- 1Centre for Ecology and Conservation in Cornwall, College of Life and Environmental Sciences, University of Exeter, Exeter, United Kingdom
- 2Department of Psychology, Centre for Research in Animal Behaviour, College of Life and Environmental Sciences, University of Exeter, Exeter, United Kingdom
- 3Department of Biology, Pacific University, Forest Grove, OR, United States
Rapid population growth and the urbanization of modern environments are markedly increasing human-wildlife conflict. Wild animals in urban landscapes can benefit from exploiting human resources, but are also exposed to increased risk of human-caused injury, which should favor the ability to perceive and respond to human cues. Although it is well known that domesticated animals use human cues that may indicate threats, less is known about wild animals living in urban environments. Herring gulls (Larus argentatus) in urban landscapes have adapted kleptoparasitic behaviors to obtain human food, often resulting in negative interactions with humans. Here we quantified both the behavioral and physiological responses of free-living urban herring gulls to human shouting. We presented urban gulls with a fake human food item and played back recordings of either a man shouting, a natural stressor (i.e., conspecific alarm call), or a neutral stimulus (i.e., robin song). We recorded behavioral responses and used non-invasive infrared thermography to measure eye-region surface temperature changes associated with the avian physiological stress response. We found that gulls exposed to shouting and to conspecific alarm calls showed similar changes in behavior (indicating high levels of vigilance) and eye-region surface temperature (indicating physiological stress). Both responses were significantly stronger than the responses to robin song. Additionally, the behavioral and physiological responses were positively correlated across individuals. Our results demonstrate that urban-dwelling gulls respond to human shouting and conspecific alarm calls in a similar way, and suggest that infrared thermography is a viable technique to monitor stress responses in free-living birds.
Introduction
As human populations and degree of urbanization are growing worldwide, the frequency of encounters between humans and wildlife is increasing dramatically (Soulsbury and White, 2015). While human-wildlife interactions can be detrimental for certain species (e.g., crested anoles Anolis cristatellus; Kolbe et al., 2016; bobcats Lynx rufus; Ordeñana et al., 2010; rufous-tailed hummingbirds Amazilia tzacatl; Biamonte et al., 2011), others appear to prosper in urban environments (e.g., brown rats Rattus norvegicus; Traweger et al., 2006; feral pigeons Columbia livia; Aronson et al., 2014; house sparrows Passer domesticus, common starlings Sturnus vulgaris; Chace and Walsh, 2006; Aronson et al., 2014). Such urban-dwelling species often benefit from easy access to human food (Oro et al., 2013; Cox and Gaston, 2018), and have adapted behavioral traits to facilitate their use of anthropogenic resources, such as foraging innovations to extract food (e.g., Aplin et al., 2013; Audet et al., 2016; Ducatez et al., 2017), opportunistic diets and kleptoparasitism (i.e., stealing food from others) (Murray et al., 2015; Ducatez et al., 2017; Méndez et al., 2020), and increased nocturnality (Gaynor et al., 2018). However, species living in urban habitats also face increased disturbance levels due to anthropogenic activities (e.g., pedestrian and vehicular traffic) (Lowry et al., 2013; Shannon et al., 2014; Loss et al., 2015), which can significantly reduce their chances of survival and reproductive success (Frid and Dill, 2002; Price, 2008). Thus, human-dominated landscapes should select for individuals that perceive and respond to human cues, to reduce the risks associated with anthropogenic activities (Møller, 2008; Audet et al., 2016; Goumas et al., 2020c). So far, relatively little attention has been paid to how urban wildlife respond to, and interact, with humans (Goumas et al., 2020c). Such understanding would contribute greatly to the mitigation of human-wildlife conflict (Johnson and Munshi-South, 2017).
Wild animals appear to rely on multiple cues to inform their response to potential human threats. For instance, work on both corvids (American crows Corvus brachyrhynchos and Western jackdaws Corvus monedula) and pigeons suggests that wild animals may recognize individual humans and distinguish dangerous people, who were previously involved in trapping efforts, from non-threatening people (Marzluff et al., 2010, 2012; Belguermi et al., 2011; Davidson et al., 2015). Additionally, wild animals use gaze direction as a cue to assess human threat (known as “gaze aversion”) (reviewed in Goumas et al., 2020c): numerous wild species have been shown to take longer to approach food (Carter et al., 2008; Goumas et al., 2019), or to take flight sooner (Bateman and Fleming, 2011; Clucas et al., 2013), when human gaze is directed at them. Thus, urban-living animals can gauge the risk posed by humans by using visual cues. There is also some evidence that cues in other sensory modalities, such as auditory cues, are used as indicators of human risk (e.g., Lynch et al., 2015). For example, wild animals may discriminate between familiar and unfamiliar human voices (McComb et al., 2014; Dutour et al., 2021). Yet, the majority of research on animals’ perception of human auditory cues to assess human risk has been conducted on captive and domesticated animals (Adachi et al., 2007; Lampe and Andre, 2012; Proops and McComb, 2012; Wascher et al., 2012; Saito and Shinozuka, 2013; Ratcliffe et al., 2014; Leroux et al., 2018), while little is known about wild urban-living animals.
Herring gulls (Larus argentatus) represent an interesting model for investigating how human auditory cues are perceived by urban wildlife. In the last five decades, gull numbers in urban environments have grown exponentially in the United Kingdom (Balmer et al., 2014; Eaton et al., 2015; Ross et al., 2016), resulting in an increased conflict with human populations in urban areas (Rock, 2005). Herring gulls are opportunistic feeders and they have adapted kleptoparasitic behaviors to obtain human food (Rock, 2005; Spencer et al., 2017). Herring gulls use human behavioral cues to assess risk in a feeding context, such as human gaze direction when approaching an anthropogenic food source (Goumas et al., 2019). Additionally, previous work has found that nesting herring gulls display similar anti-predator behavioral responses to playbacks of humans reading a book passage as they do to conspecific or heterospecific gull alarm calls (MacLean and Bonter, 2013). The latter study tested gulls that were incubating eggs, at a site where humans used to take eggs and adult birds for food. Our study complements this previous work by addressing whether gulls respond similarly in an urban setting when foraging rather than nesting.
It is likely that experiments on wild animals attracted to human food in urban environments are biased toward testing only the boldest, least neophobic individuals (Dammhahn and Almeling, 2012; Daniels et al., 2019). While such individuals may appear to behaviorally ignore human threats such as approach or shouting, such threats could still cause physiological responses that are less easily perceived by human observers. To address this issue, we quantified both behavioral and physiological responses of urban herring gulls to human threat. The quantification of changes in external body temperature represents a promising approach to determine wild animals’ physiological responses to stressors (Gormally and Romero, 2020). Acute stress responses in endotherms are characterized by sympathetically mediated vasoconstriction that redirects blood from the periphery to the core of the body, referred to as stress-induced hypothermia or SIH (Briese and Cabanac, 1991). This physiological response results in a temporary temperature drop at the body surface, which can be recorded remotely and non-invasively using infrared thermal cameras (Stewart et al., 2005; McCafferty, 2013). Numerous studies have successfully measured stress-induced temperature changes in the area around the eye (i.e., the periophthalmic ring) of birds, as demonstrated in passerines caught from the wild (Jerem et al., 2015, 2018, 2019; Andreasson et al., 2020; Robertson et al., 2020, 2021), gamebirds (Knoch et al., 2022), and poultry (Edgar et al., 2013; Herborn et al., 2015, 2018; Weimer et al., 2020). However, infrared thermography (IRT) has not been implemented yet in the context of human-wildlife interactions in the field.
Our study quantified the behavioral and physiological responses to human shouting of wild urban herring gulls feeding on human food, by combining IRT with behavioral observations. We presented wild herring gulls with a (fake) food source and, once they started pecking at it, exposed them to the playback of one of three types of auditory stimulus: a man shouting, a conspecific alarm call (a natural stressor), or a robin (Erithacus rubecula) song (a neutral stimulus). We recorded the gulls’ eye-region temperature changes using IRT, as well as their behavioral responses to the playback stimulus. We investigated whether urban herring gulls perceive human shouting to be threatening to a similar extent as the risk signaled by conspecific alarm call. We first predicted that individuals exposed to the man shouting would show a drop in eye-region surface temperature similar to the temperature drop observed in individuals exposed to the conspecific alarm call. In contrast, we predicted that individuals exposed to neutral robin song would show minimal or no temperature drop. Secondly, we predicted that the man shouting and conspecific alarm call treatments would elicit an antipredator behavioral response (e.g., vigilance or flight) of similarly high intensity, while robin song would elicit no such anti-predator behavior. Finally, we tested whether gulls’ behavioral and physiological responses would correlate, such that individuals with larger temperature drops would also show more pronounced vigilance or flight behaviors.
Materials and methods
Test subjects
We studied wild populations of herring gulls in several coastal towns in Cornwall, United Kingdom, between 7:00 and 18:20, from 27 April 2021 to 14 July 2021, during the breeding season. Herring gulls found foraging in Cornish towns are likely to be habituated to anthropogenic activities (Goumas et al., 2019, 2020a,b). The individuals we tested were all unmarked. However, because herring gulls can be territorial during the breeding season (Drury and Smith, 1968), we minimized the chance of pseudoreplication by avoiding repeated experimental trials in the same location when possible. We considered two gulls to be from different locations when they were foraging and nesting at least 150 m apart. Whilst individual gulls return to the same productive feeding location (Davis, 1975), they can travel significant distances when they forage (Fuirst et al., 2018). We therefore could not rule out that the same individuals visited multiple locations. We included “location” as a random effect in our statistical models (see below) to control for any potential pseudoreplication in our analyses. A total of 96 observational trials were conducted in 44 locations while 48 thermal measurements were conducted in 29 locations (see Supplementary Tables 1, 2 for details on treatment sample size per location, and see map of the test locations).1 For locations with large numbers of gulls and high anthropogenic activity (e.g., beach, lake), we occasionally tested gulls that were foraging within 150 m from each other, and thus identified them as being from the same location. In this case, the experimenter visually tracked the individual’s movement after testing it, to minimize the chance of repeating the next experiment on the same individual.
