- 1School of Life Sciences, Keele University, Keele, United Kingdom
- 2Department of Plant Protection, Faculty of Agriculture, Suez Canal University, Ismailia, Egypt
- 3International Centre of Insect Physiology and Ecology (icipe), Nairobi, Kenya
- 4Department of Entomology and Zoology, University of the Free State, Bloemfontein, South Africa
- 5Unit for Environmental Sciences and Management, North-West University, Potchefstroom, South Africa
Fall armyworm, Spodoptera frugiperda, is a serious invasive pest in Africa but “Push-Pull” companion cropping can substantially reduce infestation. Here, we elucidate the underpinning chemical ecology mechanisms. We hypothesized that companion crop volatiles repel herbivores (push) while attracting natural enemies (pull). Headspace volatiles collected from companion plants (Desmodium intortum, Desmodium uncinatum, Brachiaria Mulato II) were used in bioassays and electrophysiological recordings with S. frugiperda and parasitoid wasps. Insect populations, plant damage and herbivore parasitism were assessed in field plots. Coupled GC-electroantennogram (GC-EAG) recordings showed robust responses to certain aromatic and terpenoid volatile compounds. In wind tunnel bioassays, maize volatiles mixed with Desmodium volatiles were less attractive to moths than maize alone. In oviposition bioassays, S. frugiperda laid significantly fewer eggs on maize when Desmodium volatiles were present. Conversely, in an olfactometer bioassay, parasitoid wasps were attracted to the scent of both Desmodium spp. (intercrop) and the Brachiaria border crop. Our data provide evidence of the mechanisms underpinning reduced S. frugiperda infestation in the Push-Pull companion cropping system, i.e., volatiles from companion crops repel S. frugiperda while attracting its parasitoid natural enemies. These findings explain why Push-Pull field plots had fewer S. frugiperda larvae and lower crop damage than monocropped maize.
Introduction
Ecological management methods are required for management of the fall armyworm, Spodoptera frugiperda (J. E. Smith) (Lepidoptera: Noctuidae), a serious invasive pest currently spreading across the world from its native range in the Americas. It recently invaded and rapidly spread across large areas of Africa, where it now is a major threat to sustainable food production (Stokstad, 2017; Rwomushana et al., 2018; Kassie et al., 2020). Although S. frugiperda is highly polyphagous, it prefers to feed on maize, the main staple and cash crop for 300 million smallholder farmers in Africa (Day et al., 2017). Before arrival of S. frugiperda, “Push-Pull” companion cropping was developed to reduce damage by the cereal stemborers Chilo partellus (Swinhoe) and Buseola fusca (Fuller) in smallholder farms (Midega et al., 2015). Studies have shown that “Push-Pull” companion cropping substantially reduced S. frugiperda herbivory (Hailu et al., 2018; Midega et al., 2018), with an average 82.7% reduction in the latter study. Substantial yield increases were also found but the underpinning ecological mechanisms had not yet been determined. The current study sought to elucidate the mechanisms responsible for reductions in S. frugiperda infestation and damage. Better understanding of these mechanisms can aid in integrating these ecological methods with other sustainable S. frugiperda management options such as biological control and host plant resistance.
The original stemborer Push-Pull system involved intercropping cereal crops with repellent (“push”) forage legumes and planting attractive (“pull”) fodder grasses around the border of the cereal plots (Khan et al., 2000, 2010). Volatiles, such as (E)-4,8-dimethyl-1,3,7-non-atriene (DMNT), constitutively released from the intercrop repelled pest species (Khan et al., 2000), and attracted their natural enemies (Khan et al., 1997a). The original system had Melinis minutiflora P. Beauv. as the intercrop and Napier grass as the border crop (Khan et al., 1997b). The bioactive compounds were identified from M. minutiflora as (E)-β-ocimene, α-terpinolene, β-caryophyllene, humulene, and DMNT using coupled GC-electroantennography (GC-EAG) (Khan et al., 2000). Intercropping with forage legumes in the genus Desmodium spp. became more popular than M. minutiflora when it was discovered that they could suppress the devastating parasitic witchweed Striga hermonthica (Delile) Benth in addition to repelling insect pests (Khan et al., 2000, 2002). The desmodium species produced high amounts of terpenoids, particularly (E)-ocimene and DMNT, and it was shown that more parasitoids were attracted when maize was intercropped with desmodium maize than monocrop maize (Midega et al., 2009). A further development made Push-Pull companion crops “Climate Smart” by selecting more drought tolerant species that are more resilient to variable and reduced rainfall patterns associated with climate change (Midega et al., 2015). Climate smart Push-Pull has greenleaf desmodium, Desmodium intortum (Mill.) (Family: Fabaceae), as the intercrop and Brachiaria hybrid cultivar Mulato II (B. brizantha × B. decumbens × B. ruziziensis) (Family: Poaceae) as the border crop. Less is known about the volatiles from these companion crops because GC-EAG studies with stemborers were not reported for the climate smart companion crops. However, it was found that silverleaf desmodium [Desmodium uncinatum (Jacq.) DC.] produces (E)-β-ocimene and DMNT (Khan et al., 2000).
The current study tested two hypotheses relating to the volatiles released by Push-Pull companion crops: (1) That they deter female S. frugiperda from ovipositing on maize (the Desmodium intercrop was expected to be repellent and the Brachiaria border crop was expected to be attractive to moths). (2) That they attract and arrest foraging parasitoids (Figure 1). To test these hypotheses, behavioral responses of gravid S. frugiperda moths to volatiles from companion plants were tested in a wind-tunnel bioassay and their oviposition responses recorded in an oviposition bioassay. Similarly, behavioral responses of parasitoids to D. intortum, D. uncinatum, Brachiaria Mulato II, and/or maize volatiles were tested in olfactometer bioassays. Volatile samples were collected from companion plants and analyzed by GC-EAG to determine which compounds were detected by S. frugiperda and two different species of its larval endoparasitoids that are abundant in East Africa [Cotesia icipe Fernandez-Triana and Fiaboe (Hymenoptera: Braconidae) and Coccygidium luteum (Brullé) (Hymenoptera: Braconidae)] (Sisay et al., 2019). The volatile profiles of companion plants were analyzed by GC-MS and the bioactive compounds identified. Volatile samples were then tested in a wind-tunnel bioassay and oviposition responses of S. frugiperda moths were recorded in an oviposition bioassay with plants. The behavioral responses of the parasitoids to D. intortum (Mill.) Urb. (Fabaceae), D. uncinatum (Jacq.) DC. (Fabaceae), Brachiaria Mulato II (Poaceae), and/or maize volatiles were tested in olfactometer bioassays. Field experiments were conducted investigating S. frugiperda infestation, damage levels and parasitism rate under field conditions in Push-Pull maize and monocrop maize. This is the first lab to field study examining the chemical ecology of the “Push-Pull” ecological pest management technique against a devastating invasive S. frugiperda pest in Africa.
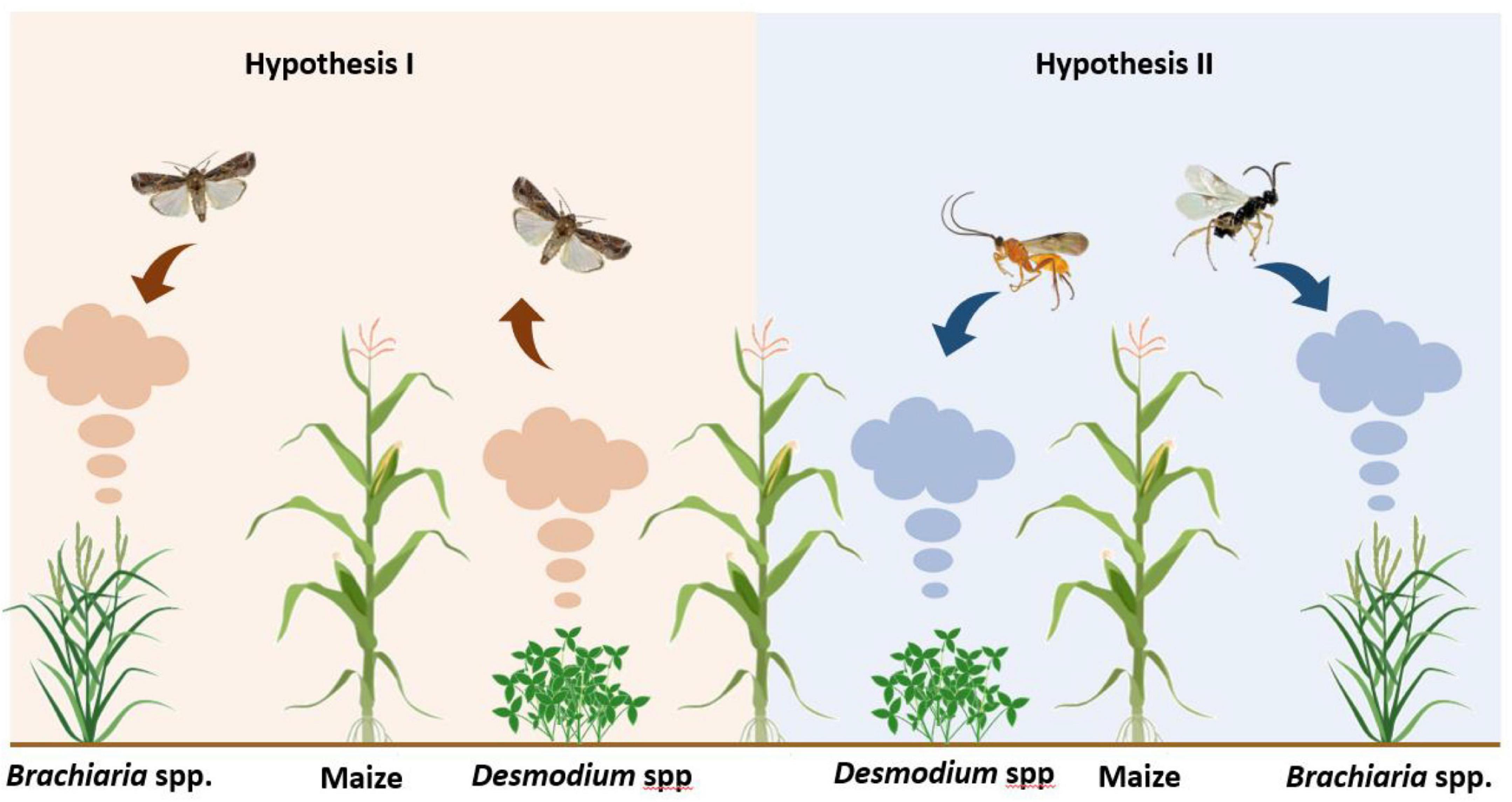
Figure 1. Schematic representation of a Push-Pull field and the research hypotheses: (1) Companion plant volatiles [Desmodium spp. intercrop (greenleaf desmodium, D. intortum or silverleaf desmodium, D. uncinatum) and border (Brachiaria Mulato II) plants] influence the behavior of female Spodoptera frugiperda and reduce oviposition on the main maize crop. (2) Companion plant volatiles attract parasitoid wasps to increase parasitism rate of the herbivore.
Materials and Methods
Experimental Plants and Insects
Greenleaf desmodium, Desmodium intortum, silverleaf desmodium D. uncinatum, Brachiaria cv. Mulato II, and maize, Zea mays, hybrid “SC Duma 43” (Seed Co, Kenya), plants were grown individually in pots filled with fertilized soil (3.5 g Diammonium phosphate-DAP, Elgon Kenya) in an insect-proof screen house at icipe-Thomas Odhiambo Campus (ITOC), Mbita Point (0°25′S, 34°12′E; c. 1,200 m above sea level), western Kenya. All plants were grown under natural conditions (25°C; 65% RH; 12L: 12D). Maize plants used in the experiments were 2–3 weeks old.
Spodoptera frugiperda, were reared on a standard diet for multiple lepidopteran species (Southland Products Inc., Lake Village, Arkansas, United States) under controlled conditions (25 ± 2°C, 70 ± 5% RH, 12L: 12D) using methods described by Mihm (1983). The culture was initiated with field-collected insects. Field-collected insects were added on a regular basis to refresh the mass-reared culture and maintain its original behavioral characteristics (Chambers, 1977). Larval endoparasitoids Cotesia icipe and Coccygidium luteum were reared on second instar larvae of S. frugiperda. Parasitoid cultures were maintained at 24 ± 3°C, 70 ± 5% RH, 12L: 12D.
Chemicals
Chemical standards used for GC quantification and comparison of GC retention times were (E)-2-hexenal, 1-octen-3-ol, 3-octanone, (Z)-3-hexenyl acetate, β-ocimene, (S)-linalool, (E,E)-allo-ocimene, methyl salicylate (MeSA), indole, α-copaene, β-caryophyllene, and (E)-β-farnesene. All standards were purchased from Sigma Aldrich (Gillingham, United Kingdom), except α-copaene which was purchased from Cayman Chemical Ltd. (Cambridge, United Kingdom). The homoterpenes (E)-4,8-dimethyl-1,3,7-non-atriene (DMNT) (>98%) and (E,E)-4,8,12-trimethyl-1,3,7,11-tridecatetraene (TMTT) (>98%) were synthesized from geraniol and (E,E)-farnesol, respectively, by oxidation to their corresponding aldehydes followed by Wittig methylenation (Leopold, 1990).
Volatile Collection
Volatile compounds from the different plant species were collected by headspace sampling (Agelopoulos et al., 1999; Tamiru et al., 2011) for use in subsequent bioassays and electrophysiological and chemical analyses. Volatiles were collected for 48 h. Leaves of plants were enclosed in polyethyleneterephthalate (PET) bags (volume 3.2 L) heated to 150°C before use and fitted with Swagelock inlet and outlet ports. Charcoal-filtered air was pumped (600 ml min–1) through the inlet port. Volatiles were collected on Porapak Q (50 mg, 50/80 mesh; Supelco, Bellefonte, PA, United States) filters inserted in the outlet port through which air was drawn at 400 ml min–1. Porapak Q filters were conditioned before use by washing with dichloromethane. All connections were made with polytetrafluoroethylene (PTFE) tubing (Alltech Associates, Lancashire, United Kingdom) with brass ferrules and fittings and sealed with PTFE tape. After entrainment, volatiles were eluted with 0.75 ml dichloromethane (EMSURE; Merck KGaA, Darmstadt, Germany) and stored in vials (Agilent Technologies, Stockport, United Kingdom; 2 ml with solid cap, 9 mm, w/PTFE/RS liner) at −80°C until required for chemical analysis and/or bioassay.
