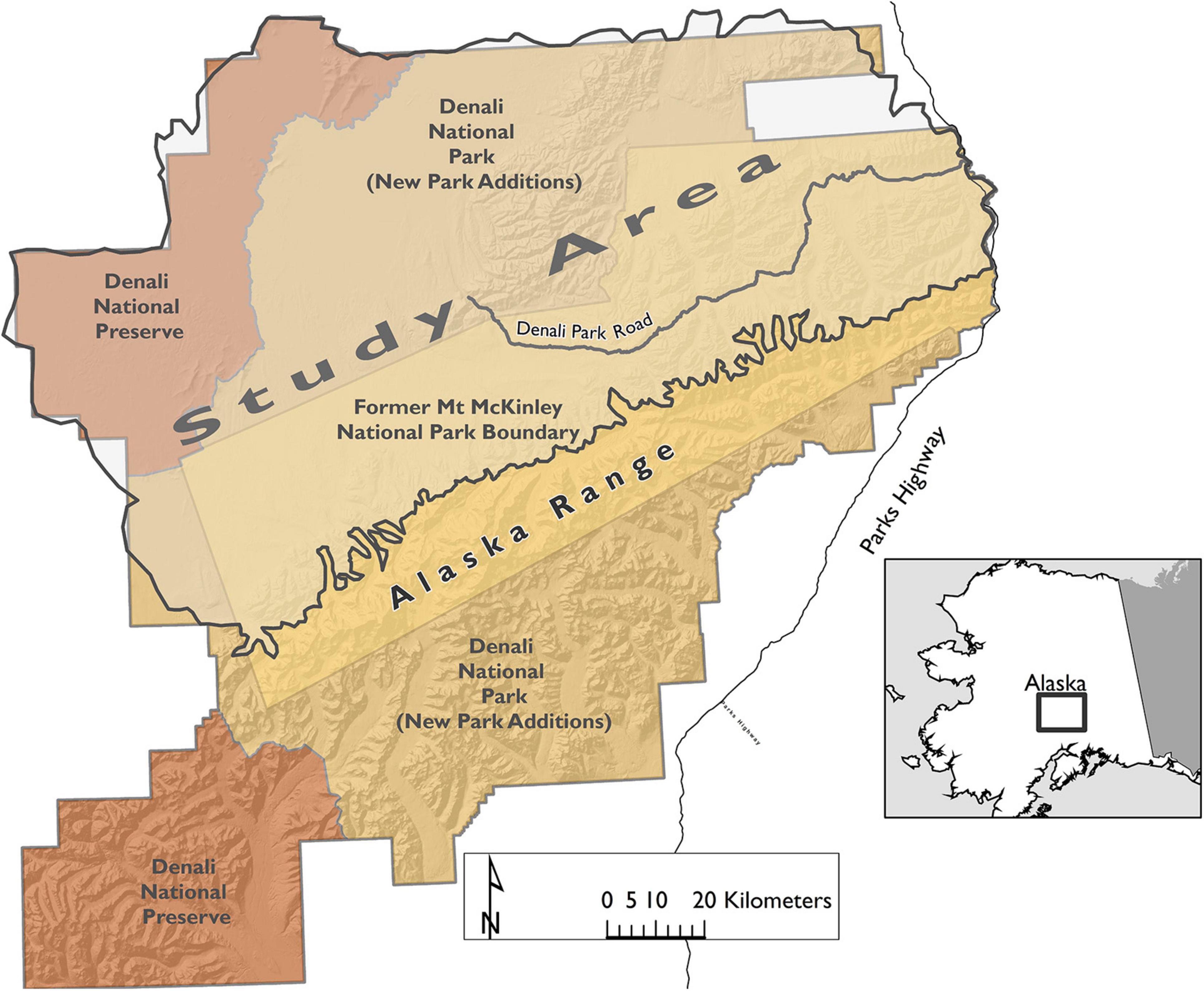
Figure 1. Wolf project study area includes all areas north of the Alaska Range within Denali National Park and Preserve, Alaska, United States. The new park additions are north and south of the original park boundary (tan) and the Preserves are in the northwest and southwest (orange). The study area is denoted by shaded area.
The climate in Denali is sub-arctic and subject to wide variations in temperature and precipitation. On the north side of the Alaska Range, a snow-shadow effect predominates, resulting in low amounts of precipitation year-round and continental interior climate patterns generated by the High Arctic prevail in this region (Sousanes, 2006). At Denali headquarters (within the study area) temperature extremes range from 33 to −48°C. Daylight varies throughout the year with more than 20 h in June to 4 h in December. Summers are short and warm, and winters are long and cold with snow cover generally present October through early May. The average high temperature in July is 19°C, and the average low temperature in January is −21°C. Total annual precipitation is relatively low and averages ∼ 38.2 cm. Most of the precipitation (20.5 cm) falls as rain during the summer months (Sousanes and Hill, 2017). Cumulative winter snowfall on the north side of the mountain ranged from 21 to 394 cm from 1986 to 2016 (Table 1; NOAA Regional Climate Centers, 2021).
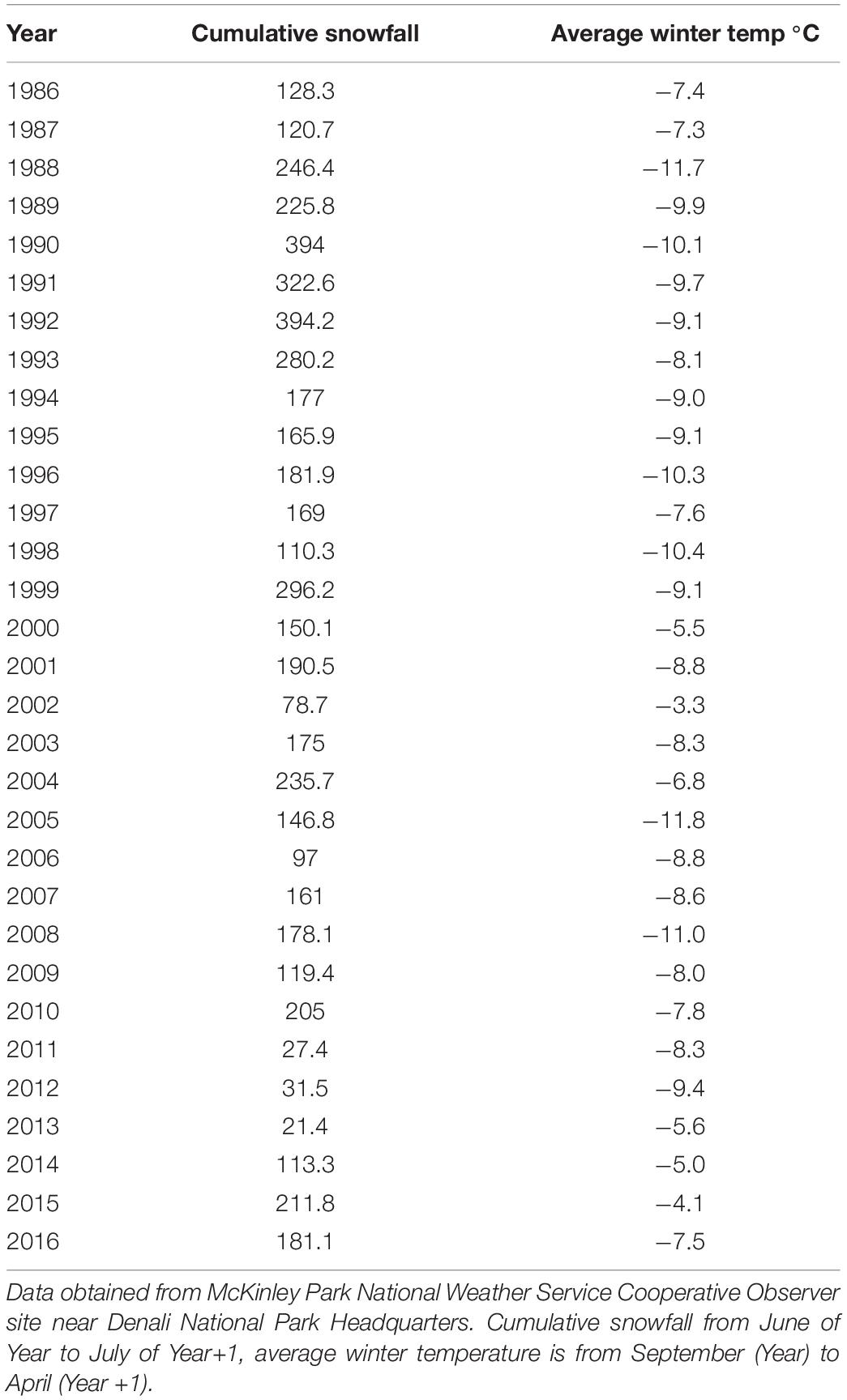
Table 1. Cumulative year snowfall and average winter temperature in Denali National Park and Preserve, Alaska, United States.
Prey Numbers and Distribution
The diversity of habitat types in the eastern region of Denali supports resident populations of caribou (Rangifer tarandus), Dall’s sheep (Ovis dalli), and moose (Alces alces) which constitute the main prey base for wolves in the region (Murie, 1944; Mech et al., 1998). High winds in the Outer Range (northwest of the Alaska Range) in winter months tend to result in wind-scoured ridges, leaving areas with relatively low snowpack and exposed vegetation. These conditions provide favorable wintering grounds for caribou, Dall’s sheep, and moose (320 moose-equivalents/1,000 km2, Adams et al., 2010). The western lowlands support lower densities of ungulates (primarily moose at 70 moose-equivalents/1,000 km2, Adams et al., 2010), and salmon are an important food source for wolves in this region (Mech et al., 1998; Adams and Roffler, 2009; Owen and Meier, 2009; Adams et al., 2010). Throughout the study area, small mammals such as snowshoe hares and arctic ground squirrels are locally abundant and can represent a large amount of available biomass available to the predator community (Boonstra et al., 2001). Wolves in the study area are known to prey on snowshoe hares (Lepus americanus), Arctic ground squirrels (Spermophilus parryii), hoary marmots (Marmota caligata), beaver (Castor canadensis) and various birds (Murie, 1944; Mech et al., 1998). We used prey population data collected by concurrent studies in Denali (specified below) as covariates in our analyses to explain variation in wolf demographic rates.
Caribou
The caribou population in Denali is predominantly composed of members of the Denali Caribou Herd (DCH). The DCH exhibits a local seasonal migration of about 80 Km from summering and winter ranges. Calving season begins in late April and early May, with peak calving typically occurring synchronously in mid-May. Calving grounds are generally located in high elevations near glaciers and snowfields near the foothills of the Alaska Range, and winter range includes a large area of typically wind-swept slopes near the northeast corner of Denali (Figure 2).
