- 1Departamento de Ecología Evolutiva, Instituto de Ecología, Universidad Nacional Autónoma de México, Mexico City, Mexico
- 2Posgrado en Ciencias Biológicas, Universidad Nacional Autónoma de México, Mexico City, Mexico
- 3Instituto de Biociencias, Universidad Autónoma de Chiapas, Tapachula, Mexico
- 4Departamento de Ecología Funcional, Instituto de Ecología, Universidad Nacional Autónoma de México, Mexico City, Mexico
- 5Consejo Nacional de Ciencia y Tecnología, Mexico City, Mexico
- 6Comisión Nacional para el Conocimiento y Uso de la Biodiversidad, Mexico City, Mexico
The Mexican fruit fly, Anastrepha ludens, is an important pest that causes widespread damage to a number of fruit crops in Mexico. The sterile insect technique (SIT) is commonly used for its control. However, the existence of natural barriers can give rise to a population structure in neutral loci and possibly behavioral or adaptive traits that interfere with SIT. For this reason, it is important to understand the genetic diversity and structure of A. ludens populations and to better understand the evolutionary ecology and population processes in view of possible expansions and possible host shifts due to climate change. We genotyped nine nuclear DNA (nDNA) microsatellite loci among fruit fly populations collected from five biogeographic areas within Mexico, namely, the Mexican Plateau, the Northeastern Coastal Plain, the Pacific Coast, the Gulf Coast of Mexico, and the Soconusco, and a laboratory strain. The nuclear genetic diversity was moderate (from He = 0.34 to He = 0.39) within the wild mexfly population. We found that populations were clustered in three genetic groups (K = 3). The diversity and the genetic structure of A. ludens are determined by environmental and geological conditions, as well as local conditions like anthropogenic perturbation, which would produce population expansion and the existence of possible predators that would affect the population density. Gene flow showed recent migration among populations. The laboratory strain showed fewer diversity than the wild samples. Large values of current and ancestral population size suggest high resistance to climatic changes, probably due to biological attributes, such as its polyphagous, multivoltine, and high dispersal characteristics. In particular, ecosystem fragmentation and perturbation as well as the existence of new plant hosts would probably increase the abundance of flies.
Introduction
The patterns of genetic variation in wild populations are the consequence of changes over time and space determined by the combined effect of evolutionary forces such as genetic drift, gene flow, and selection (Nielsen and Slatkin, 2013). Understanding these patterns is particularly important for planning management strategies to control invasive and pathogenic species, such as insect pests (Krafsur, 2005).
Fruit flies of the genus Anastrepha (Diptera: Tephritidae) are the most harmful pest of commercial fruit crops and comprise more than 300 currently recognized species distributed in the tropical American continent (Norrbom et al., 1999; Norrbom and Korytkowski, 2012, 2009). In Mexico, 37 species of economic importance have been described, including Anastrepha obliqua, Anastrepha serpentina, Anastrepha striata, Anastrepha fraterculus, and Anastrepha ludens (Hernandez-Ortiz and Aluja, 1993; Aluja, 1994; Hernandez-Ortiz, 2007). These species cause losses of up to $710 million per year (Reyes et al., 2000; Enkerlin, 2005).
In this study, we focus on A. ludens (Loew), known as the Mexican fruit fly (mexfly), which is widely distributed in Mexico and Central America (Hernandez-Ortiz and Aluja, 1993; Foote, 1994). Female flies harness fruits as oviposition substrate and develop larvae causing damage to up to 60 varieties of fruits, such as citrus, mangoes, peach, guava, sapodilla fruits, and hot peppers (Hernández-Ortíz, 1992; Hernandez-Ortiz and Aluja, 1993; Aluja, 1994; Thomas, 2004).
The sterile insect technique (SIT) is a method currently used to control A. ludens populations. The SIT involves the production of sterile male flies whose sterility is activated by gamma radiation. These flies are then released into infested areas. Sterile males mate with wild females, who then generate infertile eggs (Klassen and Curtis, 2005; Vreysen, 2005; Pérez-Staples et al., 2021). The sterilization of males of different species of flies does not affect the biology of the insect (Walder and Calkins, 1993; Rull and Barreda-Landa, 2007; Mahmoud, 2010; Panduranga et al., 2022). In the field, it is a method that allows reducing the natural population affecting the population dynamics. However, concerns about SIT effectiveness remain. For example, a sterile laboratory fly colony established in Mexico resulted in the loss of fertility among native flies (Orozco-Dávila et al., 2007). Similarly, the colony establishment must include specimens with broad genetic diversity (Parker et al., 2021). Also, the “degradation” of laboratory stocks occurs via adaptation by selection and inbreeding, with a loss of field competitiveness (Krafsur and Ouma, 2021). Predating pressure is absent in colonies, and sexual selection that operates in nature may be relaxed during laboratory adaptation (Krafsur and Ouma, 2021). Inbreeding causes a loss of genetic variation that could, in principle, reduce competitiveness, and a substantial loss of heterozygosity may occur if a release strain is formed from too few founding insects, followed by a prolonged “bottleneck” in colony size (Krafsur and Ouma, 2021). This could also be related to the loss of competitiveness of mexfly males in the field due to the artificial selection of mass-reared flies. The SIT premise supposes that there is a high genetic similarity between wild and laboratory strains that allow mating. However, sterile males usually have lower mating success with wild females compared with wild males (Pérez-Staples et al., 2013). After many decades of mass reproduction in captivity, the success of this approach has decreased because of mating incompatibility, low competitiveness in reproductive behavior (sexual selection and courtship patterns), and shorter longevity of the sterile versus the wild males (Rull et al., 2005; Rull and Barreda-Landa, 2007). Also, population isolation, such as mass rearing, leads to the appearance of homozygous individuals and inbreeding depression, which in turn causes inefficient mating (Alberti et al., 2002; Rull and Barreda-Landa, 2007). The loss of genetic variation in mass-reared insects may also reflect the loss of the wild genotypes and the loss of their natural vigor under laboratory conditions (Zygouridis et al., 2014). Furthermore, management strategies probably affect the SIT control method in a negative way by diminishing genetic variation (Dupuis et al., 2019; Ruiz-Arce et al., 2019). This impact could be more important for a species with large population sizes and this is distributed in a wide geographic area (e.g., Shi et al., 2005).