Stimulus selection
Playbacks included three types of auditory treatments: (1) man shouting, (2) herring gull alarm call, and (3) robin song. Each treatment included five exemplars (i.e., recordings) from different individuals to reduce pseudoreplication and increase generalizability. The five human voices were recorded from five British male volunteers who had no previous experience with the gulls tested. The five volunteers were asked to shout “No! It’s my food! It’s my pasty!”, simulating the potential auditory experience of a herring gull stealing a pasty (a traditional British baked pastry filled with meat and vegetables). The herring gull alarm-call treatment consisted of “yeow calls” used to alert conspecifics to the presence of a threat (Shah et al., 2015). All the recordings of herring gulls and robins were obtained from the https://Xeno-Canto.org website (herring gull recordings: XC397600, XC444037, XC511610, XC483105; robin recordings: XC627492, XC630182, XC631729, XC632323, XC639916), with the exception of one herring gull track recorded by the experimenter in St Austell, United Kingdom, as we could not obtain enough suitable herring gull recordings from the Xeno-Canto library.
We edited recordings, normalized their amplitude, and removed background noise using Audacity 3.0.4 (D.M. Mazzoni, Canada).2 Each recording was standardized to a duration of 30 s. We standardized the duty cycle of the recordings such that: (1) for the herring gull and robin treatments, each recording consisted of two loops of a 10-s sound clip followed by 5 s of silence; and (2) for the man shouting, each recording consisted of six loops of a 2-s sound clip followed by 3 s of silence. All the recordings were mono and normalized to 60 dB peak amplitude (dB A weighting; 20 μPA reference value) to imitate what gulls would experience under natural conditions (Shah et al., 2015). We measured the amplitude of recordings in the field using a sound level meter (Decibel X, SkyPaw Co., Ltd.).
Experimental protocol
The fake food item was a plush pasty (55 × 135 × 185 mm) filled with rocks to prevent the gulls from flying off with it. We chose this food item because pasties are commonly consumed in Cornwall and gulls are likely to have had previous experience with them. The experimenter located a gull that was not engaged in agonistic interactions. The experimenter then approached to approximately 10 m from the gull, pretended to eat the pasty and then threw it approximately 3 m into the air once to several times, to attract the gull’s attention. It took 36.14 ± 25.83 s (mean ± sd; n = 46) for the experimenter to attract the gull’s attention in trials where the gull approached and pecked at the pasty. Once the gull showed an interest in the pasty (either staring at the pasty and/or walking/flying toward the experimenter holding the pasty), the experimenter placed the pasty on the ground. The pasty was placed two meters away from a FLIR thermal camera (FLIR T530, f = 17 mm, resolution = 320 × 240 (76,800 pixels), thermal sensitivity = < 40 mK, 24° @ + 30°C, image frequency = 30 Hz) and a FoxPro speaker (FoxPro Fury 2, FOXPRO Inc., Lewistown, PA, United States), which were both camouflaged in a toy pram. The thermal camera was positioned 2 m away from the pasty such that gulls approaching the food were always within the camera’s field of view, while maximizing the resolution of the thermal images. Immediately after placing the pasty on the ground, the experimenter walked away to a distance of at least 10 m (Goumas et al., 2020a).
Once the focal gull started pecking at the pasty, it was left undisturbed for 15 s to control for the individual’s arousal levels, as the gull might be stressed to approach and/or excited to locate the pasty, which can affect its physiological response (Moe et al., 2012; Travain et al., 2016). We also used this period to measure the pre-treatment “baseline” body surface temperature of the gull with the thermal camera (Jerem et al., 2015). Then, the experimenter played a 30-s recording of one of the three auditory treatments (man shouting, conspecific alarm call, or robin song) to imitate what gulls would experience under natural conditions (Shah et al., 2015). The orders of each treatment and of each stimulus exemplar were assigned randomly by assigning each treatment to a number between 1 and 3 and each stimulus exemplar to a number between 1 and 5, and using a generator of random numbers (Randomizer version 3.9.8, Google Commerce Ltd.). The surface temperature of the focal gull, focused on the eye region, was recorded during those 30 s with the thermal camera, while the behavioral response of the gull to the playback was recorded with a camcorder (Panasonic HC-V770, f = 29.5 mm) positioned at least 10 m away from the pasty and either held by an observer or placed on the ground. The experimental trial was terminated at the end of the 30-s playback, or earlier if people approached the experimental set up or if the focal gull moved beyond an estimated radius of 10 m from the pasty. We also aborted the experimental trial if a loud anthropogenic noise (e.g., traffic noise, dog barking) elicited a change in the focal gull’s behavior or if another gull interacted with the focal gull. We decided to limit our experiments to 30 s, as previous work on birds suggests that temperature values drop within 10–20 s after exposure to a stressor (Jerem et al., 2015, 2019; Andreasson et al., 2020; Knoch et al., 2022). After 30 s, the experimenter moved to a different location, or waited 30 min before conducting another trial in the same location to reduce any potential carryover effects (see Supplementary Tables 1, 2). For consecutive trials conducted in the same location, the experimenter visually tracked the individual’s movement after testing it, to minimize the chance of repeating the next experiment on the same individual, and randomly chose a different stimulus for the next experimental trial.
For each experimental trial, we measured several additional variables that may have affected the behavioral and physiological responses of the focal gull. We recorded atmospheric temperature and relative humidity using a weather app (The Weather Channel, TWC Product and Technology, LLC). After each experimental trial we also measured the reflected temperature, corresponding to the radiation originating from other objects that reflect off the body surface of the focal gull, by placing aluminum foil (with a known emissivity of 1) at the pasty location and measuring its mean temperature with the thermal camera.
Thermal video processing
We recorded 48 experimental trials with the thermal camera. For each experimental trial, we extracted the maximum temperature of the eye region (area covering the eye and the exposed skin around the eye; Figure 1) for each second, using FLIR Tools software version 6.4 (FLIR Systems, Inc., 2015), and following the methods detailed by Jerem et al. (2015). We used the maximum temperature of the eye region since thermal imaging may underestimate temperature measurements due to motion blur, making the maximum temperature the most accurate measurement (Jerem et al., 2015; Tabh et al., 2021). We delineated the eye region using the box measurement tool (Figure 1). We adjusted the parameters of the thermal video to environmental conditions by entering the following variables into the software: atmospheric temperature, reflected temperature, humidity, distance of thermal camera from focal gull, and emissivity (set at 0.95). We extracted the maximum eye-region temperature (Teye) for each frame (frame rate = 30 fps). We removed frames where the eye region was blurry or not visible (due to motion), or when the focal gull was blinking (characterized by a spike of Teye for 3 frames or less). We also removed frames when the head was not perpendicular to the lens of the thermal camera, since head orientation has been shown to influence surface temperature estimates of the eye region (Herborn et al., 2015; Playà-Montmany and Tattersall, 2021; Tabh et al., 2021). Using R version 4.0.0 (R Core Team, 2020), we selected the highest and most accurate temperature measurements using a “peak search algorithm” developed by Jerem et al. (2015). We performed linear interpolation to estimate temperature values when no measurement was extracted (Jerem et al., 2015, 2019; Knoch et al., 2022), and averaged measurements for each second using the R package zoo (Zeileis and Grothendieck, 2005). We obtained one measurement per second, starting from 15 s before stimulus presentation to the end of the 30-s auditory treatment. The 15 s before stimulus presentation were used for illustrative purposes only and were not included in our statistical models (see Figure 2)—whilst only the single highest value of Teye of these 15 s was used to determine the pre-treatment temperature of Teye (hereafter referred to as “baseline temperature”). We set 0 s as the time when the stimulus was played. After completion of the thermal video processing, the data for each experimental trial consisted of: (1) a pre-stimulus period of 15 s with maximum eye-region temperature (Teye) for each second (used for illustrative purposes only), and one baseline temperature; and (2) a post-stimulus period of 30 s with maximum eye-region temperature (Teye) for each second. We removed from our dataset all trials that had fewer than 5 s of Teye post-stimulus.