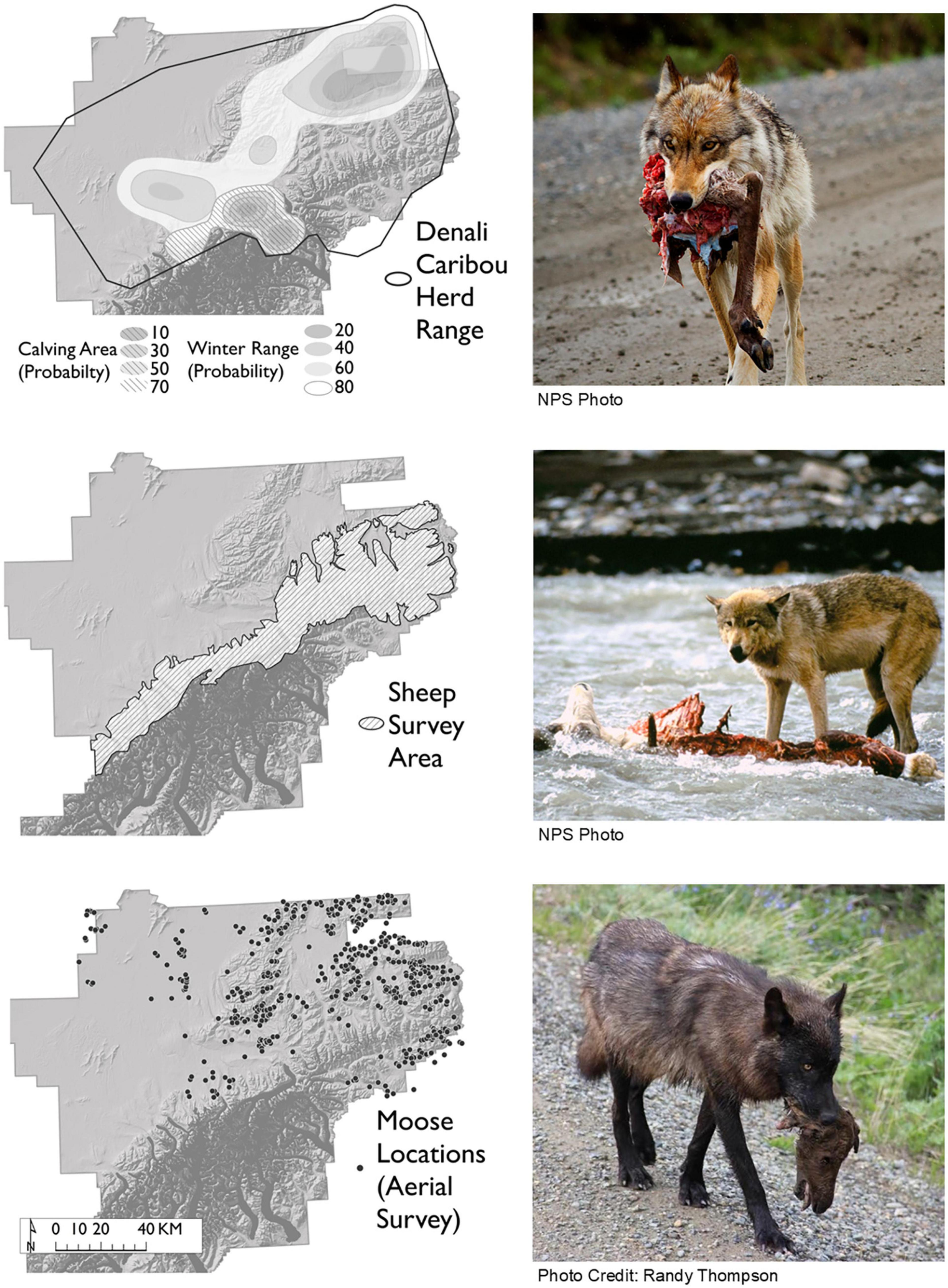
Figure 2. Approximate distribution for three ungulate species that compose the main prey base for wolves within the Denali National Park and Preserve, Alaska, United States. Denali Caribou Herd range was derived from caribou distribution data from 1986 to 2007 (Adams and Roffler, 2010), calving distribution and winter range isopleths were derived from caribou location data from 1986 to 1996 (Schirokauer and Adams, unpublished data). Dall’s sheep range is represented by the aerial survey area designed to cover a majority of sheep habitat. Moose distribution is indicated by the locations of moose groups located during aerial surveys conducted in November 2008 and 2011 (Owen and Meier, 2009; Meier and Owen, 2011). Corresponding photos show wolves feeding on each of the three ungulate species. Top two photos NPS Photos.
The DCH has been monitored since 1984 and current protocols for monitoring the caribou population have been in place since 1986. Each year, caribou were captured by helicopter darting and radio-marked to monitor their survival, productivity and movements, and to aid in conducting composition surveys and herd counts (Adams and Roffler, 2009). Population estimates were derived annually from aerial composition and count surveys. For detailed methods on capture, radio collaring, composition, and population estimation see Adams and Meier (2018). Denali Caribou Herd size estimates from 1986 to 2016 ranged from 1,760 to 3,210 animals, with an average of 2,269 (SE 68.7) from 1986 to 2016. Calf:cow ratios ranged from 6.4 to 38 calves: 100 cows and averaged 19.6 calves:100 cows (SE 1.5, Adams, 2017).
Dall’s Sheep and Moose
The Dall’s sheep population in Denali occurred in alpine areas within the Alaska Range (Figure 2), and abundance estimates ranged from 1,374 to 2,288 during the course of our study. Sheep population estimates were obtained from aerial census in 1996 (Putera and Keay, 1998) and aerial distance sampling surveys in 2011 and 2013 (Schmidt and Rattenbury, 2013). Moose occurred in relatively low density throughout the study area, with greater density occurring in the north eastern region of the Denali (Owen and Meier, 2009; Figure 2). From 1986 to 2004, moose abundance estimates were obtained from aerial censuses using a stratified random sampling technique (Gasaway et al., 1986; Meier, 1986; Meier et al., 1991; Belant and Stahlnecker, 1997; Fox, 1997; Belant et al., 2000). Moose population estimates ranged from 1,104 to 2,168, averaging 1,677 (SE 121.0). Moose density estimates in the northern study area averaged 0.175 moose/km2 (range: 0.13–0.24) and calf: cow ratios averaged 26 calves:100 cows (range: 0.22–0.39) (P. Owen, unpublished data).
Moose and sheep abundance data were too sparse for use as covariates in our analysis, although the available moose population estimates at least suggested a population trajectory similar to that of the caribou population (Figure 3). Salmon as a food source was assumed to be a relatively consistent seasonal subsidy (Adams and Roffler, 2010).
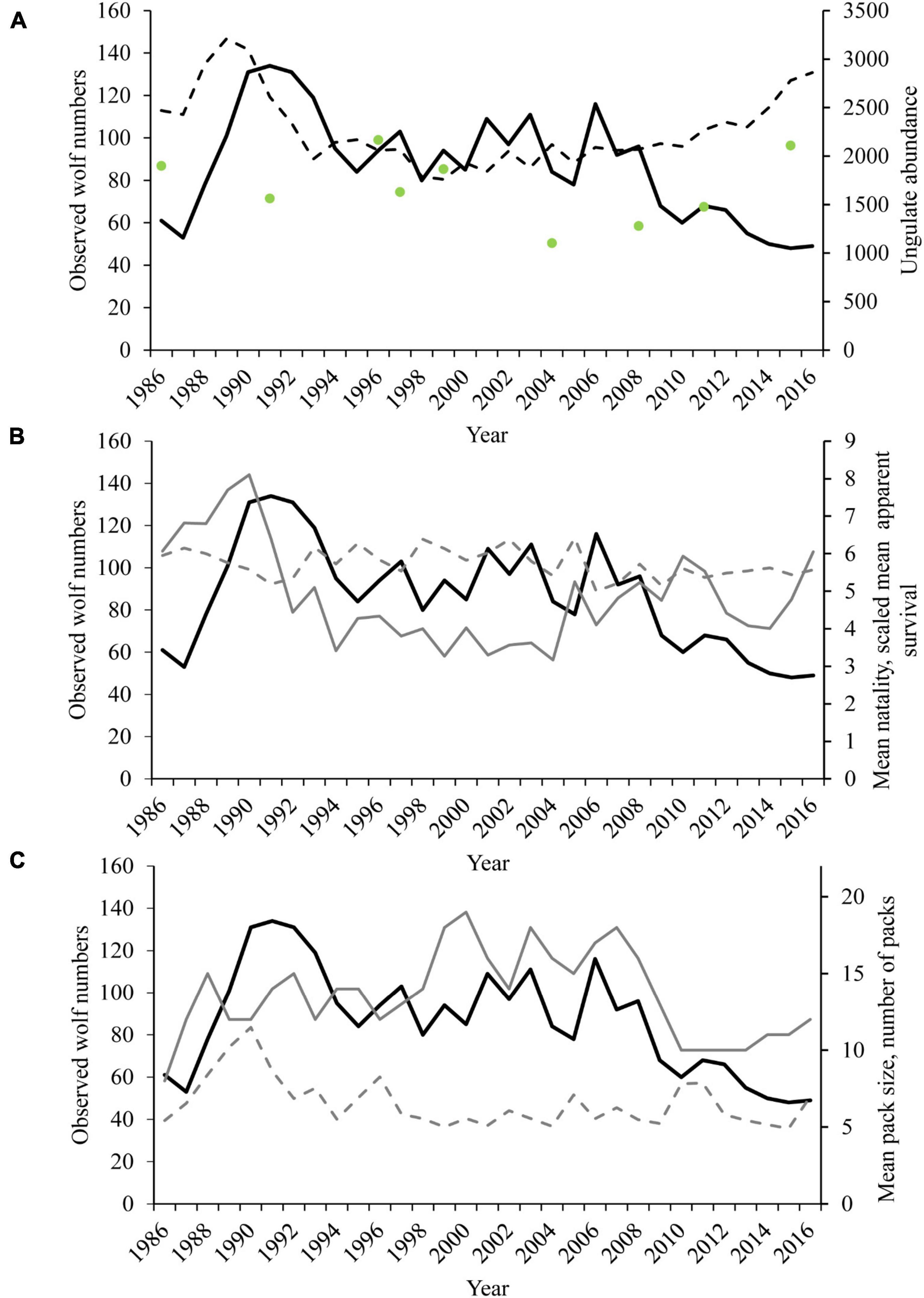
Figure 3. The annual minimum number of wolves known to exist (solid black lines) and (A) Estimates of caribou (dashed line) and moose (green dots) abundance and (B) Mean natality (solid gray line), scaled mean apparent survival (dotted gray line, scaled apparent survival = mean apparent survival multiplied by a constant [8]) and (C) number of packs (solid gray line) and mean pack size (dotted gray line) in Denali National Park and Preserve, Alaska, United States from 1986 to 2016.
Snowshoe Hare
Snowshoe hares occurred throughout the study area, although relative abundance fluctuated wildly among years reflecting the regular 9–11 year population cycle of this species (Krebs et al., 2013). Relative abundance was indexed using the average number of adults observed per day during routine field work (McIntyre and Adams, 1999; McIntyre and Schmidt, 2012; Schmidt et al., 2018a). There was considerable variation in the amplitude of the cycle peaks, with the third hare peak observed during our study period (2006–2009) being approximately fourfold larger than the previous two peaks (Schmidt et al., 2018b).
Data Collection
There is a long history of wolf research and monitoring in Denali, with research beginning in 1939 (Murie, 1944). The use of radio-telemetry for tracking and monitoring packs began in 1986 (Mech et al., 1998), and the wolf population has been continuously monitored since that time. While the wolf population within Denali represents a wolf population with relatively little human exploitation (Mech et al., 1998; Adams et al., 2010), all areas outside of the Denali boundary were open to hunting and trapping (collectively called “harvest”) under state regulation.
Beginning in 1986, we attempted to maintain collars on two or more wolves in each pack whose home range was mostly within Denali boundaries. Wolves were immobilized by darting from helicopters and collared following protocols described in Meier et al. (2009). From 1986 to 2016, 421 individual wolves were captured and radio-collared (radio-marked) with very high frequency (VHF) collars. From 2003 to 2016, 86 of the VHF collars were equipped with GPS (Telonics, Mesa, CA, United States) which provided daily locations uploaded through the Argos or Iridium satellite system (Meier et al., 2009).
We noted estimated age and breeding status during capture and collaring operations (for details see Meier et al., 2009; Schmidt et al., 2017). Additional monitoring of collared wolves and pack mates during radio tracking flights through denning, pup-rearing and subsequent seasons allowed for additional confirmation of breeding status. In instances where breeding status was not noted (in early capture records), we identified breeders following the methods in Borg et al. (2015). Some packs were monitored during the course of the study after being captured in or near the study area but were ultimately determined to not reside within the study area. These packs were generally poorly monitored, due to lack of radio-tracking flights in their vicinity and were censored from our analyses. New and establishing packs were located during aerial tracking and efforts were made to radio collar members from each pack once considered to be a resident pack within the study area. A small number of wolves may reside in newly establishing packs at any given time prior to marking.
We located radio-marked wolves by VHF signal from fixed-wing aircraft roughly twice a month and recorded location, number of pack members, pelt colors, estimated age classes (if distinguishable), and any data on prey killed or eaten. We also recorded detailed information on mortality, den site location/use, and pack affiliation (Mech et al., 1998; Meier et al., 2009). Radio-marked wolves were lost from our study either due to mortality (e.g., natural, harvest, capture related, or unknown) or dispersal. We noted mortalities of collared wolves during aerial tracking and observation and (from 2003 to 2016) through weekly GPS data checks. Cause of death was determined through a field necropsy or by wildlife veterinary staff at the University of Alaska Fairbanks (UAF) or the Alaska Department of Fish and Game (ADF&G). Natural causes of death primarily included being killed by other wolves or starvation, but also included avalanches, drowning, or unknown causes. When carcasses were too decomposed to determine cause of death or both lab and field evidence were inconclusive, and there was no evidence of human interference, we recorded cause of death as “unknown natural” and fate was categorized as natural. Hunting and trapping were a primary source of human-caused mortality. A small number (n = 10) mortalities were attributed to capture events, either directly (e.g., dart injury) or indirectly (e.g., harvested or killed by other wolves while still sedated, or died from infection related to heart valve defect). We classified wolf fate to the unknown category when a fate could not be determined, either due to loss of contact or carcass recovery long after the mortality event.