Wind Tunnel Bioassay
Behavioral responses of mated female S. frugiperda to plant volatiles were studied in a wind tunnel (25 ± 2°C; RH:70 ± 5%). The wind tunnel was made from glass (7 mm thickness) on an aluminum frame, with a design, including odor source tubes, as shown in Jaramillo et al. (2013) but with larger dimensions (122 × 32 × 32 cm). Plants were used to provide volatile stimuli in bioassays but were positioned outside the wind tunnel and not at the upwind end, in order to avoid any visual cues. Plant headspace volatiles were obtained by pumping charcoal filtered air into the headspace of 3-week-old experimental plants (4–6 leaves) covered with polyethyleneterephthalate (PET) bags (volume 3.2 L; heated to 150°C before use). Plants used were greenleaf desmodium, D. intortum (GL), silverleaf desmodium, D. uncinatum (SL), and Brachiaria cv. Mulato II (BR). These were tested alone in Series 1 and in combination with maize volatiles in Series 2. Naturally emitted volatiles from experimental plants were introduced at the upwind end of the wind tunnel, through an air inlet at 300 ml min–1. Two-day old mated female S. frugiperda moths were transferred from the insect mass rearing unit using a small Perspex cage covered with black cloth and kept in the bioassay room for 1 h before commencing the experiments for acclimatization.
Moths were carefully introduced individually through a side-panel at the downwind end of the wind tunnel, 100 cm away from the odor source tube at the upwind end. Distance flown upwind and any close approaches to the odor source were recorded during each 5 min bioassay period. It was not possible to record source contacts because the odor release point was not suitable for landing on. Bioassays were conducted at scotophase with reduced lighting provided by two bulbs (40 W, fitted with red filter) positioned at 30 cm above the wind-tunnel and angled to provide even coverage of the whole tunnel. The air velocity in the wind tunnel was 30 cm s–1. Air from the wind tunnel was continuously blown out of the bioassay room through rear air exhaust tube aided by a suction pump (50/60 Hz, 10A). Twenty replicates of individual female moths were used for each treatment and each female was tested in the wind tunnel once only.
The above wind tunnel bioassay procedure was also used to test moth responses to synthetic standards of plant volatiles that showed electrophysiological activity with female of S. frugiperda in GC-EAG recordings (described below). Blends of these synthetic plant volatiles were formulated at the same concentration and ratio as in natural headspace samples of the companion and maize plants. Authentic standards were obtained as liquid, weighed out and diluted in hexane to obtain the required concentration. Different volumes of higher concentration stock solution (1 mg/ml or 100 ng/μl) were mixed together to get the required final amounts. Details of concentrations and ratios of blend components are provided in Supplementary Table 1. In each replicate (n = 20) of the bioassay, 10 μl of test solution was released from a piece of filter paper.
Oviposition Bioassay
To assess oviposition behavior of S. frugiperda on plants, an oviposition bioassay was carried out under greenhouse conditions (25 ± 2°C, 65 ± 5% RH, 12L:12D) in a no-choice bioassay following modifications of methods described by Khan et al. (2007). Six different plant treatments were tested: (1) Brachiaria cv. Mulato II (BR), (2) Greenleaf Desmodium, D. intortum (GL), (3) Silverleaf Desmodium, D. uncinatum (SL), (4) Maize (cv. “SC Duma 43”) as a control. (5) Maize + GL. (6) Maize + SL. Given that Brachiaria plants are normally planted as a border crop in Push-Pull fields, no combined treatment of Brachiaria and maize was included in this assay. All plants were grown in pots (4 L) with standard ITOC agricultural soil, under the same conditions mentioned above. For the combined treatments (5 and 6), 50 Desmodium seeds were sown first, then maize (one seed per pot) was sown in the same pot 15 days later. This was because Desmodium grows more slowly than maize. Maize plants when used for the experiment were 12–15 days-old and had two fully developed leaves. In all cases, plants were grown and kept in separate screenhouse compartments before bioassay, to minimize unwanted induction of plant defense or “priming” effects by plant–plant volatile interactions between treatments (Sobhy et al., 2020).
Firstly, to investigate moth oviposition behavior under realistic conditions, with access to plants, oviposition cage experiments were carried out. Potted plants with the six different plant treatments (described above) were placed at the center of oviposition cages (80 × 80 × 100 cm) constructed of fine mesh attached to a wooden frame. Thereafter, five gravid S. frugiperda females (2–3 days old) were introduced into each cage before dusk and allowed to oviposit overnight under natural conditions of L12:D12. The numbers of S. frugiperda eggs were then counted on two oviposition sites: eggs deposited on plants and eggs deposited on the cage. For Maize + GL and Maize + SL treatments, eggs deposited on both maize and desmodium plants were counted. Ten replicates were conducted for each treatment. All cages were supplied with a 10-cm diameter wad of cotton wool moistened with water for moths to drink ad libitum.
Secondly, to investigate responses to volatiles, moth oviposition behavior was observed in compartments where access to plants was prevented but moths could still detect volatiles from the plants. This experiment was designed to test if eggs were laid further away from the odor source when exposed to companion plant volatiles. Compartments were made from polyethylene (PET) drink bottles with the bottom and the top cut off (20 cm height × 20 cm diameter), as described in Erb et al. (2011), and were inserted into the soil at the edge of each pot (Supplementary Figure 1). To ensure air circulation and prevent any direct contact between moths and plants, the upper openings of the bottles were covered with a sheet of mesh cloth which was held in place with a rubber band around the cylinder of the bottle. Another polyethylene (PET) cylinder (20 cm, height × 20 cm, diameter) was then placed on the top of the first cylinder with potted plants. Then five gravid S. frugiperda females (2–3 days old) were released for oviposition in the top cylinder. The upper opening of the oviposition unit was closed with a sheet of mesh cloth, identical to the bottom layer and held in place with a rubber band after releasing the moths (Supplementary Figure 1). The whole setup was covered with a dark cloth and moths were allowed to oviposit overnight (15 h). Subsequently, the number of S. frugiperda eggs was counted on two oviposition areas: Top = upper half sides of the bottle and the top mesh; and Bottom = lower half sides of the bottle as well as the bottom mesh, using a dissecting microscope. Fifteen replicates were conducted for each treatment.
Olfactometer Bioassay
Behavioral responses of larval endoparasitoid wasps, C. luteum and C. icipe to plant volatiles were tested in a Perspex four-arm olfactometer (Webster et al., 2010; Tamiru et al., 2011), in a controlled environment room (25 ± 2°C, 70 ± 5% RH) between 9:00 a.m. and 5:00 p.m. The olfactometer was placed on a table that was homogeneously illuminated by fluorescent tubes. Air was drawn through the four arms to exit through a hole at the center at 260 ml min–1. Headspace samples (10 μl aliquots) were applied, using a micropipette (Drummond “microcap,” Drummond Scientific Co., Broomall, PA, United States), to a piece of filter paper (4 × 25 mm; Whatman Filter Paper, Maidstone, United Kingdom). The solvent was allowed to evaporate for 30 s then paper strips were placed in an inlet port at the entrance to each olfactometer arm. Headspace samples were in dichloromethane. Prior to assay, mated female parasitoids (2–3 day-old) were provided contact experience with maize leaves damaged by S. frugiperda larvae (Turlings et al., 1989). Subsequently, experienced females were transferred individually into the central chamber of the olfactometer using a custom-made piece of glass tubing. Time spent in each olfactometer arm was recorded with Olfa software (F. Nazzi, Udine, Italy) for 12 min and after every 3 min the position of the olfactometer was rotated clockwise by 90° to eliminate bias. The experiments were replicated at least 12 times. A choice test was carried out to compare insect responses to plant volatile headspace samples vs. solvent control. One arm had the plant volatiles, whereas the other three control arms were treated similarly with the same volume of solvent (dichloromethane) on filter paper strips.
Olfactometer bioassays were also used to test insect responses to synthetic standards of plant volatiles that showed electrophysiological activity with C. luteum and C. icipe females in GC-EAG recordings (described below). Blends of these synthetic plant volatiles were formulated at the same concentration and ratio as in the headspace samples of the companion and maize plants. Details of concentrations and ratios of blend components are provided in Supplementary Table 1. Similar to the headspace samples, 10 μl of each synthetic formulated blend were applied using a micropipette to a piece of filter paper.
Gas Chromatography Coupled Electroantennogram Analysis
Analysis of plant volatiles, to determine which compounds could be detected by insects, was carried out using a Gas Chromatography Coupled Electroantennogram (GC-EAG) system, in which the effluent from a gas chromatograph (GC) column was simultaneously directed to the antennal preparation and the GC detector, as described by Wadhams (1990). Compounds were separated with a 7,820 GC (Agilent Technologies) fitted with flame ionization detector (FID) and a non-polar HP5-MS capillary column (30 mm × 0.25 mm × 0.25 μm film thickness; Agilent Technologies), which used hydrogen carrier gas at a constant flow rate of 1.2 ml/min. Manual injections of 1 μl were made in splitless mode (285°C) with the oven temperature maintained at 35°C for 5 min, and then programmed to increase at 10°C min–1 to 285°C, run time 45 min. The column effluent was split using a salinized glass Y-tube connector (Syntech, Kirchzarten, Germany). One arm of this connector was connected with fused silica tubing (50 cm × 0.32 mm i.d.) to the FID (250°C) and the other to an equal length of deactivated silica tubing passing through a heated (250°C) transfer line (Syntech) into a glass tube (4 mm i.d.) through which air passed (15 cm s–1) over the EAG preparation (Meza et al., 2020).
Electroantennogram recordings were made using an IDAC-2 acquisition controller (Syntech) connected to an antennal preparation as the second detector of the GC. Glass electrodes containing electrolyte solution (0.1 M potassium chloride) were attached to silver wires held in micromanipulators (Syntech). Female adult S. frugiperda, C. icipe, and C. luteum antennae (2–4 days old) were prepared for GC-EAG analysis by excising the head after being chilled in ice for 5 min, and the tips of antennae were removed to ensure a good contact. The reference electrode was either inserted into the back of the head or the antenna was excised and proximal segments of the antenna were mounted to the reference electrode, depending on the species of insect. Then, the circuit was completed by bringing the recording electrode into contact with the distal tip of one antenna (Meza et al., 2020). Both the FID and EAG signals were monitored simultaneously after passing through a high impedance amplifier and analyzed with Syntech software (v4.6.1). For each sample, at least 5 replicate antennal preparations were used for GC-EAG recordings (Sobhy et al., 2017). Thereafter, volatile compounds that stimulated consistent responses in at least three different antennae preparations were considered bioactive and replicable.
GC-MS Analysis
To identify bioactive compounds that elicited responses in GC-EAG recordings, volatile samples were analyzed using an Agilent 7820A GC coupled to a mass spectrometer (5977B single quad mass selective detector, Agilent Technologies). The GC was fitted with a non-polar HP5-MS capillary column (30 mm length × 0.25 mm inner diam. × 0.25 μm film thickness) coated with (5%-phenyl)-methylpolysiloxane (Agilent Technologies). Hydrogen was used as carrier gas at a constant flow rate of 1.2 ml min–1. Automated injections of 1 μl were made using a G4513A autosampler (Agilent Technologies) in splitless mode (285°C), with oven temperature maintained at 35°C for 5 min then programmed at 10°C min–1 to 285°C. Ionization was by electron impact at 70 eV, 250°C (source temperature). Identifications of volatiles were made by comparison of spectra with mass spectral databases (NIST, 2017) and retention index relative to retention times of n-alkanes, and co-chromatography with authentic compounds. Subsequently, authentic standards of bioactive compounds were co-injected with the natural samples of plant volatiles to confirm their tentative identification by peak enhancement (Pickett, 1990). The amounts (in nanograms) of identified volatiles present in collected headspace samples were quantified using a single point external standard quantification method using an authentic sample of 100 ng/μl (E,E)-allo-ocimene (Skelton et al., 2010). HP Chemstation software was used for data analyses.
Field Survey (in Farmers’ Fields)
To obtain data on current performance of Push-Pull companion cropping, extensive sampling of S. frugiperda infestation and damage levels, in farmers’ fields, was carried out in six different counties (Migori, Homa Bay, Siaya, Kisumu, Vihiga, and Bungoma) in the region around icipe-Mbita (0°40′ to 0°58S) in Western Kenya. Nine districts were sampled in the short rains season 2019 and 12 districts were sampled in the long rains season 2019. In each district, 30 smallholder farmers were randomly selected from the list of farmers practicing Push-Pull companion cropping with an adjacent maize monocrop, for data collection. In total 9 × 30 = 270 farms were sampled in the short rains 2019 and 12 × 30 = 360 farms were sampled in the long rains 2019.
The treatments were laid out in two plots at each farm sampled: Push-Pull companion cropping and monocropped maize for comparison of S. frugiperda infestation levels in the two farming systems. The plot sizes ranged from 20 m × 20 m to 30 × 30 m for each treatment. Maize was planted, according to a standard procedure, with 75 cm between rows and a 30 cm spacing between plants in a row, thinned to one plant per planting hole. In the Push-Pull plot, maize was intercropped with greenleaf desmodium (D. intortum; one row between each maize row) and Brachiaria cv. Mulato II was planted, as a border crop, around the field with a 50 cm spacing between plants. Phosphorus fertilizer (di-ammonium phosphate) was applied at planting at the rate of 60 kg ha–1 in each plot and top-dressed with nitrogen fertilizer (calcium ammonium nitrate) at 4 weeks after emergence at the rate of 60 kg ha–1 in both Push-Pull and maize monocrop plots. At each farm where data were collected, plot sizes were the same for Push-Pull and maize monocrop plots. There was a separation distance of at least 10 m between Push-Pull and maize monocrop plots.
Five sampling cells measuring 3 × 3 m were randomly marked in each plot. In each cell, 20 randomly selected plants were observed individually for presence/absence of S. frugiperda damage symptoms in leaves, whorl, tassel, and cobs. Thus, a total of 100 plants was inspected in each plot for S. frugiperda infestation. Data were collected 10–11 weeks after emergence of maize. Furthermore, percentage parasitism was assessed in larvae collected from Push-Pull and monocrop maize fields in the Homa Bay (0.6221°S, 34.3310°E), Migori (0.9366°S, 34.4198°E), Siaya (0.0917°S, 34.7680°E), and Kisumu (0.0617°S, 34.2422°E) districts. Spodoptera frugiperda larvae (2nd–4th instar) were collected from the whorl of maize plants grown in Push-Pull and maize monocrop plots. Larvae (60–122 per treatment) were then kept individually in small glass tubes (10 × 2.5 cm) and provided with artificial diet (Southland Products Inc.) and reared until pupation or parasitoid emergence. The latter was monitored daily and recorded.
