- 1School of Biological Sciences, University of Nebraska-Lincoln, Lincoln, NE, United States
- 2Department of Psychology, West Chester University, West Chester, PA, United States
- 3Institute of Biological, Environmental and Rural Sciences, Aberystwyth University, Aberystwyth, United Kingdom
Wolf spiders within the genus Schizocosa have become a model system for exploring the form and function of multimodal communication. In terms of male signaling, much past research has focused on the role and importance of dynamic and static visual and substrate-borne vibratory communication. Studies on S. retrorsa, however, have found that female-male pairs were able to successfully mate in the absence of both visual and vibratory stimuli, suggesting a reduced or non-existent role of these signaling modalities in this species. Given these prior findings, it has been suggested that S. retrorsa males may utilize an additional signaling modality during courtship—air particle movement, often referred to as near-field sound—which they likely produce with rapid leg waving and receive using thin filiform sensory hairs called trichobothria. In this study, we tested the role of air-particle movement in mating success by conducting two independent sets of mating trials with randomly paired S. retrorsa females and males in the dark and on granite (i.e., without visual or vibratory signals) in two different signaling environments—(i) without (“No Noise”) and (ii) with (“Noise”) introduced air-particle movement intended to disrupt signaling in that modality. We also ran foraging trials in No Noise/Noise environments to explore the impact of our treatments on overall behavior. Across both mating experiments, our treatments significantly impacted mating success, with more mating in the No Noise signaling environments compared to the Noise environments. The rate of leg waving—a previously assumed visual dynamic movement that has also been shown to be able to produce air particle displacement—was higher in the No Noise than Noise environments. Across both treatments, males with higher rates of leg waving had higher mating success. In contrast to mating trials results, foraging success was not influenced by Noise. Our results indicate that artificially induced air particle movement disrupts successful mating and alters male courtship signaling but does not interfere with a female’s ability to receive and assess the rate of male leg waving.
Introduction
Animals communicate with each other for a multitude of reasons with displays that can often be received and processed by different sensory systems (Hebets, 2011; Higham and Hebets, 2013). Wild spotted hyenas (Crocuta crocuta), for example, receive visual signals from relaxed open mouths and head-bobbing behavior of conspecifics that leads to the initiation of play fights with playmates (Nolfo et al., 2021); Oriental magpie robins (Copsychus saularis) receive information from conspecifics about threats, submission, or distress through different acoustic signals (Manshor and Augustine Gawin, 2020); red-eyed treefrog males (Agalychnis callidryas) assess their opponents’ size and status through their vibratory signals, or tremulations (Caldwell et al., 2010); and ovulating female sea lamprey (Petromyzon marinus) are attracted to males by their pheromones, or olfactory signals (Teeter, 1980; Siefkes et al., 2005). Animal communication research has focused on many animal systems such as these where the signal is presumed to operate through a single sensory modality. There is also widespread recognition, however, that many animal displays incorporate signals in multiple sensory modalities, i.e., many animals engage in multimodal signaling (Rowe and Guilford, 1996; Partan and Marler, 1999; Hebets and Papaj, 2005; Hebets and McGinley, 2019). Multimodal communication displays that incorporate signals of various form can also be received and processed through multiple independent sensory systems (Hebets, 2011; Higham and Hebets, 2013; Halfwerk et al., 2019).
A first step in understanding the evolution and function of multimodal signaling is to identify potential sensory systems that might be involved (Halfwerk et al., 2019). To date, much research in this area has focused on presumed bimodal signaling systems, or on the reaction of receivers to signals received by two sensory systems. Male fowl (Gallus gallus), for example, have been studied for their use of movements (visual) and calls (acoustic—air borne sound) to attract a female’s attention (Smith et al., 2011). Estrildid finches (Family Estrildidae) are known to use singing (acoustic), dancing (visual), and color patterns (visual) as part of their multimodal courtship displays (Gomes et al., 2017); and many frog species incorporate combinations of visual, acoustic, substrate-borne vibratory (hereafter “vibratory”), and chemical components during signaling (reviewed in Starnberger et al., 2014). Similarly, within the multimodal courtship signaling of male wolf spiders, studies to date on the genus Schizocosa have focused predominantly on the female’s reactions to static and dynamic visual and vibratory signaling, and their potential interactions (Hebets, 2005; Hebets et al., 2011, 2021; Uetz et al., 2016; Hebets and McGinley, 2019; Choi and Hebets, 2021; Starrett et al., 2022).
The classification of signaler displays as unimodal vs. bimodal represents our current best attempts to understand signal form and function given our human sensory biases, our limitations in understanding the sensory physiology of focal taxa, and sometimes limitations to experimental designs. In all the previous examples of bimodal signaling, for example, signal detection might be informed by more than two different sensory systems. Dynamic movements that we presume to be visual signals, for example, may generate air particle movement that is detectable by receivers. Indeed, past discoveries of the involvement of previously unknown or underappreciated sensory systems have been arguably among the most exciting advances in animal communication research. We now know, for example, that big brown bats (Eptesicus fuscus) use ultrasonic calls not only to find food but that their calls also have unique properties to recognize individuals and thus function in communication (Masters et al., 1995). California ground squirrels (Spermophilus beecheyi) can add a thermal component to their tail flagging to defend themselves against infrared-sensitive rattlesnakes (Rundus et al., 2007). Several species of moths, including the Asian corn borer (Ostrinia furnacalis), produce ultrasonic frequencies of sound at a low level as a part of their courtship ritual so that their mates can hear them, but predators remain unaware (Nakano et al., 2009). More recent findings show that bottlenose dolphins (Tursiops truncatus) can respond to electric fields using the vibrissal crypts on their snouts (Hüttner et al., 2022) and orb-weaving spiders (Larinioides sclopetarius) can detect airborne sounds using their webs (Zhou et al., 2022). In many of these examples, the focal animals have sensory systems that differ markedly to those of humans, and it is only through an appreciation of these sensory abilities that signaling traits and interactions can be understood (Eakin, 1972; Budelmann, 1992).
There is huge variation in sensory systems across animal taxa and many of these systems are poorly understood. Within the arthropods, for example, we observe multiple spectral classes of photoreceptors in mantis shrimp (Order Stomatopoda) (Marshall, 1988; Marshall et al., 2007), the Johnston’s organ in insects (Order Diptera) (Johnston, 1855; Boekhoff-Falk, 2005; Gibson and Russell, 2006), pectines of scorpions (Order Scorpiones) (Brownell, 1988; Wolf, 2017), malleoli of solifugae (Order Solifugae) (Brownell and Farley, 1974), elongate sensory legs of amblypygids (Order Amblypygi) (Igelmund, 1987; Santer and Hebets, 2011) and enlarged anterior median eyes of jumping spiders (Order Araneae, Family Salticidae) (Land, 1969; Harland and Jackson, 2002), among others. For many of these sensory organs, we have a very superficial understanding of their function. Several orders of arachnids also possess a type of particularly fine filiform hair sensilla called trichobothria (Görner and Andrews, 1969; Barth et al., 1993; Barth, 2000). Trichobothria and similar hair sensilla in crustaceans and insects are extremely sensitive to very small medium particle displacements (Görner and Andrews, 1969; Breithaupt, 2002; Shimozawa et al., 2003). Spiders can possess multiple trichobothria on their legs and the currently known hair functions include prey capture and predator avoidance (Barth et al., 1995; Suter, 2003), with no existing examples (in spiders) of their use in receiving air particle movement signals as part of courtship assessment.
The potential incorporation of air particle movement in the production and reception of communication displays has not received much research attention beyond a few focal taxonomic groups. The well-known waggle dance of honeybees, for example, generates air particle movement (265–350 Hz) that enables hive mates to find the dancer even in the dark (Tsujiuchi et al., 2007). Directional air particle movement produced by the wings of male Drosophila is an important aspect of their courtship display (Tauber and Eberl, 2003). In courtship displays of African cave crickets (Phaeophilacris spectrum), males perform a series of wing flicks at a low frequency of 8–12 Hz which the females respond to by calming down into a receptive state for copulation (Heidelbach et al., 1991; Heidelbach and Dambach, 1997). Finally, during agonistic contests in the amblypygid Phrynus marginemaculatus, individuals rapidly vibrate their antenniform legs close to their opponent at a frequency of around 29 Hz (Santer and Hebets, 2008). The duration of these antenniform leg vibrations is predictive of contest winners (Fowler-Finn and Hebets, 2006; Santer and Hebets, 2008) and trichobothria were shown to be responsive to the displayed frequencies of air particle movement (Santer and Hebets, 2008). In a follow-up study, when these trichobothria were ablated, contests increased in duration (Santer and Hebets, 2011), consistent with the idea that the production and reception of air particle movement is a critical part of agonistic signaling in this species.
While a communication function has only been explored in a handful of arthropod taxa, the production and reception of air particle movements is important in many other contexts. The trichobothria of wandering spiders (Cupiennius salei), for example, can respond to the wingbeats of a fly more than 25 cm away (Barth et al., 1995). In the fishing spider, Dolomedes triton, air particle movements detected by trichobothria enable spiders to respond faster to attacks by predators (Suter, 2003). Caterpillars Baratha brassicae L. (Lepidoptera, Noctuidae) exhibit defensive displays in response to low frequency stimuli which they receive with filiform hairs on their dorsal surface (Markl and Tautz, 1975), and mosquitoes (Toxorhynchites brevipalpis) use the Johnston’s organ at the base of the antennae to sense the air particle movement generated by the wingbeats of other mosquitoes (Gibson and Russell, 2006). Given the prevalence and importance of air particle movement in other contexts, we propose that air particle movement is a likely “hidden” modality in the communication displays of many arthropods.
Like in other taxonomic groups, studies of male courtship signaling in spiders have thus far focused predominantly on bimodal (in this case static and dynamic visual and vibratory) signaling and sensory reception. Jumping spiders in the genus Habronattus, for example, are impressive in their coordination of sexually dimorphic ornamentation and associated dynamic visual displays with their complex vibratory songs that can consist of up to 20 elements (Elias et al., 2003, 2012). Similarly, decades of research on wolf spiders in the genus Schizocosa have explored the form and function of visual (ornamentation and dynamic movements) and vibratory signaling (reviewed in Uetz and Roberts, 2002; Stratton, 2005; Hebets et al., 2013; Uetz et al., 2016; Hebets and McGinley, 2019; Starrett et al., 2022). Despite major advances in our understanding of bimodal signal interactions in select species [e.g., S. ocreata (Scheffer et al., 1996; Uetz et al., 2016); S. uetzi (Hebets, 2005); S. stridulans, (Hebets et al., 2011); S. floridana (Rosenthal and Hebets, 2012)], there remains one Schizocosa species where the visual and vibratory signaling appear non-functional from the female’s perspective—Schizocosa. retrorsa (Banks, 1911).
Schizocosa retrorsa males engage in a multimodal courtship display that conspicuously (to humans) includes visual and vibratory signaling (Rundus et al., 2010; Hebets et al., 2013; Hebets and McGinley, 2019). With their blackened femurs contrasting against the adjacent lightened foreleg segments (Stratton, 2005; Starrett et al., 2022), males extend their legs and rapidly wave them up and down in an “extended leg tap” (hereafter “leg wave”) followed by a “push-up” display (Hebets et al., 1996). There are distinct vibratory signals that accompany the production of both dynamic visual movements (Supplementary Video 1). Nonetheless, multiple studies have now confirmed that S. retrorsa males can successfully mate when females are unable to detect signals in either, or both, of these signaling modalities (Hebets et al., 2008; Rundus et al., 2010; Choi et al., 2019). In mating trials run in complete darkness [without the potential for females to detect visual signaling (DeVoe et al., 1969; DeVoe, 1972)] and on granite (removing the potential for females to detect vibratory signals Elias et al., 2004), pairs were still able to mate as successfully as in trials where they could detect both visual and vibratory stimuli (Rundus et al., 2010). Furthermore, across all signaling environments, even in the absence of visual and vibratory stimuli, the rate of male leg waving was predictive of mating success. Males who engaged in more bouts of leg waving had higher copulation success regardless of the presence or absence of visual or vibratory signals (Rundus et al., 2010), suggesting that females are attending to and assessing the leg waving rate itself. The authors of this latter study calculated the leg waving frequency to be 13.55 Hz and argued that the male’s dynamic leg waving could generate air particle movement that was detectable by a female S. retrorsa (Rundus et al., 2010). Multiple studies have now suggested the potential presence of air particle movement signals in the courtship display of S. retrorsa (Rundus et al., 2010; Choi et al., 2019), but the actual involvement of this signaling modality has not been tested until now.