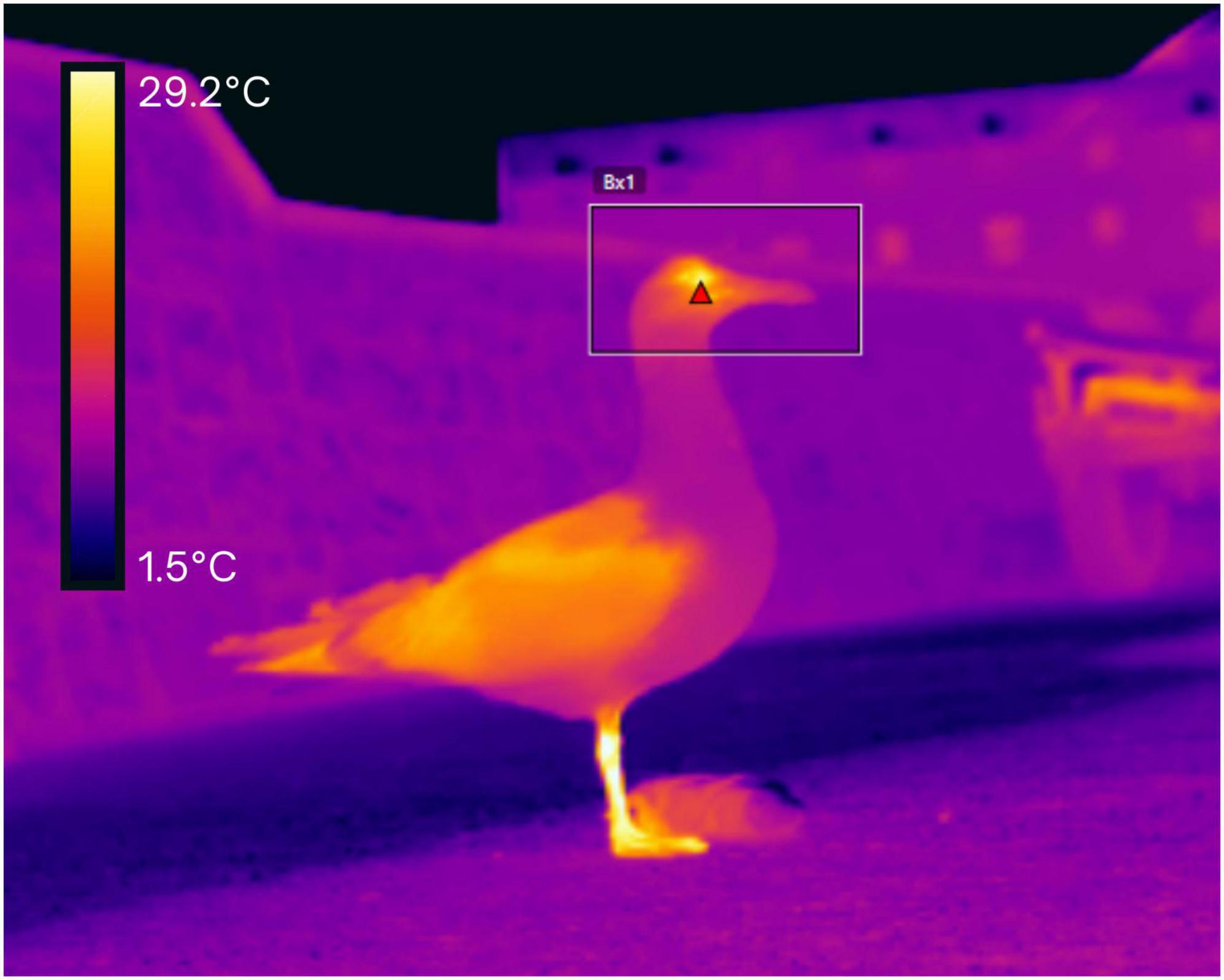
Figure 1. Thermal image of a herring gull after the experimenter played back the conspecific alarm call stimulus. The red triangle pinpoints the maximum eye-region temperature (Teye). The periophthalmic ring is the warmest region (27.6°C here) of the head (delineated by the box). On the ground, next to the gull, is the fake food item (plush pasty) used to lure the gull within view of the thermal camera (the plush pasty is placed 2 m away from the thermal camera). The temperature scale is indicated in the top-left corner.
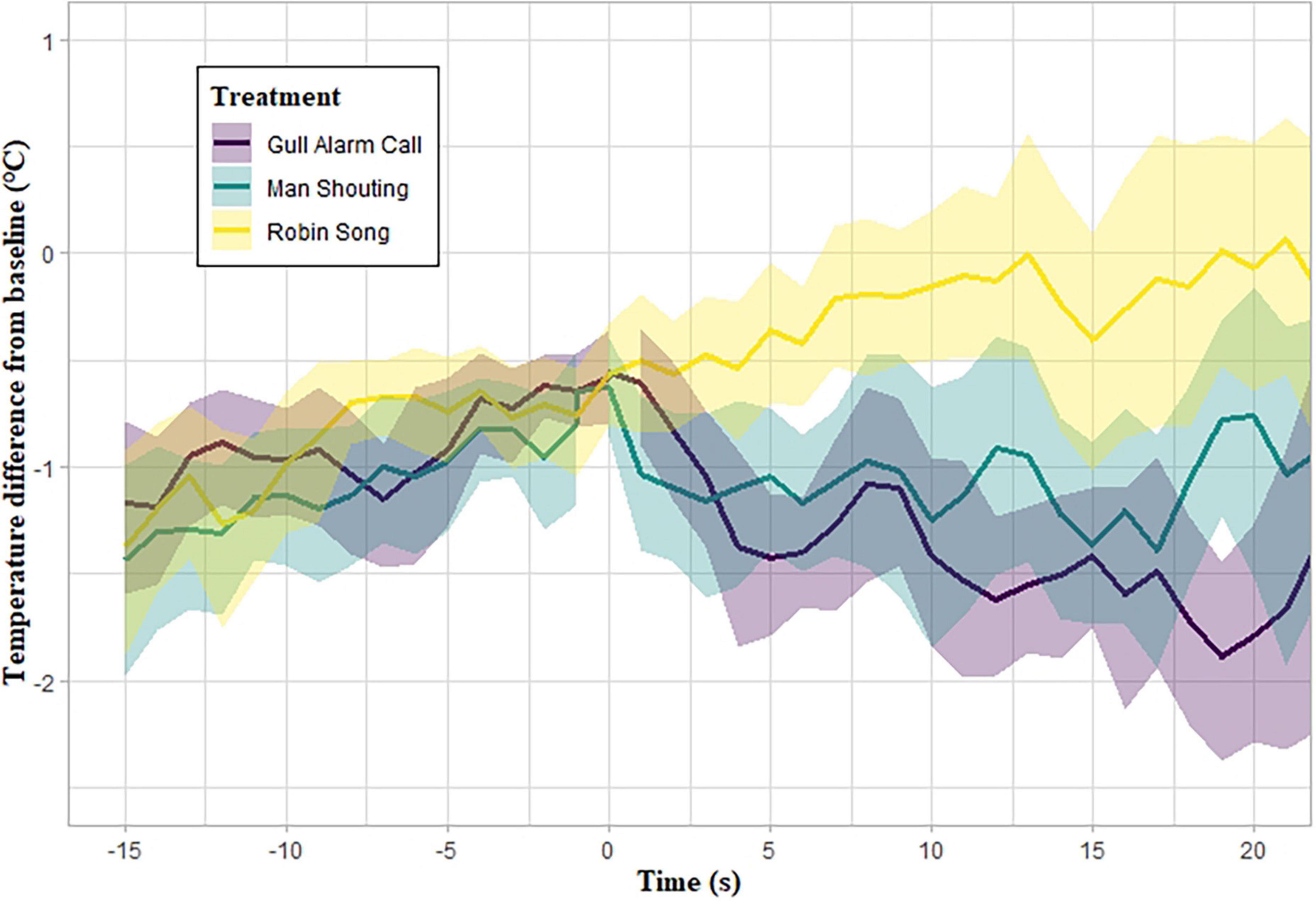
Figure 2. Mean difference from baseline in maximum eye-region temperature (Teye) of herring gulls in the three treatments [conspecific alarm call (n = 17 gulls) in purple, man shouting (n = 15) in blue, and robin song (n = 16) in yellow] with their 95% confidence intervals (ribbons). The auditory treatment was played at 0 s for a duration of 30 s. Missing temperature values were replaced using linear interpolation.
Behavioral observations
We recorded 96 experimental trials with the camcorder, and 47 thermal measurements were within this set of trials. For each experimental trial, we graded the maximum behavioral response of the focal gull on a scale from 0 to 4 (Table 1). Each number described a set of behaviors (based on Tinbergen, 1961; MacLean and Bonter, 2013; Shah et al., 2015), with higher values representing more pronounced behavioral responses to the stimulus. Gulls display alertness behaviors by stretching their neck to scan for threats (Tinbergen, 1961; MacLean and Bonter, 2013). We described gulls as “walking away” when they moved beyond an estimated radius of 10 m from the pasty, and within 20 s from the start of the stimulus playback to control for individuals that lost interest in the pasty at the end of the trial. We differentiated gulls that walked away as a vigilant behavioral response to the stimulus from gulls that walked away because they lost interest in the pasty (i.e., gulls that did not show any vigilant behavioral response as described in Table 1). In the latter case, the gull’s departure was not considered to be an escape and was scored as 0.
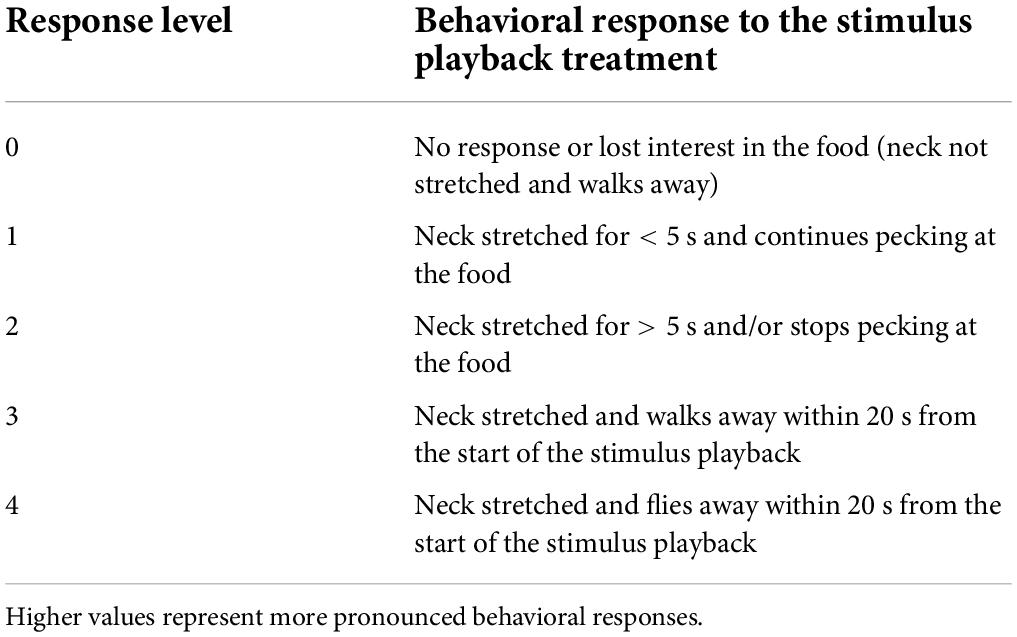
Table 1. Ethogram used to classify the behavioral responses of foraging herring gulls to the auditory stimulus treatments.