Statistical Analyses
Prior to analysis, data were examined for normality with the Shapiro–Wilk test, and homogeneity of variances was tested by the Levene test before analysis. Wind tunnel bioassay data were analyzed using univariate analysis of variance (ANOVA) to investigate whether the flight behavior of S. frugiperda females differed when exposed to volatiles from companion plants and maize. For the first oviposition bioassay, two-way ANOVA was used to evaluate the effects of plant treatments [Brachiaria cv. Mulato II (BR); greenleaf Desmodium, D. intortum (GL); silverleaf Desmodium, D. uncinatum (SL); Maize (cv. “SC Duma 43”); Maize + GL; Maize + SL], oviposition sites (plants and cage) and their interaction of the number of S. frugiperda eggs laid in the oviposition cage experiment. Two-way ANOVA was also used to analyze the second oviposition bioassay (in compartments). The factors (plant treatments -described above) and oviposition sites (Top vs. Bottom) and their interactions were evaluated. In both wind tunnel and oviposition bioassays, comparisons among means were performed using the Holm-Sadek method. Oviposition data were Log transformed to meet assumptions of normality and homogeneity of variances. Parasitoid responses (attraction) in the olfactometer choice test were recorded using OLFA software (F. Nazzi, Udine, Italy), which provides a summary of time spent by the parasitic wasps in each of the four olfactometer arms. Bioassay data (time spent) were analyzed by one-way ANOVA using general linear model (GLM) after converting the data into proportions to address dependence of visiting time by parasitoids within the olfactometer fields, and log-ratio transformation to account for the compositional nature of the proportions (Aitchison, 1982; Tamiru et al., 2011). Comparisons of means were performed using the Student–Newman–Keuls (SNK) post hoc test (P < 0.05).
To visualize the overall differences in volatile profiles emitted from Desmodium spp., Brachiaria, and maize plants, a principal component analysis (PCA) was performed using the concentrations of the detected volatiles as dependent variables. Loading and score plots were derived after mean-centering and cube root transformation of volatile data. Average linkage hierarchical clustering based on Ward clustering algorithm of the Euclidean distance measure for the differentially emitted volatiles detected by ANOVA (P < 0.05) was used to construct a heatmap displaying the concentrations of different volatiles. Visualization together with hierarchical clustering of volatile data was done using the comprehensive online tool suite MetaboAnalyst 4.0 (Chong et al., 2018). A multivariate analysis of variance (MANOVA) was performed with plant species (i.e., Desmodium spp., Brachiaria, and maize) as a fixed factor and the concentrations of each volatile compound as dependent variables using SPSS software (IBM SPSS Statistics version 22.0, Armonk, NY, United States). Subsequently, univariate analyses of variances were performed to investigate whether the concentrations of the individual volatile compounds differed between treatments. Comparisons among means were performed using the Holm-Sidak method.
For the field survey, two-way ANOVA was used to compare the effects of sampling locations (different districts) and farming system (Push-Pull and monocrop maize) and their interaction on S. frugiperda infestation (larvae per 100 plants), damage level, and parasitism rate. All univariate analyses were performed with SIGMAPLOT 12.3 (Systat Software Inc., San Jose, CA, United States), except parasitoid responses which were analyzed using SAS version 9.2 (SAS Institute Inc, 2008).
Results
Fall Armyworm Flight Response to Plant Volatiles in Wind Tunnel
To test the hypothesis that companion plants have repellent activity, the flight behavior of mated female S. frugiperda moths was observed when exposed to volatiles from maize and companion plants (alone and in combination). In the first series of bioassays (Figure 2A), with volatiles from single plant species, maize volatiles were significantly more attractive than volatiles of any of the three companion crops tested, greenleaf desmodium, D. intortum (GL), silverleaf desmodium, D. uncinatum (SL), and Brachiaria cv. Mulato II (BR). Females of S. frugiperda flew significantly further up the upwind from the release point and flew closer to the odor source with maize than to volatiles of GL, SL, or BR [F(3, 76) = 7.916; P < 0.001].
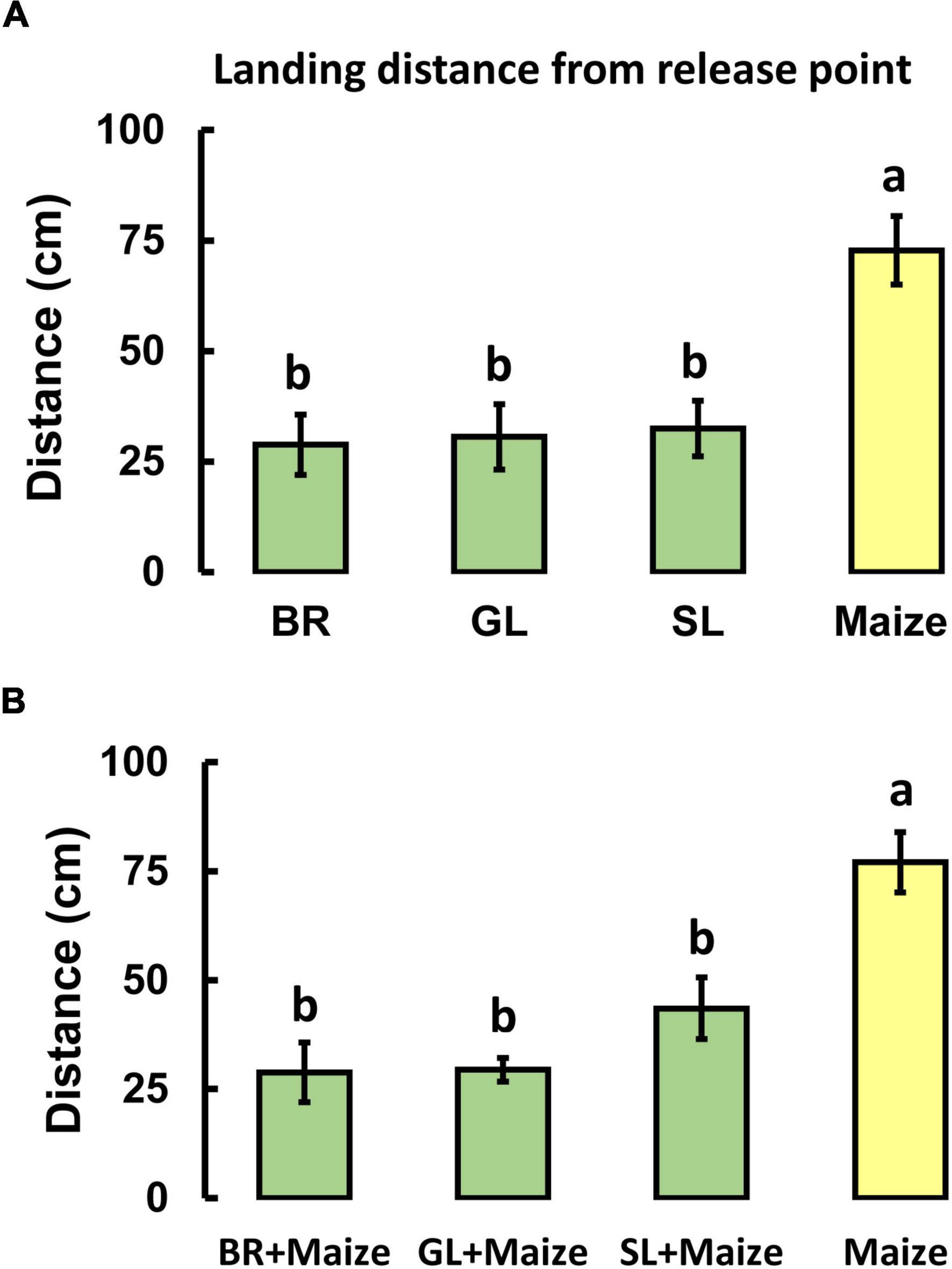
Figure 2. Response of Spodoptera frugiperda females in a wind tunnel to plant volatiles emitted by intact Brachiaria Mulato II grass (BR), greenleaf desmodium, D. intortum (GL), silverleaf desmodium, D. uncinatum (SL), and maize (SC Duma) either alone (A) or in combination (B). The results shown are means (± SE) of landing distance from the release point (n = 20). Different letters indicate statistically significant differences (P < 0.05), (F-test; Holm-Sidak method).
In the second series of bioassays, when maize volatiles were presented in combination with volatiles from Push-Pull companion plants (desmodium species or Brachiaria) a significant reduction in upwind flight toward maize plants was observed [F(3, 73) = 9.009; P < 0.001; Figure 2B].
Fall Armyworm Oviposition Bioassay
In oviposition cage experiments where S. frugiperda females have the chance to contact and lay eggs on plants, there were significant differences between plant treatments in the total number of deposited eggs on the plants [two-way ANOVA, F(5, 108) = 7.054, P < 0.001; Figure 3A]. Significantly more eggs were laid on the treatment containing maize alone compared to the rest of the treatments where companion plants were present. Overall, there were no significant differences between eggs laid on the plants compared to those laid on the cage [two-way ANOVA, F(1, 108) = 1.642, P = 0.203; Supplementary Table 2 and Figure 3A]. However, females laid significantly more eggs on the cages inner surface with the GL, SL, and M + SL treatments while they laid more eggs on plants with the maize treatment. This underscores the repellent activity of desmodium plants, particularly SL. There was a significant interaction between both factors investigated (i.e., plant treatments and oviposition sites [two-way ANOVA, F(5, 108) = 9.382, P < 0.001; Supplementary Table 2 and Figure 3A]. In the combined treatments (i.e., Maize + GL and Maize + SL), moths laid significantly more eggs on maize than on GL (P = 0.02) and SL (P = 0.01) plants (Figure 3B).
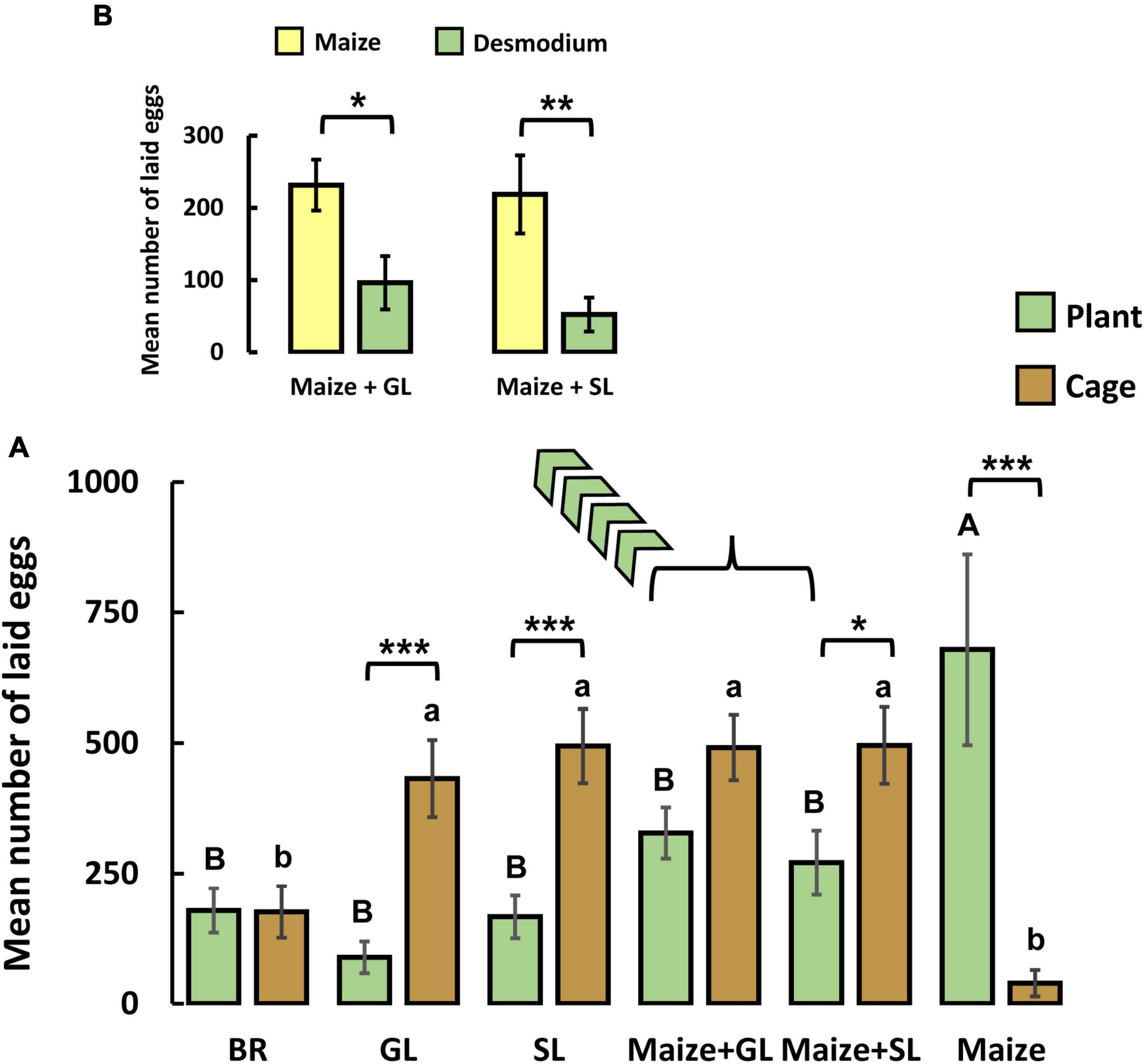
Figure 3. Mean (+ SE) number of eggs laid by five Spodoptera frugiperda females (A) when exposed to different treatments [BR = Brachiaria brizantha cv. Mulato II, GL = greenleaf desmodium (D. intortum), SL = silverleaf desmodium (D. uncinatum), Maize + GL = maize plant (SC Duma) + greenleaf desmodium, Maize + SL = maize plant (SC Duma) + silverleaf desmodium, Maize = maize plant (SC Duma)]. Egg masses were recorded on two oviposition sites (on plants and on the cage). (B) A breakdown of the recorded laid eggs by S. frugiperda females on the combined treatments (i.e., Maize + GL and Maize + SL; detail of which plants eggs were laid on is shown). Significance levels of a two-way ANOVA are shown for the factors “plant treatments,” “oviposition sites” and their interaction. Asterisks (***P < 0.001; *0.01 ≤ P ≤ 0.05) indicate statistically significant differences between “oviposition sites” (i.e., plant vs. cage within a treatment). Uppercase and lowercase letters indicate significant differences between plant treatments (two-way ANOVA; Holm-Sidak method). Asterisks in (B) indicate significant differences in eggs laid on maize and desmodium (i.e., plants offered to moths in the combined treatment) (Student’s t-test, ** = P < 0.01, * = P < 0.05).