This study tests the hypothesis that air particle displacement generated by the dynamic leg waving display of S. retrorsa males is crucial for a male’s mating success. Support for this hypothesis would indicate that females rely on a previously overlooked sensory system to detect and assess courtship signaling. The (substrate-borne) vibrations that are common and important in many Schizocosa species’ courtship displays, for example (Hebets et al., 2013), are assumed to be detected by lyriform slit sense organs on the walking legs. These sensory organs are the vibration receptors of spiders and detect physical deformation or cuticular strain in response to vibrations on the surface in contact with the legs (Walcott, 1969; Barth and Bohnenberger, 1978; Barth, 2002). The specific type of vibrations (leg taps or pedipalp drumming by males) that these organs respond to can vary by the placement of the organs on the legs (Knowlton and Gaffin, 2019). Air particle movement, in contrast, is assumed to be detected by filiform sensory hairs (trichobothria) which are deflected by the force of air particles (Barth and Holler, 1999; Barth, 2002). Depending on the frequency of air particle movements, the trichobothria are deflected at certain angles to elicit responses (Barth et al., 1993, 1995) and this can also provide the spider with directional information (Friedel and Barth, 1997). In this study, in the absence of visual or vibratory signals, we aim to test the hypothesis that female S. retrorsa can detect and assess males through the air particle movement generated during courtship leg waving.
To test our hypothesis, we conducted mating trials in the absence (No Noise) and presence (Noise) of artificially introduced air particle movement, or “noise,” intended to reduce the air particle movement signal-to-noise ratio. We also ran foraging trials in No Noise and Noise treatments to ensure that our experimental manipulations did not have overarching effects on behavior. In our mating trials, if air particle movement is a critical signaling channel for S. retrorsa males, we would expect higher mating success in the No Noise treatment group compared to the Noise treatment group. We would also expect that the rate of leg waving would still predict a male’s mating success, even in the absence of visual or vibratory stimuli. Finally, if air particle movement noise reduces or removes a female’s capacity to assess the leg waving display, then we would expect that leg waving rate would only predict mating success in the No Noise signaling environments.
Materials and Methods
We conducted two similar, yet independent, experiments to explore the impact of artificially induced air particle movement (i.e., Noise that would presumably impede the detection of air particle movement signals) on the mating success of Schizocosa retrorsa. Experiment 1 (n = 14 per treatment) was conducted in 2008 at a laboratory on the University of Nebraska-Lincoln’s (UNL) city campus and used white noise as a stimulus for artificially induced air particle movement. Experiment 2 (n = 26 per treatment) was conducted in 2021 at UNL’s field station in western Nebraska (Cedar Point Biological Station) and used a 100 Hz frequency stimulus. The experiment was repeated (Exp 2) with a larger sample size in order to increase our confidence in the results. Given the slightly different experimental design, we analyzed the two experiments separately.
Study Animals
We collected juvenile Schizocosa retrorsa from Marshall, Co., Mississippi, United States near Wall Doxey State Park (34°40′N, 89°28′W) on 19th May 2008 (Exp 1) and from Panola Co., Mississippi, United States near Sardis Dam T8S R6W Sect. 13 (34′23′N 89′47′30″W) on 5th May 2021 (Exp 2). We transported spiders to the laboratory where we housed them individually in plastic cages (5.8 × 5.8 × 7.9 cm, AMAC Plastic Products, United States) covered with masking tape to visually isolate individuals. The top of the cage had a hole fitted with a cork for feeding and the bottom had a hole with a wick that sucked in water. The inside of the cages had screening on 2 sides so the spiders could climb. We placed all cages on top of chicken wire mesh inside a plastic tub (65 × 37 × 14.5 cm) filled with 2–3 cm of water and in a controlled light environment (12 h light, 12 h dark) and constant temperature of 25°C. We fed the spiders twice a week with two 1/16th inch crickets (Gryllodes sigillatus from Ghann’s Cricket Farm). We checked spiders every 1–2 days for molts (shed exoskeleton indicating growth and often sexual maturation) and used only mature females and males in our experiments. We identified mature females by observing the opening of their epigynum on the ventral surface of their abdomen and mature males by their bulbous pedipalps (Foelix, 1996) and black pigmentation on the femur of the first pair of legs (Hebets et al., 1996).
Environmental Treatments (Exp 1 and 2)
To test the influence of artificially introduced air particle movement on the mating success of S. retrorsa, we assessed mating success and associated courtship behavior of males under two experimental signaling environments: (i) No Noise (Figure 1A) and (ii) artificially induced air particle movement or Noise (Figure 1B). We created our No Noise environment by removing the speaker cone from the first speaker (DD Audio, Model: DB65A, Figure 1C, left image) to prevent the introduction of air particle movement. We created a Noise environment by using an identical speaker (DD Audio, Model: DB65A), with the speaker cone intact (Figure 1C, right image) to introduce artificially induced air particle movement into the mating arena.
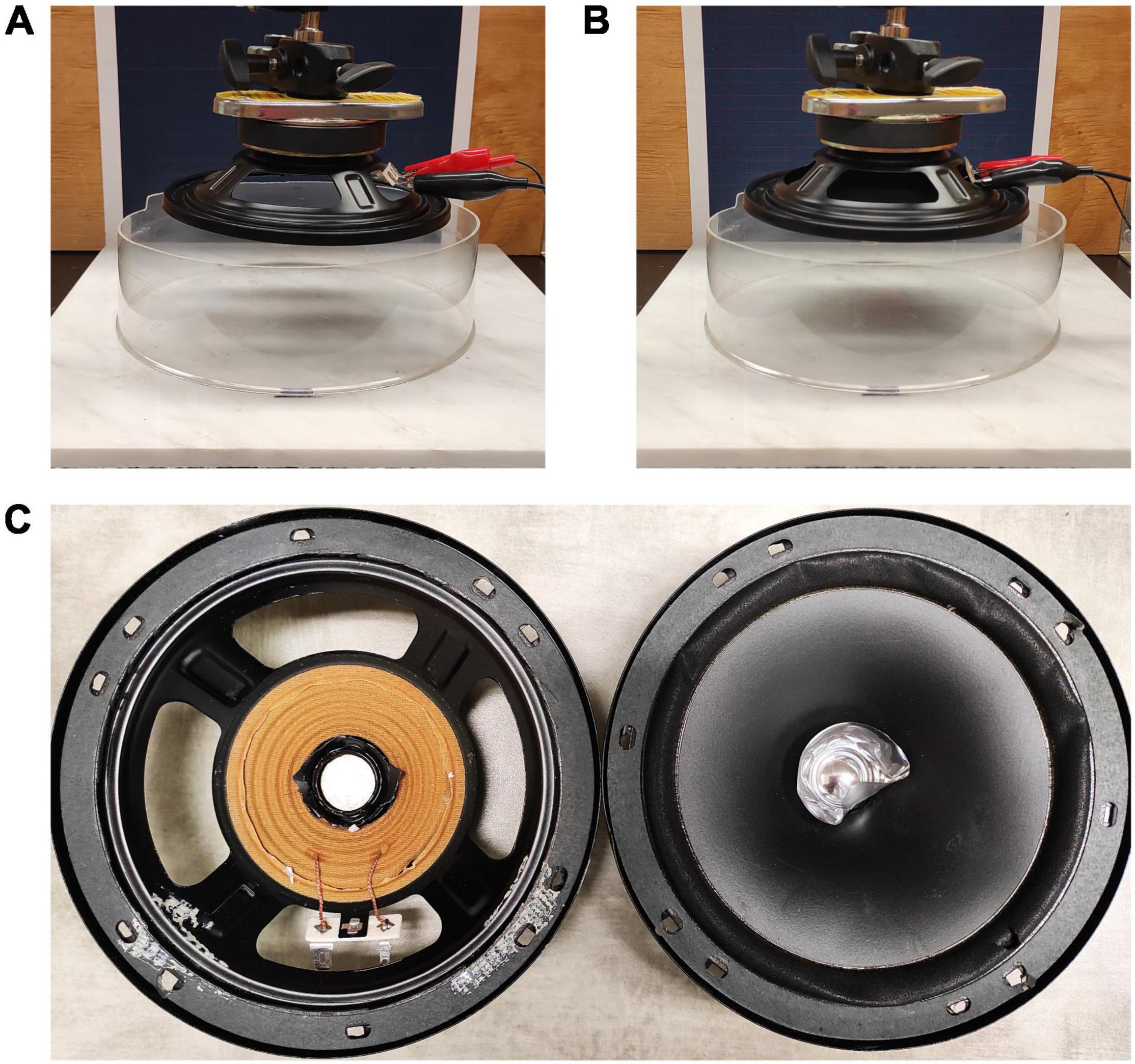
Figure 1. Environmental Treatments—No Noise and Noise Signaling Environments. The mating arenas were set on a granite platform with the speakers held on top of the arena with mechanical arms (A,B). One of the speakers had the speaker cone removed (A,C left) and thus did not introduce air particle movement. The other speaker had the speaker cone intact (B,C right) and introduced air particle movement. All trials were run in the dark.
We positioned the speakers directly above the mating arenas (the outer rim of the speakers was 7.5 cm from the arena floor) using two mechanical arms with magnets (Brand: StrongHand, Model: Snake Magnet, Supplier: StrongHand Tools, United States) attached to the countertop. We connected the speakers to an amplifier (Brand: Rolls, Model: PA71plus MicroMix Power Amplifier) which connected to the laptop. The laptop screen remained completely dark for the entire duration of each trial.
We employed 22–250 Hz white noise oscillations (Exp 1) or constant 100 Hz oscillations of the speaker cone (Exp 2) in order to stimulate trichobothria of a range of lengths on the walking legs of S. retrorsa. The walking legs of spiders are equipped with large numbers of trichobothria of varying lengths, and the frequency tuning of each trichobothrium depends upon its length (Barth, 2002). In the relatively larger wandering spider Cupiennius salei, the best frequencies of trichobothria range from 40 to 600 Hz, but each hair can respond to a relatively broad range of frequencies, and both short and long trichobothria could reliably follow medium oscillations between 10 and 950 Hz (Barth, 2002). We verified that trichobothria were stimulated in both our experiments by observing their movement under a dissecting microscope in response to the stimulus using the speaker with speaker cone intact (Noise, Supplementary Video 2). We also verified that there was no movement of the trichobothria when we used the speaker without speaker cone (No Noise). We adjusted the speaker with the speaker cone intact to a sound level of ∼88 dB (Exp 1) and ∼74 dB (Exp 2) using a digital sound level meter (Brand: RadioShack, Model: Digital Sound Level Meter 33-2055). The speaker with its cone removed was driven with matched amplifier level settings.
To ensure that pairs were not able to detect each other visually or through vibrations, we ran all mating trials in a dark room with no light source and on a surface of granite. Previous studies have demonstrated that granite effectively ablates the transmission of spider courtship signals (Elias et al., 2004). Finally, to record all trials for later analysis, we placed an infrared camera (Brand: Sony, Model: FDR-AX53) in front of the experimental setup at an angle of 10 degrees downwards from the horizontal and an infrared light source (Brand: IR Illuminator, Model: CM-IR110) close to the experimental setup.