Molecular analyses based on population genetics studies on A. ludens using mitochondrial sequence polymorphisms (Ruiz-Arce et al., 2015), biochemical markers such as isoenzymes and molecular dominant amplified fragment length polymorphism (AFLP) markers (Malavasi and Morgante, 1982; Molina-Nery et al., 2014; Pecina-Quintero et al., 2020, 2009; Ruiz-Montoya et al., 2020), and single-nucleotide polymorphisms (SNPs) throughout the genome (Dupuis et al., 2019) have contributed to the understanding of the origin of mexfly populations and indicate mixed results stemming from the extent of the geographic range analyzed and in a lesser degree from the genetic markers (dominant–codominant) employed to survey populations. Particularly, at a small scale, AFLP markers detect very low (Nuevo León, Tamaulipas; Pecina-Quintero et al., 2009) or high genetic structure (Veracruz, Nuevo León, and Tamaulipas; Pecina-Quintero et al., 2020). When using biochemical markers such as allozymes and surveying seven populations in the different States of Mexico, with different climates and vegetation types, low genetic structure for A. ludens was detected (Molina-Nery et al., 2014). In contrast, populations of this species in Chiapas, Mexico, show high genetic variation and low genetic structure between localities and moderate structure when populations are grouped by host plant used (Ruiz-Montoya et al., 2020). The latter results are in agreement with the findings of Ruiz-Arce et al. (2015), they performed the most comprehensive phylogeographic study on the genetic structure of A. ludens to date, with two mitochondrial regions (COI and ND6) covering 67 populations along the whole range of the species and collections from the northern and southern Mexican territory to Central America. Ruiz-Arce et al. (2015), found a low genetic structure between the two major groups (north south) divided by the Tehuantepec Isthmus, accounting ca. 6% of genetic variance, and significant genetic variance within groups (ca. 25%). Furthermore, this study supports a Southern Mexico/Central American origin for A. ludens (Ruiz-Arce et al., 2015). However, SNP analyses of the same populations did not detect differences in genetic diversity between populations of Central America and others, but they did detect a higher level of genetic structuring (FST = 0.09). With these markers, three main genetic clusters were identified, namely, west Mexico, east Mexico/Texas, and Isthmian Central America; high divergence in the studied strains of A. ludens and an explicit biogeographic analysis are suggested to identify the ancestral range of A. ludens (Dupuis et al., 2019). However, further molecular analyses to determine the diversity and the structure based on nuclear microsatellites may be used to better understand the dynamics of mexfly populations.
During the past decade, nuclear microsatellite tools developed for Anastrepha species have increased exponentially (Boykin et al., 2010; Islam et al., 2011; Lanzavecchia et al., 2014; Manni et al., 2015; Ruiz-Arce et al., 2019). Microsatellites are polymorphic DNAs that comprise sequences of repeated nucleotides; usually, the repeated units are composed of two to six nucleotides (motifs) (Hancock, 1999). The number of repeated units in a specific locus may differ, thus constituting alleles. Microsatellites are codominant, a relevant property that makes these markers suitable for population genetics studies, such as, genetic structure, gene flow, and genetic relationships between populations. Microsatellites are distributed along the whole genome of organisms, and although it seems that they are not strictly randomly distributed, they are present in coding and noncoding sequences. Theoretically, microsatellites are expected to have lower occurrence in coding regions due to purifying selection (see Carneiro-Vieira et al., 2016 and references therein). Although microsatellites have higher rates of mutation than other genetic markers, variations between loci and alleles within a locus have been documented (Jin et al., 1996; Carneiro-Vieira et al., 2016). Short-length microsatellite repeats appear to have lower mutation rates (Schug et al., 1997). The high polymorphism level of microsatellites makes them more suitable than other genetic markers (Carneiro-Vieira et al., 2016). Relative reproducibility in different laboratories and high-throughput genotyping (Barker, 2002; Selkoe and Toonen, 2006) have proven to be very useful to study populations. Specifically, microsatellite markers have been designed for A. obliqua, and microsatellite amplification in A. ludens DNA has been successfully tested (Islam et al., 2011).
The correlation of genetic variation with the geographic distribution of A. ludens populations has not been explored in detail. In this study, we analyzed A. ludens populations from five biogeographic areas within Mexico, namely, the Mexican Plateau, the Northeastern Coastal Plain, the Pacific Coast, the Gulf Coast of Mexico, and Soconusco, as well as a laboratory strain (25 years old = 152 generations, used for developing SIT). We used nine selected microsatellite markers to achieve the following three goals, (i) to investigate the genetic structure of the A. ludens population from different biogeographic areas in Mexico; (ii) to analyze if wild A. ludens populations from Mexico are genetically different from the laboratory strain; and (iii) to propose recommendations for pest management. We hypothesized that the population structure among A. ludens populations would be influenced by climate and the biogeographic characteristics of its distributional area. Additionally, we expect lower diversity in laboratory strain samples than in wild population samples.
Materials and methods
Sampling and genomic DNA extraction
We collected A. ludens adults from five biogeographic provinces that included the Mexican Plateau (MP), the Northeastern Coastal Plain (NCP), the Pacific Coast (PC), the Gulf Coast of Mexico (GC), and the Soconusco (SOC). These areas have been defined using the proposal by Rzedowski (1978), and it is based on plant species composition in different localities (see Supplementary Table 1). Additionally, a laboratory strain (LAB) of ∼25 years equivalent to 152 generations, currently in production and being used by the mass rearing facility (Orozco-Dávila et al., 2007, 2017), was used as a reference group to analyze the levels of genetic variation and inbreeding. The specimens were provided by the Department of Colonization and Breeding of Fruit Flies, the Development Methods of the Moscafrut Program (Metapa de Domínguez, Chiapas), and the Instituto de Ecología-INECOL (Xalapa, Veracruz, México). Flies were sorted for each locality (10 insects of each sex, 20 from each locality). In total, 120 specimens were stored at −20°C until processed for further analyses. Deoxyribonucleic acid (DNA) extractions and purifications were performed with a DNeasy Blood & Tissue Kit (Qiagen, Valencia, CA, United States) following the instructions of the manufacturer.
Genotyping microsatellite markers
We amplified DNA using nine fluorescently labeled nuclear microsatellite primers (GenBank access key: JF292994, JF292995, JF292996, JF293003, JF292998, JF293004, JF292999, JF293001, and JF293006). Amplification of microsatellite loci was carried out following the protocol described by Islam et al. (2011). PCR products of approximately 150–240 bp were confirmed on 1% agarose gel dyed with 0.1% ethidium bromide. The samples were processed at the Caver Biotechnology, University of Illinois (Champaign, United States). We determined the size of each peak by using Peak Scanner software 1.0 (Applied Biosystems).