In the oviposition compartment experiment, in a no choice test where moths were confined above plants in chambers sealed at the top and bottom with mesh cloth, there was no significant difference between plant treatments in the total number of eggs deposited on the plants [two-way ANOVA, F(4, 140) = 2.036, P = 0.093; Supplementary Table 2 and Supplementary Figure 2]. More eggs were laid at the top of the compartment than at the bottom [two-way ANOVAs, F(1, 140) = 27.413, P < 0.001; Supplementary Table 2 and Supplementary Figure 2], when moths were exposed to odors from greenleaf desmodium, D. intortum (GL), silverleaf desmodium, D. uncinatum (SL), and Brachiaria cv. Mulato II (BR). This indicates that S. frugiperda females were repelled by the volatiles of these companion plants. No interaction was found between both investigated factors [i.e., plant treatments and oviposition sites two-way ANOVA, F(4, 140) = 1.320, P = 0.265; Supplementary Table 2 and Supplementary Figure 2A]. However, moths ovipositing in the oviposition compartment above maize plants laid a similar number of eggs on the top and bottom of the compartment (Supplementary Figure 2).
Parasitoid Behavioral Responses to Natural Plant Volatile Samples
To test the hypothesis that companion plant volatiles were attractive to natural enemies, the behavioral response of two larval endoparasitoids, C. icipe and C. luteum, was assessed against the volatiles of companion plants (Figure 4) in a 4-arm olfactometer. Coccygidium luteum females spent significantly more time in the presence of volatiles from Brachiaria [F(1, 46) = 6.64, P = 0.013], D. intortum [F(1, 46) = 7.83, P = 0.007], and D. uncinatum [F(1, 46) = 9.43, P = 0.003], compared to blank controls. Similarly, C. icipe females spent significantly more time in the presence of volatiles from Brachiaria [F(1, 46) = 10.18, P = 0.002], D. intortum [F(1, 46) = 11.81, P = 0.001], and D. uncinatum [F(1, 46) = 20.15, P < 0.001] compared with blank controls.
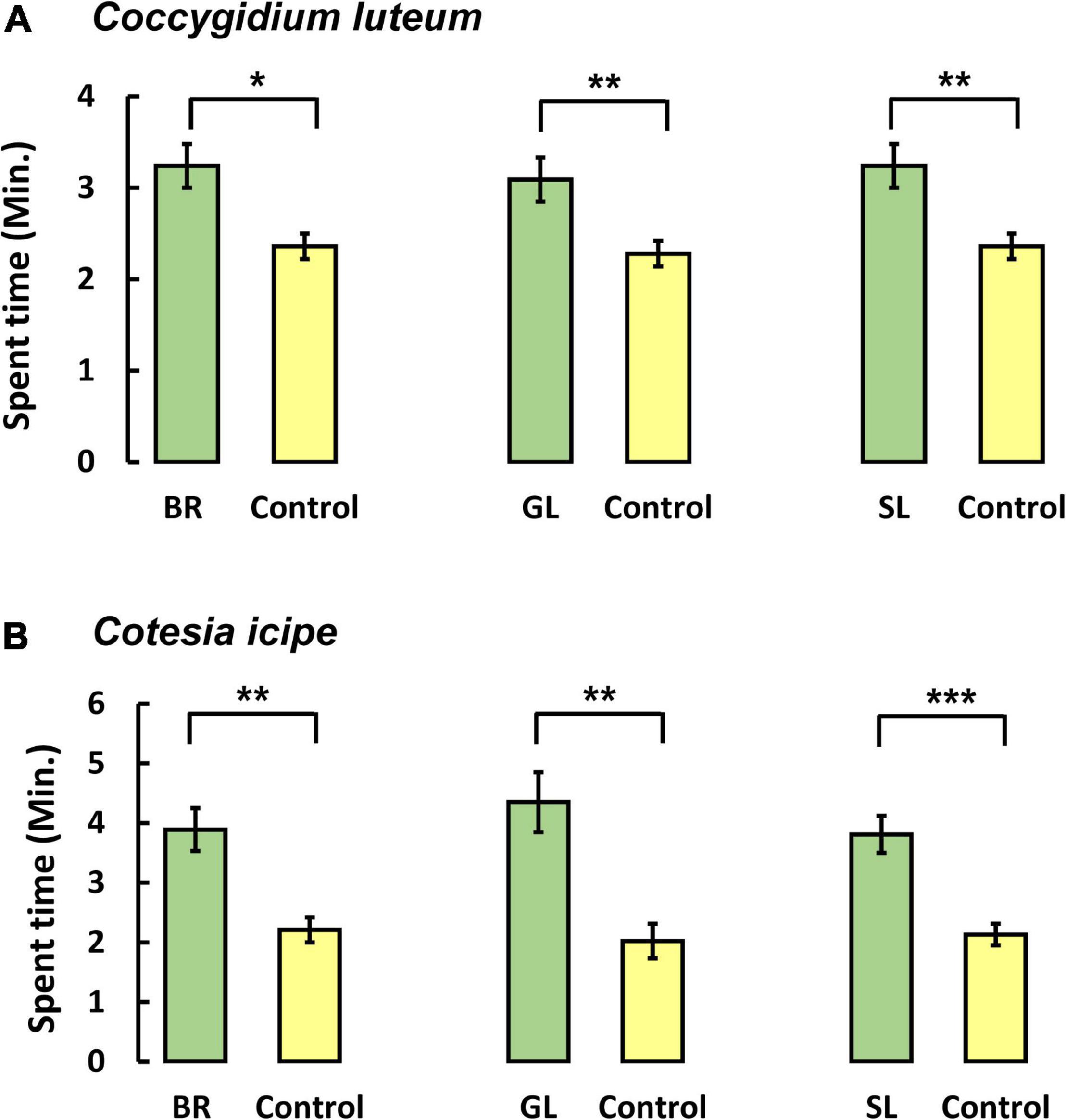
Figure 4. Behavioral responses of larval endoparasitoids (A) Coccygidum luteum (B) Cotesia icipe in a four-arm olfactometer bioassay to headspace volatiles from Brachiaria Mulato II (BR), greenleaf desmodium, D. intortum (GL) and silverleaf desmodium, D. uncinatum (SL). Each parasitoid was observed for 12 min (N = 12). Mean (± SE) for time spent (Min) in each arm of the olfactometer is shown. Parasitoid responses were compared by ANOVA after conversion of the data into proportions and log-ratio transformation. Asterisks (***P < 0.001; **0.001 ≤ P < 0.01; *0.01 ≤ P ≤ 0.05) above the bars indicate statistically significant differences based on the Student–Newman–Keuls (SNK) test (P < 0.05).
Volatile Analysis
GC-MS analysis of headspace collections from both Desmodium spp., Brachiaria Mulato II and maize detected 34 plant volatiles in eight chemical classes (alcohols, aldehydes, benzenoids, esters, homoterpenes, ketones, monoterpenes, and sesquiterpenes; Supplementary Table 2). Overall, multivariate analysis (MANOVA) revealed significant differences in the volatile profiles emitted by the different plant species, i.e., the companion plants, Desmodium spp. and Brachiaria Mulato II, and maize [Pillai’s Trace = 2.909, F(9, 72) = 3.983, P < 0.015]. Principal component analysis (PCA) of the emitted volatiles showed that the first two principal components accounted for 47.1% of the total variation in the volatile emission. Hence, these two principal components (PCs) accounted for most of the variation in the data of likely biological relevance. Furthermore, a clear separation based on the first principal component (PC1) is visible between the companion plants (i.e., Desmodium spp. and Brachiaria Mulato II) and maize (Figure 5A). Another separation based on the second principal component (PC2) is obvious between the volatile profiles of Brachiaria Mulato II and D. intortum in one cluster and D. uncinatum, suggesting that the emitted volatiles of these two groups are dissimilar.
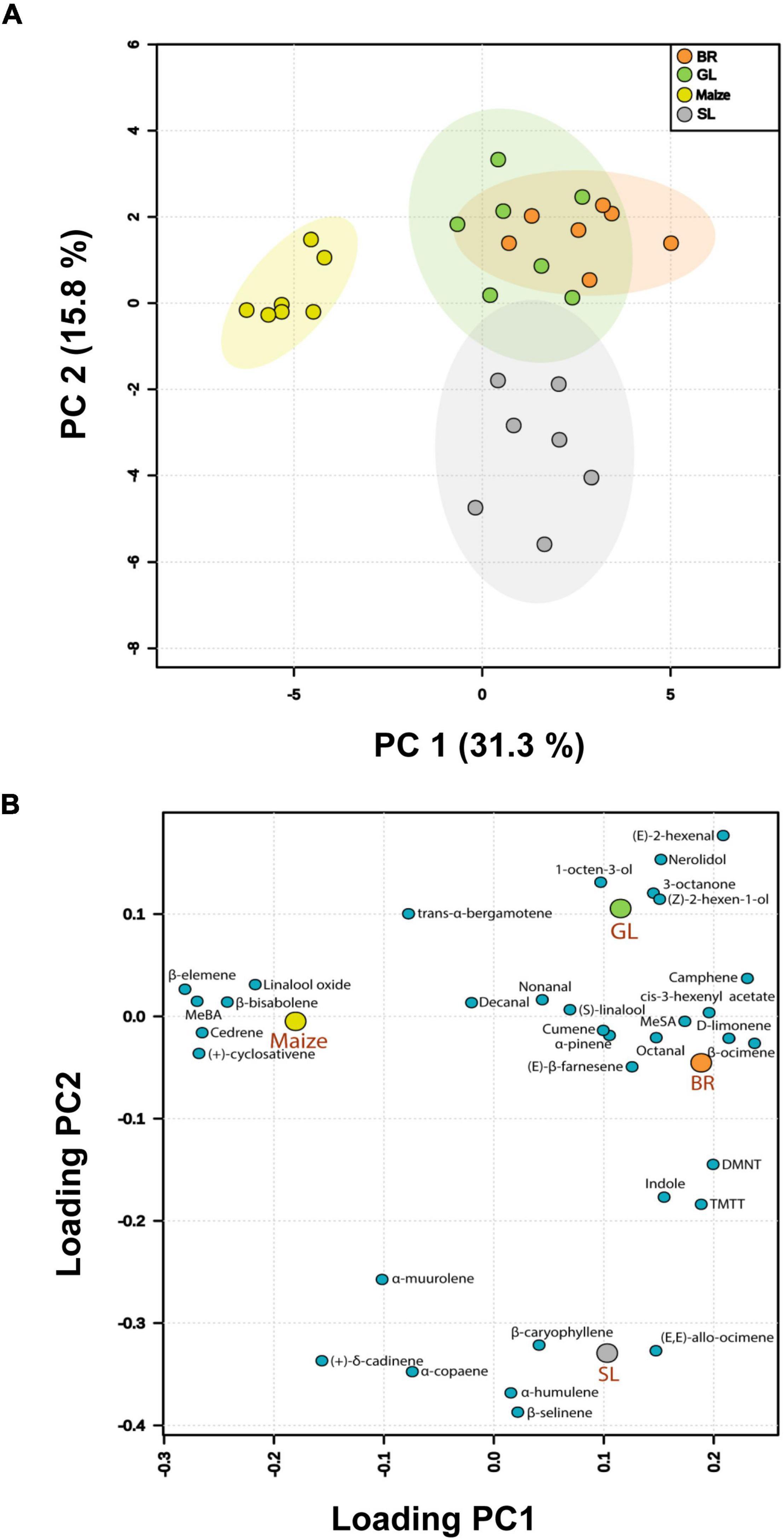
Figure 5. Principal Component Analysis (PCA) of volatile compounds emitted by intact Brachiaria Mulato II (BR), greenleaf desmodium, D. intortum (GL), sliverleaf desmodium, D. uncinatum (SL), and maize plants (n = 7) sampled for 48 h. (A) The score plot visualizes the ordination of collected samples according to the first two PCs based on the quantity of different volatiles emitted from different plant species, with the percentage of the variation explained in parentheses. The ellipses show 95% confidence regions. (B) The loading plot visualizes the contribution of each volatile to the group separation using the first two principal components.
In descending order, the greatest loadings of PC1 (Figure 5B) were for β-ocimene (0.237), camphene (0.231), D-limonene (0.215), (E)-2-hexenal (0.209), DMNT (0.199), (Z)-3-hexenyl acetate (0.196), TMTT (0.189), and methyl salicylate (0.174), whereas the major loadings of PC2 were for (E)-2-hexenal (0.176), nerolidol (0.153), 1-octen-3-ol (0.130), β-myrcene (0.120), and (Z)-2-hexen-1-ol (0.114). These volatile organic compounds (VOCs) shown to contribute to PC1 and PC2 may impact both S. frugiperda and parasitoid behavior. A heatmap showed differential magnitude of volatile emission from the companion plants (i.e., Desmodium spp. and Brachiaria Mulato II) and maize plants. Specifically, alcohols and aldehydes dominated D. intortum (GL) volatiles, but D. uncinatum (SL) profiles are characterized by the emission of sesquiterpenes. Meanwhile, Brachiaria Mulato II (BR) profiles were characterized by high emission of benzenoids and homo- and monoterpenes (Supplementary Figure 3).
Univariate analyses of variance revealed significant differences in total [F(3, 24) = 6.182, P = 0.003; Supplementary Figure 4] and individual volatile emission from the different companion plants (i.e., Desmodium spp. and Brachiaria Mulato II) and maize plants, particularly due to the high differential emission of several VOCs (Supplementary Table 3, Supporting Information).
Electrophysiology
Coupled GC-electroantennography (GC-EAG) analysis using S. frugiperda females showed electrophysiological activity for 14 volatiles from the companion plants Desmodium spp. and Brachiaria (Figure 6). The bioactive volatiles were identified by GC-MS and then confirmed by GC peak enhancement as: (Z)-3-hexenyl acetate, (S)-linalool, DMNT, (E)-β-farnesene, and TMTT from Brachiaria; (E)-2-hexenal, 3-octanone, DMNT, unknown compound, and β-caryophyllene from D. intortum; (S)-linalool, (E, E)-allo-ocimene, α-copaene, (E)-β-farnesene, β-selinene, and TMTT from D. uncinatum. In addition, S. frugiperda showed a robust EAG response to β-ocimene and MeSA, which were emitted from both Desmodium spp. and Brachiaria plants.