Mating Trials (Exp 1 and 2)
Underneath the speakers, our mating arenas consisted of a transparent plastic circular enclosure (diameter 20 cm and height 7.6 cm Exp 1 and 6.3 cm Exp 2) resting on top of a granite slab. We coated the top inner circumference of the arena wall with petroleum jelly to prevent spiders from climbing out. Before each mating trial, we cleaned the arena and granite slab with deionized water (Wilder et al., 2005). We cleaned everything with 70% ethanol at the end of each day. We always ran one No Noise and one Noise treatment simultaneously in Experiment 1 and back-to-back trials (due to limited space) in Experiment 2. In Experiment 1, the arenas were separated by ∼5.4 cm. Given that the speakers were directly above each mating arena, it was unlikely that air particle movement was being introduced into our No Noise arena from the adjacent Noise speaker. We randomly paired females and males, and each individual spider was only used once.
On the day of the experiment, we weighed focal females and placed them in the mating arena in the dark on granite for 30 min for acclimatization. During that time, we weighed the paired males. After 30 min of acclimatization, we introduced the male into the arena, but restrained him underneath a small removable barrier (a plastic cylinder, open on the ends, diameter 3 cm). We turned on the speakers and the infrared camera, released the male and allowed the pair to interact for 30 min.
After 30 min, we recorded whether the pairs were mating or not. Schizocosa retrorsa mate for an extended duration (150–160 min, Hebets et al., 1996), making it unlikely for us to have missed a mating. We also scored all the trials using our infrared recordings. Following all trials, we euthanized females and males by freezing, and we preserved them in 70% ethanol. The spiders were at the end of their natural life (life span: 1 year) and the preserved specimens are retained in our collection (Hebets laboratory) at the University of Nebraska-Lincoln.
Behavioral Scoring (Exp 1 and 2)
We used BORIS-2021-09-20: v.7.12.2 to quantify the videos of the mating trials. We calculated the “latency to courtship” as the time (in seconds) from when the males were released into the arena until the males started courting. We calculated “latency to mate” as the time (in seconds) from when the males started courting until they mated, or till the end of trial (Exp 1) or the last time they courted right before the trial ended (Exp 2). For Experiment 1 and 2, we calculated the “rate of leg waving bouts” (#/second) as the number of recorded leg waving bouts divided by “latency to mate.” We defined a “bout” as a period of leg waving separated by walking, grooming, inactivity, or push-up displays. This variable has been scored and used previously for S. retrorsa behavioral analyses (Hebets et al., 1996), and similar measures have been used in other Schizocosa species to calculate courtship rate (e.g., Rosenthal and Hebets, 2012). For Experiment 2, we additionally calculated the “rate of individual leg waves” (#/second) as the number of individual leg waves divided by “latency to mate.” Because we are interested in the potential for each leg wave to generate air particle movement, we felt this was a better measure of leg waving rate than considering only each leg waving bout. Unfortunately, the same data (i.e., # individual leg waves) were not available for Experiment 1. Nonetheless, an analysis of the relationship between rate of leg waving bouts and rate of individual leg waves in Experiment 2 shows that they are highly correlated (Supplementary Figure 1). Finally, push-up displays are generally associated with leg waving bouts and for Experiment 2, we also calculated the “rate of push-up” (#/second) as the number of recorded push-up divided by “latency to mate” (data unavailable for Exp 1).
Foraging Trials (Exp 2)
In Experiment 2, we aimed to further explore the impact that our experimental treatments had on general female and male behavior and so we examined foraging behavior. We ran foraging trials 12–20 h before the mating trials. In the foraging trials, we used the same environment—No Noise or Noise—that each individual would be exposed to for their mating trial. We released spiders into the “mating” arena (diameter: 20 cm, height: 6.3 cm; in the dark; on granite) where we allowed them to roam freely for 3–4 min with the speakers playing the treatment stimulus. We then introduced a single 0.32 cm (1/8th inch) cricket (Gryllodes sigillatus) into the arena opposite to the position of the spider. We left the spider and cricket to interact for 3 min, after which time we noted whether the spider ate the cricket or not.
Statistical Analyses
We analyzed each mating experiment (Experiment 1 and 2) separately. For each, to ensure that there were no differences between our treatments (No Noise/Noise) with respect to female/male age or weight, we compared the ages and weights of females and males. Also, we compared age and weight differences of the females and males between the No Noise and Noise trials. We ran unpaired t-tests to make sure there were no significant differences in these categories.
To determine if the experimental signaling environments (No Noise/Noise) influenced mating success (yes/no), we used a Chi-square test to compare mating frequency across each signal environment. We performed a Kaplan Meier survival analysis to determine if there were differences in “latency to mate” between the signaling environments.
To determine if the experimental treatments (No Noise/Noise) influenced male courtship behavior, we compared the rate of leg waving bouts between the No Noise/Noise treatments for each experiment separately using independent two-sample t-tests. For Experiment. 2, we also compared the rate of individual leg waves, and the rate of push-ups between the signaling environments using independent two-sample t-tests. We only did this for Experiment 2 because we did not have these data for Experiment 1. We also explored the relationship between rate of leg waving bouts and rate of push-up (Exp 2) using Pearsons correlation analysis (Supplementary Figure 2).
Finally, given that we found differences in male signaling rates between the signaling environments for both Experiment 1 and 2 (see “Results” section), we built binomial logistic regression models for each experiment with predictor variables including female age, male age, female weight, male weight, signaling environment (Noise/No Noise), and rate of leg waving bouts and an interaction between signaling environment and rate of leg waving bouts. Our response variable was mating (yes/no). We did not include rate of push-up in these analyses since we were explicitly interested in the impact of air particle movement, which are suggested to be generated by the dynamic motion associated with leg waving, not push-up (Rundus et al., 2010). We dropped all the terms except the signaling environments and the rate of leg waving bouts by backward selection for significant terms. We ran a second model for Experiment 2 using the rate of individual leg waves in place of leg waving bouts. The results of the models were summarized using Anova Type II and Wald Test. Given that our results suggested an influence of leg waving (analyzed as both bouts and individual waves) on mating success in both experiments, we also compared the rate of leg waving bouts between males that mated compared to the ones that did not mate for each experiment using independent two sample t-tests.
To determine if the experimental treatments (No Noise or Noise) had an influence on foraging behavior (Exp 2 only), we built a binomial logistic regression model with foraging success (yes/no) as the response variable and signaling environments, sex, and signaling environments by sex as the predictor variables.
The data were analyzed using the R 4.1.3 binary [for macOS 10.13 (High Sierra) and higher] through RStudio Desktop. The packages used in R are tidyverse, ggplot2, survival (function Surv), car (function Anova), ggpubr (function t_test), and stats.
Results
Environmental Treatments (Exp 1 and 2)
For Experiment 1, females were on average 22.429 ± 3.91 days post-maturation, while males were 31.821 ± 2.816 days post-maturation. Females weighed 79 ± 14 mg while the males weighed 53 ± 7 mg. For Experiment 2, females were on average 13.88 ± 0.92 days post-maturation, while males were 24 ± 1.83 days post-maturation. Females weighed 56.5 ± 5 mg while the males weighed 41.88 ± 3.9 mg. There was no significant difference in the ages or weights of the females and males between the treatment groups in either Experiment 1 (Supplementary Table 1) or Experiment 2 (Supplementary Table 2) or in the pairwise difference between female/male age or weight within pairs across the treatment groups (Supplementary Tables 1, 2).
Mating Trials (Exp 1 and 2)
Mating success was influenced by the signaling environment across both experiments. In Experiment 1, significantly more pairs mated in the No Noise (7/14; 50%) than in the Noise (1/14; 7.14%) signaling environments (χ2 = 6.3, df = 1, p = 0.012*) (Figure 2A). We found comparable results in Experiment 2 where mating success was significantly higher in the No Noise (14/26; 53.85%) than in the Noise (4/26; 15.38%) signaling environments (χ2 = 8.497, df = 1, p = 0.004**) (Figure 2B).
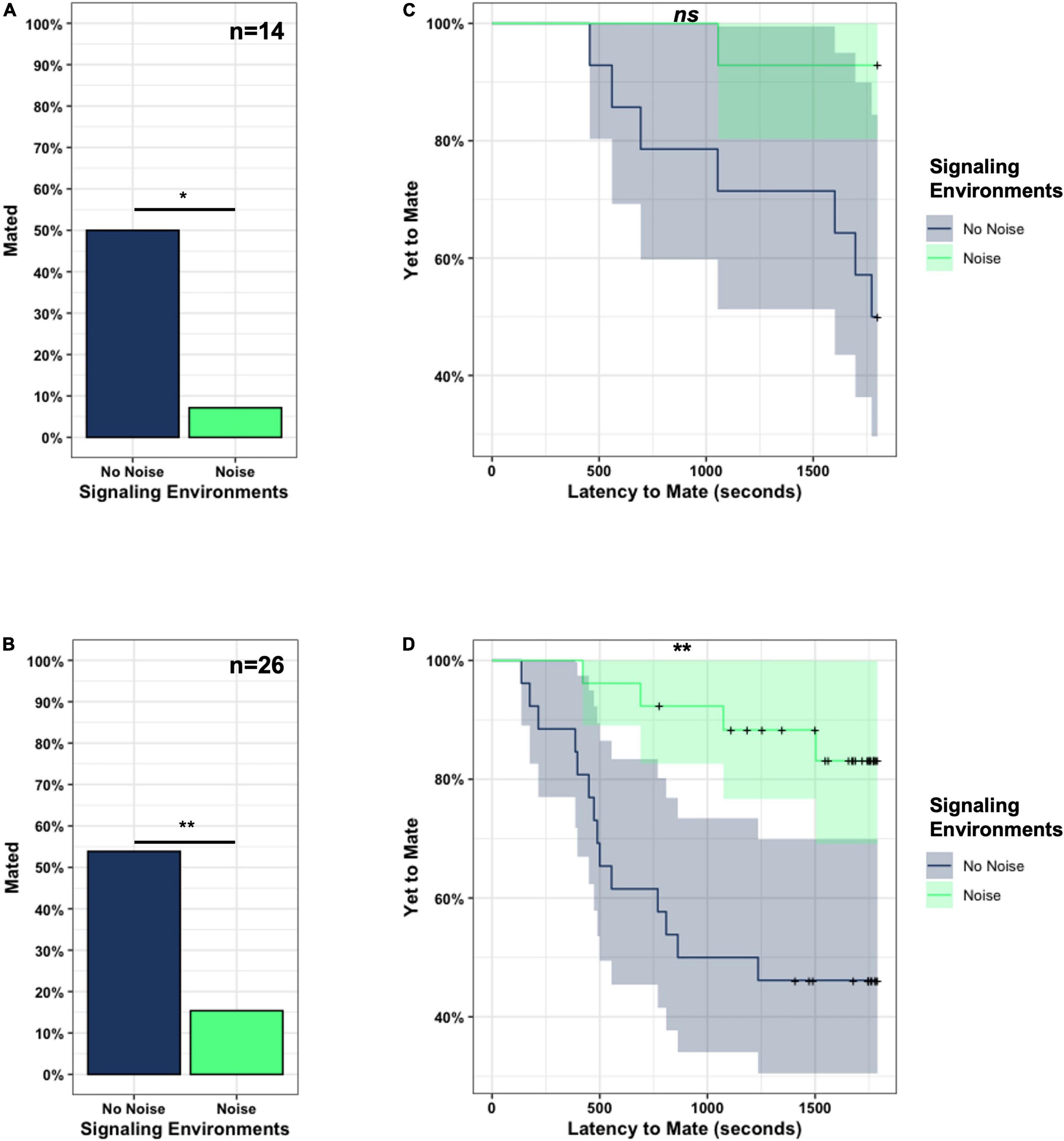
Figure 2. Mating success was higher and faster in the No Noise compared to the Noise signaling environments. In both Experiment 1 (A, *p < 0.05) and Experiment 2 (B, **p < 0.01) more pairs mated in the No Noise than in the Noise signaling environments. In Experiment 1 (C), pairs that mated also tended to do so faster in the No Noise compared to the Noise signaling environments (p = 0.07: Here, ns-not significant), but the sample size was low, with only 1 mating in Noise. In Experiment 2 (D) pairs that mated did so significantly faster in the No Noise than in the Noise signaling environments (**p < 0.01). The shaded regions in the Kaplan Meier survival analysis (C,D) are the 95% confidence intervals at each time point and “+” signs (often superimposed) indicate pairs that did not mate (censored pairs).