Statistical analysis
We conducted all analyses in R version 4.0.0 (R Core Team, 2020). To analyze variation in unstandardized post-stimulus Teye among treatments, we fitted a linear mixed model (LMM) with the package lme4 (Bates et al., 2015). As fixed effects we included treatment (conspecific alarm call, robin song, or man shouting), time (one value for each second of the post-stimulus period), and the interaction of these two terms. We included baseline temperature as a fixed effect, since individuals with higher baseline temperature may show larger temperature changes (Herborn et al., 2015). We included behavioral response (ranging from 0 to 4) as a fixed effect to test whether it was correlated with changes in eye region temperature. As random effects we included location and playback stimulus exemplar ID to control for pseudoreplication. Date was also initially included as a random effect, since seasonal changes between April and July when trials were conducted may affect the birds’ physiological response to acute stressors (Romero, 2002). However, date was subsequently removed as it did not significantly improve the model fit to the data (15.2% of residual variance explained by date; log-likelihood ratio test, X2 = 0.88, d.f. = 1, p = 0.348). We used the package car (Fox and Weisberg, 2019) to generate diagnostic plots of the residuals, which revealed that residuals were normally distributed and that their variance was similar across all predicted values of the response variable, demonstrating homoscedasticity. We compared models with and without the fixed effects by performing log-likelihood ratio tests, to determine which model best explained the variation in the data. To test whether variation in Teye differed significantly among treatments, we used the package lmerTest. We re-ordered the levels of treatment (conspecific alarm call, robin song, and man shouting) using the relevel command and compared each treatment combination, as each releveled model used a different treatment as the reference. To visualize the mean differences in Teye between playback treatments, we used the package ggplot2 (Wickham, 2016), and added non-parametric bootstrapped 95% confidence intervals for the data for each treatment using mean.cl.boot in the package Hmisc (Harrell, 2014).
To investigate whether gulls’ behavioral responses (scored on an ordinal scale) differed between treatments, we fitted a cumulative link mixed model (CLMM) in the package ordinal (Christensen, 2019). We included playback treatment as a fixed effect, and location and stimulus exemplar ID as random effects. We first included date as a covariate in the model, but it was subsequently removed as it did not significantly improve the model fit to the data (< 0.01% of residual variance explained by date; X2 < 0.01, d.f. = 1, p > 0.999). We were not able to generate diagnostic plots of the residuals since CLMM does not generate residuals. We compared models with and without the fixed effects by performing log-likelihood ratio tests, to determine which model best explained the variation in the data. We used the relevel command and compared each treatment combination. Finally, we used ggplot2 (Wickham, 2016) to visualize the differences in behavioral responses among treatments, and added standard errors using the package plotrix (Lemon, 2006).
To control for any potential case of pseudoreplication, we repeated all analysis but kept only one trial per location that was chosen randomly from trials that had both a physiological and behavioral response recorded. We removed n = 19 trials from the same location (resulting in n = 29) for analyses of the gulls’ physiological response, while we removed n = 52 trials from the same location (resulting in n = 44) for the analyses of the gulls’ behavioral response. We obtained qualitatively similar results, although statistical significance disappeared in some cases, which is likely due to the relatively large reduction in sample size (see Supplementary material).
Results
Do changes in maximum eye-region temperature (Teye) differ among playback treatments?
Maximum eye-region temperature showed an average drop of 2.00 ± 0.69°C (mean ± sd; n = 15) from pre-stimulus levels for gulls exposed to the man shouting, and 2.17 ± 0.76°C (n = 17) for gulls exposed to the herring gull alarm call, but only 1.11 ± 0.74°C (n = 16) for individuals exposed to the robin song. There was a significant effect of auditory treatment on changes in Teye (LMM; interaction term Time x Treatment: X2 = 17.13, d.f. = 2, p < 0.001). Individuals exposed to the man shouting showed a similar drop in Teye compared to individuals exposed to the conspecific alarm call (slope estimate ± se = 0.05 ± 0.28°C, t14 = 0.174, p = 0.864; Figure 2), but this temperature drop was significantly larger than that observed in individuals exposed to the robin song (slope estimate ± se = 0.75 ± 0.28°C, t13 = 2.722, p = 0.018; Figure 2). Similarly, individuals exposed to conspecific alarm calls displayed a significantly larger drop in Teye than individuals exposed to robin song (slope estimate ± se = 0.70 ± 0.28°C, t14 = 2.544, p = 0.023; Figure 2).
Contrary to our prediction, individuals with higher baseline temperature showed a smaller temperature drop than individuals with lower baseline temperatures (LMM; slope estimate ± se = 0.88 ± 0.02°C, X2 = 821.24, d.f. = 1, p < 0.001). Additionally, we found a significant effect of location on temperature changes (52.2% of residual variance explained by location; X2 = 335.96, d.f. = 1, p < 0.001), indicating that gulls from the same location responded more similarly to each other than to gulls from different locations. Finally, stimulus exemplar ID also had a significant effect on temperature change (12.1% of residual variance explained by stimulus exemplar ID; X2 = 60.45, d.f. = 1, p < 0.001).
To assess the robustness of our results, we performed the statistical analysis on the same dataset but without interpolating the temperature measurements, by leaving gaps when no measurement could be extracted from the thermal recordings and not averaging for each second. We obtained similar results to those from our original dataset (see Supplementary Table 3), with the exception of one effect estimate: the larger temperature drop in individuals exposed to the conspecific alarm call compared to those exposed to the robin song was no longer significant (slope estimate ± se = 0.64 ± 0.33, t15 = 1.928, p = 0.079). These variable results might be due to the small sample sizes.
Do gull behavioral responses differ among playback treatments?
We found a significant effect of playback treatment on the behavioral responses of gulls (d.f. = 2, p = 0.002, no X2-value generated for cumulative link models): individuals exposed to the man shouting showed a behavioral response of similar intensity to individuals exposed to the conspecific alarm call (slope estimate ± se = 0.58 ± 0.31 intensity score, z = –1.91, p = 0.056), but a significantly stronger response than individuals exposed to the robin song (slope estimate ± se = –1.26 ± 0.30 intensity score, z = –4.25, p < 0.001; Figure 3). The conspecific alarm call also elicited a stronger behavioral response than the robin song (slope estimate ± se = –0.6786 ± 0.32 intensity score, z = –2.153, p = 0.031; Figure 3). Thus, the man shouting and conspecific alarm call elicited more pronounced antipredator behavioral responses than the robin song.