The dispersal category included known dispersals of radio-marked wolves and instances where an entire pack “shifted” out of the study area. Pack shifts occurred when all known members of a pack moved from a previously held territory within the study area to new territory outside of the study area (n = 4). Packs outside of the study area were difficult to monitor due to logistical constraints, typically resulting in loss of contact with the pack. These members were censored from the time of dispersal or pack shift from the study population for the purpose of our apparent survival analyses.
Data Analysis
We estimated survival and natality rates using an integrated population modeling approach incorporating known-fate information from collared individuals and repeated counts of unmarked pack mates (Schmidt et al., 2015, 2017). We used the model structure presented by Schmidt et al. (2017) which combined cause-specific known-fate (Royle and Dorazio, 2008; Schmidt et al., 2010) and open N-mixture (Dail and Madsen, 2011) sub-models for the collar and count data, respectively.
Use of this integrated framework allowed us to make direct inference to the entire population of wolves in our study area, without relying on the assumption of a representatively marked subsample (Schmidt et al., 2017). This was important because suspected breeders were targeted for marking when possible and tended to accrue in the marked population over time due to higher survival and lower rates of dispersal. Most of the wolves in the population were unmarked, therefore formally including them in the analysis increased our power to assess annual variation in population parameters in the context of explanatory covariates. Finally, the integrated approach also accounted for temporal variation in resighting effort. This was important because variable weather conditions, funding, or other logistical challenges resulted in fluctuating sighting effort over time. Together these features provided much stronger inference than would be possible using simple known-fate data and unadjusted counts (Schmidt et al., 2015, 2017).
We estimated the probability of mortality and dispersal based on the collared subset of the population. We present estimates of true survival and dispersal as overall means because cause-specific losses were only available from our relatively small sample of collared wolves. Because the cause of loss (i.e., mortality vs. dispersal) was unknown for the much larger unmarked subset of wolves, we also estimated apparent survival, the probability of surviving and not dispersing, for the unmarked sample. We considered dispersal to be equivalent to mortality in terms of apparent survival because the individual was effectively lost from the population. We were primarily interested in the relationship between wolf population dynamics and a suite of covariates, which in the absence of cause specific mortality for the much larger unmarked subset of the population limited us to assessments of apparent survival.
Local dispersal events (i.e., an individual switching from one monitored pack to another or forming new packs) were observed in the marked sample throughout the study (n = 34) potentially affecting parameter estimates. We expect that these local dispersers would cause some upward bias in natality rates, and possibly limited negative bias in apparent survival rates. This is because while the local disperser was lost from the original pack, it was not lost from the study population. Some portion of the potentially negative pressure on estimates of apparent survival was likely mitigated by concurrent losses in the accepting pack during the interval between observations. Overall, local dispersals represented <10% of all losses of collared individuals, suggesting any bias would be limited (see section “Results”).
In instances where contact with a radio-marked wolf was lost, the period after loss of contact was right censored. If there was a lapse in time between the interval in which the wolf was last seen alive and the interval when the mortality was detected, the timing of mortality was estimated (Royle and Dorazio, 2008). With the advent of GPS collars, determining the exact date of death was more accurate and therefore estimation of date of death was less frequent after 2003. For wolves that were collared and died in the same interval (month), we assumed they were alive on the first of the month prior to the collaring and died over the interval in which collaring occurred. Radio-marked wolves that were not a member of a pack, as indicated by one or more visual confirmations of the wolf alone and without evidence of affiliation with known packs or other individual wolves were censored from the survival analyses.
As in Schmidt et al. (2017), we formulated our model based on the biological year (BY) starting in May of the current year t through April of the following year t+1. Packs were open to additions over the May-August interval, reflecting primarily pups born in May and recruited over the 3 month interval (i.e., natality). While we assume that most of these additions were of pups, some additions may have resulted from dispersing adults being accepted into existing packs. We estimated monthly apparent survival throughout the BY as well.
Our model structure directly followed that of Schmidt et al. (2017). For collared individuals, the observed state, Yit, is modeled as:
where the state for individual i at time t depends on the state at t–1 and apparent survival probability, . The models for the probabilities of surviving, , and not dispersing, , can be written as:
where xi,t are covariates and β are coefficients. For the counts of unmarked pack-mates, the surviving number of individuals, Sj,t, within each pack, j, as:
where Ri,t−1 indicates the number of individuals newly marked and then transferred to the known-fate sample. As above, we modeled in 2 parts as
where xi,t is a vector of covariates and β* represents the coefficients. Note that components of β and β* were shared (i.e., data integration). The additions, Bj,1, to each pack, j, during the May–August interval can be written as:
where γj represents the number of individuals recruited into each pack. To include covariate information, wj, natality can be parameterized as:
where ρ represents the coefficients. The counts can be modeled as:
where the observed number of individuals, nj,t, is a function of true abundance, Nj,t, minus any individuals transferred to the known-fate sample, Rjt, and detection probability, p. For packs and months when counts were not certain, we modeled p as a random effect allowed to vary by month. Please see Schmidt et al. (2015, 2017) for additional details on model structure and fitting. JAGS code for this implementation is available on FigShare (see section “Data Availability Statement”).
We considered a suite of covariates that we expected to explain variation in apparent survival and natality rates. We began by assuming that the components of apparent survival would vary by month and that individuals identified as breeders would remain in packs at higher rates than other wolves (i.e., Schmidt et al., 2017). Breeder status was assigned to radio-marked individuals on a yearly basis. If an individual wolf was identified as a known breeder in year t, and died before whelping in year t+1, it was assigned as a breeder in year t+1. This was intended to apply the covariate of breeding status to an individual wolf’s survival risk, regardless if it had the opportunity to breed in the given season.
We also included covariates related to prey abundance and availability: harest, herd sizet, calf ratiot–1, and snow deptht–1. Because pups are generally born in May, we considered harest–1, herd sizet–1, calf ratiot–1, and snow deptht–1 as potential covariates on natality, assuming that conditions prior to whelping would be related to natality. We also assumed that the loss of a breeder in BYt–1 would negatively affect natality rates (Borg et al., 2015; Schmidt et al., 2017).
Results
We monitored 8–19 wolf packs annually (Table 2) and analyzed data from 379 radio-marked wolves from 73 packs monitored between BY1986 -2016. Our sample included 194 (51%) females and 185 (49%) male wolves. Marked wolves remained in the sample an average of 1.5 years for a total of 1,151 collared wolf-years in the sample. All age classes were represented in the sample (Table 3) although older age classes were more frequent in the sample because collaring efforts targeted older individuals as they were more likely to stay within the study area. We identified 182 wolves as suspected or confirmed breeding members of the pack. On average, wolves were breeders in their pack for 2.8 years, with a maximum of 10 years.
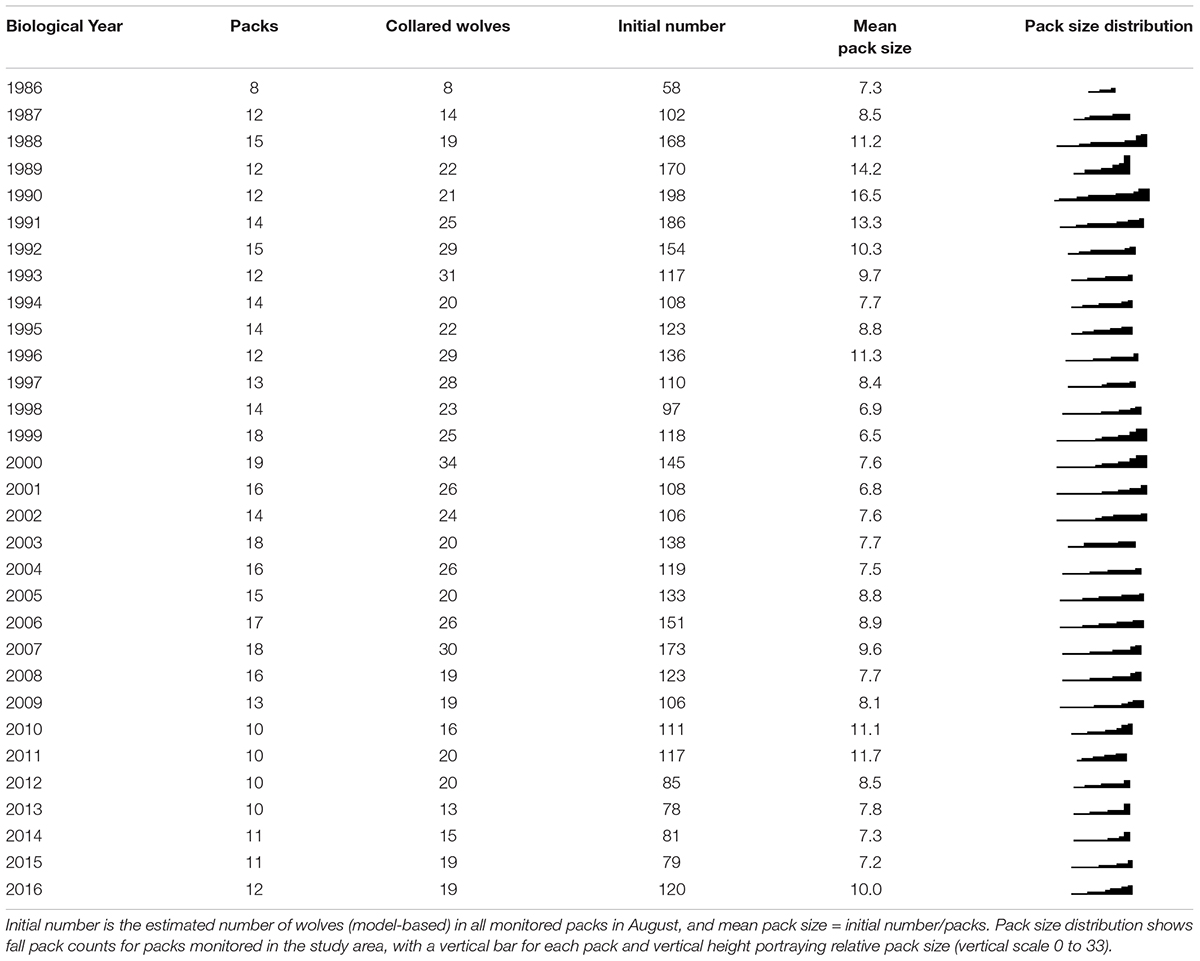
Table 2. Numbers of packs monitored and wolves marked with radio-collars for biological year (May–April) in Denali National Park and Preserve, Alaska, United States.
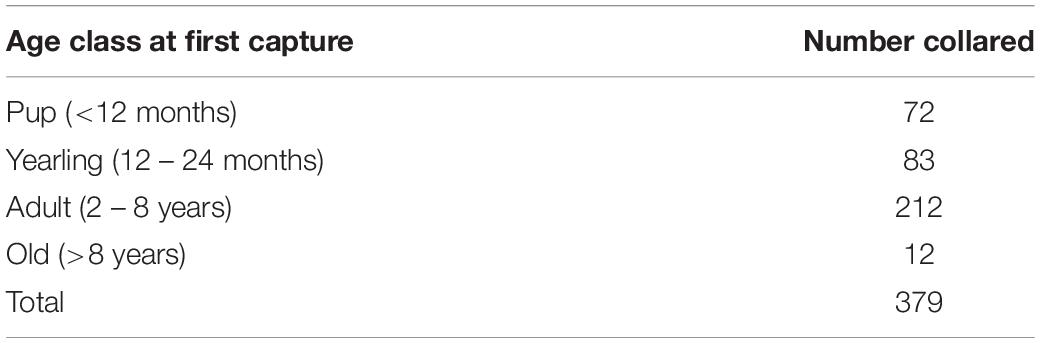
Table 3. Number of wolves by estimated age at first capture in Denali National Park and Preserve, Alaska, United States between biological years 1986 to 2016.
In the collared sample, more wolves were lost to death by natural causes (i.e., intraspecific strife, starvation, drowning) than any other fate (52% natural causes, Supplementary Figure 1). Harvest-related mortalities, unknown cause of death, and losses from packs due to dispersal occurred at similar rates in the collared sample (harvest 15%, dispersal, 16%, Supplementary Figure 1). Capture-related mortalities were rare, only occurring in 10 out of 421 capture events. After accounting for unknown fates of dispersing wolves, the majority were ultimately harvested (49%, Supplementary Figure 1).
The probability of detecting unmarked wolves during pack counts was lower during the late summer/early fall months (May–September), corresponding to periods with little or no snow cover and the presence of obscuring vegetation (Supplementary Figure 2A). Probability of detection was consistently high in winter months, peaking in March when increased daylight hours, snow cover, and increased flight efforts related to capture operations improved detection. Monthly survival probabilities were high and relatively consistent throughout the year (Supplementary Figure 2B). Dispersal was more likely to occur in the summer (May–August) or late winter [February, corresponding to the pre-breeding season (Borg et al., 2015)] and was less likely during the early to mid-winter months (Supplementary Figure 2C).