The signaling environments also influenced the time to mate once courtship started (i.e., “latency to mate”). Pairs tended to mate more quickly in the No Noise environments. This trend was marginally non-significant in Experiment 1 (Figure 2C, latency to mate- No Noise: 1459.643 ± 522.776 s, Noise: 1746.857 ± 198.842 s, Test-statistic = −1.921, df = 16.684, p = 0.072), and significant in Experiment 2 (Figure 2D, latency to mate—No Noise: 1062.142 ± 629.598 s, Noise: 1472.138 ± 382.298 s, Test-statistic = −2.838, df = 41.229, p = 0.007**).
The signaling environments significantly influenced the rate of leg waving bouts in both experiments. In Experiment 1, males had a significantly higher rate of leg waving bouts in No Noise than in Noise signaling environments (No Noise: 0.02 ± 0.007 #leg waving bouts/s; Noise: 0.01 ± 0.007 #leg waving bouts/s, test-statistic = 3.938, df = 25.905, p < 0.001***; Figure 3A). In Experiment 2, males also showed a significantly higher rate of leg waving bouts in No Noise (0.021 ± 0.011 #leg waving bouts/s) compared to Noise (0.012 ± 0.01 #leg waving bouts/second) signaling environments (test-statistic = 3.009, df = 49.81, p = 0.004**; Figure 3B). In Experiment 2, we also examined the rate of individual leg waves and rate of push-ups. We found that rate of individual leg waves was significantly higher in No Noise (0.224 ± 0.122 #individual leg waves/s) compared to Noise (0.14 ± 0.137 #individual leg waves/s) signaling environments (test-statistic = 2.324, df = 49.363, p = 0.024*; Figure 3C). The rate of push-ups was also significantly higher for No Noise (0.038 ± 0.018 #push-ups/s) than for Noise (0.022 ± 0.019 #push-ups/s) signaling environments (test-statistic = 3.074, df = 49.983, p = 0.003**, Figure 3D). As described in previous studies (Hebets et al., 1996; Rundus et al., 2010), rate of leg waving bouts and rate of push-ups are correlated in the courtship of S. retrorsa (Supplementary Figure 2).
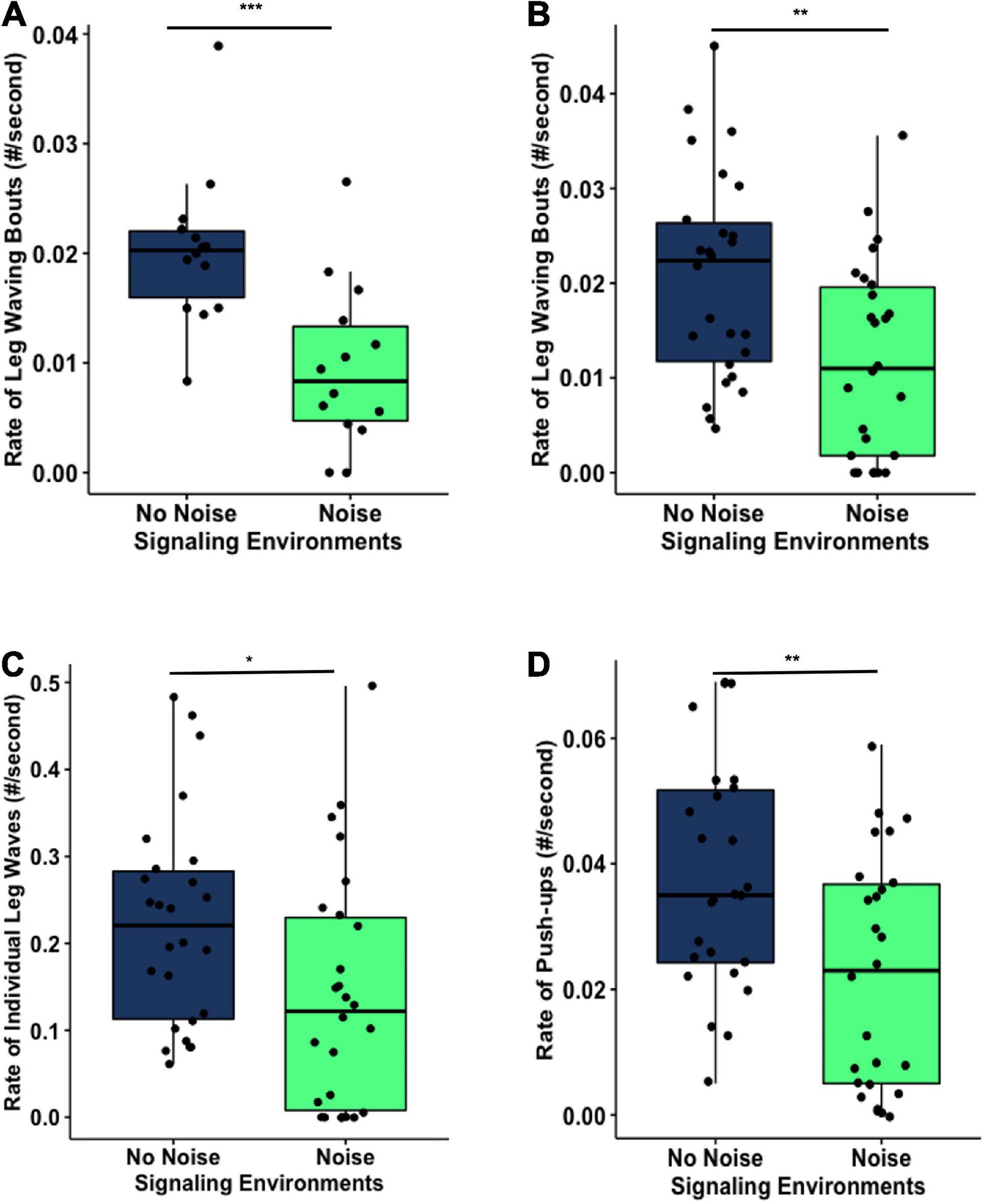
Figure 3. Male courtship behavior is environment dependent. The rate of leg waving bouts by the males in the No Noise environment was significantly higher than that in the Noise environment in Experiment 1 (A, ***p < 0.001) and in Experiment 2 (B, **p < 0.01). The rate of individual leg waves (C, *p < 0.05) and rate of push-ups (D, **p < 0.01) in Experiment 2 were also significantly higher in the No Noise environment than in the Noise environment.
To evaluate the relative importance of the signaling environment (Figure 2) and rate of leg waving bouts (Figure 3) on mating success, we used a binomial logistic regression model. With the smaller sample size of Experiment 1, our overall model was marginally non-significant (Wald χ2 = 4.7, df = 2, p = 0.094). For Experiment 2, when we used the rate of leg waving bouts for the model, our overall model was significant (Wald χ2 = 12.2, df = 2, p = 0.002**) with rate of leg waving bouts being highly significant (LR χ2 = 13.857, df = 1, p < 0.001***) and the signaling environment marginally non-significant (LR χ2 = 3.243, df = 1, p = 0.072). When we used the rate of individual leg waves for the Experiment 2 model, the overall model is again significant (Wald χ2 = 10.6, df = 2, p = 0.005**), and both the rate of individual leg waves (LR χ2 = 7.4515, df = 1, p = 0.006**) and the signaling environment (LR χ2 = 5.409, df = 1, p = 0.02*) showed a significant influence on mating success.
In Experiment 1, males that mated had significantly higher rates of leg waving bouts (Mated: 0.034 ± 0.013 leg wave/s; non-Mated: 0.015 ± 0.008 leg wave/s; test-statistic = 4.238, df = 12.40168, p = 0.001**; Figure 4A). We found the same result in Experiment 2 (Mated: 0.025 ± 0.01 leg wave/s; non-Mated: 0.012 ± 0.009 leg wave/s; test-statistic = 3.399, df = 31.177, p < 0.001***; Figure 4B).
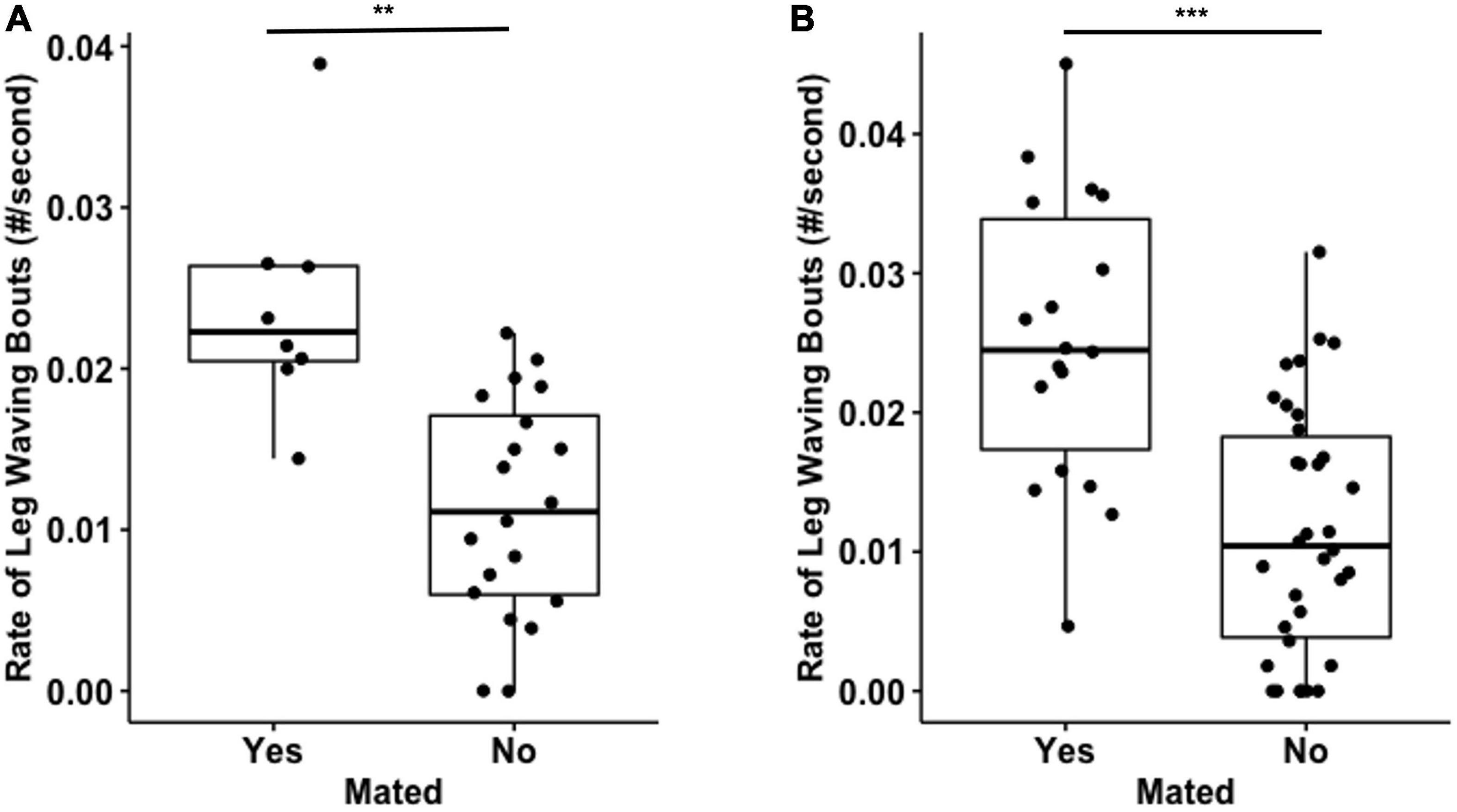
Figure 4. Mating males displayed higher rates of leg waving bouts. The rate of leg waving bouts by males that mated was significantly higher than by males that did not mate in Experiment 1 (A, **p < 0.01) and in Experiment 2 (B, ***p < 0.001).