Estimation of genetic diversity
We calculated the number of alleles (Ae), the observed (Ho) and expected (He) heterozygosity, Hardy-Weinberg equilibrium (HWE), allelic richness (A), and effective population size (Ne) with Arlequin 3.5.2.2 (Excoffier and Lischer, 2010). Also, we assessed the inbreeding coefficient (FIS) and the statistics F using GenePop 4.6 (Rousset, 2008).
Structure and gene flow of populations
We estimated the degree of genetic differentiation from RST and FST values using Arlequin 3.5.2.2. The genetic grouping was performed using Samova 1.0, and the analysis of molecular variance (AMOVA) was performed using Arlequin 3.5.2.2. The genetic structure for collections was examined using the ancestry admixture model runs with 100,000 burn-in, 1,000,000 MCMC, and 25 iterations for each value K (1−8) with STRUCTURE 2.3.4 (Pritchard et al., 2000). We estimated the most probable K value, the highest value of ΔK was calculated by implementing the Evanno method (Evanno et al., 2005) using Structure Harvester v0.6.94 (Earl and vonHoldt, 2012), the structure runs were summarized using CLUMPP (Jakobsson and Rosenberg, 2007), and the results were plotted in Distruct v1.1 and CLUMPP v1.1.2 (Rosenberg, 2004). The dendrogram was calculated with the genetic distance using the Neighbor-Joining with Population v1.2.30 (Langella, 1999). The tree was exported, visualized, and edited using figtree-1.4.3 (Rambaut, 2012). For the isolation by distance (IBD), we calculated population pairwise FST values (Slatkin’s Distance) with 9,999 permutations (Arlequin 3.5.2.2), and we tested IBD using a Mantel test with 9,999 permutations by testing for a correlation between genetic distance and geographic distance (Arlequin 3.5.2.2). We detected the genetic barriers using the Monmonier algorithm based on linearized FST with Barrier 2.2 (Slatkin, 1995; Manni et al., 2004) and evaluated the gene flow between genetic groups with Migrate-N 3.6 (Beerli, 2006).
Results
Genetic diversity of populations
The genetic diversity exhibited 40 alleles from wild fly collections, two alleles for the laboratory strain, nine private alleles from the wild fly, and eleven private alleles among all collections (Supplementary Table 2). The HWE test for wild and laboratory flies showed homozygous excess at the nine microsatellite loci, but we only found significant deviations in six of them (Supplementary Table 3). The highest allelic richness was observed for Soconusco (A = 3.22), and the lowest allelic richness was observed for the laboratory strain (A = 2.11). The highest genetic diversity of Nei was found in the Gulf Coast of Mexico (He = 0.39) and Soconusco (He = 0.38). The lowest values were found in the population of the Mexican Plateau (He = 0.35) and the laboratory strain (He = 0.34). The positive values and significant inbreeding coefficients (FIS p < 0.05) showed heterozygosity deficiency for all loci (Table 1).
Structure and gene flow of populations
The comparison of pairwise RST and FST values displayed genetic differentiation among all populations with microsatellites (Table 2), principally, among SOC and PC (RST = 0.191; FST = 0.065) and LAB among SOC (RST = 0.154; FST = 0.110). The analysis of molecular variance presented ΦST = 0.115 in nDNA microsatellite loci among wild populations and the laboratory strain (Table 3). According to the genetic structure analysis, the best K value was three genetic populations, K = 3 showed the highest value of ΔK, and the second most relevant K value is 4, suggesting ancestry on one fraction of mixed alleles, probably genetic migrants, except Soconusco and the laboratory strain (Figure 1A and Supplementary Figure 1). Moreover, the dendrogram supported a pattern of genetic clustering with three groups (Figure 1C). The Mantel test did not show a significant relationship between geographic and genetic distances with microsatellites < r = 0.275, p > 0.05 > (Supplementary Figure 2).

Table 3. Analysis of molecular variance and genetic differentiation index in nDNA microsatellite loci among wild populations and the laboratory strain.
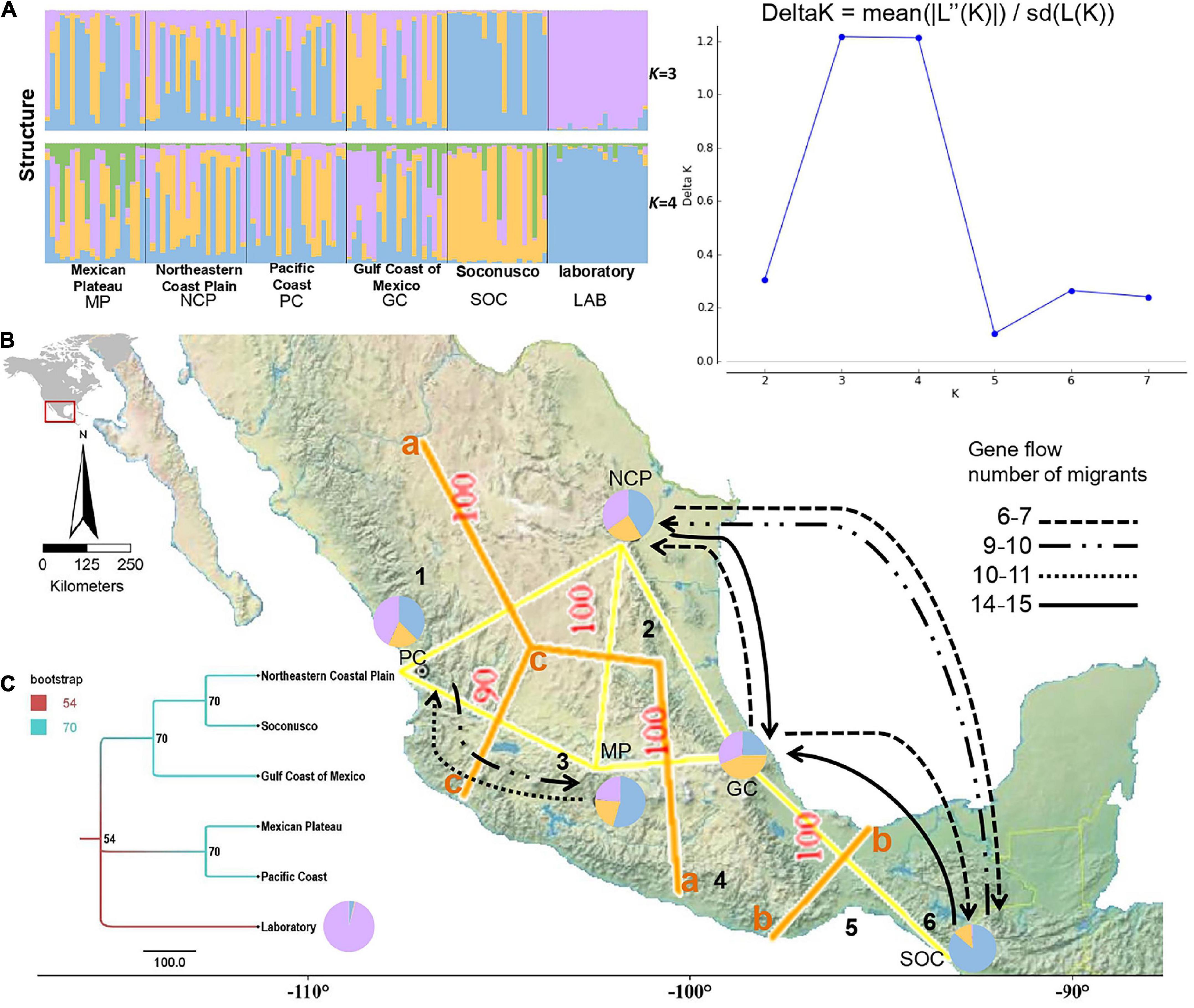