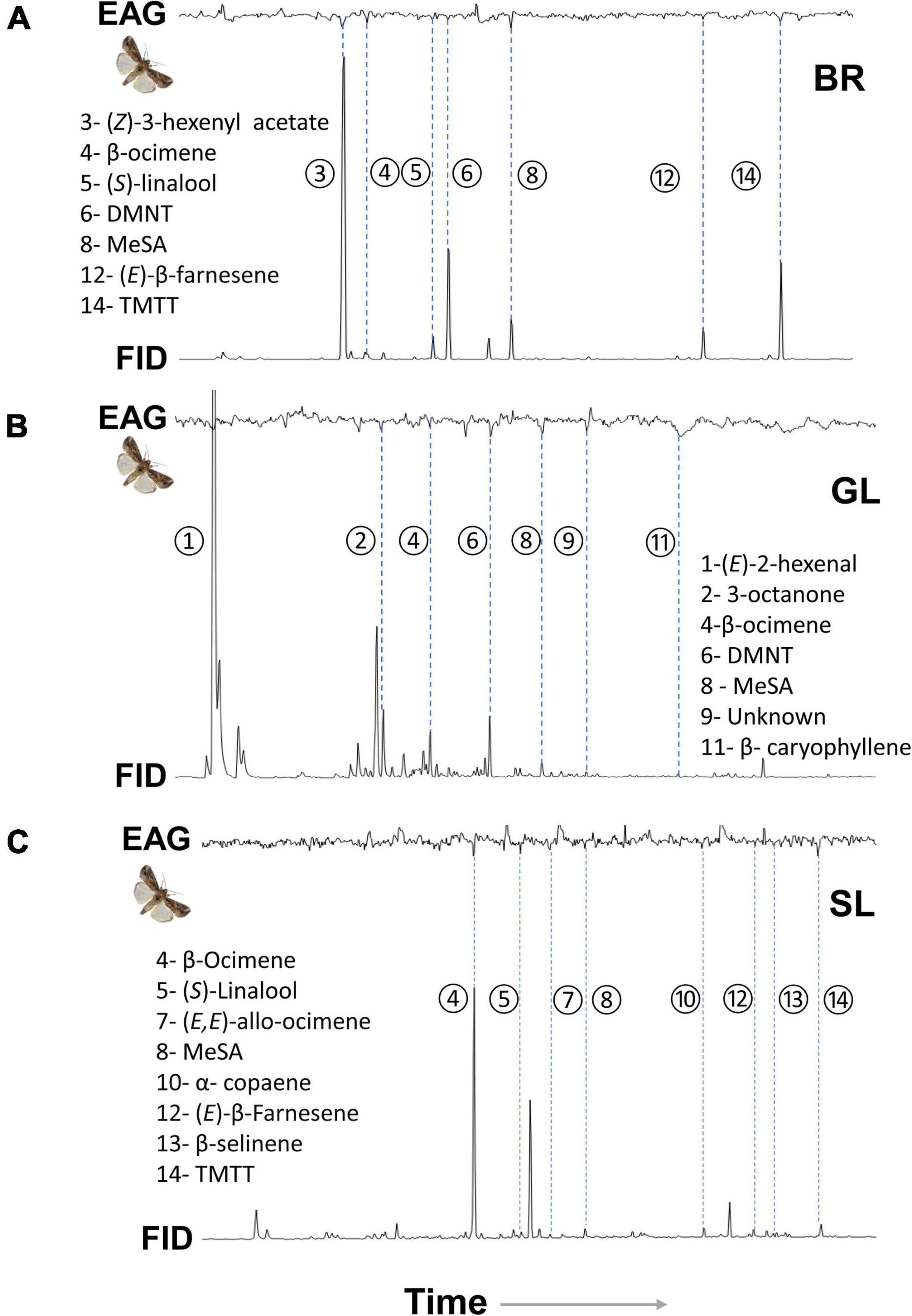
Figure 6. Coupled GC-EAG analysis showing antennal response of Spodoptera frugiperda females to headspace samples of plant volatiles collected from intact (A) Brachiaria Mulato II, (B) greenleaf desmodium, D. intortum (GL), and (C) sliverleaf desmodium, D. uncinatum (SL) plants. The EAG-active VOCs for S. frugiperda were identified as: (1) (E)-2 hexenal; (2) 3-octanone; (3) (Z)-3-hexenyl acetate; (4) β-ocimene; (5) (S)-linalool; (6) (E)-4,8-dimethyl-1,3,7-non-atriene (DMNT); (7) (E,E)-allo-ocimene; (8) methyl salicylate (MeSA); (9) unknown; (10) α-copaene; (11) β-caryophyllene; (12) (E)-β-farnesene; (13) β-selinene; (14) (E,E)-4,8,12-trimethyl-1,3,7,11-tridecatetraene (TMTT).
GC-EAG recordings from the antennae of C. luteum females showed electrophysiological activity for 11 compounds from the companion plants Desmodium spp. and Brachiaria (Figure 7A). The bioactive volatiles were tentatively identified by GC-MS and then confirmed by GC peak enhancement as: (S)-linalool, DMNT, MeSA, indole, and β-caryophyllene from Brachiaria; (E)-2-hexenal, 1-octen-3-ol, (Z)-3-hexenyl acetate, and β-ocimene from D. intortum; DMNT, (E, E)-allo-ocimene, β-caryophyllene, and TMTT from D. uncinatum.
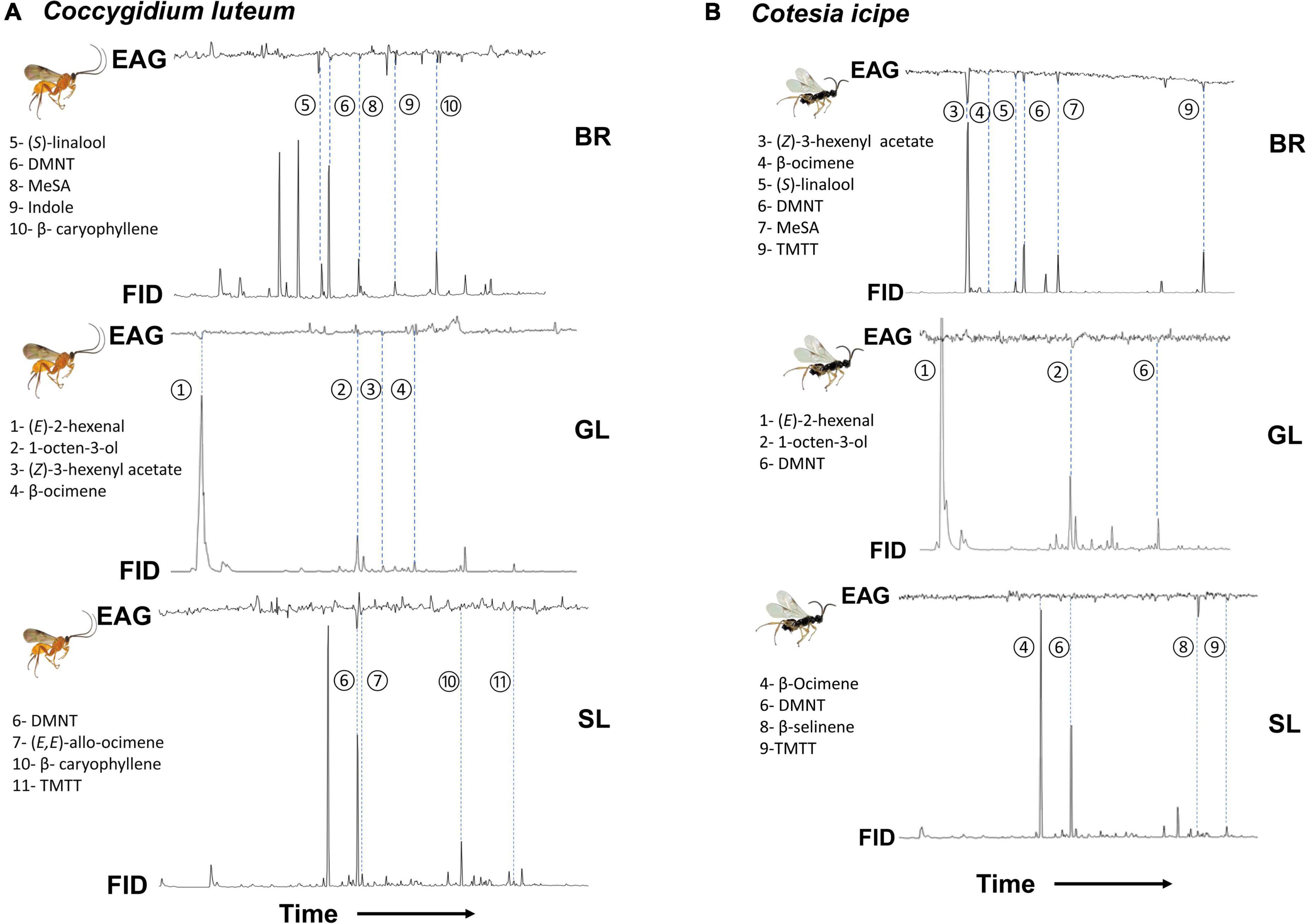
Figure 7. Coupled GC-EAG analysis showing antennal response of the female parasitoids (A) Coccygidium luteum and (B) Cotesia icipe to headspace samples of plant volatiles collected from intact Brachiaria Mulato II (BR), greenleaf desmodium, D. intortum (GL), and sliverleaf desmodium, D. uncinatum (SL) plants. The EAG-active compounds for C. luteum were identified as: (1) (E)-2 hexenal, (2) 1-octen-3-ol, (3) (Z)-3-hexenyl acetate, (4) β-ocimene, (5) (S)-linalool, (6) (E)-4,8-dimethyl-1,3,7-non-atriene (DMNT), (7) (E,E)-allo-ocimene, (8) methyl salicylate (MeSA), (9) indole, (10) β-caryophyllene and (11) (E,E)-4,8,12-trimethyl-1,3,7,11-tridecatetraene (TMTT). Whereas (E)-2 hexenal (1), 1-octen-3-ol (2), (Z)-3-hexenyl acetate (3), β-ocimene (4), (S)-linalool (5), DMNT (6), MeSA (7), β-selinene (8), and TMTT (9) were identified as EAG-active compounds for C. icipe.
GC-EAG recordings from the antennae of C. icipe females showed electrophysiological activity for 9 volatiles from the companion plants Desmodium spp. and Brachiaria (Figure 7B). The bioactive volatiles were tentatively identified by GC-MS and then confirmed by GC peak enhancement as: (Z)-3-hexenyl acetate, β-ocimene, (S)-linalool, MeSA, and TMTT from Brachiaria; (E)-2-hexenal and 1-octen-3-ol from D. intortum; β-ocimene, β-selinene, and TMTT from D. uncinatum. In addition, C. icipe showed a robust EAG response to DMNT, which was emitted from both Desmodium spp. and Brachiaria plants.
Fall Armyworm Flight Response to Synthetic Compounds in Wind Tunnel
To determine the role of companion plant volatiles in modifying S. frugiperda flight behavior, we tested blends of the synthetic plant volatiles formulated at the same concentration and ratio as in the headspace samples of the companion plants (Supplementary Table 1). Moth responses to maize volatiles presented alone and in combination with the greenleaf desmodium (GL) and silverleaf desmodium (SL) synthetic blends were compared. In the wind tunnel bioassay, S. frugiperda showed attraction to maize alone by flying further upwind to headspace volatiles of maize plants presented alone compared to maize volatile released in combination with synthetic blends of companion plant volatiles or solvent control [F(3, 67) = 7.32, P < 0.001] (Figure 8). Moreover, when presented with maize volatiles in combination with companion plant volatiles, S. frugiperda moths made fewer close approaches to the odor sources and made more return flights back down the tunnel, toward the release point, compared to maize volatiles presented alone.
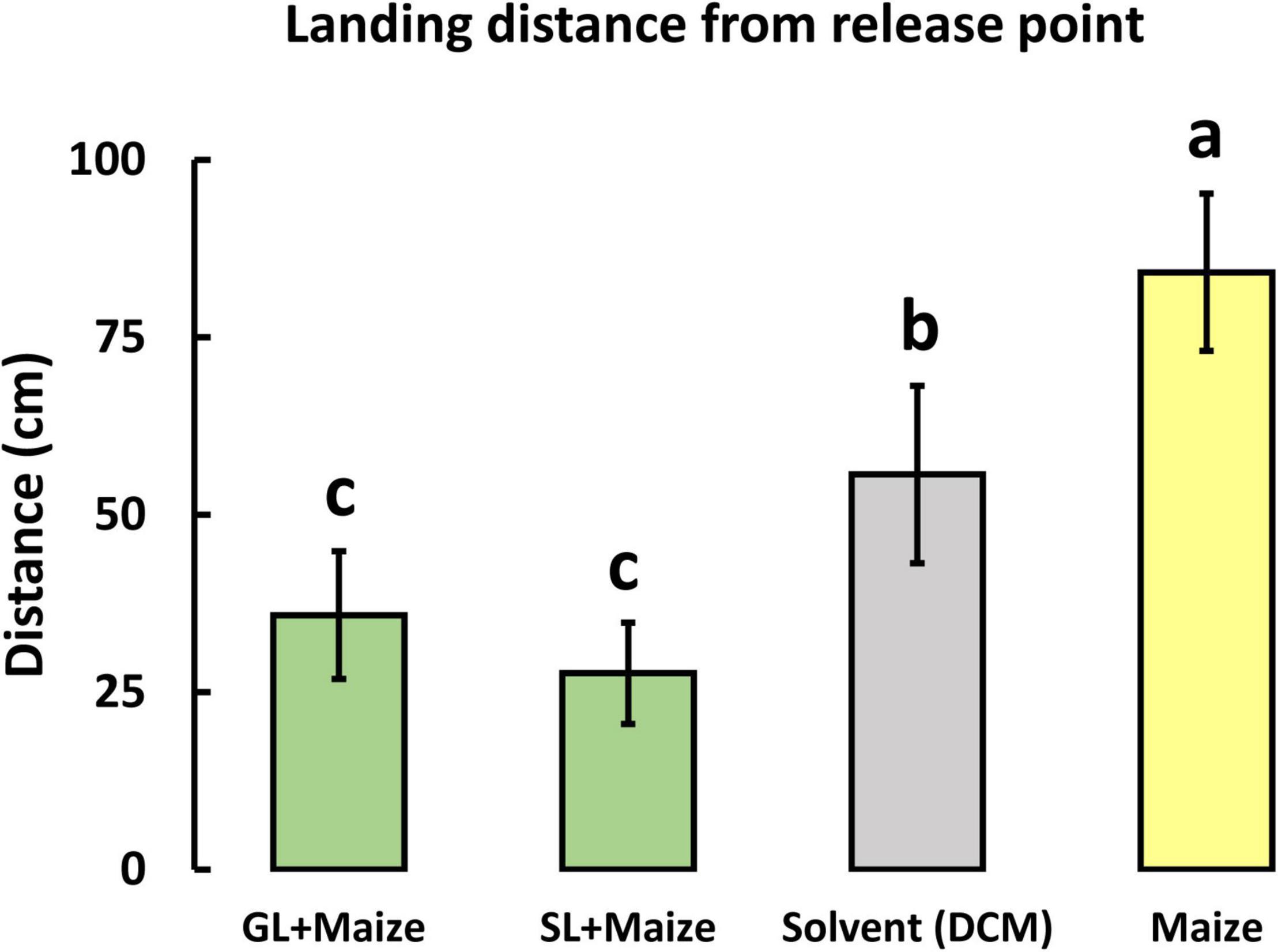
Figure 8. Response of Spodoptera frugiperda females in a wind tunnel to synthetic blends of electrophysiological active volatiles emitted by intact maize (SC Duma) either alone (Maize) or in combination with D. intortum—greenleaf desmodium (GL + Maize), D. uncinatum—silverleaf desmodium (SL + Maize) and solvent (dichloromethane) control. The results shown are mean (± SE) landing distance from the release point (n = 20). Different letters indicate statistically significant differences (P < 0.05; F-test with Holm-Sidak method).