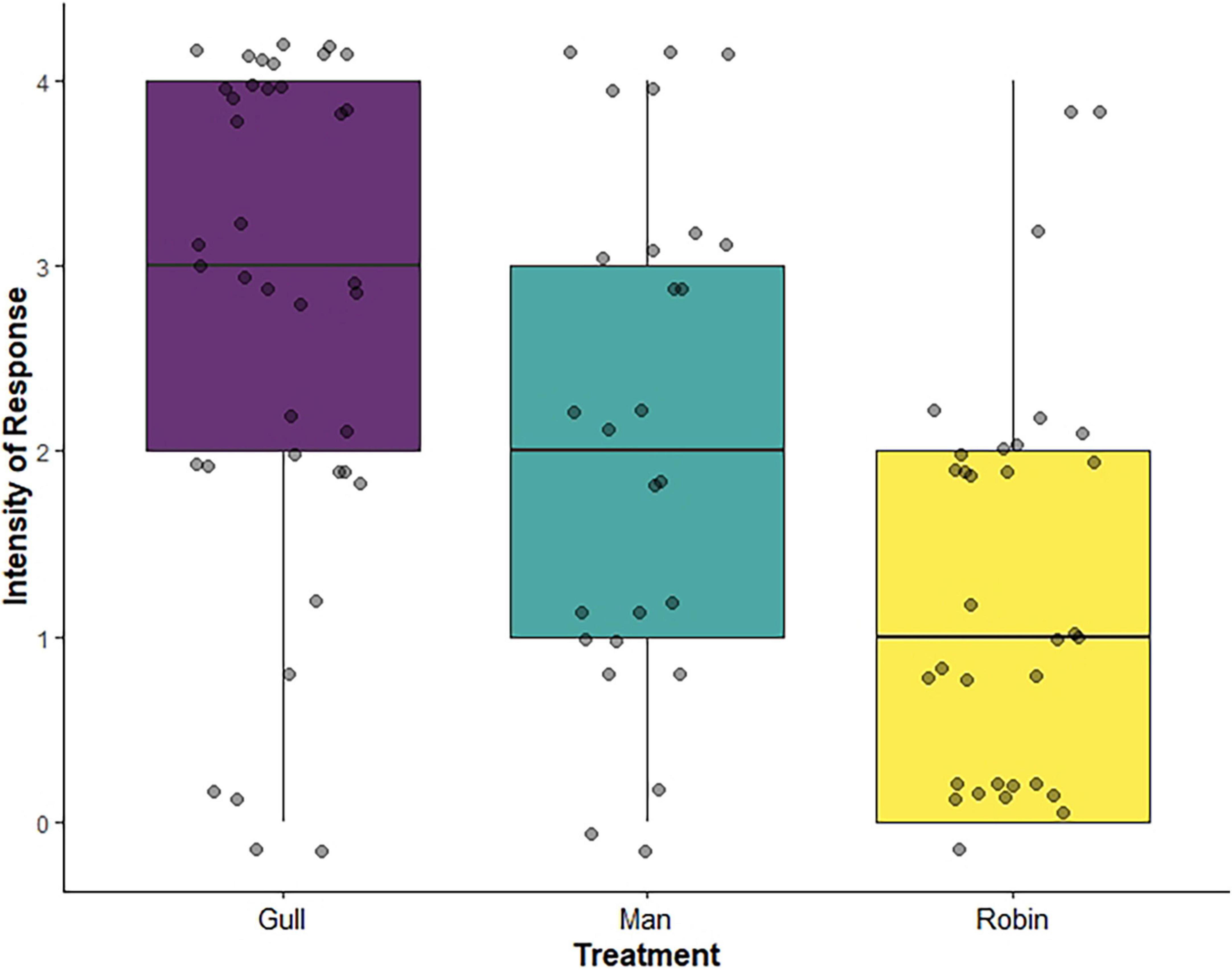
Figure 3. Intensity of the behavioral responses of herring gulls to the three playback treatments [conspecific alarm call (n = 38) in purple, man shouting (n = 26) in blue, and robin song (n = 32) in yellow]. Each point represents the behavioral response of one gull, jittered to reduce overlap, while the boxplots show the median, upper and lower quartiles and range of values. An intensity of 0 corresponds to no behavioral response of the gull to the playback treatment, while an intensity of 3 or 4 corresponds to a strong behavioral response (i.e., walking or flying away from the pasty). This figure is for illustrative purposes only and does not capture the random effect structure of the statistical analysis.
We found a significant effect of location on the behavioral response of gulls (> 99% of residual variance explained by location; X2 = 4.33, d.f. = 1, p = 0.037), but no significant effect of stimulus exemplar ID (<0.01% of residual variance explained by exemplar ID; X2 < 0.01, d.f. = 1, p = 0.998).
Are gulls’ temperature changes correlated with their behavioral response scores?
The behavioral response score of a gull was significantly and positively associated with its eye-region temperature change (LMM; slope estimate ± se = 0.28 ± 0.05, X2 = 30.51, d.f. = 1, p < 0.001): individuals with more pronounced behavioral responses to the playback treatments showed larger temperature drops (Figure 4). We obtained these results from the LMM of the mean temperature drop in the gull’s eye region in response to the treatments (section “Do changes in maximum eye-region temperature (Teye) differ among playback 362 treatments?”).
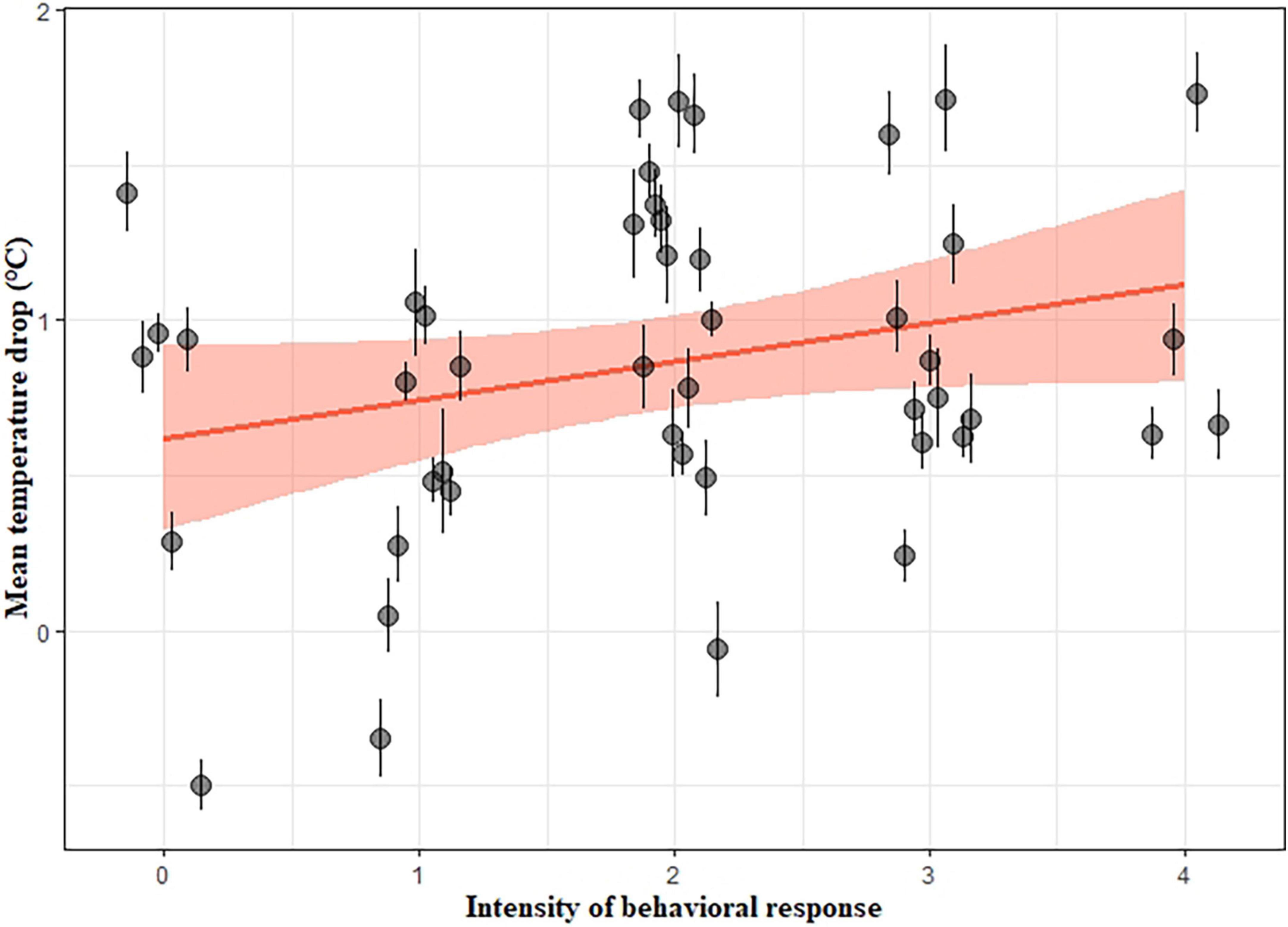
Figure 4. Mean temperature drop in the gull’s eye region in response to the playback treatments, as a function of the intensity of the behavioral response, showing the regression line (in red) and the 95% confidence intervals (red ribbon). Each point represents the mean temperature difference from baseline as a function of the behavioral response score for one gull, with its standard errors. Temperature difference from baseline was calculated by subtracting baseline temperature from post-stimulus temperature (Tdiff = Tpost–stimulus – Tbaseline). We selected the largest temperature difference from baseline to represent the amplitude of the temperature drop. Temperature difference from baseline was used for illustrative purposes only and was not included in the analyses.
To confirm the robustness of the correlation, we repeated our statistical analysis on the same dataset but without interpolating the temperature measurements, and obtained similar results to those from our original dataset (Supplementary Table 3).
Discussion
In this study, we quantified the physiological and behavioral responses of foraging wild urban herring gulls to human shouting, by combining infrared thermography with behavioral observations. Consistent with predictions, we found that gulls exposed to the man shouting and conspecific alarm call showed similar drops in eye-region surface temperature, whilst gulls exposed to the robin song showed a minimal temperature drop. In addition, gulls exposed to the man shouting and conspecific alarm call showed vigilance and fleeing responses of similarly high intensity, and these were more pronounced than the behavioral responses of gulls exposed to the robin song. Finally, we found a positive correlation between individuals’ behavioral and physiological responses, such that gulls with more pronounced behavioral responses to the playback treatments displayed larger temperature drops. Our results suggest that the temperature drops observed in gulls responding to the man shouting and conspecific alarm call reflected stressor-induced physiological responses, and that the sound of a man shouting was perceived by the wild urban herring gulls we tested to be as stressful as a conspecific alarm call. This is consistent with previous work that has found that the playback of a human voice reading a book passage elicited the same intensity of antipredator behavioral response as the playback of a conspecific alarm call in nesting herring gulls (MacLean and Bonter, 2013). Taken together, these findings suggest that gulls might find various types of human sound threatening, but future studies need to test this directly by measuring responses to a range of different human sound treatments.