Figure 1. (A) Genetic structure of mexfly based on the nDNA microsatellite loci estimated using STRUCTURE and visualized with Distruct. The bar plot and pie chart indicating the blue, yellow, and purple colors of graphics indicate three genetic groups with Admixture model: K = 3, 25 iterations of each K (1−8) and delta K. (B) Map of five collected localities in Mexico showing the three main genetic barriers with nDNA and the orange line (a, b, and c) found by the Monmonier algorithm; thickness and the number on the side of the barriers indicate the percentage of bootstrap support. The map shows the migration of mexfly populations, and the arrows represent the effective number of migrants in a generation. The main physiographic features and/or regions’ biogeographical boundaries for fruit fly are delimited with lines: (1) SMOcc: Sierra Madre Occidental, (2) SMO: Sierra Madre Oriental, (3) TMVB: Trans Mexican Volcanic Belt, (4) SMS: Sierra Madre del Sur, (5) IT: Isthmus of Tehuantepec, (6) SCh: Sierra Madre de Chiapas. (C) Genetic distance of mexfly populations obtained by Neighbor-Joining of nDNA.
We identified three main genetic barriers contributing to the population structure: “a,” “b” (bootstrap support = 100), and “c” (bootstrap support = 90). Genetic barrier “a” separates populations to the east of the country (Northeastern Coastal Plain and Gulf Coast). Genetic barrier “b” likely is located at the Isthmus of Tehuantepec, separating Soconusco from the rest of the populations. Genetic barrier “c” separates the Mexican Plateau from the Pacific Coast (Figure 1B). For genetic flow, our result about the most probable migration model was based on the Neighbor-Joining dendrogram (Table 4) calculated with Migrate-N 3.6 [AICmin-AICi = 0, p(Bezier) = 1]. The migration was observed only among neighboring populations (Soconusco↔Gulf Coast of Mexico↔Northeastern Coastal Plain, and Mexican Plateau↔Pacific Coast), with migration being higher from south to north, and it was not found between the two groups (Figure 1B and Table 4).
Discussion
By studying the genetic variation of nDNA microsatellites in A. ludens, the data reveal moderate genetic diversity and population structure (K = 3) within species, including wild populations and laboratory strains. The genetic diversity and population structure may be influenced by climate with a close association in the species’ distribution (Santos et al., 2020) and the biogeographic characteristics of the regions of this study, because we identified genetic barriers delineating populations, showing high bootstrap support. Those genetic barriers are delineated according to physiographic features from the Sierra Madre Oriental and the Sierra Madre Occidental, the Sierra Madre del Sur, and the Isthmus of Tehuantepec. Thus, migration was observed only among neighboring populations.
Genetic variability
Moderate Nei’s genetic diversity and low allelic richness can be observed on nDNA among populations of A. ludens. Ruiz-Arce et al. (2015) reported low genetic diversity of A. ludens (hd = 0.58, π = 0.00184) with 68 haplotypes (COI+ND6). The low allelic richness and moderate Nei’s genetic diversity in wild populations were reported in the same species (Malavasi and Morgante, 1982; Pecina-Quintero et al., 2009; Molina-Nery et al., 2014). Also, relative low diversity for the laboratory strain has been previously reported in nuclear markers (Isozymes: A = 1.55 and 1.95) within this species (Malavasi and Morgante, 1982; Molina-Nery et al., 2014). A moderate genetic diversity within our samples, when compared with other Anastrepha species that have been studied with nuclear microsatellites (A. fraterculus, Lanzavecchia et al., 2014; Parreño et al., 2014; A. obliqua, Ruiz-Arce et al., 2019; A. suspensa, Boykin et al., 2010), is likely due to its geographic distribution and effective population size, which are associated with host availability and climate conditions as rain and environmental heterogeneity have a strong influence on the distribution of the species (Aluja, 1994; Celedonio-Hurtado et al., 1995; Hernandez-Ortiz, 2007). Also, the strong wind dispersal capability (up to 135 km) of A. ludens, or human-assisted spread through the movement of infested fruits and pupae on the ground, probably has influenced the moderate genetic diversity (Christenson and Foote, 1960; Aluja, 1994; European Food Safety Authority [EFSA] et al., 2019, 2021). Natural populations of A. ludens are discontinuously distributed, and gene flow varies among these populations depending on historical, geographical, and environmental factors and locality conditions such as humidity, host variability, competition for space, nutrition or mating, parasitism, and competition for resources with another species (Krafsur and Ouma, 2021; Parker et al., 2021). The geographic range of many pest species is very large, and locally adapted populations probably may exist. There may be local selection regimes that cause one or more populations to differ biologically in ways that could make the SIT less effective due to mating barriers. Such barriers might be ecological, temporal, or behavioral (Krafsur and Ouma, 2021; Parker et al., 2021).