Foraging Trials (Exp 2)
In the No Noise signaling environment, 14/26 females successfully captured prey (54%) while 13/26 females (50%) did so in the Noise environment. For males, 11/26 successfully captured prey (42%) in the No Noise environment while 9/26 males (35%) did so in the Noise environment. Our model exploring the influence of sex and environment on the likelihood to forage was not significant (Overall Model: Wald χ2 = 2.3, df = 3, p = 0.52), indicating that there was no influence of the signaling environment, nor was there an interaction between signaling environment and sex, on the likelihood of successfully catching a cricket.
Discussion
Our results demonstrate that the addition of air particle movement into the environment disrupts the mating success of S. retrorsa and alters male courtship behavior but does not impact foraging success. These results confirm that S. retrorsa can detect air particle movement and suggest that this previously under-explored sensory modality is critical in their courtship communication. The dynamic motion display of S. retrorsa leg waving, formerly presumed to be important in visual signal production/detection, had been proposed to (i) generate stimuli detectable by the thin filiform sensory hairs (trichobothria) located on S. retrorsa walking legs and (ii) be important in female mating decisions (Rundus et al., 2010). Our results support these hypotheses. Across two similar yet independent experiments, we observed higher mating success in environments without (No Noise) compared to with (Noise) artificially induced air particle movement, or “noise.” Mating also happened faster in the No Noise as compared to the Noise signaling environments. Notably, in addition to influencing mating success, our manipulated signaling environments also affected male behavior, with overall rates of leg waving bouts, individual leg waves and push-ups being higher in the No Noise compared to Noise environments. Regardless of this plasticity in male courtship effort, however, females appeared to use leg waving rate as a means of assessing males independent of the signaling environment. Across all treatments in both experiments, male leg waving rate was a good predictor of mating success, with no observed interaction with the signaling environment. Given that all mating trials were run in environments that presumably prevented the female’s detection of visual and vibratory signals/cues, we propose that leg waving rate was detected with the female’s trichobothria, which are sensitive to air particle movement. Although previous research alluded to a role of air particle movement in the courtship display of Schizocosa retrorsa (Rundus et al., 2010; Choi et al., 2019), this study provides more direct support for its importance.
Mating success was higher and the latency to mate was shorter in the No Noise than the Noise signaling environments in both Experiment 1 and 2. Presumably, this is because females are more easily able to assess male leg waving in the absence of artificially induced air particle movement and are thus able to make mate choice decisions based on leg waving rate more quickly. The quality of decision making across animals is proposed to depend on speed-accuracy tradeoffs (Chittka et al., 2009). In our Noise environment, since the accuracy of the information that males were communicating (i.e., leg waving rate) was disrupted by the addition of air particle movement, or at least the signal-to-noise ratio was reduced, assessment challenges were likely imposed on females. The outcome was that fewer females accepted males, and those that did accept males took longer to make that decision. Speed-accuracy tradeoffs with respect to mating decisions have been observed in other taxa as well. Fiddler crabs (Uca annulipes) must accurately time their larval release to coincide with the next nocturnal spring tide. At the beginning of sampling period, the female crabs are choosy and sample the bigger males of the population. However, toward end of the sampling period, females are less selective due to the time constraints (Backwell and Passmore, 1996). When female sand gobies (Pomatoschistus minutus) were exposed to a high female to male ratio, the mate choice decisions were sped up and these females end up choosing males with low fecundity. However, in the opposite situation where female to male ratio was lower, female sand gobies took longer to make spawning decisions and chose males with high fecundity and good parenting skills (Diaz Pauli and Lindström, 2021).
An alternative explanation to air particle noise disrupting a female’s ability to assess male courtship is that it disrupted the behavior of females and/or males, leading to lower mating rates. To attempt to address this concern, as part of Experiment 2 we conducted foraging trials under both signaling environments (No Noise/Noise) and found no impact of the signaling environment on prey capture. A lack of difference in foraging success across our treatments suggests that overall behavior of females and males (e.g., movement rate, motivation to forage, and/or prey detection) were likely not impacted by our environmental manipulations. These foraging trials were also run in the dark and on granite, presumably removing visual and vibratory cues for prey detection and thus leaving air particle movement, chemical, and tactile (e.g., running into the prey) cues as means of detecting prey. The lack of a treatment effect on foraging success suggests the spiders in our experiment did not use air particle movement to detect prey, leaving open the possibility of chemical and/or tactile prey detection. Although it remains possible that spiders might also be good at filtering out air particle noise when detecting environmental stimuli. Regardless, our foraging trials provide no evidence of general behavioral impacts of our experimental treatments on S. retrorsa females or males.
In contrast to the results of our foraging trials, we found that our environmental treatments in the mating trials did influence male courtship effort. Male leg waving rates and push-up displays were significantly higher in the No Noise than in the Noise conditions across both experiments. These results suggest that the detection of artificial air particle movement by males influenced their courtship behavior specifically (i.e., not foraging behavior), causing them to court less intensely or less vigorously. Previous studies have similarly shown S. retrorsa male courtship behavior to be dependent upon environmental conditions. In a study that examined the influence of age on courtship, younger S. retrorsa engaged in more courtship toward virgin females (and associated chemical signals) while older males courted virgin and mated females indiscriminately (Rundus et al., 2015). More recently, it was shown that vibratory cues associated with male density can influence a S. retrorsa male’s courtship effort (Choi and Hebets, 2021). Our findings of behavioral plasticity in courtship corroborate these earlier findings and highlight the importance of the signaling environment on male signaling in S. retrorsa.
The continually observed relationship between courtship rate (i.e., leg waving rate) and mating success in S. retrorsa (Rundus et al., 2010; present study) suggests that females choose males based on motor performance, or vigor (see Byers et al., 2010). If such behavior is energetically costly, and if males perceive that the signaling environment may not be conducive to signal transmission (for example, due to noise in the environment), they may reduce their effort, resulting in a lower leg waving rate. This is but one potential explanation for our observed reduction in courtship rate in the presence of noise. A few other possibilities are that males are simply distracted by the addition of noise, or that there are sensory constraints on processing, for example. To explore the possibility that our noise treatment distracted males or led to a decrease in overall movement of males, including courtship behavior, we used data from Experiment 2 to examine the relationship between the number of female-male contacts (a potential proxy of “activity” or “movement”) and male leg waving rate. We did not find a significant relationship between rate of contacts and rate of leg waving bouts (R = 0.037, p = 0.8) or between rate of contacts and rate of individual leg waves (R = −0.033, p = 0.82). While certainly not definitive, these results indicate that our mating differences are more likely driven by female detection of male-generated air particle movement than by overall male behavior itself.
Environmental noise is known to have broad-ranging effects on wildlife (reviewed in Shannon et al., 2016) and the more we look, the more examples we find of animals that adjust their signaling to overcome environmental noise. The alteration or adjustment of signaling in response to environmental noise has been documented in numerous diverse taxa. For example, common cuttlefish (Sepia officinalis) change their color (visual display) more frequently in presence of anthropogenic noise, even though these fish do not rely on acoustic signaling (Kunc et al., 2014), and Bornean rock frogs (Staurois parvus) use multiple visual signals and modified air-borne acoustic signals to communicate with conspecifics when presented with a noisy environment (Grafe et al., 2012). Similarly, in response to environmental noise, tokay geckos (Gekko gecko) lengthen certain high amplitude call syllables (Brumm and Zollinger, 2017); male great tits (Parus major) sing at a higher average frequency (Slabbekoorn and Peet, 2003); budgerigars (Melopsittacus undulatus) and cotton top tamarins (Saguinus oedipus) increase the amplitude of their signal in the presence of background noise (Manabe et al., 1998; Roian Egnor and Hauser, 2006); and greater mouse-eared bat (Myotis myotis) actively avoid areas with noise while foraging (Schaub et al., 2008), to name a few. Thus, while it is not particularly surprising that male S. retrorsa alter their signaling rate in the presence of air particle noise, it does highlight that behavioral plasticity and responses to the environment are common across all taxonomic groups.
Noise or disturbance in the signaling environment can also alter the receiver’s behavior. In Painted Goby (Pomatoschistus pictus), females depended more on visual signals as opposed to acoustic signals from the male for mate choice decisions when additional noise was introduced in the system (de Jong et al., 2018). Female three-spined stickleback (Gasterosteus aculeatus) paid more attention to visual cues than olfactory cues in clear water but in turbid water, olfactory cues were more important (Heuschele et al., 2009). Recent work on female tungara frog, Physalaemus pustulosus, demonstrated that air-borne noise influences the expression of female mating preferences (Taylor et al., 2021). In our study, noise impacted signaler behavior but its effect on mate choice of the receivers still needs to be investigated further.
In Experiment 1, our higher mating success in the No Noise signaling environment appears to be driven by male behavior. Males courted less vigorously in the No Noise treatment, and since courtship rate predicts mating success, our results could be explained solely by changes in male behavior across the environments. Because our sample size in Experiment 1 was low, and the influence of male courtship rate on mating success was so high, we were unable to detect or disentangle an influence of the signaling environment itself on mate choice. Thus, we repeated the experiment. In Experiment 2, with a larger sample size, we were able detect the influence of both male behavior (i.e., rate of leg waving bouts as well as individual leg waves) and the overall signaling environment on mating success. Results of Experiment 2 suggest that lower mating success in the Noise condition was driven by both (a) changes in male behavior and (b) an overall effect of the signaling environment on female mate choice. Indeed, when we use individual leg waves in our model for Experiment 2, we find an effect of both rate of individual leg waves and signaling environment on mating success.
Overall, our results support the possibility that female S. retrorsa use detected air particle movement from male leg waving displays to make mate choice decisions. Regardless of whether noise was absent or present, females mated more frequently with males that displayed higher rates of leg waving across both experiments. If our artificially induced air particle movement completely disrupted a female’s ability to detect the air particle movement generated by male leg waving displays, we would have expected to find a significant interaction between leg waving rate and signaling environment on mating success. In particular, we would have expected leg waving rate to predict mating success in the No Noise, but not the Noise environments. The absence of this result suggests that the female’s ability to assess the “rate” of male leg waving, presumably by the detection of air particle movement, was not impacted by our noise treatment; though overall detection and speed of detection was likely compromised (see previous discussion). We note, however, that even in the case of a significant interaction, the signal might still work, just less effectively.
An alternative explanation to female’s detecting air particle movement is that leg waving rate is correlated with a signal in another modality, such as chemical signaling, that females are still able to detect. If, for example, males release a pheromone and use leg waving as a means of dispersing it, then we might expect the same results as those observed across our experiments. This possibility remains to be tested. There are currently no known airborne chemical signals in Schizocosa communication, but there are many examples of silk-borne cues/signals (Roberts and Uetz, 2005; Baruffaldi and Costa, 2010; Vaccaro et al., 2010). Confirmation of the importance of air particle detection per say will require some combination of trichobothria removal experiments and electrophysiology.
The absence of an interaction between signaling environment and leg waving rate in either experiment may be due, in part, to our choice of “noise” stimuli. In Experiment 1, our artificial stimulus was a white noise band with frequencies of 22–250 Hz while in Experiment 2, it was a 100 Hz. Previous calculations of S. retrorsa leg waving rate were 13.55 Hz, which is lower than either of our artificial stimuli. This non-overlap in frequency range between our introduced noise and male leg waving rate is the simplest explanation for why female mate choice did not appear disrupted by air particle “noise.” We would expect that if a noise stimulus that directly overlaps with male signaling rates were introduced, female mate assessment would be compromised. Future studies could test this prediction.
We are confident that our artificial air particle movement stimuli were sufficient to elicit responses from S. retrorsa trichobothria. First, air particle movement receptors are incredibly sensitive (Shimozawa and Kanou, 1984; Humphrey and Barth, 2007) and are often tuned to low frequencies (<500 Hz) (Rinberg and Davidowitz, 2000; Shamble et al., 2016; Raboin and Elias, 2019). Second, we confirmed the deflection of trichobothria from our stimuli by viewing their movement under a dissecting scope prior to the start of our trials. Third, we observed a change in male behavior in the presence of introduced air particle movement, i.e., reduced leg waving and push-up rate. Our choice of stimuli for introducing air particle movement was based upon working knowledge of general sensitivities of trichobothria (Barth, 2002). This working knowledge all comes from one model spider system, Cupiennius salei, but it is assumed to be generalizable across spiders.