Based on the basic integrated model with no covariates and no random effects, mean annual apparent survival probability was 0.65 (0.61, 0.68) for known breeders and 0.48 (0.45, 0.51) for other wolves (Supplementary Figure 3). Mean annual survival probability (dispersal excluded) for known breeders (0.68 [0.65, 0.72]) was slightly higher than estimates for other wolves (0.63 [0.59, 0.67]) and mean estimated annual dispersal probability was much lower for known breeders (0.05 [0.03, 0.07]) than for other wolves (0.24 [0.21, 0.29], Supplementary Figure 3).
Several covariates explained variation in wolf apparent survival. Cumulative snowfall in the preceding winter and higher calf:cow ratios in the DCH were associated with higher apparent survival in wolf packs (Figures 4, 5). In contrast, the size of the DCH and the index of hare abundance were negatively related to apparent survival although the confidence interval for the effect of hare abundance slightly overlaps 0 (Table 4 and Figures 4, 5).
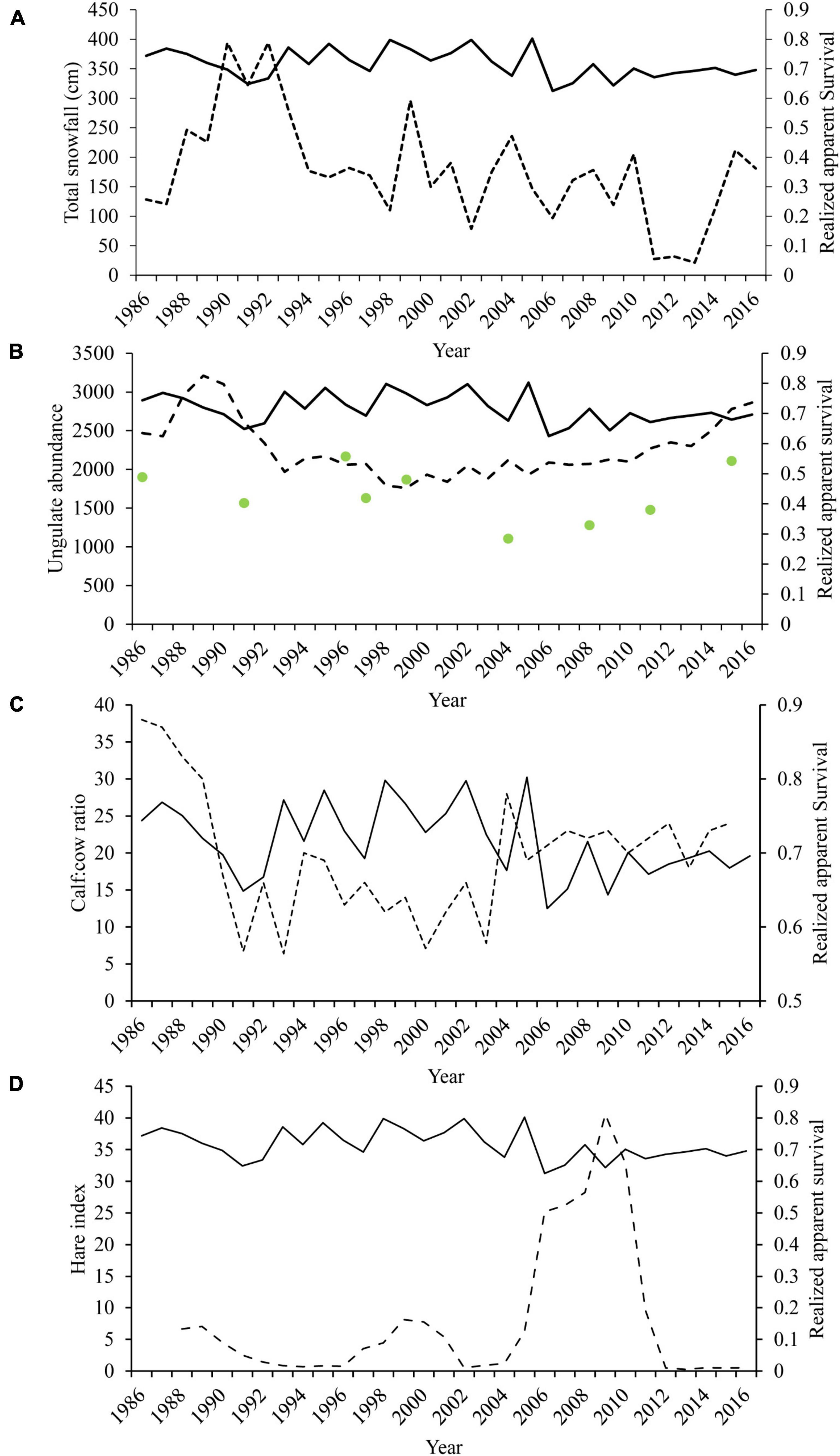
Figure 4. Estimated annual apparent survival of wolves (a proportional combination of known breeders and wolves of unknown status) in Denali National Park and Preserve, Alaska, United States for biological years 1986–2016. Annual estimated apparent survival (solid lines) is plotted in relation to (A) total annual snowfall (in cm), (B) caribou (dashed line) and moose (green dots) abundance, (C) caribou cow:calf ratios (dashed line), and (D) the snowshoe hare abundance index (dashed line).
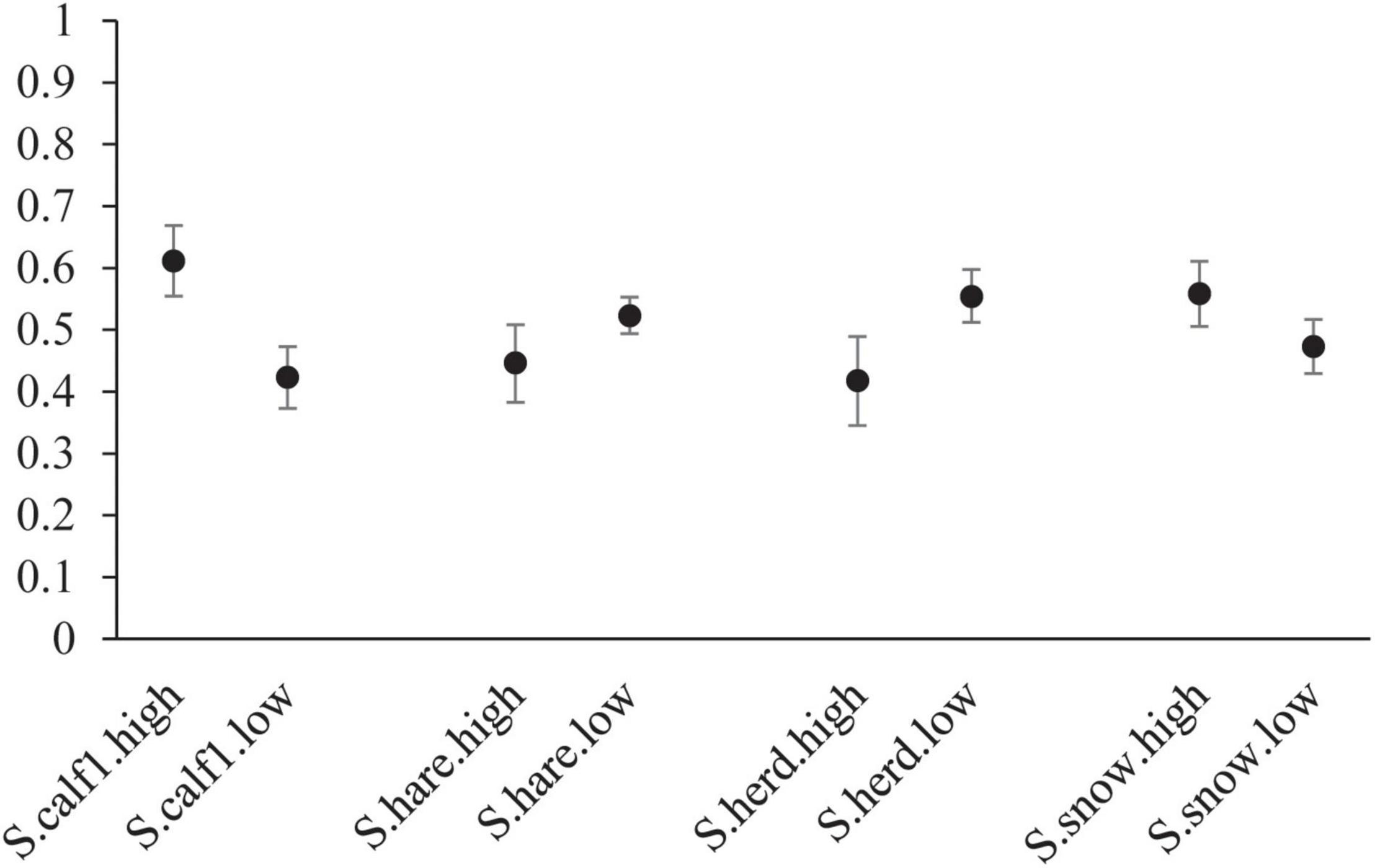
Figure 5. Comparison of covariate values on estimated survival for wolves in Denali National Park and Preserve, Alaska, United States. Conditions included are: high caribou calf:cow ratio in the preceding year (S.calf.high), low caribou calf:cow ration in the preceding year (S.calf.low), high hare index in the current year (S.hares.high), low hare index in the current year (S.hares.low), high caribou herd size in the current year (S.herd.high), low caribou herd size in the current year (S.herd.low), high snowfall in the previous winter (S.snow.high), and low snowfall in the previous winter (S.snow.low). Values used represent the range of values observed during our study. Error bars represent 95% credible intervals. For each scenario all other covariates are held at mean values.
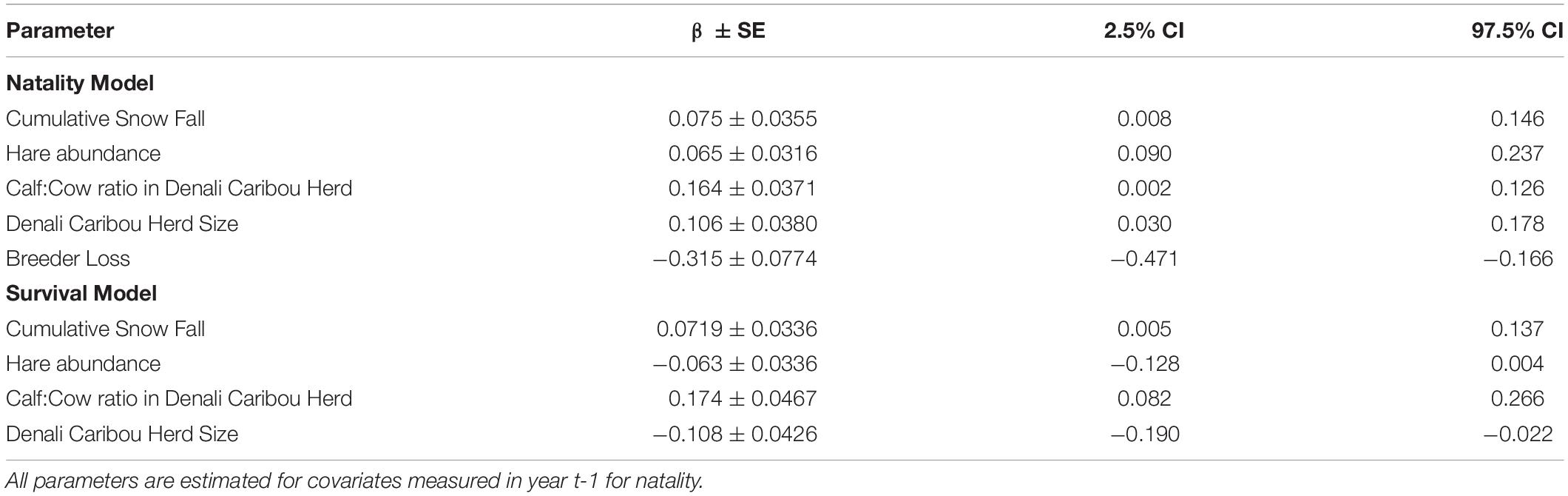
Table 4. Parameter estimates for models evaluating the effect of covariates on wolf natality (number of wolves added to the population) and survival (number of wolves lost from the population) over three decades in Denali National Park and Preserve, Alaska, United States.