The low allelic richness reveals a decrease in genetic diversity that can be explained by a lower effective population size when compared with other Anastrepha species where nuclear microsatellites have also been used (A. fraterculus, Lanzavecchia et al., 2014; Parreño et al., 2014; A. obliqua, Ruiz-Arce et al., 2019; A. suspensa, Boykin et al., 2010), probably due to adaptive response to environmental and demographic changes, and that indicates that A. ludens is at the limit of the tropical distribution. Also, allelic richness is sensitive to the effects of short and severe bottlenecks that affect the distribution of allele frequencies (Franks et al., 2011; Nielsen and Slatkin, 2013). Thus, temporal allelic richness in mexfly is likely due to effective population size variation during fruiting, related to host availability, and due to their polyphagous and multivoltine behavior (Hernandez-Ortiz and Aluja, 1993; Thomas, 2003; Aluja and Mangan, 2008).
Structure, genetic barriers, and migration of populations
We found moderate differentiation, three genetic groups within A. ludens populations were inferred by STRUCTURE, and no pattern of isolation by distance was detected. This is similar to other studies that have found moderate differentiation among groups FST = 0.302 (Ruiz-Arce et al., 2015), FST = 0.058 (Pecina-Quintero et al., 2009), FST = 0.105 (Molina-Nery et al., 2014), and FST = 0.047 (Dupuis et al., 2019) within populations from Mexico. Specifically, the Soconusco population presented a high differentiation with respect to others; this is probably because the Soconusco population is isolated by the mountains of the Sierra Madre del Sur and by the environmental barrier due to trade winds from the Isthmus of Tehuantepec (Lugo-Hubp, 1990). Also, this supports the notion that the origin of this fly may have been southern Mexico/Central America (Ruiz-Arce et al., 2015).
Our results, based on population structure, demonstrated that the laboratory strain remains genetically differentiated and has lower genetic diversity than wild populations, probably due to inbreeding associated with mass rearing. Similar results were found by Dupuis et al. (2019) that included rearing strains of A. ludens to provide context to the structure of wild populations, and all rearing strains were quite divergent from the wild populations. Despite the genetic differentiation, the laboratory strain has been refreshed with wild populations of Mexico, principally Chiapas (Orozco-Dávila et al., 2007). However, the original laboratory strain is a mixture of a strain similar to the initial one from Mission, Texas, United States, whose specimens were collected in Nuevo León and Tamaulipas (México), and wild flies collected from different regions in Mexico for renewing laboratory strain (Orozco-Dávila et al., 2007). Until now, the original strain is still used for mass rearing (Orozco-Dávila et al., 2007, 2017). Also, mass rearing involves breeding about 175 million pupas weekly, intended for the liberation of these flies (Domínguez et al., 2010). Although rearing lines are to be highly bottlenecked and inbreeding causes a loss of genetic variation that could reduce the competitiveness of males (Krafsur and Ouma, 2021), these lines can still be expected to continue to evolve over time and may share some artificially selected traits due to common artificial rearing conditions (Dupuis et al., 2019). A progressive loss of diversity in laboratory colonies, coupled with a progressive decay in physiological quality control indices (e.g., egg hatch, larval development time, pupa size, emergence, flight ability, pheromone production, vision, longevity, and mating compatibility) (FAO/IAEA/USDA, 2019; Parker et al., 2021), could provide evidence of degradation and possibly predict a decline in field competitiveness (Krafsur and Ouma, 2021).
Nevertheless, the genetic structure found in wild populations due to genetic barriers is similar to a metapopulation dynamic (Hanski and Gilpin, 1991; Hanski, 1998; Wegier et al., 2011), that is, a group of spatially separated populations of a species that interact at some level. Each population functions in relative independence from other populations and may eventually become extinct, but immigrants may recolonize another population that is declining or that has become extinct, so the metapopulation persists as long as the colonization rate is equal to the extinction rate (Hanski and Gilpin, 1991; Hanski, 1998; Hanski and Gaggiotti, 2004). The population structure corresponds to the biogeographic provinces in Mexico (Rzedowski, 1978). For example, the main genetic barrier (Figure 1, letter b) matches the existence of physical barriers from the Sierra Madre Oriental and the Sierra Madre Occidental. A second genetic barrier, which is isolating the Soconusco population, is likely related to the Sierra Madre del Sur and the Isthmus of Tehuantepec, the inter-oceanic pass of low altitude in Mexico where the winds range from 18 to 90 km/h (Figure 1, letter b). Similar to previous population genetic studies, our population structuring is high and matches with the biogeographic zones, for example, Dupuis et al. (2019) found that the west Mexico cluster is bounded using SNPs by the southwestern and western extents of the Sierra Madre Occidental, while Ruiz-Arce et al. (2015) found the support for genetic structure between the northern and southern parts of A. ludens’ range, corresponding to the Isthmus of Tehuantepec, using two mitochondrial genes (Ruiz-Arce et al., 2015).
The migration model indicated that natural barriers strongly influence dispersion among populations. However, between neighbor populations, bidirectional genetic flow was significant. This suggests that migration is an important factor in the variability of genetic interchange and in reducing the genetic structure. The more the gene flow, the less the population structure. Therefore, migration under a stepping-stone model, where nearby populations have higher bidirectional gene flow, seems to better explain the distribution of genetic diversity rather than IBD alone. In addition, mexfly has been a species with the adaptation capacity to diverse climates. The gene flow and the genetic structure could be influenced by the high dispersion that occurred due to considerable distance (>30 km) by wind, climate conditions, altitude, and dissemination of larvae for fruits’ commerce activity, allowing mexfly to find the availability of hosts and the refuge for reproduction (Alberti et al., 2002; Shi et al., 2005; Aluja et al., 2009).
Implications of genetic diversity for sterile insect technique management of Anastrepha ludens
At present, old concerns about SIT effectiveness are still applicable (Krafsur, 2005; Pérez-Staples et al., 2021). The results of the current study of mexfly are relevant to understanding the reduced effectiveness of SIT programs. This study found that the genetic diversity of the laboratory strain is lower than that from the wild populations of A. ludens; also, the population structure estimates for the laboratory strain revealed less admixture than wild populations, probably due to massive breeding. This factor may contribute negatively to the SIT efficacy. Furthermore, local landscape, natural barriers, and metapopulation structure may contribute to the moderate genetic diversity, gene flow leading to genetic panmixia, moderate differentiation, and genetic structure found in wild mexfly species.