Previous dogma has understood that the reception, and thus importance, of air particle movement in communication would be limited because it only occurs at short distances from the source—approximately 0.5–1 wavelength (Kinsler, 1999; Jacobsen, 2007; Raboin and Elias, 2019). Recent studies, however (e.g., Shamble et al., 2016; Menda et al., 2019; Stafstrom et al., 2020) suggest that the detection of air particle movement may take place at much longer distances than previously thought (reviewed in Raboin and Elias, 2019), making it a viable mode of communication. Nonetheless, air particle movement (also referred to as near-field sound) has been overlooked and underappreciated as a signaling modality, and the impact of noise on this understudied signaling modality is even more unknown (Raboin and Elias, 2019).
Air particle movement in the natural environment is produced by numerous sources including abiotic (e.g., wind) as well as biotic (e.g., anthropogenic, animal movements) sources. Many sources of anthropogenic noise, for example, occur in the same low frequencies often associated with air particle movement, e.g., noise from roads (Hayek, 1990) and railways (Talotte et al., 2003), among others (reviewed in Raboin and Elias, 2019). In terms of biological sources, bees, flies, and related insects have wing beat frequencies ranging from 48 to 250 Hz (Corben, 1983). Thus, is seems likely that spiders, and other arthropods, are exposed to air particle noise. The extent to which this disrupts communication in nature requires additional exploration (Raboin and Elias, 2019).
In our study, results suggest that S. retrorsa males produce air particle movement using dynamic leg waving displays during courtship and that this movement is likely detected and assessed by females. The detection of such air particle movement is likely coincident with the detection of visual movement and vibratory courtship songs (Hebets et al., 1996). If and how females integrate these components, received by different sensory organs (vision—eyes; vibration—slit sensilla; air particle movement—trichobothria), during their assessment of male courtship remains an open question. Multimodal integration, or the combining of information received from multiple sensory modalities to influence decision-making (see Munoz and Blumstein, 2012) is not well understood in most animal systems. Within arthropods, the best studied systems include insects such as female mosquitos, Aedes aegypti, that integrate carbon dioxide, visual, and thermal cues (McMeniman et al., 2014); female bush crickets, Requena verticalis, that integrate visual and acoustic cues (Bailey et al., 2003); and the fly model Drosophila melanogaster that integrates visual, auditory, chemosensory, and mechanosensory signals during courtship (Spieth, 1974; Hall, 1994; Lasbleiz et al., 2006); among others (reviewed in Thiagarajan and Sachse, 2022). Within arachnids, recent evidence suggests that the amblypygid Phrynus marginemaculatus integrates visual and tactile cues during navigation (Flanigan et al., 2021). In wolf spider genus Schizocosa, numerous studies have demonstrated an interactive effect of multimodal signals on receiver behavior (Hebets, 2011; Higham and Hebets, 2013; Halfwerk et al., 2019). In our experimental design, we prevented the integration of modalities, as we purposefully created environments where females could not detect visual or vibratory stimuli. It will be worthwhile to explore these possibilities in S. retrorsa with respect the female’s perception and potential integration of multisensory signal components.
Conclusion
In summary, our mating trials were run in environments where females were unable to detect visual and substrate-borne vibratory signals, and we found that (i) the presence of air particle noise reduced mating frequency and (ii) increased the latency to mating. Additionally, across all signaling environments (iii) mating was predicted by leg waving rate, despite (iv) leg waving rate being influenced by the signaling environment. These results suggest that male courtship is plastic and results are consistent with the hypothesis that the dynamic motion of leg waving generates air particle movement that is detected and assessed by females during mate choice.
The understanding of multimodal communication in organisms that are more distantly related to humans, like arachnids, is often limited because of our own sensory biases and experimental constraints. It is also often challenging to determine which sensory systems receivers rely upon while assessing complex communication displays. Finally, the potential integration of sensory stimuli and how that influences decision making is still not understood in most animal signaling systems. Our study demonstrates the potential importance of a previously underappreciated signaling modality and we suspect that many similar instances exist across disparate animal groups.
Data Availability Statement
The raw data supporting the conclusions of this article will be made available by the authors, without undue reservation.
Author Contributions
AR, RS, and EH conceptualized and designed the experiment. AR conducted experiment 1. PK conducted experiment 2. NC helped with experiment 2 set-up. All authors contributed to data analysis, interpretation, writing and editing.
Conflict of Interest
The authors declare that the research was conducted in the absence of any commercial or financial relationships that could be construed as a potential conflict of interest.
Publisher’s Note
All claims expressed in this article are solely those of the authors and do not necessarily represent those of their affiliated organizations, or those of the publisher, the editors and the reviewers. Any product that may be evaluated in this article, or claim that may be made by its manufacturer, is not guaranteed or endorsed by the publisher.
Acknowledgments
We acknowledge Madison Hays, Drs. Rowan McGinley, Guilherme Oyarzabal da Silva, Gail Stratton, and Pat Miller for their help with collecting the spiders. We thank Dr. Gail Stratton and Pat Miller for food and lodging during collecting trips. We thank members of the Hebets, Basolo, Wagner, and Shizuka laboratories for their feedback on the experiments. We thank Dr. Rowan McGinley for Supplementary Video 1. We thank our funding sources NSF (IOS—1556153, IOS—1037901, IOS—1456817), the Searle Scholars Program and UNL QLSI Summer Graduate Student Fund. We thank Cedar Point Biological Station for food, lodging and a place to run Experiment 2. Finally, we also thank the editors of this special issue for the invitation to contribute and we are especially grateful to two reviewers that provided incredibly thoughtful and helpful comments that strengthened this manuscript greatly.
Supplementary Material
The Supplementary Material for this article can be found online at: https://www.frontiersin.org/articles/10.3389/fevo.2022.939133/full#supplementary-material
Supplementary Figure 1 | Rate of leg waving bouts and rate of individual leg waves are correlated. There is a strong correlation between the rate of leg waving bouts and rate of individual leg waves (R = 0.9, p < 2.2e-16).
Supplementary Figure 2 | Rate of leg waving bouts and rate of push-ups are correlated. There is a strong relationship between the rate of leg waving bouts and the rate of push-ups (R = 0.74, p = 5.2e-10).
Supplementary Table 1 | Comparison of age and weight of the spiders based on sex and signaling environments for Experiment 1. When the age and weight of females and males were compared for the two signaling environments, there was no significant difference between the groups (weights of two females from each No Noise and Noise groups weremissing). There was no significant difference between the age difference and weight difference of the females and males in each of the groups either.
Supplementary Table 2 | Comparison of age and weight of the spiders based on sex and signaling environments for Experiment 2. When the age and weight of females and males were compared for the two signaling environments, there was no significant difference between the groups. There was no significant difference between the age difference and weight difference of the females and males in each of the groups either.
Supplementary Video 1 | Male Schizocosa retrorsa courtship behavior. Male Schizocosa retrorsa displaying courtship behavior including push-up and leg waves.
Supplementary Video 2 | Observed movement of the trichobothria on female Schizocosa retrorsa. To provide a more focused noise stimulus in Experiment 2 while still ensuring that the spiders could detect the air particle movement, we examined the movement of foreleg trichobothria under a dissecting scope at sound frequencies of 0–150 Hz using the speaker with speaker cone intact (Figure 1C, right). The trichobothria were observed to be moving between 70 and 130 Hz. This video shows the clear movement of trichobothria at 100 Hz in the Noise signaling environment.
References
Backwell, P. R. Y., and Passmore, N. I. (1996). Time constraints and multiple choice criteria in the sampling behaviour and mate choice of the fiddler crab, Uca annulipes. Behav. Ecol. Sociobiol. 38, 407–416. doi: 10.1007/s002650050258
Bailey, W. J., Ager, E. I., O’Brien, E. K., and Watson, D. L. (2003). Searching by visual and acoustic cues among bushcrickets (Orthoptera: Tettigoniidae): will females remain faithful to a male who stops calling? Physiol. Entomol. 28, 209–214. doi: 10.1046/j.1365-3032.2003.00334.x
Barth, F. G. (2000). How to catch the wind: spider hairs specialized for sensing the movement of air. Naturwissenschaften 87, 51–58. doi: 10.1007/s001140050010
Barth, F. G., and Bohnenberger, J. (1978). Lyriform slit sense organ: thresholds and stimulus amplitude ranges in a multi-unit mechanoreceptor. J. Comp. Physiol. 125, 37–43. doi: 10.1007/BF00656829
Barth, F. G., and Holler, A. (1999). Dynamics of arthropod filiform hairs. V. The response of spider trichobothria to natural stimuli. Philos. Trans. Biol. Sci. 354, 183–192.
Barth, F. G., Humphrey, J. A. C., Wastl, U., Halbritter, J., and Brittinger, W. (1995). Dynamics of arthropod filiform hairs. III. Flow patterns related to air movement detection in a spider (Cupiennius salei KEYS.). Philos. Trans. R. Soc. Lond. B Biol. Sci. 347, 397–412. doi: 10.1098/rstb.1995.0032
Barth, F. G., Wastl, U., Humphrey, J. A. C., and Devarakonda, R. (1993). Dynamics of arthropod filiform hairs. II. Mechanical properties of spider trichobothria (Cupiennius salei Keys.). Philos. Trans. R. Soc. Lond. B Biol. Sci. 340, 445–461. doi: 10.1098/rstb.1993.0084
Baruffaldi, L., and Costa, F. G. (2010). Changes in male sexual responses from silk cues of females at different reproductive states in the wolf spider Schizocosa malitiosa. J. Ethol. 28, 75–85. doi: 10.1007/s10164-009-0158-8
Boekhoff-Falk, G. (2005). Hearing in Drosophila: development of Johnston’s organ and emerging parallels to vertebrate ear development. Dev. Dyn. 232, 550–558. doi: 10.1002/dvdy.20207
Breithaupt, T. (2002). “Sound perception in aquatic crustaceans,” in The Crustacean Nervous System, ed. K. Wiese (Berlin: Springer), 548–558. doi: 10.1007/978-3-662-04843-6_41
Brownell, P. H. (1988). Properties and functions of the pectin chemosensory system of scorpions. Chem. Senses 13:677.
Brownell, P. H., and Farley, R. D. (1974). The organization of the malleolar sensory system in the solpugid, Chanbria sp. Tissue Cell 6, 471–485.