Different people can represent different levels of threat, by having reactions toward gulls ranging from positive to negative (Goumas et al., 2020c). For example, some people may directly feed urban-dwelling animals (e.g., Marion et al., 2008), while others may persecute them (e.g., Harris, 1984). Previous work has demonstrated that wild Australian magpies (Gymnorhina tibicen dorsalis) and captive carrion crows (Corvus corone corone) are able to discriminate between voices of familiar and unfamiliar humans (Wascher et al., 2012; Dutour et al., 2021). Thus, some bird species may discriminate between threatening and non-threatening humans using acoustic cues. Gulls may also be capable of discriminating between human voices, since we observed a significant effect of stimulus exemplar ID on the gulls’ physiological response. This suggests that some human voices were more threatening than others, and that gulls may have perceived and responded to the voice’s nuances differently. However, the design of our study did not allow us to test whether gulls can distinguish threatening from non-threatening humans based on acoustic cues. We also used different recording techniques for our treatments, which might introduce a small experimental confound. Future work should therefore investigate whether gulls show different physiological and behavioral responses to human voices conveying different levels of threat (e.g., aggressive compared with neutral voice tone), or to human voices associated with different past events (e.g., threatening compared with rewarding or neutral events). This would provide valuable insights into the extent to which urban-dwelling animals modulate their responses toward humans depending on the risk they pose.
To our knowledge, our study is the first to use infrared thermography to measure stressor-induced physiological responses of wild, freely moving birds, and to implement this method to understand urban animals’ perception of human auditory cues. Our experimental design consisted of attracting a focal gull to a fake human food item (pasty), which allowed us to control for the context of the human-gull interaction (foraging) and to standardize the distance between gull and thermal camera. However, the gull might have been too excited or scared to approach the pasty, which may have resulted in a physiological response before we started the playback of the sound stimuli (Moe et al., 2012; Travain et al., 2016). This potential lowering of the eye-region surface temperature before exposure to the auditory treatments may have limited further cooling as a response to our playback treatments (Jerem et al., 2015). We attempted to mitigate this effect by waiting for 15 s after the gull’s approach to play the auditory stimulus. This “refractory period” allowed the gull’s eye-region surface-temperature to recover from potential prior physiological responses, as suggested by the upward trajectory of the eye region temperature before the onset of the playback stimulus in Figure 2. We were subsequently able to successfully quantify and distinguish between physiological responses to potentially threatening stimuli (i.e., man shouting and conspecific alarm call) and neutral stimuli (i.e., robin song). Our findings therefore demonstrate that infrared thermography is a promising non-invasive method to quantify stressor-induced physiological responses of wild, freely moving birds in field settings.
Individual gulls’ behavioral and physiological responses were positively correlated, suggesting that they both reflect the activation of the sympathetic-adrenal-medullary (SAM) system (Briese and Cabanac, 1991; Sapolsky, 1992; Sapolsky et al., 2000). The perception of the playback treatments may activate the gull’s SAM system, inducing the production of catecholamines and the mobilization of energy to produce a behavioral response (Sapolsky, 1992). During this response, a sympathetically mediated vasoconstriction redirects the blood from the periphery to the core of the body, causing a temperature drop in the eye region (Briese and Cabanac, 1991; Sapolsky et al., 2000). Previous avian studies using thermal imaging to measure stressor-induced temperature changes performed behavioral observations to validate the surface temperature changes observed (Edgar et al., 2013; Herborn et al., 2015, 2018; Weimer et al., 2020). However, these studies did not assess the individual-level relationship between the intensity of the behavioral response and the magnitude of the physiological response. Thus, our findings highlight the relationship between the stressor-induced temperature changes and the antipredator behavioral response to stressors, and demonstrate that stressor-induced temperature changes in the eye-region are a reliable physiological marker for assessing changes in the state of vigilance in birds.
Playing different stimulus exemplars for each treatment had a significant effect on the gulls’ physiological responses, while it did not have a significant effect on the gulls’ behavioral responses. This indicates that solely measuring the behavioral response of the gulls would have underestimated the effect of stimulus exemplar ID. Furthermore, gulls that showed the strongest behavioral responses (i.e., walking away and/or flying away from the experimental set-up when the playback started) displayed the strongest physiological responses, suggesting that individuals that stayed the longest near the experimental set-up were likely to be individuals that were the least physiologically disturbed by the playback treatments. These findings therefore indicate that behavioral experiments on urban-dwelling animals may be biased toward testing only the boldest individuals and highlight the importance of also measuring the stressor-induced physiological responses to obtain a more representative sample of the study population.
In summary, our results indicate that gulls respond to human shouting in a similar way to how they respond to natural stressors, suggesting that gulls use auditory cues (potentially alongside visual or other cues) to assess risk from humans. Gulls might find human sounds threatening, and having a better understanding of their response to human cues could help developing strategies to prevent negative human-wildlife interactions. Our study also demonstrates that infrared thermography can provide valuable insights into the mechanisms used by urban wildlife while interacting with humans, and advocates for its application to measure stressor-induced physiological changes in wild, freely moving animals. Future research is necessary to understand how wild animals integrate human cues from multiple modalities, and should focus on the acoustic cues that wild animals in urban landscapes use to discriminate between threatening and non-threatening humans. Such work would shed light on how wildlife perceive humans and would contribute to the mitigation of human-wildlife conflict by understanding what cues cause wildlife to interact with, or avoid, humans.
Data availability statement
The raw data supporting the conclusions of this article will be made available by the authors, without undue reservation.
Ethics statement
The animal study was reviewed and approved by the University of Exeter CLES (College of Life and Environmental Sciences) Penryn Ethics Committee (ref: eCORN003799).
Author contributions
JD and NB conceived and designed the study with input from CT and TF. JD and SR conducted the experiments. JD and TF analyzed the data. JD wrote the original draft, while NB, CT, and TF reviewed and edited the draft. NB supervised the study. All authors have read and approved the final manuscript.
Funding
NB was funded by the Royal Society Dorothy Hodgkin Research Fellowship (DH140080).
Acknowledgments
We would like to thank Joah Madden for lending us the thermal imaging camera and instructing us how to use it. We thank Ewan Fenelon, Scarlett Weston, Maria Giulia Checchi, Ollie King, Tiana Thomas, and Rupli Moores-Mason for their valuable help collecting data in the field. We extend our thanks to the Project C-Gull team, including (but not exclusive to) Rosie Berkin, Madeleine Goumas, Emma Inzani and, Laura Kelley for useful discussion.
Conflict of interest
The authors declare that the research was conducted in the absence of any commercial or financial relationships that could be construed as a potential conflict of interest.
Publisher’s note
All claims expressed in this article are solely those of the authors and do not necessarily represent those of their affiliated organizations, or those of the publisher, the editors and the reviewers. Any product that may be evaluated in this article, or claim that may be made by its manufacturer, is not guaranteed or endorsed by the publisher.
Supplementary material
The Supplementary Material for this article can be found online at: https://www.frontiersin.org/articles/10.3389/fevo.2022.891985/full#supplementary-material
Footnotes
- ^ https://www.google.com/maps/d/edit?mid=1KvGnWXsaHdXr4LaFXZVFh_FHYXyp0AR8&usp=sharing
- ^ https://www.audacityteam.org/
References
Adachi, I., Kuwahata, H., and Fujita, K. (2007). Dogs recall their owner’s face upon hearing the owner’s voice. Anim. Cogn. 10, 17–21. doi: 10.1007/s10071-006-0025-8
Andreasson, F., Nord, A., and Nilsson, J. (2020). Body temperature responses of Great Tits Parus major to handling in the cold. Ibis 162, 836–844. doi: 10.1111/ibi.12737
Aplin, L. M., Sheldon, B. C., and Morand-Ferron, J. (2013). Milk bottles revisited: Social learning and individual variation in the blue tit. Cyanistes caeruleus. Anim. Behav. 85, 1225–1232. doi: 10.1016/j.anbehav.2013.03.009
Aronson, M. F. J., La Sorte, F. A., Nilon, C. H., Katti, M., Goddard, M. A., Lepczyk, C. A., et al. (2014). A global analysis of the impacts of urbanization on bird and plant diversity reveals key anthropogenic drivers. Proc. R. Soc. B 281:20133330. doi: 10.1098/rspb.2013.3330
Audet, J. N., Ducatez, S. D., and Lefebvre, L. (2016). The town bird and the country bird: Problem solving and immunocompetence vary with urbanization. Behav. Ecol. 27, 637–644. doi: 10.1093/beheco/arv201
Balmer, D. E., Gillings, S., Caffrey, B., Swann, B., Downie, I., and Fuller, R. (2014). Bird Atlas 2007–11: the breeding and wintering birds of Britain and Ireland. New York, NY: HarperCollins Publishers
Bateman, P. W., and Fleming, P. A. (2011). Who are you looking at? Hadeda ibises use direction of gaze, head orientation and approach speed in their risk assessment of a potential predator. J. Zool. 285, 316–323. doi: 10.1111/j.1469-7998.2011.00846.x
Bates, D., Mächler, M., Bolker, B., and Walker, S. (2015). Fitting Linear Mixed-Effects Models Using lme4. J. Stat. Softw. 67, 1–48. doi: 10.18637/jss.v067.i01
Belguermi, A., Bovet, D., Pascal, A., Prévot-Julliard, A. C., Jalme, M. S., Rat-Fischer, L., et al. (2011). Pigeons discriminate between human feeders. Anim. Cogn. 14, 909–914. doi: 10.1007/s10071-011-0420-7
Biamonte, E., Sandoval, L., Chacón, E., and Barrantes, G. (2011). Effect of urbanization on the avifauna in a tropical metropolitan area. Landsc. Ecol. 26, 183–194. doi: 10.1007/s10980-010-9564-0
Briese, E., and Cabanac, M. (1991). Stress hyperthermia: Physiological arguments that it is a fever. Physiol. Behav. 49, 1153–1157. doi: 10.1016/0031-9384(91)90343-m
Carter, J., Lyons, N. J., Cole, H. L., and Goldsmith, A. R. (2008). Subtle cues of predation risk: Starlings respond to a predator’s direction of eye-gaze. Proc. R. Soc. B Biol. Sci. 275, 1709–1715. doi: 10.1098/rspb.2008.0095
Chace, J. F., and Walsh, J. J. (2006). Urban effects on native avifauna: A review. Landsc. Urban Plan. 74, 46–69. doi: 10.1016/j.landurbplan.2004.08.007
Christensen, R. H. B. (2019). ordinal—Regression Models for Ordinal Data. R package version 2019.12-10. Available online at: https://CRAN.R-project.org/package=ordinal (accessed August 20, 2021).