Metrics of prey abundance and availability also influenced wolf natality. Cumulative winter snowfall in the winter preceding was positively related to wolf natality (Table 4) with peak natality occurring following a winter of severe snowfall in winter 1989-90 (Figures 6, 7). The DCH calf:cow ratio and herd size in the previous year were positively associated with the number of wolves added to packs in the spring. The index of hare abundance was also positively associated with wolf natality (Table 4 and Figure 7). Conversely, the loss of a breeder in the preceding year decreased natality rates for wolf packs (Table 4).
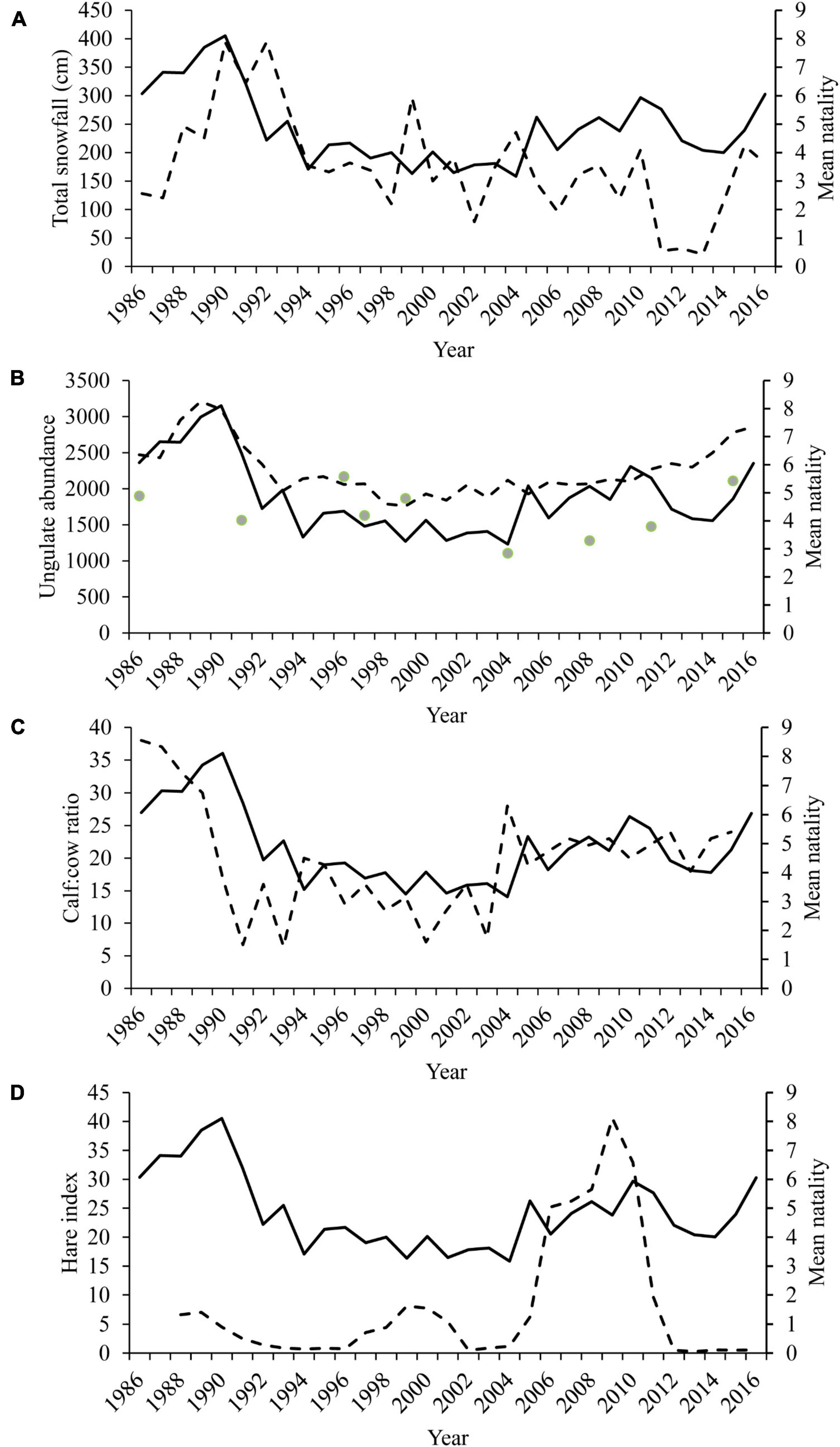
Figure 6. Estimated mean number of individuals added to each pack annually (mean natality; solid line) in Denali National Park and Preserve, Alaska, United States for biological years 1986–2016. Natality (mean natality, solid line) is plotted in relation to (A) observed total snowfall (dashed line) in the winter immediately preceding each biological year, (B) caribou (dashed line) and moose (green dots) abundance, (C) caribou cow:calf ratio (dashed line), and (D) the snowshoe hare abundance index (dashed line).
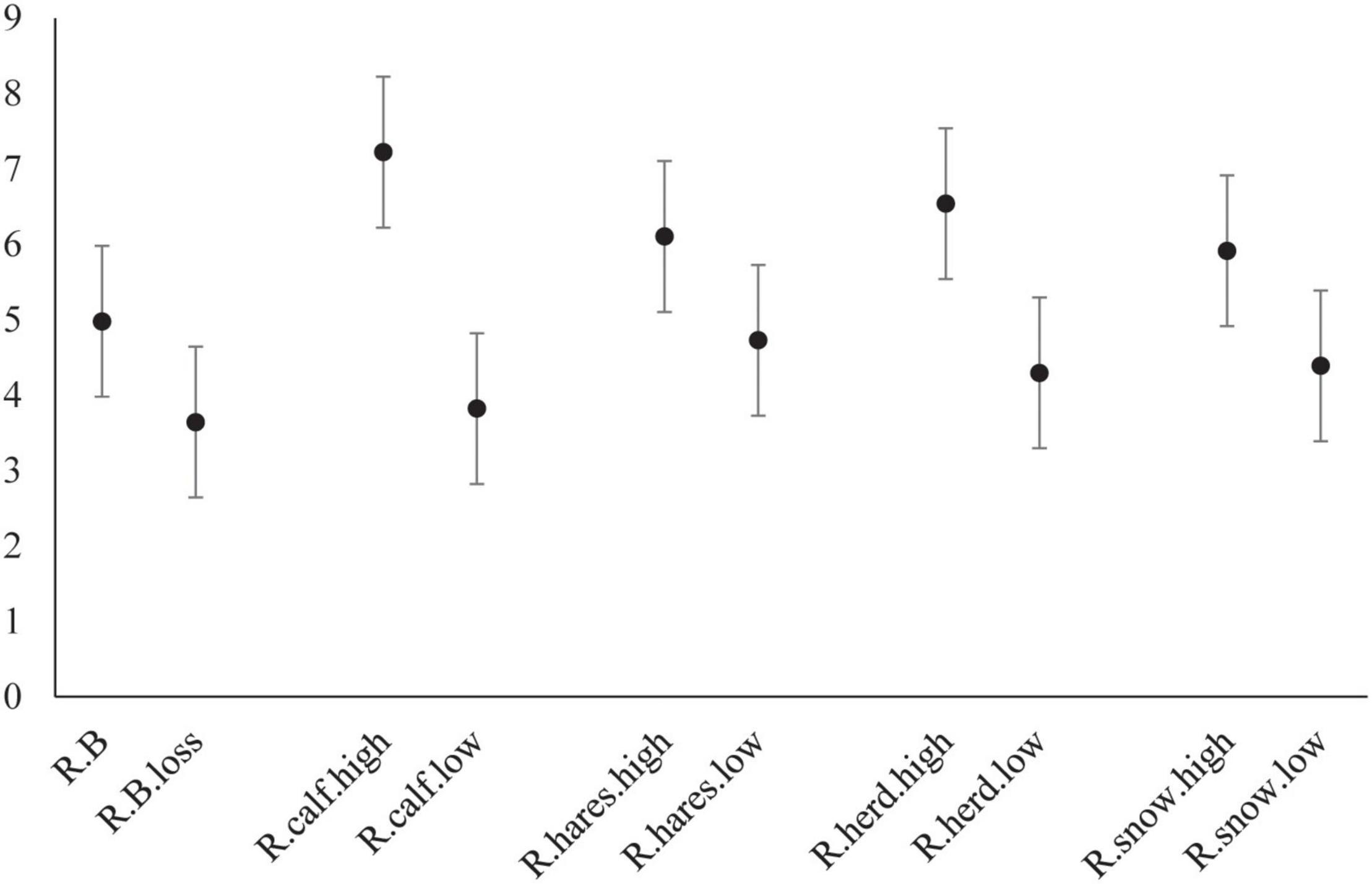
Figure 7. Comparison of covariate values on estimated average number of individuals added to each wolf pack in Denali National Park and Preserve, Alaska, United States. Conditions included are: no breeder lost (R.B), loss of a breeder (R.B.loss), high caribou calf:cow ratio in the preceding year (R.calf.high), low caribou calf:cow ration in the preceding year (R.calf.low), high hare index in the preceding year (R.hares.high), low hare index in the preceding year (R.hares.low), high caribou herd size in the preceding year (R.herd.high), low caribou herd size in the preceding year (R.herd.low), high snowfall in the previous winter (R.snow.high), and low snowfall in the previous winter (R.snow.low). Values used represent the range of values observed during our study. Error bars represent 95% credible intervals. For each scenario all other covariates are held at mean values.
Spring population estimates derived from the model ranged from 58 wolves at the start of the study in 1986 to a high of 198 wolves in 1990 following a dramatic increase in caribou herd numbers (Figures 3A, 8). There was an interaction between mean pack size and the number of packs observed. In years with high prey availability, mean pack size increased, as seen following during a period of high snowfall in the late 1980s and early 1990s and again during the peak hare index around 2010 (Figure 3C). From the early 1990s to 2000s, the number of packs was high and variable, with relatively low variability in mean pack size (Figure 3C and Table 2).
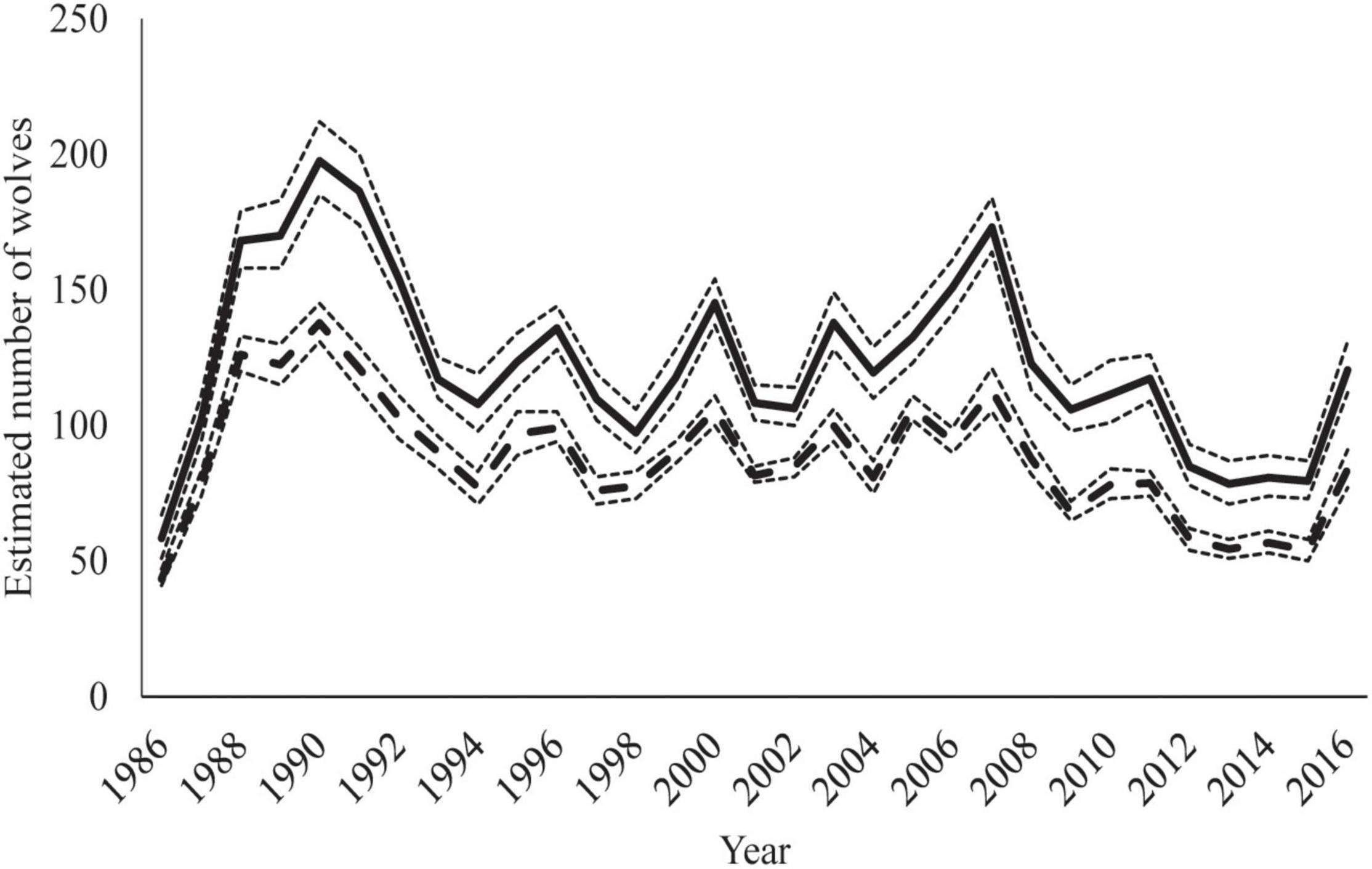
Figure 8. Estimated number of wolves in study area population in Denali National Park and Preserve, Alaska, United States derived from model for spring (solid line) and fall (dashed line). Dotted lines around estimates indicate 95% credible intervals.