The population size of A. ludens has been kept high, and there have been small changes in allele frequencies caused by the effect of genetic drift and bottleneck. Regarding our results, considering that the diversity and Ne of the strain are lower than the wild populations, probably due to the bottleneck effect and inbreeding, they could contribute to reduced effectiveness of SIT (Krafsur and Ouma, 2021), so one proposal would be to control the pupae stage where A. ludens are less likely to disperse. Also, genetic data such as inbreeding coefficients and genetic distances should be estimated continuously as part of the quality control program for target populations and for laboratory colonies and their source populations (Krafsur and Ouma, 2021). According to our STRUCTURE results, genetic data showed considerable admixture in wild populations, and it could be by natural spread. A. ludens is considered a strong flier (Centre for Agriculture and Bioscience International [CABI], 2019), which flies as far as 135 km (Christenson and Foote, 1960) using the wind for displacement (Aluja, 1994). The maximum natural spreading distance that A. ludens is expected to cover in 1 year is approximately 9.4 km (with a 95% uncertainly range of 1–34 km). However, the introduction of the pest into areas could occur by human-assisted spread through the movement of infested fruits, such as imports of fruit commodities or fruits in passenger luggage and pupae in soil or another growing medium with host plants (European Food Safety Authority [EFSA] et al., 2019, 2021). As such, we suggest establishing population management units; the first control unit would comprise Soconusco–Gulf Coast of Mexico–Northeastern Coastal Plain, and the second would integrate the Mexican Plateau and the Pacific Coast. In particular, the states of Veracruz (in the Gulf Coast of Mexico) and Tamaulipas (in Northeastern Coastal Plain) grow and harvest citrus. Similarly, Sinaloa and Nayarit (in Pacific Coast) and Chiapas (in Soconusco) grow and harvest mangoes (SAGARPA, 2022). Where each management unit could be colonized by sterile specimens derived from the same unit. This would also require developing strains compatible with each local population, by utilizing genomics differentiation in A. ludens populations and associations with different host plants and predicting the geographic and environmental ranges and relative abundances of invasive species using ecological niche modeling to give policies on a scientific basis (Santos et al., 2020; Aguirre-Ramirez et al., 2021; Gutierrez et al., 2021).
Data availability statement
The original contributions presented in this study are included in the article/Supplementary material, and the input files of the microsatellite loci from this project have been deposited in the Dryad repository, available at: https://doi.org/10.5061/dryad.xwdbrv1gw; further inquiries can be directed to the corresponding authors.
Ethics statement
The animal study was reviewed and approved by the Instituto de Ecología and posgrado en Ciencias Biologicas, UNAM.
Author contributions
DP, MS-F, and NG-R conceived and designed the study. NG-R performed the laboratory work, processed the microsatellites data, and performed the analyses. DP, NS, AM-Y, MS-F, and JN-F supervised the analyses and contributed to the discussion. NG-R and DP led the manuscript writing with contributions from all authors. All authors approved the final version of the manuscript.
Funding
Funding was provided by the Instituto de Ecología, UNAM to DP and NS.
Acknowledgments
We thank Larissa Guillén-Conde and Emilio Hernández-Ortiz for providing the samples. We sincerely thank reviewers of the manuscript Marco Suárez-Atilano and Julio Cesar García-Zebadúa for the useful comments to the manuscript. This manuscript represents fulfillment of the graduate program of maestría en Ciencias Biologicas of the Universidad Nacional Autónoma de México (UNAM) to NG-R. We are grateful for the scholarship of the Consejo Nacional de Ciencia y Tecnología, México (CONACyT: 412884).
Conflict of interest
The authors declare that the research was conducted in the absence of any commercial or financial relationships that could be construed as a potential conflict of interest.
Publisher’s note
All claims expressed in this article are solely those of the authors and do not necessarily represent those of their affiliated organizations, or those of the publisher, the editors and the reviewers. Any product that may be evaluated in this article, or claim that may be made by its manufacturer, is not guaranteed or endorsed by the publisher.
Supplementary material
The Supplementary Material for this article can be found online at: https://www.frontiersin.org/articles/10.3389/fevo.2022.948640/full#supplementary-material
References
Aguirre-Ramirez, E., Velasco-Cuervo, S., and Toro-Perea, N. (2021). Genomic traces of the fruit fly anastrepha obliqua associated with its polyphagous nature. Insects 12:1116. doi: 10.3390/insects12121116
Alberti, A. C., Rodriguero, M. S., Cendra, P. G., Saidman, B. O., and Vilardi, J. C. (2002). Evidence indicating that argentine populations of Anastrepha fraterculus (Diptera: Tephritidae) belong to a single biological species. Ann. Entomol. Soc. Am. 95, 505–512.
Aluja, M. (1994). Bionomics and management of anastrepha. Annu. Rev. Entomol. 39, 155–178. doi: 10.1146/annurev.en.39.010194.001103
Aluja, M., and Mangan, R. L. (2008). Fruit fly (Diptera: Tephritidae) host status determination: critical conceptual, methodological, and regulatory considerations. Annu. Rev. Entomol. 53, 473–502. doi: 10.1146/annurev.ento.53.103106.093350
Aluja, M., Rull, J., Pérez-Staples, D., Díaz-Fleischer, F., and Sivinski, J. (2009). Random mating among Anastrepha ludens (Diptera: Tephritidae) adults of geographically distant and ecologically distinct populations in Mexico. Bull. Entomol. Res. 99, 207–214. doi: 10.1017/S0007485308006299
Barker, G. C. (2002). Microsatellite DNA: a tool for population genetic analysis. Trans. R. Soc. Trop. Med. Hyg. 96, S21–S24. doi: 10.1016/S0035-9203(02)90047-7
Beerli, P. (2006). Comparison of Bayesian and maximum-likelihood inference of population genetic parameters. Bioinformatics 22, 341–345. doi: 10.1093/bioinformatics/bti803
Boykin, L. M., Shatters, R. G., Hall, D. G., Dean, D., and Beerli, P. (2010). Genetic variation of Anastrepha suspensa (Diptera: Tephritidae) in Florida and the caribbean using microsatellite DNA markers. J. Econ. Entomol. 103, 2214–2222. doi: 10.1603/EC10128
Carneiro-Vieira, M. L., Santini, L., Diniz, A. L., and Munhoz, C. D. F. (2016). Microsatellite markers: what they mean and why they are so useful. Genet. Mol. 39, 312–328.
Celedonio-Hurtado, H., Aluja, M., and Liedo, P. (1995). Adult population fluctuations of anastrepha species (Diptera: Tephritidae) in tropical orchard habitats of chiapas, Mexico. Environ. Entomol. 24, 861–869. doi: 10.1093/ee/24.4.861
Centre for Agriculture and Bioscience International [CABI] (2019). Invasive Species Compendium. Datasheet Anastrepha ludens (Mexican fruit fly) 19/11/1919. Wallingford: CAB International.