Parasitoid Behavioral Responses to Synthetic Compounds
Parasitoid responses to synthetic blends of bioactive companion plant volatiles formulated in the same concentration and ratio as the natural headspace samples were tested. Observed results confirmed behavioral responses of the parasitic wasps to the identified companion crop volatiles. In four-way olfactometer bioassays, the synthetic blends (0.5 μg dose) of D. intortum volatiles elicited potent attraction of both C. luteum [F(1, 46) = 7.37, P = 0.009] and C. icipe [F(1, 42) = 25.95, P < 0.001]. Similarly, the parasitic wasps were significantly attracted to synthetic equivalents of D. uncinatum [C. luteum: F(1, 42) = 10.73, P = 0.002; C. icipe: F(1, 46) = 4.68, P = 0.035], and Brachiaria volatiles [C. luteum: F(1, 42) = 11.04, P = 0.001; C. icipe: F(1, 42) = 5.42, P = 0.024] compared to solvent controls (Figure 9). Responses to synthetic blends were similar to natural samples indicating that the correct bioactive compounds had been identified. Olfactometer response (time spent in the treated area minus time spent in the control area) was compared using an unpaired t-test. For both parasitoid species (C. luteum and C. icipe), there was no significant difference in response to natural plant headspace samples and synthetic blends for all three plant species (C. luteum: P = 0.62, P = 0.64, P = 0.36 for D. intortum, D. uncinatum, and Brachiaria, respectively; C. icipe: P = 0.95, P = 0.94, P = 0.90 for D. intortum, D. uncinatum, and Brachiaria, respectively).
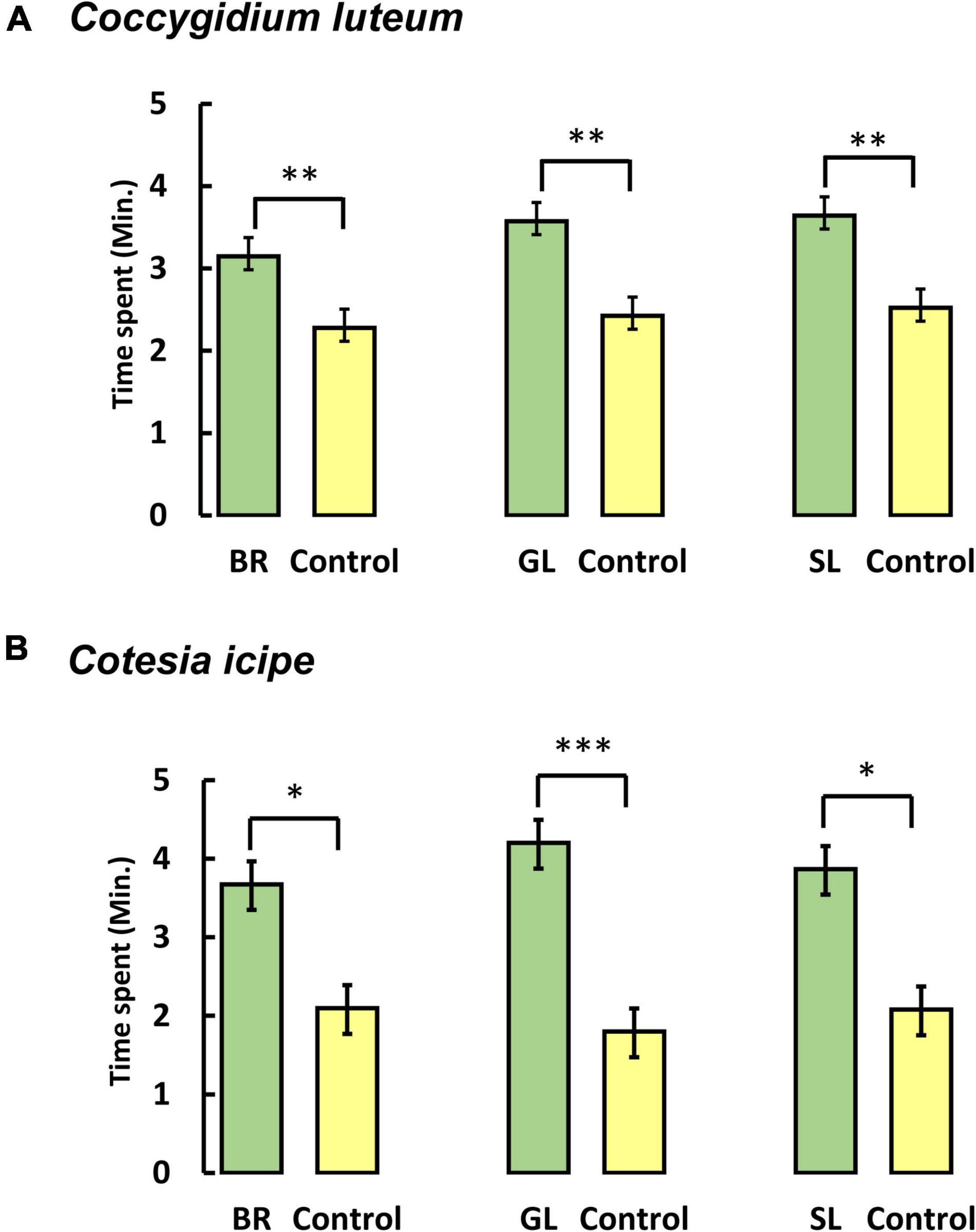
Figure 9. Behavioral responses of the larval endoparasitoids (A) Coccygidum luteum (B) Cotesia icipe in a four-arm olfactometer bioassay to synthetic blends of electrophysiological active headspace volatiles emitted by Brachiaria Mulato II (BR), greenleaf desmodium, D. intortum (GL), and silverleaf desmodium, D. uncinatum (SL). Each parasitoid female was observed for 12 min (N = 12). Mean (± SE) time spent in each arm of the olfactometer is shown. Parasitoid responses were compared by ANOVA after conversion of the data into proportions and log-ratio transformation. Asterisks (***P < 0.001; **0.001 ≤ P < 0.01; *0.01 ≤ P ≤ 0.05) above the bars indicate statistically significant differences based on the Student–Newman–Keuls (SNK) test (P < 0.05).
Field Survey
Extensive field surveys confirmed earlier reports and showed that S. frugiperda infestation and damage levels were substantially reduced when Push-Pull companion cropping was used (Figure 10). Overall mean (±S.E.) infestation level (larvae per 100 plants) was 6.6 times lower in Push-Pull plots in the short rains 2019 (Push-Pull: 3.69 ± 0.34; Monocrop maize: 24.68 ± 0.60) and 7.7 times lower in the long rains 2019 (Push-Pull: 3.21 ± 0.10; Monocrop maize: 24.85 ± 0.34). Mean damage level was 3.6 times lower in the short rains 2019 (Push-Pull: 13.04 ± 0.15; Mono maize: 47.95 ± 0.16) and 4.89 times lower in the long rains 2019 (Push-Pull: 9.30 ± 0.18; Monocrop maize: 45.51 ± 0.17). Two-way ANOVA, taking districts (i.e., different sampling locations) and farming system (i.e., Push-Pull vs. monocrop maize) as factors, revealed that the overall S. frugiperda infestation level and damage level were significantly affected by both factors and their interactions (Supplementary Table 4 and Figure 10A). Furthermore, similar findings were observed during the long rains season that districts, farming system and their interactions significantly affect the overall S. frugiperda infestation level and damage level (Supplementary Table 2 and Figure 10B).
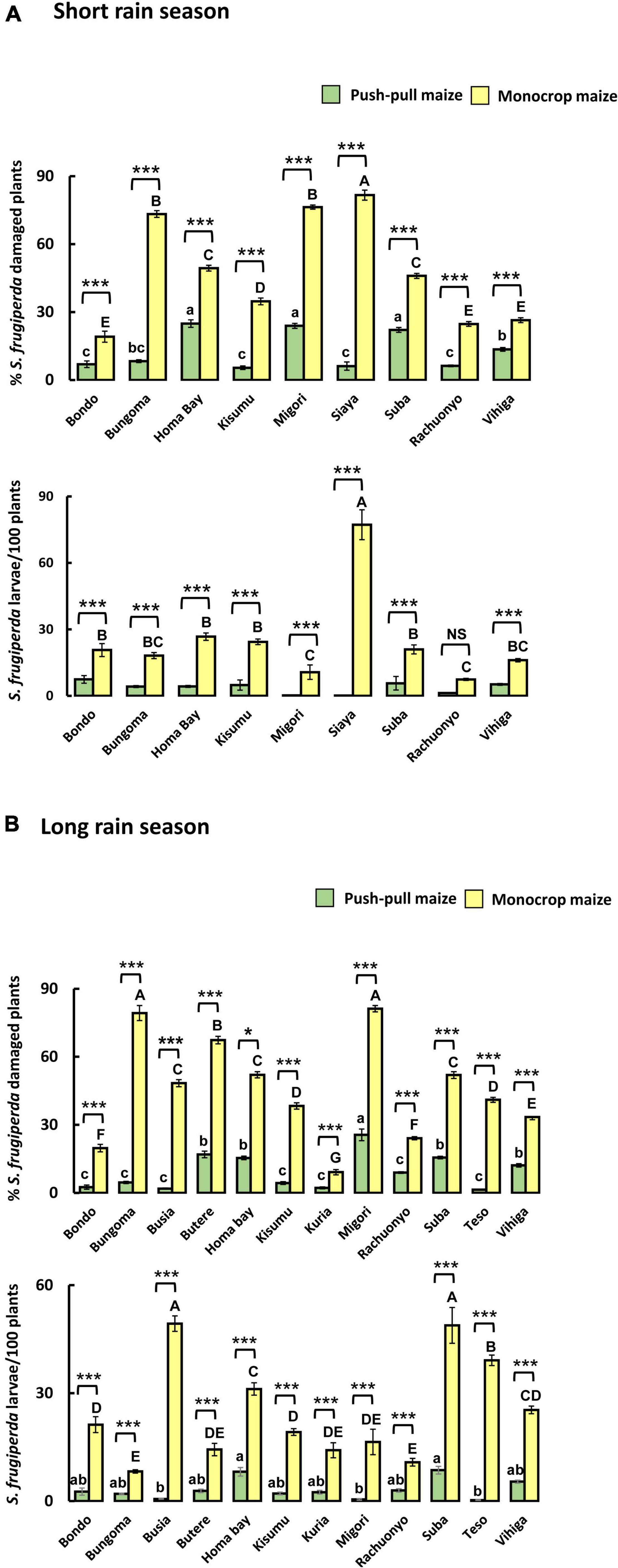
Figure 10. Mean (± SE) number of fall armyworm (FAW) (Spodoptera frugiperda) larvae and proportion of maize plants damaged in plots of maize planted in single stands (monocrop) or in push–pull fields in different sub-counties in western Kenya. Means are of 30 different farms in the short (A) and long (B) rains seasons. Significance levels of a two-way ANOVA are shown for the factors “farming systems,” “districts” and their interaction. Asterisks (***P < 0.001; *P < 0.05) indicate statistically significant differences between “farming systems” within “districts,” whereas different letters indicate significant differences between “districts” within “farming systems.” Uppercase letters indicate differences between monocrop fields, whereas lowercase letters indicate differences between Push-Pull fields in different districts. NS indicates non-significant effects (P < 0.05) (two-way ANOVA; Holm-Sadik method).
Higher percentage parasitism was found in larvae collected from companion cropped maize in all four districts sampled. The mean parasitism level of larvae sampled from Push-Pull companion cropped maize was 18.7%, whereas it was only 2.1% in monocropped maize plots. Two-way ANOVA analysis showed that “farming system” [two-way ANOVA, F(1, 44) = 28.452, P < 0.001] and “districts” [two-way ANOVA, F(3, 44) = 3.026, P = 0.039] had a statistically significant impact on parasitism rate (Supplementary Figure 5), but no interaction effect occurred [two-way ANOVA, F(3, 44) = 2.034, P = 0.123].
Discussion
The aim of our study was to elucidate how “Push-Pull” companion cropping reduces herbivore damage in maize by the fall armyworm, S. frugiperda, an invasive crop pest in Africa (Midega et al., 2018). Field studies had shown an approximately fivefold reduction in S. frugiperda crop damage in plots of Push-Pull maize planted with specific companion crop species, compared to monocropped maize plots (Hailu et al., 2018; Midega et al., 2018). It was hypothesized that mechanisms such as repellence of S. frugiperda moths and attraction of larval parasitoid wasps were responsible but bioactive semiochemicals had not been identified or characterized previously. Previous studies highlighted the low attraction and survival of S. frugiperda larvae in companion crops (Scheidegger et al., 2021). However, the details of the chemical ecology of the insect-plant interactions involved were not previously known and adult behavior in response to plant volatiles had not been studied. Push-Pull plots consist of an intercrop between maize rows and a border crop surrounding small plots. Our findings suggest that reduced herbivory is due to constitutive emission of key volatiles, identified here, that have two effects: first they repel egg laying S. frugiperda (push) and second they attract parasitoid wasp natural enemies (pull) that provide biological control.