Discussion
We found a strong influence of climatic conditions in the form of cumulative snowfall on wolf survival, natality, and population size. Wolves experienced greater apparent survival and natality and population size increased during periods with more cumulative snow fall. We also found that survival, dispersal, and population size all declined as the caribou population grew, in direct contrast to the expectation that increased prey biomass should result in higher wolf population size (reviewed in Fuller et al., 2003). In addition, our findings provide evidence that non-ungulate prey can impact wolf population dynamics, subsidizing ungulate resources when abundant. Together these results clarify the nature of bottom-up effects in wolf -ungulate systems, indicating that the influence is not simply a density-dependent relationship between large ungulate prey and predator populations. Our work provides insights into the role of weather and secondary prey resources in driving wolf populations, and our results offer important context for wolf management and conservation, particularly in Alaska where wolf control is implemented widely.
One of the most important questions in the field of ecology, particularly wolf ecology, is how populations of predators affect those of their prey. Our findings are consistent with those from other components of the Denali ecosystem (Schmidt et al., 2018a,b) and previous work (Murie, 1944; Mech et al., 1998) suggesting that bottom-up forces play a large role in predator-prey dynamics in this system. While bottom-up drivers in food webs are linked to primary productivity, whereby increased primary productivity leads to increases in herbivores which leads to increases in predators (Paine, 1980), a more intricate food web paradigm may better explain the wolf-prey dynamics (Eisenberg et al., 2013).
We found that prey vulnerability affected wolf demographics in ways that were distinct from effects of prey abundance. Although measuring vulnerable prey biomass can be challenging (Fuller et al., 2003), in several systems key factors influencing vulnerability are so dominant that they provide relatively reliable indices of prey vulnerability. For example, in Isle Royale the number of moose 10 years and older has been shown to be a strong predictor of wolf population trends (Peterson, 1977; Peterson and Page, 1988) and evidence for bottom-up control (Sand et al., 2012). Previous work determined prey availability and corresponding trends in wolf populations in Denali were driven largely by snow depth (Mech et al., 1998), and indeed we found that greater snowfall in the preceding year increased survival and natality in the Denali wolf population (Figures 5, 7). Additionally, we saw that increasing vulnerable prey as seen through higher calf ratios increased wolf survival and natality. This evidence supports the effect of prey base, moderated by factors that influence vulnerability, are strong drivers of the wolf demographic rates. Because ungulate prey face similar nutritional and mobility stressors with increased snow depth, snow depth is likely to be broadly applicable as an index of vulnerability across prey species in regions with seasonal snow cover.
While winter conditions can have immediate effects on prey vulnerability, our findings indicate that cumulate impacts over time may also be important. Prenatal nutrition can impact fetuses during severe winters and persist across additional generations (Zamenhof and Van Marthens, 1978; Mech et al., 1991; Messier, 1995), and consecutive winters with deep snowpack pose a cumulative effect on prey vulnerability (Mech et al., 1987; McRoberts et al., 1995; Messier, 1995). In general, weather conditions in the Denali study are have been mild since 1992, with several consecutive winters with low cumulative snowfall and very low number of days with snow on ground over 53 cm, and a consistent decrease in snow depth over time (Table 1). In conjunction, plant biomass (as measured by running NDVI) increased almost linearly through 2008 (Schmidt et al., 2018b). Recent increases in caribou and moose numbers (Figure 3) and the effect of improved nutrition coupled with decreased energetic costs in winter may be evidence that ungulates are in good condition, and thus harder for wolves to catch. Thus, reductions in ungulate vulnerability mediated through changing environmental conditions can release prey from low density, even in the presence of unregulated wolf numbers, as also seen in a weather-related increase in the Forty-Mile Caribou Herd prior to implementation of wolf control actions (Boertje et al., 2017).
We found that when conditions allow for the increase in a resident caribou herd, as seen in the latter part of our study, wolf natality increased, yet contrary to expectations wolf population size declined (Figures 3A,B). Intrinsic social characteristics and territoriality may moderate wolf population response to growing prey populations (Fuller et al., 2003), and we speculate that territoriality in our study area may play a restricting role on population growth. The decline in wolf population size is attributed to fewer packs in recent years, rather than a decrease in mean pack size (Figure 2C and Table 2). As prey becomes harder to catch, wolf packs respond by increasing search distance for vulnerable prey, requiring increased territory sizes (Johnson et al., 2013). As growing ungulate populations are evidence of reduced ungulate vulnerability, this can result in increased wolf territory sizes and fewer packs within same area. The upper limit on the number of territories that can be supported effectively caps breeding by a cooperatively breeding social carnivore, limiting the influence of increased natality on population growth (Fuller et al., 2003). This limitation may be evidence of how territoriality can be a self-regulating mechanism for a population (Wallach et al., 2015).
Mean pack size has been proposed as an alternate to wolf density measures for tracking changes within a study area as density estimates alone are problematic (Schmidt et al., 2017). However, mean pack size and pack territory size or the number of territories that a given area can support must also be considered. As wolf natality and apparent survival increase in response to prey vulnerability, we expect mean pack sizes to increase, as seen following the increase in caribou vulnerability of the late 1980s and early 1990s and during the high hare peak around 2010 (Table 2). While mean pack size increases can lead to increases in population during these periods of increased prey vulnerability, during periods without dramatic shifts in prey vulnerability, the number of packs in the study area drives more interannual variation in the population (Figure 2C). The interaction between group size and number of groups, as mediated by food availability and environmental conditions is likely to be important for determining density for many social, territorial species.
The concurrent long-term studies on caribou and wolves in the Denali ecosystem allowed for a unique long-term analysis of predator-prey dynamics. The effects of other large ungulates and carnivores in this system were less clear due to lack of consistent data (see section “Materials and Methods”). Despite this limitation, wolf natality responded strongly to caribou numbers but was insensitive to an apparent moose increase in the mid-1990s (Figure 6). Although DCH abundance measures may reflect a relatively coarse metric of ungulate biomass (caribou comprised 39% of kills from 1986 to 1993, Adams and Roffler, 2010), it does provide a valuable index of change in the abundance of a key ungulate prey species for wolves in this system.
We found evidence for the influence of secondary prey on metrics of wolf demographics as hare abundance prior to whelping had a strong effect on wolf natality rates. Previous work posited that high pup survival rate estimated in Denali may have be in part due to the presence of small prey such as ground squirrels, marmots, beavers, and hares during summer months (Mech et al., 1998; Haber and Holleman, 2013). Biologist Adoph Murie documented the use of hares by wolves in the 1940’s and suggested that hares may play a significant role in subsidizing wolves (Murie, 1944). Nutritional condition of females at breeding and pregnancy determines litter size (Sadleir, 1969) and this principle applies to wolves (Boertje and Stephenson, 1992). Thus, hare abundance during this time period may result in improved prenatal condition of breeding females and increased litter size and early survival of pups (Sadleir, 1969; Boertje and Stephenson, 1992).
The influence of a secondary prey source such as hares on wolf natality further supports our findings implying that wolf productivity is otherwise limited by prey availability. The influence of primary productivity on subsequent hare abundance further supports the prevalence of bottom up processes (Schmidt et al., 2017, 2018a,b). Interestingly, apparent wolf survival decreased with increased hare numbers. It is possible that presence of hares may increase time individuals spend traveling alone during summer months as they take advantage of abundant small prey (Benson and Patterson, 2015) and time spent away from packs may increase mortality risk or dispersal. Alternatively, increased natality may put more pressure for provisioning on packs during the winter months leading to decreased survival or increased dispersal (Mech et al., 1998).
Comparing vital rates and predator-prey associations from our study in Denali to those in other areas with different management regimes (e.g., predator control) and prey population characteristics (e.g., migratory prey) can allow managers to make more informed and effective decisions regarding the conservation and management of both wolves and their ungulate prey in a variety of systems. Overall, annual apparent survival rates in Denali were relatively consistent (Figure 5). We found that apparent survival of breeding wolves was lower in Denali (0.68) than for breeding wolves in Yukon-Charley (∼0.8) or all wolves in Brooks Range (∼0.8), whereas survival for non-breeding wolves in Denali (0.63) was higher than that in Yukon-Charley (Adams et al., 2008; Schmidt et al., 2017). Models based on Yukon-Charley data suggested that apparent survival should be approximately 0.9 for breeding wolves and 0.6 for non-breeding wolves in interior Alaska (Schmidt et al., 2017). The finding that survival rates in the lightly harvested population of wolves in Denali was lower than expected for breeding wolves is intriguing. Intraspecific strife is the leading cause of natural mortality for wolves in Denali (Mech et al., 1998, this paper) and breeders may be at greater risk for mortality in these conflicts (Cassidy, 2013; Cubaynes et al., 2014; Cassidy et al., 2017) although more recent work suggests that breeders are associated with increased risk of attack but not necessarily mortality (K. Cassidy, pers. comm.). Prey base may also be implicated in the reduced survival of wolves in Denali, because when caribou are less vulnerable and wolves switch to sheep and moose (Murdoch, 1969; Mech et al., 1998), they may have greater risk of injury or mortality in hunting (Mech et al., 2015), especially as breeders take a leadership role in hunting (Mech, 2000; Peterson et al., 2002; MacNulty et al., 2011).
We found an inverse relationship between wolf natality and apparent survival. Because apparent survival is a composite of mortality and dispersal from the population, it was difficult to clearly determine if mortality or dispersal was increasing in response to increased natality. Interpack competition may act to increase both sources of loss from the population (Messier, 1985; Ballard et al., 1987; Peterson and Page, 1988; Gese and Mech, 1991; Boyd and Pletscher, 1999). However, dispersal and survival rates from the known fate collared sample indicates that dispersal, rather than survival was inversely correlated with natality rates. One hypothesis for this is that large litters increase interpack competition by putting more pressure on other pack members to leave or travel more, leading to higher dispersal and reducing apparent survival (Mech et al., 1998; Adams et al., 2008; Smith et al., 2010). However, the timing of dispersal in our study coincided with the pre-breeding and breeding season, showing similarity with studies in the Brooks Range and Yukon-Charley (Adams et al., 2008; Schmidt et al., 2017) and suggesting that pressures due to breeding may pre-dominate as precursors to dispersal.
Our results contribute to a growing body of evidence that environmental conditions may ultimately determine prey vulnerability and predator dynamics. Leveraging data from two concurrent, long-term studies allowed us to view predator-prey dynamics over a time scale commensurate with a changing climate. Global climate change is occurring more rapidly at northern latitudes (Intergovernmental Panel on Climate Change [IPCC] et al., 2013), underscoring the importance of understanding the mediating role environmental conditions play in a predator-prey-climate system.
Data Availability Statement
The datasets presented in this study can be found in online repositories. The names of the repository/repositories and accession number(s) can be found below: https://irma.nps.gov/DataStore/Reference/Edit/2288285 and https://doi.org/10.6084/m9.figshare.17054369.
Author Contributions
BB conducted the data collection from 2010 to 2021, prepared the data, conducted the analyses, and wrote the manuscript. DS oversaw the biological program research and provided support and input on the wolf research project and this manuscript.
Funding
This study was provided by the National Park Service, U.S. Geological Survey, and the National Park Service Inventory and Monitoring Program.
Conflict of Interest
The authors declare that the research was conducted in the absence of any commercial or financial relationships that could be construed as a potential conflict of interest.
Publisher’s Note
All claims expressed in this article are solely those of the authors and do not necessarily represent those of their affiliated organizations, or those of the publisher, the editors and the reviewers. Any product that may be evaluated in this article, or claim that may be made by its manufacturer, is not guaranteed or endorsed by the publisher.