Christenson, L. D., and Foote, R. H. (1960). Biology of fruit flies. Annu. Rev. Entomol. 5, 171–192.
Domínguez, J., Artiaga, T., Solís, E., and Hernandez, E. (2010). “Métodos de colonización y cría masiva,” in Moscas de la Fruta: Fundamentos y Procedimientos Para su Manejo, eds P. Montoya, J. Toledo, and E. Hernández (México D.F: S y G Editores).
Dupuis, J. R., Ruiz-Arce, R., Barr, N. B., Thomas, D. B., and Geib, S. M. (2019). Range-wide population genomics of the Mexican fruit fly: toward development of pathway analysis tools. Evol. Appl. 12, 1641–1660. doi: 10.1111/eva.12824
Earl, D. A., and vonHoldt, B. M. (2012). Structure harvester: a website and program for visualizing STRUCTURE output and implementing the Evanno method. Conserv. Genet. Resour. 4, 359–361. doi: 10.1007/s12686-011-9548-7
Enkerlin, W. R. (2005). “Impact of fruit fly control programmes using the sterile insect technique,” in Sterile Insect Technique: Principles and Practice in Area-Wide Integrated Pest Management, eds V. A. Dyck, J. Hendrichs, and A. S. Robinson (Dordrecht: Springer Netherlands), 651–676. doi: 10.1007/1-4020-4051-2_25
European Food Safety Authority [EFSA], Baker, R., Gilioli, G., Behring, C., Candiani, D., Gogin, A., et al. (2019). Anastrepha Ludens – Pest Report to Support Ranking of EU Candidate Priority Pests by the EFSA Working Group on EU Priority Pests. Parma: EFSA.
European Food Safety Authority [EFSA], Schenk, M., Mertens, J., Delbianco, A., and Graziosi I Vos, S. (2021). Pest Survey Card on Anastrepha ludens. Parma: EFSA.
Evanno, G., Regnaut, S., and Goudet, J. (2005). Detecting the number of clusters of individuals using the software STRUCTURE: a simulation study. Mol. Ecol. 14, 2611–2620.
Excoffier, L., and Lischer, H. E. L. (2010). Arlequin suite ver 3.5: a new series of programs to perform population genetics analyses under Linux and Windows. Mol. Ecol. Resour. 10, 564–567. doi: 10.1111/j.1755-0998.2010.02847.x
FAO/IAEA/USDA (2019). Product Quality Control for Sterile Mass-Reared and Released Tephritid Fruit Flies, Version 7.0. Vienna: International Atomic Energy Agency.
Foote, B. A. (1994). Handbook of the fruit flies (Diptera: Tephritidae) of America North of Mexico. Ann. Entomol. Soc. Am. 87, 400–401. doi: 10.1093/aesa/87.3.400
Franks, S. J., Pratt, P. D., and Tsutsui, N. D. (2011). The genetic consequences of a demographic bottleneck in an introduced biological control insect. Conserv. Genet. 12, 201–211. doi: 10.1007/s10592-010-0133-5
Gutierrez, A. P., Ponti, L., Neteler, M., Suckling, D. M., and Cure, J. R. (2021). Invasive potential of tropical fruit flies in temperate regions under climate change. Commun. Biol. 4:1141. doi: 10.1038/s42003-021-02599-9
Hancock, J. M. (1999). “Microsatellites and other simple sequences: genomic context and mutational mechanisms,” in Microsatellites. Evolution and Applications, eds D. B. Goldstein and C. Schlötterer (Oxford: Oxford University Press).
Hanski, I., and Gaggiotti, O. (2004). “1 - metapopulation biology: past, present, and future,” in Ecology, Genetics and Evolution of Metapopulations, eds I. Hanski and O. E. Gaggiotti (Burlington: Academic Press).
Hanski, I., and Gilpin, M. (1991). Metapopulation dynamics: brief history and conceptual domain. Biol. J. Linn. Soc. 42, 3–16. doi: 10.1111/j.1095-8312.1991.tb00548.x
Hernández-Ortíz, V. (1992). El Género Anastrepha Schiner en México (Diptera: Tephritidae), Taxonomía, Distribución y sus Plantas Huéspedes. Xalapa: Sociedad Mexicana de Entomología.
Hernandez-Ortiz, V. (2007). “Diversidad y biogeografía del género anastrepha en Mexico,” in Moscas de La Fruta En Latinoamérica (Diptera: Tephritidae): Diversidad, Biología y Manejo, ed. V. Hernández-Ortiz (México: Distrito Federal).
Hernandez-Ortiz, V., and Aluja, M. (1993). Listado de especies del genero neotropical anastrepha (Diptera: Tephritidae) con notas sobre su distribución y plantas hospederas. Folia Entomol. Mex. 88, 89–105.
Islam, M. S., Ruiz-Arce, R., and McPheron, B. A. (2011). Microsatellite markers for the West Indian fruit fly (Anastrepha obliqua) and cross species amplification in related pest species. Conserv. Genet. Resour. 3, 549–551. doi: 10.1007/s12686-011-9401-z
Jakobsson, M., and Rosenberg, N. A. (2007). CLUMPP: a cluster matching and permutation program for dealing with label switching and multimodality in analysis of population structure. Bioinformatics 23, 1801–1806. doi: 10.1093/bioinformatics/btm233
Jin, L., Macaubas, C., Hallmayer, J., Kimura, A., and Mignot, E. (1996). Mutation rate varies among alleles at a microsatellite locus: phylogenetic evidence. Proc. Natl. Acad. Sci. U S A. 93, 15285–15288.
Klassen, W., and Curtis, C. F. (2005). “History of the sterile insect technique,” in Sterile Insect Technique: Principles and Practice in Area-Wide Integrated Pest Management, eds V. A. Dyck, J. Hendrichs, and A. S. Robinson (Dordrecht: Springer Netherlands).
Krafsur, E. S. (2005). “Role of population genetics in the sterile insect technique,” in Sterile Insect Technique: Principles and Practice in Area-Wide Integrated Pest Management, eds V. A. Dyck, J. Hendrichs, and A. S. Robinson (Dordrecht: Springer Netherlands).