In an earlier Push-Pull system, used against stemborers, the intercrop was repellent while the border crop was attractive to herbivores and functioned as a trap crop (Khan et al., 1997b). Here, we provide evidence showing that Desmodium spp. intercrops emit volatiles that repel egg laying S. frugiperda moths, as was hypothesized, and identify the bioactive compounds mediating the interaction. This is a “push” component of the Push-Pull system. More surprisingly, we found that the border crop, Brachiaria Mulato II, also functions as a “push” companion plant: it significantly reduced upwind flight toward maize in a wind tunnel bioassay. A border crop that is repellent to S. frugiperda moths contrasts with the earlier Push-Pull system that had Napier grass, Pennisetum purpureum Schumach, as the border crop and was attractive to stemborers (Khan et al., 1997b). So, the question arises as to whether the current system should still be called a “Push-Pull” system? If we were only considering the behavior of the herbivore, then it would simply be a repellent companion cropping system without any “pull.” However, we also hypothesized that companion crop volatiles could attract natural enemies of the herbivore. If insects at the third trophic level are attracted, as they were in the original Push-Pull system, then this would provide a “pull” component. We will return to this later in the discussion as parasitoid attraction was our second hypothesis and herbivore repulsion was considered first.
In wind tunnel bioassays, S. frugiperda moths flew significantly further upwind toward maize volatiles than to volatiles of Desmodium spp. and Brachiaria, indicating that maize volatiles were attractive. However, when maize volatiles were presented in a combination, mixed with desmodium volatiles (either silverleaf D. uncinatum—SL or greenleaf D. intortum—GL), moths made significantly less upwind flight compared to maize volatiles alone. This indicated that the desmodium intercrop had a repellent effect and switched off moth attraction. In cage experiments, significantly fewer eggs were laid on maize plants when accompanied by either Desmodium species (GL or SL). In no choice oviposition bioassays, S. frugiperda laid significantly fewer eggs near D. intortum, preferring to lay more eggs on the upper side of the oviposition chambers. Taken together, these results indicate that moths were repelled by the companion plant volatiles. Our findings contrast with a very recent and surprising preprint by Erdei et al. (2022) who claim that D. intortum does not release volatiles constitutively at all. Our chemical analysis data, together with insect electrophysiological and behavioral data, show (1) that D. intortum clearly releases volatiles and (2) that insects are sensitive to the volatiles released that are reported here.
The bioactive volatiles that elicited responses from female S. frugiperda antennae were identified as (Z)-3-hexenyl acetate, (S)-linalool, DMNT, (E)-β-farnesene, TMTT, (E)-2-hexenal, 3-octanone, DMNT, β-caryophyllene, (E, E)-allo-ocimene, α-copaene, β-selinene, and β-ocimene. Bioactive compounds were selected on the basis of EAG activity and some were minor components of the plant volatile profile. Previous studies have shown that minor components can be important for eliciting insect responses (D’Alessandro et al., 2009) and increased responses have even been observed with lower volatile emission provided the volatile profiles are enriched with the right bioactive compounds (Bruce et al., 2010). How plant volatiles are perceived in the insect brain is not the same as what is shown in the PCA. The PCA is based on amounts of compounds quantified by flame ionization detector (FID) response whereas the insect will not be sensitive to all these compounds. Indeed, this is the reason why we did GC-EAG studies so that we could use the insect antenna as a biological detector to see which compounds the insects were sensitive to. Our aim was to test the effect of volatiles constitutively released by the companion plants before arrival of herbivores. Volatiles were therefore collected from uninfested plants because this is the situation before the moths arrive.
The volatiles identified here that are constitutively emitted by Desmodium and Brachiaria companion crops are similar to those emitted from maize upon S. frugiperda herbivory (Pinto-Zevallos et al., 2016; De Lange et al., 2020). It is well documented that the volatiles constitutively emitted from Desmodium and Brachiaria companion crops, i.e., (E)-2-hexenal, (Z)-3-hexenyl acetate, (E)-β-ocimene, (S)-linalool, DMNT, MeSA, indole, β-caryophyllene, (E)-β-farnesene, and TMTT, also serve as herbivore-induced plant volatiles (HIPVs) in maize and repel herbivores and attract their natural enemies (Turlings et al., 1991; De Moraes et al., 1998, 2001; Kessler and Baldwin, 2001; Tamiru et al., 2015; Richter et al., 2016; Magara et al., 2020). It seems plausible that emission of these volatile compounds can explain the reduced infestation of S. frugiperda in maize intercropped with companion crops. Desmodium uncinatum (SL) and Brachiaria Mulato II emitted significantly more volatiles compared to D. intortum (GL) due to their high emission of terpenoids. The volatile profiles of companion crops were different; whereas alcohols, aldehydes and ketones dominated D. intortum volatiles, Brachiaria Mulato II, and D. uncinatum profiles were characterized by the emission of benzenoids, esters, and terpenoids.
In contrast to the herbivore, we found evidence that two key larval parasitoids of fall armyworm, C. icipe and C. luteum, were attracted to the volatiles of all three companion plant species in the olfactometer bioassay. Furthermore, in the field, the percentage parasitism of larvae collected from Push-Pull plots was nine times higher than in monocrop maize plots. Attraction of parasitoids is of interest because recent studies have demonstrated that native natural enemies such as C. icipe and C. luteum can reduce damage caused by S. frugiperda in maize (Sisay et al., 2019; Agboyi et al., 2020). Our data show that 11 volatiles constitutively emitted by companion plants elicited electrophysiological responses from parasitoids and that parasitoids were attracted to natural samples and synthetic blends of companion plant volatiles in an olfactometer bioassay. These bioactive volatiles, emitted from companion plants, are well known as attractants to parasitoids and are commonly emitted from plants upon herbivore attack as “call for help” signals (Turlings and Wäckers, 2004), even if the primary function of HIPVs is still subject to debate (Turlings and Erb, 2018). Specifically, the electrophysiologically active homoterpenes DMNT and TMTT, which are emitted from both Desmodium and Brachiaria plants, are very attractive to braconid parasitoids (Turlings et al., 1991; Khan et al., 1997a; Mutyambai et al., 2015; Tamiru et al., 2015). In addition, other electrophysiologically active volatiles such as (E)-2-hexenal, (Z)-3-hexenyl acetate, (E)-β-ocimene, 1-octen-3-ol, (S)-linalool, MeSA, indole, and β-caryophyllene, which are constitutively emitted from Desmodium and Brachiaria plants, are well documented as bioactive plant volatiles induced in response to caterpillar herbivory and egg laying (Turlings et al., 1991; Kigathi et al., 2009; Tamiru et al., 2011), and are also considered key attractants for parasitic wasps (Turlings et al., 1991; De Moraes et al., 1998; Snoeren et al., 2010; Tamiru et al., 2015). In a recent genome wide association study of C. partellus egg-induced parasitoid attraction in maize, we found candidate genes that are involved in volatile biosynthesis (Tamiru et al., 2020).
A meta-analysis of studies has shown that diversified crops enhance natural enemy populations, increase pest suppression, and lower crop damage (Letourneau et al., 2011). In particular, diversified maize fields had the highest richness and abundance of natural enemies, including several parasitoids that are reported to attack S. frugiperda (Quispe et al., 2017). Our field results showed higher parasitism of S. frugiperda in Push-Pull plots despite its recent invasion to the region. Although it is possible that invasive herbivores such as S. frugiperda could disrupt chemically mediated interactions between plants and higher trophic users of HIPVs such as parasitoids (Desurmont et al., 2014), it has been shown that changing the chemical complexity of the habitat through the release of certain volatile compounds, similar to our Push-Pull fields, can significantly increase the foraging behavior and abundance of natural enemies such as parasitoids (Letourneau et al., 2011). Although being a new invasive pest, a number of native parasitoid species have already been reported from S. frugiperda-infested maize fields in East Africa (Sisay et al., 2019). Hence, reduced plant damage and infestation by S. frugiperda in Push-Pull companion cropping system can be partly attributed to enhanced ecosystem services by attracting these native natural enemies. Furthermore, the observed increase in parasitism suggests that parasitoids are attracted to companion plant volatiles under realistic field conditions as observed in the lab study. Intercrops that constitutively emit volatiles to repel C. partellus and B. fusca stemborers and attract their parasitoids have been used widely in East Africa for over 20 years to manage cereal stemborer pests (Khan et al., 1997a,2010). This suggests that there is enough of a selective advantage for insects to continue responding to the volatiles for habituation not to occur. Parasitoids are highly active and in the absence of hosts would move on after initial attraction but when C. partellus, B. fusca, or S. frugiperda are present then parasitism increases.
There is a need to redesign agroecosystems to reduce their vulnerability to pests, reduce reliance on pesticides, and enhance biological control (Pretty and Bharucha, 2014). The initial response to the S. frugiperda invasion in sub-Saharan Africa was widespread use of broad-spectrum insecticides. While this might give short-term reductions in pest populations, there are problems relating to the evolution of insecticide resistance and collateral damage to natural enemy populations. The use of companion plants that repel insect pests and attract their natural enemies is more compatible with other eco-friendly pest management options such as conservation biological control and can play an important role in managing the invasive pest S. frugiperda. Further research is required into how parasitoid foraging can be optimized. While the current results are encouraging in terms of the increased parasitism observed in Push-Pull plots, it is possible that higher efficiency could be attained if HIPV emission from the maize itself was increased after herbivory. We have recently identified maize germplasm that releases HIPVs after C. partellus oviposition (Tamiru et al., 2020). However, De Lange et al. (2020) have shown that S. frugiperda appears to be better adapted to maize because it suppresses HIPV emission. This reduction in indirect defense capacity in maize could mean that companion plants attractive to parasitoids may be particularly helpful for managing S. frugiperda.
Data Availability Statement
The original contributions presented in the study are included in the article/Supplementary Material, further inquiries can be directed to the corresponding author/s.
Author Contributions
IS, AT, XC, CM, ZK, and TB conceived the ideas and designed the experiments. IS performed the electrophysiological recordings and gas chromatography analyses. AT performed behavioral bioassays with wind tunnel and olfactometer. XC and FC performed oviposition assays. CM and DN performed the field experiments. IS, AT, XC, FC, and DN analyzed the data. IS and TB identified volatiles and led the writing of the manuscript. All authors contributed critically to the drafts and gave final approval for publication.
Funding
This work was funded by the BBSRC Research grant BB/R020795/1 and EU FAW−IPM project (FOOD/2018/402−634). We gratefully acknowledge the financial support for icipe research by European Union, UK’s Foreign, Commonwealth and Development Office (FCDO), the Swedish International Development Cooperation Agency (Sida), the Swiss Agency for Development and Cooperation (SDC), and the Federal Democratic Republic of Ethiopia and the Government of the Republic of Kenya.
Conflict of Interest
The authors declare that the research was conducted in the absence of any commercial or financial relationships that could be construed as a potential conflict of interest.
Publisher’s Note
All claims expressed in this article are solely those of the authors and do not necessarily represent those of their affiliated organizations, or those of the publisher, the editors and the reviewers. Any product that may be evaluated in this article, or claim that may be made by its manufacturer, is not guaranteed or endorsed by the publisher.
Acknowledgments
We thank Derrick Odhiambo, Sheryl Ondasi, Amos Gadi, Collins Onjura, Isaac Odera, Silas Ouko, Basilio Njiru, and Onesmus K. Wanyama for technical assistance, insect rearing and field experiments. IS gratefully acknowledges the permission of Suez Canal University, Ismailia, Egypt for a postdoctoral leave of absence at Keele University, United Kingdom.
Supplementary Material
The Supplementary Material for this article can be found online at: https://www.frontiersin.org/articles/10.3389/fevo.2022.883020/full#supplementary-material
References
Agboyi, L. K., Goergen, G., Beseh, P., Mensah, S. A., Clottey, V. A., Glikpo, R., et al. (2020). Parasitoid complex of fall armyworm, Spodoptera frugiperda, in Ghana and Benin. Insects 11:68. doi: 10.3390/insects11020068
Agelopoulos, N. G., Hooper, A. M., Maniar, S. P., Pickett, J. A., and Wadhams, L. J. (1999). A Novel approach for isolation of volatile chemicals released by individual leaves of a plant in situ. J. Chem. Ecol. 25, 1411–1425.
Aitchison, J. (1982). The statistical analysis of compositional data. J. R. Stat. Soc. Ser. B 44, 139–177.