Acknowledgments
Joshua H. Schmidt conducted the statistical analysis for the integrated wolf population model and provided considerable assistance with the manuscript. Layne Adams collected and curated the data on the Denali Caribou Herd from 1986 to 2016 and on the Denali wolves for 1986–2003. John Burch conducted wolf captures in Denali from 1986 to 2003. Tom Meier oversaw the Denali wolf research program and was the Biological Program manager from 2004 to his untimely death in 2012. This work is dedicated to Tom and his legacy of leadership and work in Denali. Stephan Arthur oversaw the Denali wolf research from 2012 to 2016. Carol McIntyre collected and provided the hare index data for analysis. Maggie MacCluskie, Kaija Klauder, and Laura Prugh all provided valuable insight, input, and editing that lead to significant improvement in the manuscript.
Supplementary Material
The Supplementary Material for this article can be found online at: https://www.frontiersin.org/articles/10.3389/fevo.2022.791161/full#supplementary-material
References
Adams, L. G. (2017). Dynamics Of The Denali Caribou Herd, Denali National Park, Alaska: Progress Report (October 2015-September 2016). Alaska, USA: National Park Service.
Adams, L. G., Farley, S. D., Stricker, C. A., Demma, D. J., Roffler, G. H., Miller, D. C., et al. (2010). Are inland wolf - ungulate systems influenced by marine subsidies of Pacific salmon? Ecol. Appl. 20, 251–262.
Adams, L. G., and Meier, T. J. (2018). Monitoring Protocol for the Denali Caribou Herd, Denali National Park and Preserve, Alaska: Central Alaska Inventory and Monitoring Program. Natural Resource Report NPS/CAKN/NRR-2018/1754. Fort Collins, CO: National Park Service.
Adams, L. G., and Roffler, G. (2009). Dynamics Of The Denali Caribou Herd, Denali National Park, Alaska: Progress Report. Alaska, USA: National Park Service.
Adams, L. G., and Roffler, G. H. (2010). Dynamics of the Denali Caribou Herd, Denali National Park, Alaska: Progress Report (October 2008 - September 2009)-Excerpt Regarding Seasonal Distribution of Caribou from this Progress Report. Anchorage: USGS Alaska Science Center, 1–12. doi: 10.1017/CBO9781107415324.004
Adams, L. G., Singer, F. J., and Dale, B. W. (1995). Caribou Calf Mortality in Denali National Park, Alaska. J. Wildl. Manag. 59, 584–594.
Adams, L. G., Stephenson, R. O., Dale, B. W., Ahgook, R. T., and Demma, D. J. (2008). Population Dynamics and Harvest Characteristics of Wolves in the Central Brooks Range, Alaska. Wildl. Monogr. 170, 1–25. doi: 10.2193/2008-012
Allen, B. L., Allen, L. R., Andrén, H., Ballard, G., Boitani, L., Engeman, R. M., et al. (2017). Can we save large carnivores without losing large carnivore science? Food Webs. 12, 64–75. doi: 10.7717/peerj.10738
Ballard, W. B., Whitman, J. S., and Gardner, C. L. (1987). Ecology of an exploited wolf population in south-central Alaska. Wildl. Monogr. 51, 1–54.
Belant, J., and Stahlnecker, K. E. (1997). 1997 Aerial Moose Survey, Denali National Park And Preserve. Denali Park, Ak: National Park Service.
Belant, J. L., Wilson, K., and Owen, P. A. (2000). 1999 Aerial Moose Survey, Denali National Park and Preserve. Denali Park, Ak: National Park Service.
Benson, J. F., and Patterson, B. R. (2015). Spatial overlap, proximity, and habitat use of individual wolves within the same packs. Wildl. Soc. Bull. 39, 31–40. doi: 10.1002/wsb.506
Berger, J., Stacey, P. B., Bellis, L., and Johnson, M. P. (2001). A mammalian predator-prey imbalance: grizzly bear and wolf extinction affect aviain neotropical migrants. Ecol. Appl. 11, 947–960. doi: 10.2307/3061004
Boertje, R. D., Gardner, C. L., Ellis, M. M., Bentzen, T. W., and Gross, J. A. (2017). Demography of an increasing caribou herd with restricted wolf control. J. Wildl. Manag. 81, 429–448. doi: 10.1002/jwmg.21209
Boertje, R. D., Keech, M. A., and Paragi, T. F. (2010). Science and Values Influencing Predator Control for Alaska Moose Management. J. Wildl. Manag. 74, 917–928. doi: 10.1093/conphys/coaa130
Boertje, R. D., and Stephenson, R. O. (1992). Effects of ungulate availability on wolf reproductive potential in Alaska. Canadian. Field Naturalist 70, 2441–2443.
Boertje, R. D., Valkenburg, P., and McNay, M. E. (1996). Increases in moose, caribou, and wolves following wolf control in Alaska. J. Wildl. Manag. 60, 474–489. doi: 10.2307/3802065
Boonstra, R., Krebs, C. J., Gilbert, B. S., and Schweiger, S. (2001). “Herbivores: voles and mice,” in Ecosystem Dynamics of The Boreal Forest, eds C. J. Krebs, S. Boutin, and R. Boonstra (New York, N Y: Oxford University Press), 215–239.
Borg, B. L., Brainerd, S. M., Meier, T. J., and Prugh, L. R. (2015). Impacts of breeder loss on social structure, reproduction and population growth in a social canid. J. Anim. Ecol. 84:414. doi: 10.1111/1365-2656.12256
Borg, B. L., and Burch, J. (2014). Vital signs monitoring of wolf (Canis lupus) distribution and abundance in Denali National Park and Preserve, Central Alaska Network: Biological year 2012 report. Natural Resource Data Series. Fort Collins (CO): National Park Service. Report NPS/CAKN/NRDS–2014/738. Denali Park, Ak: National Park Service.
Boyd, D. K., and Pletscher, D. H. (1999). Characteristics of dispersal in a colonizing wolf population in the central Rocky Mountains. J. Wildl. Manage 63, 1094–1108. doi: 10.2307/3802828
Cassidy, K. A. (2013). Group composition effects on inter-pack aggressive interactions of gray wolves in Yellowstone National Park. Behav. Ecol. 26, 1352–1360. doi: 10.1093/beheco/arv081
Cassidy, K. A., Mech, L. D., MacNulty, D. R., Stahler, D. R., and Smith, D. W. (2017). Sexually dimorphic aggression indicates male gray wolves specialize in pack defense against conspecific groups. Behav. Proces. 136, 64–72. doi: 10.1016/j.beproc.2017.01.011
Caughley, G. (1976). The elephant problem- an alternative hypothesis. African. J. Ecol. 14, 265–283. doi: 10.1111/j.1365-2028.1976.tb00242.x
Crête, M. (1999). The distribution of deer biomass in North America supports the hypothesis of exploitation ecosystems. Ecol. Lett. 2, 223–227. doi: 10.1046/j.1461-0248.1999.00076.x
Cubaynes, S., Macnulty, D. R., Stahler, D. R., Quimby, K. A., Smith, D. W., and Coulson, T. (2014). Density-dependent intraspecific aggression regulates survival in northern Yellowstone wolves (Canis lupus). J. Anim. Ecol. 83, 1344–1356. doi: 10.1111/1365-2656.12238
Dail, D., and Madsen, L. (2011). Models for estimating abundance from repeated counts of an open metapopulation. Biometrics 67, 577–587.
Eisenberg, C., Seager, S. T., and Hibbs, D. E. (2013). Wolf, elk, and aspen food web relationships:context and complexity. For. Ecol. Manag. 299, 70–80. doi: 10.1016/j.foreco.2013.01.014
Estes, J. A. (2005). “Carnivory and Trophic Connectivity in Kelp Forests. Pages 61–80,” in Large Carnivores and the Conservation of Biodiversity, eds J. Ray, K. H. Redford, R. Steneck, and J. Berger (Island Press: Washington, D.C. USA).
Fox, K. (1997). 1996 North-side Aerial Moose Survey. Denali National Park and Preserve. Denali Park, Ak: National Park Service.
Fuller, T. K. (1989). Population Dynamics of Wolves in North-Central Minnesota. Wildl. Monogr. 105, 3–41.
Fuller, T. K., Mech, L. D., and Cochrane, J. F. (2003). “Wolf population dynamics. Pages 161–191,” in Wolves: Behavior, Ecology and Conservation, eds L. D. Mech and L. Boitani (Chicago: University of Chicago Press).
Fuller, T. K., and Murray, D. L. (1998). Biological and logistical explanations of variation in wolf population density. Anim. Conserv. 1, 153–157. doi: 10.1111/j.1469-1795.1998.tb00023.x
Gable, T. D., Windels, S. K., Romanski, M. C., and Rosell, F. (2018). The forgotten prey of an iconic predator: a review of interactions between grey wolves Canis lupus and beavers Castor spp. Mamm. Rev. 48, 123–138. doi: 10.1111/mam.12118
Gasaway, W. C., Boertje, R. D., Grangaard, D. V., Kellyhouse, D. G., Stephenson, R. O., and Larsen, D. G. (1992). The role of predation in limiting moose at low densities in Alaska and Yukon and implications for conservation. Wildl. Monogr. 120, 1–59. doi: 10.1007/978-94-007-6173-5_11-2
Gasaway, W. C., DuBois, S. D., Reed, D. J., and Harbo, S. J. (1986). Estimating Moose Population Parameters from Aerial Surveys. Alaska: University of Alaska, 108.
Gasaway, W. C., Stephenson, R. O., Davis, J. L., Shepherd, P. E. K., and Burris, O. E. (1983). Interrelationships of Wolves, Prey, and Man in Interior Alaska. Wildl. Monogr 84, 1–50. doi: 10.1002/wmon.1026
Gese, E. M., and Mech, L. D. (1991). Dispersal of Wolves (Canis-Lupus) in Northeastern Minnesota, 1969-1989. Can. J. Zool. Can. Zool. 69, 2946–2955. doi: 10.1139/z91-415
Hairston, N. G., Smith, F. E., and Slobodkin, L. B. (1960). Community structure, population control and competition. Am. Nat. 94, 421–425. doi: 10.1086/282146
Houston, D. B. (1982). The northern Yellowstone elk: Ecology and Management. New York, NY: MacMillan.
Hugie, R. D. (1973). A Winter Study of Deer Mobility in West-Central Maine. Doctoral dissertation. Orono, ME: University of Maine.
Intergovernmental Panel on Climate Change [IPCC], Stocker, T. F., Qin, D., and Xia, Y. (2013). Climate Change 2013 The Physical Science Basis. Cambridge: Cambridge University Press.
Johnson, I., Brinkman, T., Lake, B., and Brown, C. (2017). Winter hunting behavior and habitat selection of wolves in a low-density prey system. Wildl. Biol. 2017. doi: 10.2981/wlb.00290
Keech, M. A., Lindberg, M. S., Boertje, R. D., Valkenburg, P., Taras, B. D., Boudreau, T. A., et al. (2011). Effects of predator treatments, individual traits, and environment on moose survival in Alaska. J. Wildl. Manage 75, 1361–1380. doi: 10.1002/jwmg.188
Klein, D. R. (1991). Limiting factors in caribou population ecology. Rangifer 11:30. doi: 10.7557/2.11.4.990
Krebs, C. J., Kielland, K., Bryant, J., Donoghue, M. O., Doyle, F., Mcintyre, C., et al. (2013). Synchrony in the snowshoe hare (Lepus americanus) cycle in northwestern North America, 1970-2012. Can. J. Zool. 572, 562–572. doi: 10.1139/cjz-2013-0012
MacNulty, D. R., Smith, D. W., Mech, L. D., Vucetich, J. A., and Packer, C. (2011). Nonlinear effects of group size on the success of wolves hunting elk. Behav. Ecol. 23, 75–82. doi: 10.1093/beheco/arr159
McIntyre, C. L., and Adams, L. G. (1999). Reproductive characteristics of migratory golden eagles in Denali National Park. Alaska. Condor 101, 115–123.
McIntyre, C. L., and Schmidt, J. H. (2012). Ecological and environmental correlates of territory occupancy and breeding performance of migratory golden eagles Aquila chrysaetos in interior Alaska. Ibis 154, 124–135.
McRoberts, R. E., Mech, L. D., and Peterson, R. O. (1995). The cumulative effect of consecutive winters’ snow depth on moose and deer populations: a defence. J. Anim. Ecol. 64, 131–135. doi: 10.2307/5834
Mech, L. D. (1966). The Wolves of Isle Royale, 7th Edn. Washington, DC: Fauna National Park Service.
Mech, L. D. (1986). Wolf Population in the Central Superior National Forest, 1967-1985. Madison: North Central Forest Experiment Station, 1–6.
Mech, L. D. (1995). A ten-year history of the demography and productivity of an arctic wolf pack. Arctic 48, 329–332.