Clucas, B., Marzluff, J. M., Mackovjak, D., and Palmquist, I. (2013). Do American crows pay attention to human gaze and facial expressions? Ethology 119, 296–302. doi: 10.1111/eth.12064
Cox, D. T. C., and Gaston, K. J. (2018). Human–nature interactions and the consequences and drivers of provisioning wildlife. Proc. R. Soc. B 373:20170092. doi: 10.1098/rstb.2017.0092
Dammhahn, M., and Almeling, L. (2012). Is risk taking during foraging a personality trait? A field test for cross-context consistency in boldness. Anim. Behav. 84, 1131–1139. doi: 10.1016/j.anbehav.2012.08.014
Daniels, S. E., Fanelli, R. E., Gilbert, A., and Benson-Amram, S. (2019). Behavioral flexibility of a generalist carnivore. Anim. Cogn. 22, 387–396. doi: 10.1007/s10071-019-01252-7
Davidson, G. L., Clayton, N. S., and Thornton, A. (2015). Wild jackdaws, Corvus monedula, recognize individual humans and may respond to gaze direction with defensive behaviour. Anim. Behav. 108, 17–24. doi: 10.1016/j.anbehav.2015.07.010
Davis, J. W. F. (1975). Specialization in Feeding Location by Herring Gulls. J. Anim. Ecol. 44, 795–804. doi: 10.2307/3719
Drury, W. H. Jr., and Smith, W. J. (1968). Defense of feeding areas by adult herring gulls and intrusion by young. Evolution 22, 193–201. doi: 10.1111/j.1558-5646.1968.tb03462.x
Ducatez, S., Audet, J. N., Rodriguez, J. R., Kayello, L., and Lefebvre, L. (2017). Innovativeness and the effects of urbanization on risk-taking behaviors in wild Barbados birds. Anim. Cogn. 20, 33–42. doi: 10.1007/s10071-016-1007-0
Dutour, M., Walsh, S. L., Speechley, E. M., and Ridley, A. R. (2021). Female Western Australian magpies discriminate between familiar and unfamiliar human voices. Ethology 127, 979–985. doi: 10.1111/eth.13218
Eaton, M., Aebischer, N., Brown, A., Hearn, R., Lock, L., Musgrove, A., et al. (2015). Birds of Conservation Concern 4: The population status of birds in the UK. Channel Islands and Isle of Man. Br. Birds 108, 708–746.
Edgar, J., Nicol, C., Pugh, C., and Paul, E. (2013). Surface temperature changes in response to handling in domestic chickens. Physiol. Behav. 119, 195–200. doi: 10.1016/j.physbeh.2013.06.020
FLIR Systems, Inc. (2015). Available online at: https://www.flir.co.uk/
Fox, J., and Weisberg, S. (2019). An R Companion to Applied Regression, 3rd Edn. Thousand Oaks, CA: Sage.
Frid, A., and Dill, L. (2002). Human-caused disturbance stimuli as a form of predation risk. Conserv. Ecol. 6, 11–26. doi: 10.5751/ES-00404-060111
Fuirst, M., Veit, R. R., Hahn, M., Dheilley, N., and Thorne, L. H. (2018). Effects of urbanization on the foraging ecology and microbiota of the generalist seabird Larus argentatus. PLoS One 13:e0209200. doi: 10.1371/journal.pone.0209200
Gaynor, K. M., Hojnowski, C. E., Carter, N. H., and Brashares, J. S. (2018). The influence of human disturbance on wildlife nocturnality. Science 360, 1232–1235. doi: 10.1126/science.aar7121
Gormally, B. M. G., and Romero, M. L. (2020). What are you actually measuring? A review of techniques that integrate the stress response on distinct time-scales. Funct. Ecol. 34, 2030–2044. doi: 10.1111/1365-2435.13648
Goumas, M., Lee, V. E., Kelley, L. A., and Thornton, A. (2020c). The role of animal cognition in human-wildlife interactions. Front. Psychol. 11:589978. doi: 10.3389/fpsyg.2020.589978
Goumas, M., Boogert, N. J., and Kelley, L. A. (2020a). Urban herring gulls use human behavioural cues to locate food. R. Soc. Open Sci. 7:1959. doi: 10.1098/rsos.191959
Goumas, M., Collins, T. R., Fordham, L., Kelley, L. A., and Boogert, N. J. (2020b). Herring gull aversion to gaze in urban and rural human settlements. Anim. Behav. 168, 83–88. doi: 10.1016/j.anbehav.2020.08.008
Goumas, M., Burns, I., Kelley, L. A., and Boogert, N. J. (2019). Herring gulls respond to human gaze direction. Biol. Lett. 15:20190405. doi: 10.1098/rsbl.2019.0405
Harrell, F. E. (2014). Hmisc: A package of miscellaneous R functions. Available online at: https://hbiostat.org/R/Hmisc/
Harris, S. (1984). Ecology of Urban Badgers Meles meles: Distribution in Britain and Habitat Selection, Persecution, Food and Damage in the City of Bristol. Biol. Conserv. 28, 349–375. doi: 10.1016/0006-3207(84)90041-7
Herborn, K. A., Graves, J. L., Jerem, P., Evans, N. P., Nager, R., McCafferty, D. J., et al. (2015). Skin temperature reveals the intensity of acute stress. Physiol. Behav. 152, 225–230. doi: 10.1016/j.physbeh.2015.09.032
Herborn, K. A., Jerem, P., Nager, R., McKeegan, D. E. F., and McCafferty, D. J. (2018). Surface temperature elevated by chronic and intermittent stress. Physiol. Behav. 191, 47–55. doi: 10.1016/j.physbeh.2018.04.004
Jerem, P., Herborn, K., McCafferty, D., McKeegan, D., and Nager, R. (2015). Thermal Imaging to Study Stress Non-invasively in Unrestrained Birds. J. Vis. Exp. 105:e53184. doi: 10.3791/53184
Jerem, P., Jenni-Eiermann, S., Herborn, K., McKeegan, D., McCafferty, D. J., and Nager, R. (2018). Eye region surface temperature reflects both energy reserves and circulating glucocorticoids in a wild bird. Sci. Rep. 8:1907. doi: 10.1038/s41598-018-20240-4
Jerem, P., Jenni-Eiermann, S., McKeegan, D., McCafferty, D. J., and Nager, R. G. (2019). Eye region surface temperature dynamics during acute stress relate to baseline glucocorticoids independently of environmental conditions. Physiol. Behav. 210:112627. doi: 10.1016/j.physbeh.2019.112627
Johnson, M. T. J., and Munshi-South, J. (2017). Evolution of life in urban environments. Science 358:eaam8327. doi: 10.1126/science.aam8327
Knoch, S., Whiteside, M. A., Madden, J. R., Rose, P. E., and Fawcett, T. W. (2022). Hot-headedpeckers: Thermographic changes during aggression among juvenile pheasants(Phasianus colchicus). Phil. Trans. R. Soc. B. 377:20200442. doi: 10.1098/rstb.2020.0442
Kolbe, J. J., VanMiddlesworth, P., Battles, A. C., Stroud, J. T., Buffum, B., Forman, R. T. T., et al. (2016). Determinants of spread in an urban landscape by an introduced lizard. Landsc. Ecol. 31, 1795–1813. doi: 10.1007/s10980-016-0362-1
Lampe, J. F., and Andre, J. (2012). Cross-modal recognition of human individuals in domestic horses (Equus caballus). Anim. Cogn. 15, 623–630. doi: 10.1007/s10071-012-0490-1
Leroux, M., Hetem, R. S., Hausberger, M., and Lemasson, A. (2018). Cheetahs discriminate familiar and unfamiliar human voices. Sci. Rep. 8:15516. doi: 10.1038/s41598-018-33971-1
Loss, S. R., Will, T., and Marra, P. P. (2015). Direct Mortality of Birds from Anthropogenic Causes. Annu. Rev. Ecol. Evol. Syst. 46, 99–120. doi: 10.1146/annurev-ecolsys-112414-054133
Lowry, H., Lill, A., and Wong, B. B. M. (2013). Behavioural responses of wildlife to urban environments. Biol. Rev. 88, 537–549. doi: 10.1111/brv.12012
Lynch, E., Northrup, J. M., McKenna, M. F., Anderson, C. R. Jr., Angeloni, L., and Wittemyer, G. (2015). Landscape and anthropogenic features influence the use of auditory vigilance by mule deer. Behav. Ecol. 26, 75–82. doi: 10.1093/beheco/aru158
MacLean, S. A., and Bonter, D. N. (2013). The sound of danger: Threat sensitivity to predator vocalizations, alarm calls, and novelty in gulls. PLoS One 8:e82384. doi: 10.1371/journal.pone.0082384
Marion, J. L., Dvorak, R. G., and Manning, R. E. (2008). Wildlife feeding in parks: Methods for monitoring the effectiveness of educational interventions and wildlife food attraction behaviors. Hum. Dimens. Wildl. 13, 429–442. doi: 10.1080/10871200802270158
Marzluff, J. M., Miyaoka, R., Minoshima, S., and Cross, D. J. (2012). Brain imaging reveals neuronal circuitry underlying the crow’s perception of human faces. PNAS 109, 15912–15917. doi: 10.1073/pnas.1206109109
Marzluff, J. M., Walls, J., Cornell, H. N., Withey, J. C., and Craig, D. P. (2010). Lasting recognition of threatening people by wild American crows. Anim. Behav. 79, 699–707. doi: 10.1016/j.anbehav.2009.12.022
McCafferty, D. J. (2013). Applications of thermal imaging in avian science. Ibis 155, 4–15. doi: 10.1111/ibi.12010