Bruce, T. J. A., Midega, C. A. O., Birkett, M. A., Pickett, J. A., and Khan, Z. R. (2010). Is quality more important than quantity? Insect behavioural responses to changes in a volatile blend after stemborer oviposition on an African grass. Biol. Lett. 6, 314–317. doi: 10.1098/rsbl.2009.0953
Chambers, D. L. (1977). Quality control in mass rearing. Annu. Rev. Entomol. 22, 289–308. doi: 10.1146/annurev.en.22.010177.001445
Chong, J., Soufan, O., Li, C., Caraus, I., Li, S., Bourque, G., et al. (2018). MetaboAnalyst 4.0: Towards more transparent and integrative metabolomics analysis. Nucleic Acids Res. 46, W486–W494. doi: 10.1093/nar/gky310
D’Alessandro, M., Brunner, V., von Mérey, G., and Turlings, T. C. J. (2009). Strong attraction of the parasitoid Cotesia marginiventris towards minor volatile compounds of maize. J. Chem. Ecol. 35, 999–1008. doi: 10.1007/s10886-009-9692-7
Day, R., Abrahams, P., Bateman, M., Beale, T., Clottey, V., Cock, M., et al. (2017). Fall armyworm: impacts and implications for Africa. Outlooks Pest Manag. 28, 196–201. doi: 10.1564/v28
De Lange, E. S., Laplanche, D., Guo, H., Xu, W., Vlimant, M., Erb, M., et al. (2020). Spodoptera frugiperda caterpillars suppress herbivore-induced volatile emissions in Maize. J. Chem. Ecol. 46, 344–360. doi: 10.1007/s10886-020-01153-x
De Moraes, C. M., Lewis, W. J., Paré, P. W., Alborn, H. T., Tumlonson, J. H., and Pare, P. W. (1998). Herbivore-infested plants selectively attract parasitoids. Nature 393, 570–573. doi: 10.12688/f1000research.16927.2
De Moraes, C. M., Mescher, M. C., and Tumlinson, J. H. (2001). Caterpillar-induced nocturnal plant volatiles repel conspecific females. Nature 410, 577–580. doi: 10.1038/35069058
Desurmont, G. A., Harvey, J., Van Dam, N. M., Cristescu, S. M., Schiestl, F. P., Cozzolino, S., et al. (2014). Alien interference: Disruption of infochemical networks by invasive insect herbivores. Plant Cell Environ. 37, 1854–1865. doi: 10.1111/pce.12333
Erb, M., Balmer, D., de Lange, E. S., von Mérey, G., Planchamp, C., Robert, C. A. M., et al. (2011). Synergies and trade-offs between insect and pathogen resistance in maize leaves and roots. Plant Cell Environ. 34, 1088–1103. doi: 10.1111/j.1365-3040.2011.02307.x
Erdei, A. L., David, A. B., Savvidou, E. C., Džemedžionaitė, V., Chakravarthy, A., Molná, B. P., et al. (2022). The push-pull intercrop Desmodium does not repel, but intercepts and kills pests. bioRxiv [Preprint]. doi: 10.1101/2022.03.08.482778
Hailu, G., Niassy, S., Khan, Z. R., Ochatum, N., and Subramanian, S. (2018). Maize–legume intercropping and push–pull for management of fall armyworm, stemborers, and striga in Uganda. Agron. J. 110, 2513–2522. doi: 10.2134/agronj2018.02.0110
Jaramillo, J., Torto, B., Mwenda, D., Troeger, A., Borgemeister, C., Poehling, H. M., et al. (2013). Coffee berry borer joins bark beetles in coffee klatch. PLoS One 8:e74277. doi: 10.1371/journal.pone.0074277
Kassie, M., Wossen, T., De Groote, H., Tefera, T., Sevgan, S., and Balew, S. (2020). Economic impacts of fall armyworm and its management strategies: Evidence from southern Ethiopia. Eur. Rev. Agric. Econ. 47, 1473–1501. doi: 10.1093/erae/jbz048
Kessler, A., and Baldwin, I. T. (2001). Defensive function of herbivore-induced plant volatile emissions in nature. Science 291, 2141–2144. doi: 10.1126/science.291.5511.2141
Khan, Z. R., Ampong-Nyarko, K., Chiliswa, P., Hassanali, A., Kimani, S., Lwande, W., et al. (1997a). Intercropping increases parasitism of pests. Nature 388, 631–632. doi: 10.1038/41681
Khan, Z. R., Chiliswa, P., Ampong-Nyarko, K., Smart, L. E., Polaszek, A., Wandera, J., et al. (1997b). Utilisation of wild gramineous plants for management of cereal stemborers in Africa. Insect Sci. Appl. 17, 143–150. doi: 10.1017/s1742758400022268
Khan, Z. R., Hassanali, A., Overholt, W., Khamis, T. M., Hooper, A. M., Pickett, J. A., et al. (2002). Control of witchweed Striga hermonthica by intercropping with Desmodium spp., and the mechanism defined as allelopathic. J. Chem. Ecol. 28, 1871–1885. doi: 10.1023/A:1020525521180
Khan, Z. R., Midega, C. A. O., Bruce, T. J. A., Hooper, A. M., and Pickett, J. A. (2010). Exploiting phytochemicals for developing a “push-pull” crop protection strategy for cereal farmers in Africa. J. Exp. Bot. 61, 4185–4196. doi: 10.1093/jxb/erq229
Khan, Z. R., Midega, C. A. O., Wadhams, L. J., Pickett, J. A., and Mumuni, A. (2007). Evaluation of Napier grass (Pennisetum purpureum) varieties for use as trap plants for the management of African stemborer (Busseola fusca) in a push-pull strategy. Entomol. Exp. Appl. 124, 201–211. doi: 10.1111/j.1570-7458.2007.00569.x
Khan, Z. R., Pickett, J. A., van Den Berg, J., Wadhams, L. J., and Woodcock, C. M. (2000). Exploiting chemical ecology and species diversity: stem borer and striga control for maize and sorghum in Africa. Pest Manag. Sci. 962, 957–962. doi: 10.1002/1526-4998(200011)56:11<957::aid-ps236>3.0.co;2-t
Kigathi, R. N., Unsicker, S. B., Reichelt, M., Kesselmeier, J., Gershenzon, J., and Weisser, W. W. (2009). Emission of volatile organic compounds after herbivory from Trifolium pratense (L.) under laboratory and field conditions. J. Chem. Ecol. 35, 1335–1348. doi: 10.1007/s10886-009-9716-3
Leopold, E. J. (1990). Selective hydroboration of a 1,3,7-triene: Homogeraniol. Org. Synth. 64, 164–170. doi: 10.15227/orgsyn.064.0164
Letourneau, D. K., Ambrecht, I., Rivera, B. S., Lerma, J. M., Carmona, E. J., Daza, M. C., et al. (2011). Does plant diversity benefit agroecosystems? A synthetic review. Ecol. Appl. 21, 9–21. doi: 10.1890/09-2026.1
Magara, H. J. O., Mutyambai, D. M., Charles, M. A. O., Otieno, S. A., Nyaga, T. M., Niassy, S., et al. (2020). Responses of stemborer Chilo partellus to volatiles emitted by maize landraces exposed to signal grass (Brachiaria brizantha). J. Plant Interact. 15, 345–357. doi: 10.1080/17429145.2020.1827056
Meza, F. C., Roberts, J. M., Sobhy, I. S., Okumu, F. O., Tripet, F., and Bruce, T. J. A. (2020). Behavioural and electrophysiological responses of female Anopheles gambiae mosquitoes to volatiles from a mango bait. J. Chem. Ecol. 46, 387–396. doi: 10.1007/s10886-020-01172-8
Midega, C. A. O., Bruce, T. J. A., Pickett, J. A., Pittchar, J. O., Murage, A., and Khan, Z. R. (2015). Climate-adapted companion cropping increases agricultural productivity in East Africa. F. Crop. Res. 180, 118–125. doi: 10.1016/j.fcr.2015.05.022
Midega, C. A. O., Khan, Z. R., Van den Berg, J., Ogol, C. K. P. O., Bruce, T. J., and Pickett, J. A. (2009). Non-target effects of the “push-pull” habitat management strategy: Parasitoid activity and soil fauna abundance. Crop. Prot. 28, 1045–1051. doi: 10.1016/j.cropro.2009.08.005
Midega, C. A. O., Pittchar, J. O., Pickett, J. A., Hailu, G. W., and Khan, Z. R. (2018). A climate-adapted push-pull system effectively controls fall armyworm, Spodoptera frugiperda (J E Smith), in maize in East Africa. Crop. Prot. 105, 10–15. doi: 10.1016/j.cropro.2017.11.003
Mihm, J. A. (1983). Efficient mass-rearing and infestation techniques to screen for host plant resistance to fall armyworm, Spodoptera frugiperda. Cent. Int. Mejor. Maiz y Trigo CIMMYT, El-Batan, Mex 1983:16.
Mutyambai, D. M., Bruce, T. J. A., Midega, C. A. O., Woodcock, C. M., Caulfield, J. C., Van Den Berg, J., et al. (2015). Responses of parasitoids to volatiles induced by Chilo partellus oviposition on teosinte, a wild ancestor of maize. J. Chem. Ecol. 41, 323–329. doi: 10.1007/s10886-015-0570-1
NIST (2017). NIST mass spectral database for NIST/EPA/NIH and mass spectral search program (version 2.3). Natl. Inst. Stand. Technol. NIST 2017, 1–73.
Pickett, J. A. (1990). “Gas chromatography-mass spectrometry in insect pheromone identification: three extreme case histories,” in Chromatography and isolation of insect hormones and pheromones, eds A. R. McCaffery and I. D. Wilson (New York, NY: Plenum Publishing Corporation), 299–309. doi: 10.1007/978-1-4684-8062-7
Pinto-Zevallos, D. M., Strapasson, P., and Zarbin, P. H. G. (2016). Herbivore-induced volatile organic compounds emitted by maize: Electrophysiological responses in Spodoptera frugiperda females. Phytochem. Lett. 16, 70–74. doi: 10.1016/j.phytol.2016.03.005
Pretty, J., and Bharucha, Z. P. (2014). Sustainable intensification in agricultural systems. Ann. Bot. 114, 1571–1596. doi: 10.1093/aob/mcu205
Quispe, R., Mazón, M., and Rodríguez-Berrío, A. (2017). Do refuge plants favour natural pest control in maize crops? Insects 8:71. doi: 10.3390/insects8030071
Richter, A., Schaff, C., Zhang, Z., Lipka, A. E., Tian, F., Köllner, T. G., et al. (2016). Characterization of biosynthetic pathways for the production of the volatile homoterpenes DMNT and TMTT in Zea mays. Plant Cell 28, 2651–2665. doi: 10.1105/tpc.15.00919
Rwomushana, I., Bateman, M., Beale, T., Beseh, P., Cameron, K., Chiluba, M., et al. (2018). Fall Armyworm: Impacts and implications for Africa. Evidence Note Update. CABI 2018, 1–51.
SAS Institute Inc (2008). Statistical Analysis System, Version 9.2 [computer programme]. Sas Institute Inc: Cary, NC.
Scheidegger, L., Niassy, S., Midega, C., Chiriboga, X., Delabays, N., Lefort, F., et al. (2021). The role of Desmodium intortum, Brachiaria sp. and Phaseolus vulgaris in the management of fall armyworm Spodoptera frugiperda (J. E. Smith) in maize cropping systems in Africa. Pest Manag. Sci. 77, 2350–2357. doi: 10.1002/ps.6261
Sisay, B., Simiyu, J., Mendesil, E., Likhayo, P., Ayalew, G., Mohamed, S., et al. (2019). Fall armyworm, Spodoptera frugiperda infestations in East Africa: Assessment of damage and parasitism. Insects 10:195. doi: 10.3390/insects10070195
Skelton, A. C., Cameron, M. M., Pickett, J. A., and Birkett, M. A. (2010). Identification of neryl formate as the airborne aggregation pheromone for the American house dust mite and the European house dust mite (Acari: Epidermoptidae). J. Med. Entomol. 47, 798–804. doi: 10.1603/ME09295
Snoeren, T. A. L., Mumm, R., Poelman, E. H., Yang, Y., Pichersky, E., and Dicke, M. (2010). The herbivore-induced plant volatile methyl salicylate negatively affects attraction of the parasitoid Diadegma semiclausum. J. Chem. Ecol. 36, 479–489. doi: 10.1007/s10886-010-9787-1
Sobhy, I. S., Caulfield, J. C., Pickett, J. A., and Birkett, M. A. (2020). Sensing the danger signals: cis -Jasmone reduces aphid performance on potato and modulates the magnitude of released volatiles. Front. Ecol. Evol. 7:499. doi: 10.3389/fevo.2019.00499
Sobhy, I. S., Woodcock, C. M., Powers, S. J., Caulfield, J. C., Pickett, J. A., and Birkett, M. A. (2017). cis-Jasmone elicits aphid-induced stress signalling in potatoes. J. Chem. Ecol. 43, 39–42. doi: 10.1007/s10886-016-0805-9
Stokstad, E. (2017). New crop pest takes Africa at lightning speed. Science 356, 473–474. doi: 10.1126/science.356.6337.473
Tamiru, A., Bruce, T. J. A., Woodcock, C. M., Birkett, M. A., Midega, C. A. O., Pickett, J. A., et al. (2015). Chemical cues modulating electrophysiological and behavioural responses in the parasitic wasp Cotesia sesamiae. Can. J. Zool. 93, 281–287. doi: 10.1139/cjz-2014-0266
Tamiru, A., Bruce, T. J. A., Woodcock, C. M., Caulfield, J. C., Midega, C. A. O., Ogol, C. K. P. O., et al. (2011). Maize landraces recruit egg and larval parasitoids in response to egg deposition by a herbivore. Ecol. Lett. 14, 1075–1083. doi: 10.1111/j.1461-0248.2011.01674.x
Tamiru, A., Paliwal, R., Manthi, S. J., Odeny, D. A., Midega, C. A. O., Khan, Z. R., et al. (2020). Genome wide association analysis of a stemborer egg induced “call − for − help” defence trait in maize. Sci. Rep. 10:11205. doi: 10.1038/s41598-020-68075-2
Turlings, T. C. J., and Erb, M. (2018). Tritrophic interactions mediated by herbivore-induced plant volatiles: Mechanisms, ecological relevance, and application potential. Annu. Rev. Entomol. 63, 433–452. doi: 10.1146/annurev-ento-020117-043507
Turlings, T. C. J., Tumlinson, J. H., Heath, R. R., Proveaux, A. T., and Doolittle, R. E. (1991). Isolation and identification of allelochemicals that attract the larval parasitoid, Cotesia marginiventris (Cresson), to the microhabitat of one of its hosts. J. Chem. Ecol. 17, 2235–2251. doi: 10.1007/BF00988004
Turlings, T. C. J., Tumlinson, J. H., Lewis, W. J., and Vet, L. E. M. (1989). Beneficial arthropod behavior mediated by airborne semiochemicals. VIII. Learning of host-related odors induced by a brief contact experience with host by-products in Cotesia marginiventris (Cresson), a generalist larval parasitoid. J. Insect Behav. 2, 217–225. doi: 10.1007/bf01053293
Turlings, T. C. J., and Wäckers, F. (2004). “Recruitment of predators and parasitoids by herbivore-injured plants,” in Advances in Insect Chemical Ecology, eds R. T. Carde and J. G. Millar (Cambridge, MA: Cambridge University Press), 21–75. doi: 10.1017/cbo9780511542664.003
Wadhams, L. J. (1990). “The use of coupled gas chromatography: electrophysiological techniques in the identification of insect pheromones,” in Chromatography and isolation of insect hormones and pheromones, eds A. R. McCaffery and I. D. Wilson (New York, NY: Plenum Press), 289–298. doi: 10.1007/978-1-4684-8062-7_28
Keywords: volatiles, tritrophic interactions, Push-Pull, maize, herbivory, companion crops, chemical ecology, behavioral response
Citation: Sobhy IS, Tamiru A, Chiriboga Morales X, Nyagol D, Cheruiyot D, Chidawanyika F, Subramanian S, Midega CAO, Bruce TJA and Khan ZR (2022) Bioactive Volatiles From Push-Pull Companion Crops Repel Fall Armyworm and Attract Its Parasitoids. Front. Ecol. Evol. 10:883020. doi: 10.3389/fevo.2022.883020
Received: 24 February 2022; Accepted: 22 March 2022;
Published: 11 April 2022.
Edited by:
Maria Carolina Blassioli Moraes, Brazilian Agricultural Research Corporation (EMBRAPA), BrazilReviewed by:
Cesar Rodriguez-Saona, Rutgers, The State University of New Jersey, United StatesDiego Martins Magalhães, University of São Paulo, Brazil
Copyright © 2022 Sobhy, Tamiru, Chiriboga Morales, Nyagol, Cheruiyot, Chidawanyika, Subramanian, Midega, Bruce and Khan. This is an open-access article distributed under the terms of the Creative Commons Attribution License (CC BY). The use, distribution or reproduction in other forums is permitted, provided the original author(s) and the copyright owner(s) are credited and that the original publication in this journal is cited, in accordance with accepted academic practice. No use, distribution or reproduction is permitted which does not comply with these terms.
*Correspondence: Amanuel Tamiru, YXRhbWlydUBpY2lwZS5vcmc=; Toby J. A. Bruce, dC5qLmEuYnJ1Y2VAa2VlbGUuYWMudWs=
†These authors have contributed equally to this work