Mech, L. D. (2004). Is climate change affecting wolf populations in the High Arctic? Clim. Chang 67, 87–93.
Mech, L. D., Adams, L. G., Meier, T. J., Burch, J. W., and Dale, B. W. (1998). The Wolves of Denali. Minneapolis, MN: University of Minnesota Press.
Mech, L. D., McRoberts, R. E., Peterson, R. O., and Page, R. E. (1987). Relationship of deer and moose populations to previous winters’ snow. J. Anim. Ecol. 56, 615–627.
Mech, L. D., Nelson, M. E., and McRoberts, R. E. (1991). Effects of Maternal and Grandmaternal Nutrition on Deer Mass and Vulnerability to Wolf Predation. J. Mammal. 72, 146–151. doi: 10.2307/1381989
Mech, L. D., and Peterson, R. O. (2006). “Wolf-Prey Relations,” in Wolves: Behavior, Ecology and Conservation, eds L. D. Mech and L. Boitani (Chicago: The University of Chicago Press), 131–160.
Mech, L. D., Smith, D. W., and Macnulty, D. R. (2015). Wolves on the Hunt the Behavior of Wolves Hunting Wild Prey. Chicago, IL: University of Chicago Press.
Mech, L. D., Smith, D. W., Murphy, K. M., and Macnulty, D. R. (2001). Winter severity and wolf predation on a formerly wolf-free elk herd. J. Wildife Manag. 65, 998–1003.
Meier, T. J. (1986). 1986 Aerial Moose Census- Denali National Park and Preserve. Alaska, USA: National Park Service.
Meier, T. J., Burch, J. W., Wilder, D., and Cook, M. (2009). Wolf monitoring protocol for Denali National Park and Preserve, Yukon-Charley Rivers National Preserve and Wrangell-St. Elias National Park and Preserve, Alaska. Fort Collins, CO: National Park Service.
Meier, T. J., Keay, J. A., Van Horn, J., and Burch, J. W. (1991). 1991 Aerial Moose Survey, Denali National Park and Preserve. Alaska, USA: National Park Service.
Meier, T. J., and Owen, P. A. (2011). 2011 Aerial Moose Survey. Anchorage, AK: Denali Natioal Park and Preserve.
Messier, F. (1985). Solitary living and extra-territorial movements of wolves in relation to social status and prey abundance. Can. J. Zool. 63, 239–245.
Messier, F. (1995). Is there evidence for a cumulative effect of snow on moose and deer populations. J. Anim. Ecol. 64, 136–140. doi: 10.2307/5835
Miller, B., Dugelby, B., Foreman, D., Martinez del Rio, C., Noss, R., Phillips, M., et al. (2001). The Importance of Large Carnivores to Healthy Ecosystems. Endanger. Species Updat. 18, 202–210.
Murdoch, W. W. (1969). Switching in generalist predators: experiments on prey specificity and stability of prey populations. Ecol. Monogr. 39, 335–354. doi: 10.2307/1942352
Nelson, M. P., Vucetich, J. A., Peterson, R. O., and Vucetich, L. M. (2011). The Isle Royale wolf-moose project (1958-present) and the wonder of long-term ecological research. Endeavour 35, 30–38.
Newsome, T. M., Boitani, L., Chapron, G., Ciucci, P., Dickman, C. R., Dellinger, J. A., et al. (2016). Food habits of the world’s grey wolves. Mamm. Rev. 46, 255–269. doi: 10.1111/mam.12067
NOAA Regional Climate Centers (2021). What is xmACIS. Available online at: http://xmacis.rcc-acis.org/ (accessed December 27, 2017).
Oksanen, L., and Oksanen, T. (2000). The Logic and Realism of the Hypothesis of Exploitation Ecosystems. Am. Nat. 155, 703–723. doi: 10.1086/303354
Owen, P. A., and Meier, T. J. (2009). 2008 Aerial Moose Survey, Denali National Park and Preserve. Denali Park, AK: National Park Service.
Paine, R. T. (1980). Food Webs:linkage, Interaction Strength and Community Infrastructure. J. Anim. Ecol. 47, 667–685. doi: 10.2307/4220
Peterson, R. O. (1977). Wolf ecology and prey relationships on Isle Royale. Missouri: Park Service Scientific.
Peterson, R. O., and Allen, D. L. (1974). Snow conditions as a parameter in moose-wolf relationships. Nat. Can. 101, 481–492.
Peterson, R. O., and Ciucci, P. (2006). “The Wolf as a Carnivore,” in Wolves: Behavior, Ecology and Conservation, eds L. D. Mech and L. Boitani (Chicago: The University of Chicago Press), 104–130.
Peterson, R. O., Jacobs, A. K., Drummer, T. D., Mech, L. D., and Smith, D. W. (2002). Leadership behavior in relation to dominance and reproductive status in gray wolves, Canis lupus. Can. J. Zool. 1412, 1405–1412. doi: 10.1139/Z02-124
Peterson, R. O., and Page, R. E. (1988). The rise and fall of the Isle Royle wolves. J. Mammal. 69, 89–99. doi: 10.2307/1381751
Putera, J., and Keay, J. (1998). Effect of Vehicle Traffic on Dall Sheep Migration in Denali National Park. Denali Park, AK: National Park Service.
Ripple, W. J., and Beschta, R. L. (2004). Wolves and the Ecology of Fear:can Predation Risk Structure Ecosystems. Bioscience 54, 755–766. doi: 10.1007/s00442-020-04816-4
Ripple, W. J., and Beschta, R. L. (2012). Trophic cascades in Yellowstone:the first 15 years after wolf reintroduction. Biol. Conserv. 145, 205–213. doi: 10.1016/j.biocon.2011.11.005
Ripple, W. J., Chapron, G., Lopez-Bao, J. V., Durant, S. M., Macdonald, D. W., Lindsey, P. A., et al. (2016). Saving the World’s Terrestrial Megafauna. Bioscience 66, 807–812. doi: 10.1093/biosci/biw092
Ripple, W. J., Estes, J. A., Beschta, R. L., Wilmers, C. C., Ritchie, E. G., Hebblewhite, M., et al. (2014). Status and ecological effects of the world’s largest carnivores. Science 343, 151–162. doi: 10.1126/science.1241484
Royle, J. A., and Dorazio, R. M. (2008). Hierarchical Modeling and Inference in Ecology: the Analyses of Data from Populations, Metapopulations and Communities. Amsterdam: Elsevier.
Sadleir, R. M. F. S. (1969). The Ecology of Reproduction in Wild and Domestic Animals. Breed. Environ. 166, 592–593.
Sand, H., Vucetich, J. A., Zimmermann, B., Wabakken, P., Wikenros, C., Pedersen, H. C., et al. (2012). Assessing the influence of prey-predator ratio, prey age structure and packs size on wolf kill rates. Oikos 121, 1454–1463. doi: 10.1111/j.1600-0706.2012.20082.x
Schmidt, J. H., Burch, J. W., and MacCluskie, M. C. (2017). Effects of control on the dynamics of an adjacent protected wolf population in interior Alaska. Wildl. Monogr. 198, 1–30.
Schmidt, J. H., Johnson, D. S., Lindberg, M. S., and Adams, L. G. (2015). Estimating demographic parameters using a combination of known-fate and open N-mixture models. Ecology 96, 2583–2589. doi: 10.1890/15-0385.1
Schmidt, J. H., Rextad, E. A., Roland, C. A., Mcintyre, C. L., MacCluskie, M. C., and Flamme, M. J. (2018b). Weather-driven change in primary productivity explains variation in the amplitude of two herbivore population cycles in a boreal system. Oecologia 186, 435–446. doi: 10.1007/s00442-017-4004-3
Schmidt, J. H., McIntyre, C. L., Roland, C. A., MacCluskie, M. C., and Flamme, M. J. (2018a). Bottom-up processes drive reproductive success in an apex predator. Ecol. Evol. 8, 1833–1841. doi: 10.1002/ece3.3800
Schmidt, J. H., and Rattenbury, K. L. (2013). Reducing effort while improving inference: Estimating Dall’s sheep abundance and composition in small areas. J. Wildl. Manag. 77, 1048–1058. doi: 10.1002/jwmg.557
Schmidt, J. H., Walker, J. A., Lindberg, M. S., Johnson, D. S., and Stephens, S. E. (2010). A general Bayesian hierarchical model for estimating survival of nests and young. Auk 127, 379–386. doi: 10.1525/auk.2009.09015
Schmitz, O. J. (2006). Predators have large effects on ecosystem properties by changing plant diversity, not plant biomass. Ecology 87, 1432–1437. doi: 10.1890/0012-9658
Sinclair, A. R. E. (1977). The African Buffalo: A Study of Resource Limitation of Population. Chicago: University of Chicago Press.
Smith, D. W., and Bangs, E. E. (2009). “Reintroduction of wolves to Yellowstone National Park: history, values, and ecosystem restoration,” in Reintroduction of Top-Order Predators, eds M. W. Hayward and M. J. Somers (Hoboken, N J: Wiley-Blackwell), 92–125.
Smith, D. W., Stahler, D. R., Macnulty, D. R., Raymond, R., Cassidy, K., and Albers, E. (2010). Wolf Pack SDtability Promotes Dispersal and Recovery Area Connectivity. In SCB 24th Annual Meeting. Hoboken, N J: Wiley-Blackwell.
Sousanes, P. (2006). Climate Monitoring Protocol for Central Alaska Network. Alaska: National Park Service.
Sousanes, P. J., and Hill, K. (2017). Annual Climate Summary 2014O Central Alaska Network. Colorado: Fort Collins.
Telfer, E. S. (1978). Cervid distribution, browse and snow cover in Alberta. J. Wildl. Manage. 42, 352–361.
Terborgh, J. W. (1988). The big things that run the world- a sequel to E. O. Wilson. Conserv. Biol. 2, 402–403. doi: 10.1111/j.1523-1739.1988.tb00207.x
Terborgh, J. W., Feeley, K., Silman, M., Nunez, P., and Balukjian, B. (2006). Vegetation dynamics of predator-free land-bridge islands. J. Ecol. 94, 253–263. doi: 10.1111/j.1365-2745.2006.01106.x
Titus, K. (2007). “Intensive Management of Wolves and Ungulates in Alaska,” in Transactions of the 72nd North American Wildlife and Natural Resources Conference, (Hoboken, NJ: Wiley-Blackwell), 366–377.
Valkenburg, P., Dale, B. W., Davis, J. L., Ellis, M. M., Boertje, R., Keech, M. A., et al. (2016). Monitoring Caribou Herds in Alaska, 1970–2008, With Focus on the Delta Caribou Herd, 1979–2007. Fairbanks, AK: Alaska Department of Fish and Game, Division of Wildlife Conservation.
Vucetich, J. A., Peterson, R. O., and Schaefer, C. C. (2002). The effect of prey and predator densities on wolf predation. Ecology 83, 3003–3013.
Vucetich, J. A., Smith, D. W., and Stahler, D. R. (2005). Influence of harvest, climate and wolf predation on Yellowstone elk, 1961-2004. Oikos 111, 259–270. doi: 10.1111/j.0030-1299.2005.14180.x
Wallach, A. D., Izhaki, I., Toms, J. D., Ripple, W. J., and Shanas, U. (2015). What is an apex predator? Oikos 124, 1453–1461. doi: 10.1111/oik.01977
Wallmo, O. C., and Gill, R. B. (1971). “Snow, winter distribution, and population dynamics of mule deer in the central Rocky Mountains,” in Proceedings of the Snow and Ice Symposium, (Ames, IA), 1–15.
Keywords: Alaska, Canis lupus, demography, natality, population dynamics, predator prey, survival, wolf
Citation: Borg BL and Schirokauer DW (2022) The Role of Weather and Long-Term Prey Dynamics as Drivers of Wolf Population Dynamics in a Multi-Prey System. Front. Ecol. Evol. 10:791161. doi: 10.3389/fevo.2022.791161
Received: 08 October 2021; Accepted: 10 January 2022;
Published: 04 March 2022.
Edited by:
Paul Robbins, University of Wisconsin–Madison, United StatesReviewed by:
Robby R. Marrotte, Trent University, CanadaTimothy Van Deelen, University of Wisconsin–Madison, United States
Copyright © 2022 Borg and Schirokauer. This is an open-access article distributed under the terms of the Creative Commons Attribution License (CC BY). The use, distribution or reproduction in other forums is permitted, provided the original author(s) and the copyright owner(s) are credited and that the original publication in this journal is cited, in accordance with accepted academic practice. No use, distribution or reproduction is permitted which does not comply with these terms.
*Correspondence: Bridget L. Borg, YnJpZGdldF9ib3JnQG5wcy5nb3Y=