McComb, K., Shannon, G., Sayialel, K. N., and Moss, C. (2014). Elephants can determine ethnicity, gender, and age from acoustic cues in human voices. PNAS 111, 5433–5438. doi: 10.1073/pnas.1321543111
Méndez, A., Montalvo, T., Aymí, R., Carmona, M., Figuerola, J., and Navarro, J. (2020). Adapting to urban ecosystems: Unravelling the foraging ecology of an opportunistic predator living in cities. Urban Ecosyst. 23, 1117–1126. doi: 10.1007/s11252-020-00995-3
Moe, R. O., Stubsjoen, S. M., Bohlin, J., Flo, A., and Bakken, M. (2012). Peripheral temperature drop in response to anticipation and consumption of a signaled palatable reward in laying Hens (Gallus domesticus). Physiol. Behav. 106, 527–533. doi: 10.1016/j.physbeh.2012.03.032
Møller, A. P. (2008). Flight distance of urban birds, predation, and selection for urban life. Behav. Evol. Sociobiol. 63, 63–75. doi: 10.1007/s00265-008-0636-y
Murray, M., Cembrowski, A., Latham, A. D. M., Lukasik, V. M., Pruss, S., and St Clair, C. C. (2015). Greater consumption of protein-poor anthropogenic food by urban relative to rural coyotes increases diet breadth and potential for human–wildlife conflict. Ecography 38, 1235–1242. doi: 10.1111/ecog.01128
Ordeñana, M. A., Crooks, K. R., Boydston, E. E., Fisher, R. N., Lyren, L. M., Siudyla, S., et al. (2010). Effects of urbanization on carnivore species distribution and richness. J. Mammal. 91, 1322–1331. doi: 10.1644/09-MAMM-A-312.1
Oro, D., Genovart, M., Tavecchia, G., Fowler, M. S., and Martínez-Abraín, A. (2013). Ecological and evolutionary implications of food subsidies from humans. Ecol. Lett. 16, 1501–1514. doi: 10.1111/ele.12187
Playà-Montmany, N., and Tattersall, G. J. (2021). Spot size, distance, and emissivity errors in field applications of infrared thermography. Methods Ecol. Evol. 1–13. doi: 10.1111/2041-210X.13563
Price, M. (2008). “The impact of human disturbance on birds: A selective review,” in Too Close for Comfort: Conflicts in Human Wildlife Encounters, eds D. Lunney, A. Munn, and W. Meikle (Sydney: Royal Zoological Society of NSW), 163–196. doi: 10.7882/FS.2008.023
Proops, L., and McComb, K. (2012). Cross-modal individual recognition in domestic horses (Equus caballus) extends to familiar humans. PNAS 279, 3131–3138. doi: 10.1098/rspb.2012.0626
R Core Team (2020). R: A language and environment for statistical computing. Vienna, AUS: R Foundation for Statistical Computing.
Ratcliffe, V. F., McComb, K., and Reby, D. (2014). Cross-modal discrimination of human gender by domestic dogs. Anim. Behav. 91, 127–135. doi: 10.1016/j.anbehav.2014.03.009
Robertson, J. K., Mastromonaco, G. F., and Burness, G. (2020). Evidence that stress-induced changes in surface temperature serve a thermoregulatory function. J. Exp. Biol. 223:jeb213421. doi: 10.1242/jeb.213421
Robertson, J. K., Mastromonaco, G. F., and Burness, G. (2021). Thermal flexibility is a repeatable mechanism to cope with environmental stressors in a passerine bird. bioRxiv [preprint]. doi: 10.1101/2021.02.03.429657
Romero, L. M. (2002). Seasonal changes in plasma glucocorticoid concentrations in free-living vertebrates. Gen. Comp. Endocrinol. 128, 1–24. doi: 10.1016/S0016-6480(02)00064-3
Ross, K. E., Burton, N. H. K., Balmer, D. E., Humphreys, E. M., Austin, G. E., Goddard, B., et al. (2016). Urban Breeding Gull Surveys: A Review of Methods and Options for Survey Design. BTO. move111306404 0b00866ca8c7Research Report No. 680move111306404 Thetford: British Trust for Ornithology.
Saito, A., and Shinozuka, K. (2013). Vocal recognition of owners by domestic cats (Felis catus). Anim. Cogn. 16, 685–690. doi: 10.1007/s10071-013-0620-4
Sapolsky, R., Romero, L., and Munck, A. (2000). How Do Glucocorticoids Influence Stress Responses? Integrating Permissive, Suppressive, Stimulatory and Preparative Actions. Endocr. Rev. 21, 55–89. doi: 10.1210/edrv.21.1.0389
Sapolsky, R.M. (1992). “Neuroendocrinology of the stress-response,” In: Behavioural Endocrinology (eds). JB Becker, SM Breedlove, and D Crews (Cambridge: MIT Press), 28
Shah, S. S., Greig, E. I., MacLean, S. A., and Bonter, D. N. (2015). Risk-based alarm calling in a nonpasserine bird. Anim. Behav. 106, 129–136. doi: 10.1016/j.anbehav.2015.05.011
Shannon, G., Angeloni, L. M., Wittemyer, G., Fristrup, K. M., and Crooks, K. R. (2014). Road traffic noise modifies behaviour of a keystone species. Anim. Behav. 94, 135–141. doi: 10.1016/j.anbehav.2014.06.004
Soulsbury, C. D., and White, P. C. L. (2015). Human-wildlife interactions in urban areas: A review of conflicts, benefits and opportunities. Wildl. Res. 42, 541–553. doi: 10.1071/WR14229
Spencer, R., Russell, Y. I., Dickins, B. J. A., and Dickins, T. E. (2017). Kleptoparisitism in gulls Laridae at an urban and a coastal foraging environment: An assessment of ecological predictors. Bird Study 64, 12–19. doi: 10.1080/00063657.2016.1249821
Stewart, M., Webster, J. R., Schaefer, A. L., Cook, N. J., and Scott, S. L. (2005). Infrared thermography as a non-invasive tool to study animal welfare. Anim. Welf. 14, 319–325.
Tabh, J. K. R., Burness, G., Wearing, O. H., Tattersall, G. J., and Mastromonaco, G. F. (2021). Infrared thermography as a technique to measure physiological stress in birds: Body region and image angle matter. Physiol. Rep. 9:e14865. doi: 10.14814/phy2.14865
Tinbergen, N. (1961). The Herring Gull’s World: a Study of the Social Behaviour of Birds. New York, NY: Basic Books.
Travain, T., Colombo, E. S., Grandi, L. C., Heinzl, E., Pelosi, A., Previde, E. P., et al. (2016). How good is this food? A study on dogs’ emotional responses to a potentially pleasant event using infrared thermography. Physiol. Behav. 159, 80–87. doi: 10.1016/j.physbeh.2016.03.019
Traweger, D., Travnitzky, R., Moser, C., Walzer, C., and Bernatzky, G. (2006). Habitat preferences and distribution of the brown rat (Rattus norvegicus Berk.) in the city of Salzburg (Austria): Implications for an urban rat management. J. Pest. Sci. 79, 113–125.
Wascher, C. A., Szipl, G., Boeckle, M., and Wilkinson, A. (2012). You sound familiar: Carrion crows can differentiate between the calls of known and unknown heterospecifics. Anim. Cogn. 15, 1015–1019. doi: 10.1007/s10071-012-0508-8
Weimer, S. L., Wideman, R. F., Scanes, C. G., Mauromoustakos, A., Christensen, K. D., and Vizzier- Thaxton, Y. (2020). Broiler stress responses to light intensity, flooring type, and leg weakness as assessed by heterophil to lymphocyte ratios, serum corticosterone, infrared thermography, and latency to lie. Poult. Sci. 99, 3301–3311. doi: 10.1016/j.psj.2020.03.028
Wickham, H. (2016). ggplot2: Elegant Graphics for Data Analysis. New York, NY: Springer-Verlag. doi: 10.1007/978-3-319-24277-4
Keywords: alarm call, infrared thermography, herring gull, human voice, human - wildlife conflict
Citation: Di Giovanni J, Fawcett TW, Templeton CN, Raghav S and Boogert NJ (2022) Urban gulls show similar thermographic and behavioral responses to human shouting and conspecific alarm calls. Front. Ecol. Evol. 10:891985. doi: 10.3389/fevo.2022.891985
Received: 08 March 2022; Accepted: 11 August 2022;
Published: 20 September 2022.
Edited by:
Rebecca Rimbach, Duke University, United StatesReviewed by:
Ronald Sarno, Hofstra University, United StatesNina Jayne O’Hanlon, British Trust for Ornithology, United Kingdom
Copyright © 2022 Di Giovanni, Fawcett, Templeton, Raghav and Boogert. This is an open-access article distributed under the terms of the Creative Commons Attribution License (CC BY). The use, distribution or reproduction in other forums is permitted, provided the original author(s) and the copyright owner(s) are credited and that the original publication in this journal is cited, in accordance with accepted academic practice. No use, distribution or reproduction is permitted which does not comply with these terms.
*Correspondence: Julien Di Giovanni, amQ4MDhAZXhldGVyLmFjLnVr