Brumm, H., and Zollinger, S. A. (2017). Vocal plasticity in a reptile. Proc. R. Soc. B Biol. Sci. 284:20170451. doi: 10.1098/rspb.2017.0451
Budelmann, B. U. (1992). “Hearing in nonarthropod invertebrates,” in The Evolutionary Biology of Hearing, eds D. B. Webster, A. N. Popper, and R. R. Fay (New York, NY: Springer), 141–155. doi: 10.1007/978-1-4612-2784-7_10
Byers, J., Hebets, E., and Podos, J. (2010). Female mate choice based upon male motor performance. Anim. Behav. 79, 771–778. doi: 10.1016/j.anbehav.2010.01.009
Caldwell, M. S., Johnston, G. R., McDaniel, J. G., and Warkentin, K. M. (2010). Vibrational signaling in the agonistic interactions of red-eyed treefrogs. Curr. Biol. 20, 1012–1017. doi: 10.1016/j.cub.2010.03.069
Chittka, L., Skorupski, P., and Raine, N. E. (2009). Speed–accuracy tradeoffs in animal decision making. Trends Ecol. Evol. 24, 400–407. doi: 10.1016/j.tree.2009.02.010
Choi, N., Bern, M., Elias, D. O., McGinley, R. H., Rosenthal, M. F., and Hebets, E. A. (2019). A mismatch between signal transmission efficacy and mating success calls into question the function of complex signals. Anim. Behav. 158, 77–88. doi: 10.1016/j.anbehav.2019.09.017
Choi, N., and Hebets, E. A. (2021). The effects of conspecific male density on the reproductive behavior of male Schizocosa retrorsa (Banks, 1911) wolf spiders (Araneae: Lycosidae). J. Arachnol. 49, 347–357. doi: 10.1636/JoA-S-20-079
Corben, H. C. (1983). Wing-beat frequencies, wing-areas and masses of flying insects and hummingbirds. J. Theor. Biol. 102, 611–623. doi: 10.1016/0022-5193(83)90394-6
de Jong, K., Amorim, M. C. P., Fonseca, P. J., and Heubel, K. U. (2018). Noise affects multimodal communication during courtship in a marine fish. Front. Ecol. Evol. 6:113. doi: 10.3389/fevo.2018.00113
DeVoe, R. D. (1972). Dual sensitivities of cells in wolf spider eyes at ultraviolet and visible wavelengths of light. J. Gen. Physiol. 59, 247–269. doi: 10.1085/jgp.59.3.247
DeVoe, R. D., Small, R. J. W., and Zvargulis, J. E. (1969). Spectral sensitivities of wolf spider eyes. J. Gen. Physiol. 54, 1–32. doi: 10.1085/jgp.54.1.1
Diaz Pauli, B., and Lindström, K. (2021). Trade-off between mate choice speed and decision accuracy under mating competition in female sand gobies. J. Ethol. 39, 55–64. doi: 10.1007/s10164-020-00673-z
Eakin, R. M. (1972). “Structure of invertebrate photoreceptors,” in Photochemistry of Vision, Vol. 7, ed. H. J. A. Dartnall (Berlin: Springer), 625–684. doi: 10.1007/978-3-642-65066-6_16
Elias, D. O., Maddison, W. P., Peckmezian, C., Girard, M. B., and Mason, A. C. (2012). Orchestrating the score: complex multimodal courtship in the Habronattus coecatus group of Habronattus jumping spiders (Araneae: Salticidae): MULTIMODAL COURTSHIP IN HABRONATTUS. Biol. J. Linn. Soc. 105, 522–547. doi: 10.1111/j.1095-8312.2011.01817.x
Elias, D. O., Mason, A. C., and Hoy, R. R. (2004). The effect of substrate on the efficacy of seismic courtship signal transmission in the jumping spider Habronattus dossenus (Araneae: Salticidae). J. Exp. Biol. 207, 4105–4110. doi: 10.1242/jeb.01261
Elias, D. O., Mason, A. C., Maddison, W. P., and Hoy, R. R. (2003). Seismic signals in a courting male jumping spider (Araneae: Salticidae). J. Exp. Biol. 206, 4029–4039. doi: 10.1242/jeb.00634
Flanigan, K. A. S., Wiegmann, D. D., Hebets, E. A., and Bingman, V. P. (2021). Multisensory integration supports configural learning of a home refuge in the whip spider Phrynus marginemaculatus. J. Exp. Biol. 224:jeb238444. doi: 10.1242/jeb.238444
Fowler-Finn, K. D., and Hebets, E. A. (2006). An examination of agonistic interactions in the whip spider Phrynus marginemaculatus (Arachnida, Amblypygi). J. Arachnol. 34, 62–76. doi: 10.1636/S04-104.1
Friedel, T., and Barth, F. G. (1997). Wind-sensitive interneurones in the spider CNS (Cupiennius salei): directional information processing of sensory inputs from trichobothria on the walking legs. J. Comp. Physiol. A 180, 223–233. doi: 10.1007/s003590050043
Gibson, G., and Russell, I. (2006). Flying in tune: sexual recognition in mosquitoes. Curr. Biol. 16, 1311–1316. doi: 10.1016/j.cub.2006.05.053
Gomes, A. C. R., Funghi, C., Soma, M., Sorenson, M. D., and Cardoso, G. C. (2017). Multimodal signalling in estrildid finches: song, dance and colour are associated with different ecological and life-history traits. J. Evol. Biol. 30, 1336–1346. doi: 10.1111/jeb.13102
Görner, P., and Andrews, P. (1969). Trichobothrien, ein Ferntastsinnesorgan bei Webespinnen (Araneen). Z. Vgl. Physiol. 64, 301–317. doi: 10.1007/BF00340548
Grafe, T. U., Preininger, D., Sztatecsny, M., Kasah, R., Dehling, J. M., Proksch, S., et al. (2012). Multimodal communication in a noisy environment: a case study of the Bornean rock frog Staurois parvus. PLoS One 7:e37965. doi: 10.1371/journal.pone.0037965
Halfwerk, W., Varkevisser, J., Simon, R., Mendoza, E., Scharff, C., and Riebel, K. (2019). Toward testing for multimodal perception of mating signals. Front. Ecol. Evol. 7:124. doi: 10.3389/fevo.2019.00124
Harland, D. P., and Jackson, R. R. (2002). Influence of cues from the anterior medial eyes of virtual prey on Portia fimbriata, an araneophagic jumping spider. J. Exp. Biol. 205, 1861–1868. doi: 10.1242/jeb.205.13.1861
Hayek, S. I. (1990). Mathematical modeling of absorbent highway noise barriers. Appl. Acoust. 31, 77–100. doi: 10.1016/0003-682X(90)90054-X
Hebets, E. A. (2005). Attention-altering signal interactions in the multimodal courtship display of the wolf spider Schizocosa uetzi. Behav. Ecol. 16, 75–82. doi: 10.1093/beheco/arh133
Hebets, E. A. (2011). Current status and future directions of research in complex signaling. Curr. Zool. 57, i–v. doi: 10.1093/czoolo/57.2.i
Hebets, E. A., Bern, M., McGinley, R. H., Roberts, A., Kershenbaum, A., Starrett, J., et al. (2021). Sister species diverge in modality-specific courtship signal form and function. Ecol. Evol. 11, 852–871. doi: 10.1002/ece3.7089
Hebets, E. A., Elias, D. O., Mason, A. C., Miller, G. L., and Stratton, G. E. (2008). Substrate-dependent signalling success in the wolf spider, Schizocosa retrorsa. Anim. Behav. 75, 605–615. doi: 10.1016/j.anbehav.2007.06.021
Hebets, E. A., and McGinley, R. H. (2019). “Multimodal signaling,” in Encyclopedia of Animal Behavior, eds J. Moore and M. Breed (Amsterdam: Elsevier), 487–499. doi: 10.1016/B978-0-12-809633-8.90730-1
Hebets, E. A., and Papaj, D. R. (2005). Complex signal function: developing a framework of testable hypotheses. Behav. Ecol. Sociobiol. 57, 197–214. doi: 10.1007/s00265-004-0865-7
Hebets, E. A., Stafstrom, J. A., Rodriguez, R. L., and Wilgers, D. J. (2011). Enigmatic ornamentation eases male reliance on courtship performance for mating success. Anim. Behav. 81, 963–972. doi: 10.1016/j.anbehav.2011.01.023
Hebets, E. A., Stratton, G. E., and Miller, G. L. (1996). Habitat and courtship behavior of the wolf spider Schizocosa retrorsa (Banks) (Araneae, Lycosidae). J. Arachnol. 24, 141–147.
Hebets, E. A., Vink, C. J., Sullivan-Beckers, L., and Rosenthal, M. F. (2013). The dominance of seismic signaling and selection for signal complexity in Schizocosa multimodal courtship displays. Behav. Ecol. Sociobiol. 67, 1483–1498. doi: 10.1007/s00265-013-1519-4
Heidelbach, J., and Dambach, M. (1997). Wing-flick signals in the courtship of the African cave cricket, Phaeophilacris spectrum. Ethology 103, 827–843. doi: 10.1111/j.1439-0310.1997.tb00124.x
Heidelbach, J., Dambach, M., and Bohm, H. (1991). Processing wing flick-generated air-vortex signals in the African cave cricket Phaeophilacris spectrum. Naturwissenschaften 786, 277–278.
Heuschele, J., Mannerla, M., Gienapp, P., and Candolin, U. (2009). Environment-dependent use of mate choice cues in sticklebacks. Behav. Ecol. 20, 1223–1227. doi: 10.1093/beheco/arp123
Higham, J. P., and Hebets, E. A. (2013). An introduction to multimodal communication. Behav. Ecol. Sociobiol. 67, 1381–1388. doi: 10.1007/s00265-013-1590-x
Humphrey, J. A. C., and Barth, F. G. (2007). Medium flow-sensing hairs: biomechanics and models. Adv. Insect Physiol. 34, 1–80. doi: 10.1016/S0065-2806(07)34001-0
Hüttner, T., Fersen, L., Miersch, L., Czech, N. U., and Dehnhardt, G. (2022). Behavioral and anatomical evidence for electroreception in the bottlenose dolphin (Tursiops truncatus). Anat. Rec. 305, 592–608. doi: 10.1002/ar.24773
Igelmund, P. (1987). Morphology, sense organs, and regeneration of the forelegs (whips) of the whip spider Heterophrynus elaphus (Arachnida, Amblypygi). J. Morphol. 193, 75–89. doi: 10.1002/jmor.1051930108
Jacobsen, F. (2007). “Sound intensity,” in Springer Handbook of Acoustics, ed. T. D. Rossing (New York, NY: Springer), 1093–1114. doi: 10.1007/978-1-4939-0755-7_25
Johnston, C. (1855). Original communications: auditory apparatus of the Culex mosquito. J. Cell Sci. s1-s3, 97–102. doi: 10.1242/jcs.s1-3.10.97
Knowlton, E. D., and Gaffin, D. D. (2019). Female wolf spider, Schizocosa avida1, vibration receptor responses to male courtship. Southwest. Entomol. 44, 213–228. doi: 10.3958/059.044.0124
Kunc, H. P., Lyons, G. N., Sigwart, J. D., McLaughlin, K. E., and Houghton, J. D. R. (2014). Anthropogenic Noise Affects Behavior Across Sensory Modalities (Version 1, p. 45568 bytes) [Data set]. Dryad. Available online at: https://www.journals.uchicago.edu/doi/10.1086/677545
Land, M. F. (1969). Structure of the retinae of the principal eyes of jumping spiders (Salticidae: Dendryphantinae) in relation to visual optics. J. Exp. Biol. 51, 443–470. doi: 10.1242/jeb.51.2.443
Lasbleiz, C., Ferveur, J.-F., and Everaerts, C. (2006). Courtship behaviour of Drosophila melanogaster revisited. Anim. Behav. 72, 1001–1012. doi: 10.1016/j.anbehav.2006.01.027
Manabe, K., Sadr, E. I., and Dooling, R. J. (1998). Control of vocal intensity in budgerigars (Melopsittacus undulatus): differential reinforcement of vocal intensity and the Lombard effect. J. Acoust. Soc. Am. 103, 1190–1198. doi: 10.1121/1.421227
Manshor, Z., and Augustine Gawin, D. F. (2020). Call types of the oriental magpie robin (Copsychus saularis) in suburban areas in Kota Samarahan, Sarawak. Borneo J. Resour. Sci. Technol. 10, 37–44. doi: 10.33736/bjrst.2264.2020
Markl, H., and Tautz, J. (1975). The sensitivity of hair receptors in caterpillars of Barathra brassicae L. (Lepidoptera, Noctuidae) to particle movement in a sound field. J. Comp. Physiol. A 99, 79–87. doi: 10.1007/BF01464713
Marshall, J., Cronin, T. W., and Kleinlogel, S. (2007). Stomatopod eye structure and function: a review. Arthropod Struct. Dev. 36, 420–448. doi: 10.1016/j.asd.2007.01.006
Marshall, N. J. (1988). A unique colour and polarization vision system in mantis shrimps. Nature 333, 557–560. doi: 10.1038/333557a0
Masters, W. M., Raver, K. A. S., and Kazial, K. A. (1995). Sonar signals of big brown bats, Eptesicus fuscus, contain information about individual identity, age and family affiliation. Anim. Behav. 50, 1243–1260. doi: 10.1016/0003-3472(95)80041-7
McMeniman, C. J., Corfas, R. A., Matthews, B. J., Ritchie, S. A., and Vosshall, L. B. (2014). Multimodal integration of carbon dioxide and other sensory cues drives mosquito attraction to humans. Cell 156, 1060–1071. doi: 10.1016/j.cell.2013.12.044
Menda, G., Nitzany, E. I., Shamble, P. S., Wells, A., Harrington, L. C., Miles, R. N., et al. (2019). The long and short of hearing in the mosquito Aedes aegypti. Curr. Biol. 29, 709–714.e4. doi: 10.1016/j.cub.2019.01.026
Munoz, N. E., and Blumstein, D. T. (2012). Multisensory perception in uncertain environments. Behav. Ecol. 23, 457–462. doi: 10.1093/beheco/arr220
Nakano, R., Takanashi, T., Fujii, T., Skals, N., Surlykke, A., and Ishikawa, Y. (2009). Moths are not silent, but whisper ultrasonic courtship songs. J. Exp. Biol. 212, 4072–4078. doi: 10.1242/jeb.032466
Nolfo, A. P., Casetta, G., and Palagi, E. (2021). Visual communication in social play of a hierarchical carnivore species: the case of wild spotted hyenas. Curr. Zool. 2021:zoab076. doi: 10.1093/cz/zoab076
Partan, S., and Marler, P. (1999). Communication goes multimodal. Science 283, 1272–1273. doi: 10.1126/science.283.5406.1272
Raboin, M., and Elias, D. O. (2019). Anthropogenic noise and the bioacoustics of terrestrial invertebrates. J. Exp. Biol. 222:jeb178749. doi: 10.1242/jeb.178749
Rinberg, D., and Davidowitz, H. (2000). Do cockroaches ‘know’ about fluid dynamics? Nature 405, 756–756. doi: 10.1038/35015677
Roberts, J. A., and Uetz, G. W. (2005). Information content of female chemical signals in the wolf spider, Schizocosa ocreata: male discrimination of reproductive state and receptivity. Anim. Behav. 70, 217–223. doi: 10.1016/j.anbehav.2004.09.026
Roian Egnor, S. E., and Hauser, M. D. (2006). Noise-induced vocal modulation in cotton-top tamarins (Saguinus oedipus). Am. J. Primatol. 68, 1183–1190. doi: 10.1002/ajp.20317
Rosenthal, M. F., and Hebets, E. A. (2012). Resource heterogeneity interacts with courtship rate to influence mating success in the wolf spider Schizocosa floridana. Anim. Behav. 84, 1341–1346. doi: 10.1016/j.anbehav.2012.08.028
Rowe, C., and Guilford, T. (1996). Hidden colour aversions in domestic chicks triggered by pyrazine odours of insect warning displays. Nature 383, 520–522. doi: 10.1038/383520a0
Rundus, A. S., Biemuller, R., DeLong, K., Fitzgerald, T., and Nyandwi, S. (2015). Age-related plasticity in male mate choice decisions by Schizocosa retrorsa wolf spiders. Anim. Behav. 107, 233–238. doi: 10.1016/j.anbehav.2015.06.020
Rundus, A. S., Owings, D. H., Joshi, S. S., Chinn, E., and Giannini, N. (2007). Ground squirrels use an infrared signal to deter rattlesnake predation. Proc. Natl. Acad. Sci. U.S.A. 104, 14372–14376. doi: 10.1073/pnas.0702599104
Rundus, A. S., Santer, R. D., and Hebets, E. A. (2010). Multimodal courtship efficacy of Schizocosa retrorsa wolf spiders: implications of an additional signal modality. Behav. Ecol. 21, 701–707. doi: 10.1093/beheco/arq042
Santer, R. D., and Hebets, E. A. (2008). Agonistic signals received by an arthropod filiform hair allude to the prevalence of near-field sound communication. Proc. R. Soc. B Biol. Sci. 275, 363–368. doi: 10.1098/rspb.2007.1466
Santer, R. D., and Hebets, E. A. (2011). Evidence for air movement signals in the agonistic behaviour of a nocturnal arachnid (Order Amblypygi). PLoS One 6:e22473. doi: 10.1371/journal.pone.0022473
Schaub, A., Ostwald, J., and Siemers, B. M. (2008). Foraging bats avoid noise. J. Exp. Biol. 211, 3174–3180. doi: 10.1242/jeb.022863
Scheffer, S. J., Uetz, G. W., and Stratton, G. E. (1996). Sexual selection, male morphology, and the efficacy of courtship signalling in two wolf spiders (Araneae: Lycosidae). Behav. Ecol. Sociobiol. 38, 17–23. doi: 10.1007/s002650050212
Shamble, P. S., Menda, G., Golden, J. R., Nitzany, E. I., Walden, K., Beatus, T., et al. (2016). Airborne acoustic perception by a jumping spider. Curr. Biol. 26, 2913–2920. doi: 10.1016/j.cub.2016.08.041
Shannon, G., McKenna, M. F., Angeloni, L. M., Crooks, K. R., Fristrup, K. M., Brown, E., et al. (2016). A synthesis of two decades of research documenting the effects of noise on wildlife: effects of anthropogenic noise on wildlife. Biol. Rev. 91, 982–1005. doi: 10.1111/brv.12207
Shimozawa, T., and Kanou, M. (1984). Varieties of filiform hairs: range fractionation by sensory afferents and cereal interneurons of a cricket. J. Comp. Physiol. A 155, 485–493. doi: 10.1007/BF00611913
Shimozawa, T., Murakami, J., and Kumagai, T. (2003). “Cricket wind receptors: thermal noise for the highest sensitivity known,” in Sensors and Sensing in Biology and Engineering, eds F. G. Barth, J. A. C. Humphrey, and T. W. Secomb (Vienna: Springer), 145–157. doi: 10.1007/978-3-7091-6025-1_10
Siefkes, M. J., Winterstein, S. R., and Li, W. (2005). Evidence that 3-keto petromyzonol sulphate specifically attracts ovulating female sea lamprey, Petromyzon marinus. Anim. Behav. 70, 1037–1045. doi: 10.1016/j.anbehav.2005.01.024
Slabbekoorn, H., and Peet, M. (2003). Birds sing at a higher pitch in urban noise. Nature 424, 267–267. doi: 10.1038/424267a
Smith, C. L., Taylor, A., and Evans, C. S. (2011). Tactical multimodal signalling in birds: facultative variation in signal modality reveals sensitivity to social costs. Anim. Behav. 82, 521–527. doi: 10.1016/j.anbehav.2011.06.002
Spieth, H. T. (1974). Courtship behavior in Drosophila. Annu. Rev. Entomol. 19, 385–405. doi: 10.1146/annurev.en.19.010174.002125
Stafstrom, J. A., Menda, G., Nitzany, E. I., Hebets, E. A., and Hoy, R. R. (2020). Ogre-faced, net-casting spiders use auditory cues to detect airborne prey. Curr. Biol. 30, 5033–5039.e3. doi: 10.1016/j.cub.2020.09.048
Starnberger, I., Preininger, D., and Hödl, W. (2014). From uni- to multimodality: towards an integrative view on anuran communication. J. Comp. Physiol. A 200, 777–787. doi: 10.1007/s00359-014-0923-1
Starrett, J., McGinley, R. H., Hebets, E. A., and Bond, J. E. (2022). Phylogeny and secondary sexual trait evolution in Schizocosa wolf spiders (Araneae, Lycosidae) shows evidence for multiple gains and losses of ornamentation and species delimitation uncertainty. Mol. Phylogenet. Evol. 169:107397. doi: 10.1016/j.ympev.2022.107397
Stratton, G. E. (2005). Evolution of ornamentation and courtship behavior in Schizocosa: insights from a phylogeny based on morphology (Araneae, Lycosidae). J. Arachnol. 33, 347–376.
Suter, R. B. (2003). Trichobothrial mediation of an aquatic escape response: directional jumps by the fishing spider, Dolomedes triton, foil frog attacks. J. Insect Sci. 3:19. doi: 10.1673/031.003.1901
Talotte, C., Gautier, P.-E., Thompson, D. J., and Hanson, C. (2003). Identification, modelling and reduction potential of railway noise sources: a critical survey. J. Sound Vib. 267, 447–468. doi: 10.1016/S0022-460X(03)00707-7
Tauber, E., and Eberl, D. F. (2003). Acoustic communication in Drosophila. Behav. Processes 64, 197–210. doi: 10.1016/S0376-6357(03)00135-9
Taylor, R. C., Wilhite, K. O., Ludovici, R. J., Mitchell, K. M., Halfwerk, W., Page, R. A., et al. (2021). Complex sensory environments alter mate choice outcomes. J. Exp. Biol. 224:jeb.233288. doi: 10.1242/jeb.233288
Teeter, J. (1980). Pheromone communication in sea lampreys (Petromyzon marinus): implications for population management. Can. J. Fish. Aquat. Sci. 37, 2123–2132. doi: 10.1139/f80-254
Thiagarajan, D., and Sachse, S. (2022). Multimodal information processing and associative learning in the insect brain. Insects 13:332. doi: 10.3390/insects13040332
Tsujiuchi, S., Sivan-Loukianova, E., Eberl, D. F., Kitagawa, Y., and Kadowaki, T. (2007). Dynamic range compression in the honey bee auditory system toward waggle dance sounds. PLoS One 2:e234. doi: 10.1371/journal.pone.0000234
Uetz, G. W., Clark, D. L., and Roberts, J. A. (2016). Multimodal communication in wolf spiders (Lycosidae)—an emerging model for study. Adv. Study Behav. 48, 117–159. doi: 10.1016/bs.asb.2016.03.003
Uetz, G. W., and Roberts, J. A. (2002). Multisensory cues and multimodal communication in spiders: insights from video/audio playback studies. Brain Behav. Evol. 59, 222–230. doi: 10.1159/000064909
Vaccaro, R., Uetz, G. W., and Roberts, J. A. (2010). Courtship and mating behavior of the wolf spider Schizocosa bilineata (Araneae: Lycosidae). J. Arachnol. 38, 452–459. doi: 10.1636/Hi09-115.1
Walcott, C. (1969). A spider’s vibration receptor: its anatomy and physiology. Am. Zool. 9, 133–144.
Wilder, S. M., DeVito, J., Persons, M. H., and Rypstra, A. L. (2005). The effects of moisture and heat on the efficacy of chemical cues used in predator detection by the wolf spider Pardosa milvina (Araneae, Lycosidae). J. Arachnol. 33, 857–861. doi: 10.1636/S03-64.1
Wolf, H. (2017). Scorpions pectines – idiosyncratic chemo- and mechanosensory organs. Arthropod Struct. Dev. 46, 753–764. doi: 10.1016/j.asd.2017.10.002
Keywords: wolf spider, Schizocosa retrorsa, mating success, near-field sound, multimodal signaling, signaling environment, behavioral plasticity, environmental noise
Citation: Kundu P, Choi N, Rundus AS, Santer RD and Hebets EA (2022) Uncovering ‘Hidden’ Signals: Previously Presumed Visual Signals Likely Generate Air Particle Movement. Front. Ecol. Evol. 10:939133. doi: 10.3389/fevo.2022.939133
Received: 08 May 2022; Accepted: 16 June 2022;
Published: 05 July 2022.
Edited by:
Eunice Jingmei Tan, Yale-NUS College, SingaporeReviewed by:
Brent Stoffer, University of Cincinnati, United StatesCaroline Fabre, University of Cambridge, United Kingdom
Copyright © 2022 Kundu, Choi, Rundus, Santer and Hebets. This is an open-access article distributed under the terms of the Creative Commons Attribution License (CC BY). The use, distribution or reproduction in other forums is permitted, provided the original author(s) and the copyright owner(s) are credited and that the original publication in this journal is cited, in accordance with accepted academic practice. No use, distribution or reproduction is permitted which does not comply with these terms.
*Correspondence: Eileen A. Hebets, ZWhlYmV0czJAdW5sLmVkdQ==