Krafsur, E. S., and Ouma, J. O. (2021). “Role of population genetics in the sterile insect technique,” in Sterile Insect Technique: Principles and Practice in Area-Wide Integrated Pest Management, eds V. A. Dyck, J. Hendrichs, and A. S. Robinson (Boca Raton, FL: CRC Press).
Langella, O. (1999). Populations 1.2.30 [WWW Document]. Available online at: https://bioinformatics.org/populations/ (accessed 30 March, 2022).
Lanzavecchia, S. B., Juri, M., Bonomi, A., Gomulski, L., Scannapieco, A. C., Segura, D. F., et al. (2014). Microsatellite markers from the “South American fruit fly” Anastrepha fraterculus: a valuable tool for population genetic analysis and SIT applications. BMC Genet. 15, (Suppl. 2):S13. doi: 10.1186/1471-2156-15-S2-S13
Lugo-Hubp, J. (1990). El relieve de la República Mexicana. Revista Mexicana Ciencias Geol. 9, 82–111.
Mahmoud, M. F. (2010). Effect of gamma radiation on the sterility and quality of male peach fruit fly, Bactrocera zonata (Saunders)(Diptera: Tephritidae). Egyptian J. Biol. Pest Control 20, 71–77.
Malavasi, A., and Morgante, J. S. (1982). Genetic variation in natural populations of anastrepha (Diptera: Tephritidae). Rev. Bras Genet. 5, 263–278.
Manni, F. E., Guérard, E., and Heyer, E. (2004). Geographic patterns of (Genetic, Morphologic, Linguistic) variation: how barriers can be detected by using monmonier’s algorithm. Hum. Biol. 76, 173–190.
Manni, M., Lima, K. M., Guglielmino, C. R., Lanzavecchia, S. B., Juri, M., Vera, T., et al. (2015). Relevant genetic differentiation among brazilian populations of Anastrepha fraterculus (Diptera, tephritidae). ZooKeys 2015, 157–173. doi: 10.3897/zookeys.540.6713
Molina-Nery, M. C., Ruiz-Montoya, L., Zepeda-Cisneros, C. S., and Liedo, P. (2014). Genetic structure of populations of Anastrepha ludens (Diptera: Tephritidae) in Mexico. Fla. Entomol. 97, 1648–1661.
Nielsen, R., and Slatkin, M. (2013). An Introduction to Population Genetics. Sunderland MA: Sinauer Assoc.
Norrbom, A. L., and Korytkowski, C. A. (2009). A revision of the Anastrepha robusta species group (Diptera: Tephritidae). Zootaxa 2182, 1–91. doi: 10.11646/zootaxa.2182.1.1
Norrbom, A. L., and Korytkowski, C. A. (2012). New species of Anastrepha (Diptera: Tephritidae), with a key for the species of the megacantha clade. Zootaxa 3478, 510–552. doi: 10.11646/zootaxa.3478.1.43
Norrbom, A. L., Zucchi, R. A., and Hernández-Ortiz, V. (1999). “Phylogeny of the genera Anastrepha and Toxotrypana (Trypetinae: Toxotrypanini) based on morphology,” in Fruit Flies (Tephritidae), eds M. Aluja, and A. Norrbom (Boca Raton, FL: CRC Press), 317–360. doi: 10.1201/9781420074468
Orozco-Dávila, D., Hernández, R., Meza, S., and Domínguez, J. (2007). Sexual competitiveness and compatibility between mass-reared sterile flies and wild populations of Anastrepha ludens (Diptera: Tephritidae) from different regions in Mexico. Fla. Entomol. 90, 19–26.
Orozco-Dávila, D., Quintero, L., Hernández, E., Solís, E., Artiaga, T., Hernández, R., et al. (2017). Mass rearing and sterile insect releases for the control of Anastrepha spp. pests in mexico–a review. Entomol. Exp. Appl. 164, 176–187.
Panduranga, G. S., Sharma, K., Singh, B., and Sharma, R. K. (2022). Effect of gamma irradiation on quality parameters, sterility and mating competitiveness of melon fly. Bactrocera cucurbitae (Coquillett). Int. J. Trop. Insect Sci. 42, 875–883.
Parker, A. G., Vreysen, M. J. B., Bouyer, J., and Calkins, C. O. (2021). “Sterile insect quality control/assurance,” in Sterile Insect Technique: Principles and Practice in Area-Wide Integrated Pest Management, eds V. A. Dyck, J. Hendrichs, and A. S. Robinson (Boca Raton, FL: CRC Press), 399–440.
Parreño, M. A., Scannapieco, A. C., Remis, M. I., Juri, M., Vera, M. T., Segura, D. F., et al. (2014). Dynamics of genetic variability in Anastrepha fraterculus (Diptera: Tephritidae) during adaptation to laboratory rearing conditions. BMC Genet. 15:S14. doi: 10.1186/1471-2156-15-S2-S14
Pecina-Quintero, V., Jiménez-Becerril, M. F., Ruiz-Salazar, R., Núñez-Colín, C. A., Loera-Gallardo, J., Hernández-Delgado, S., et al. (2020). Variability and genetic structure of Anastrepha ludens Loew (Diptera: Tephritidae) populations from Mexico. Int. J. Trop. Insect Sci. 40, 657–665. doi: 10.1007/s42690-020-00117-8
Pecina-Quintero, V., López Arroyo, J. I., Loera Gallardo, J., Rull, J., Rosales Robles, E., Cortez Mondaca, E., et al. (2009). Genetic differences between Anastrepha ludens (Loew) populations stemming from a native and an exotic host in NE Mexico. Agric. Téc. En México 35, 323–331.
Pérez-Staples, D., Díaz-Fleischer, F., and Montoya, P. (2021). The sterile insect technique: success and perspectives in the neotropics. Neotrop. Entomol. 50, 172–185. doi: 10.1007/s13744-020-00817-3
Pérez-Staples, D., Shelly, T. E., and Yuval, B. (2013). Female mating failure and the failure of ‘mating’ in sterile insect programs. Entomol. Exp. Appl. 146, 66–78. doi: 10.1111/j.1570-7458.2012.01312.x
Pritchard, J. K., Stephens, M., and Donnelly, P. (2000). Inference of population structure using multilocus genotype data. Genetics 155, 945–959. doi: 10.1093/genetics/155.2.945
Rambaut, A. (2012). FigTree v.1.4.2. Available online at: http://tree.bio.ed.ac.uk/software/figtree/
Reyes, F. J., Santiago, M. G., and Hernandez, M. P. (2000). “The Mexican fruit fly eradication programme,” in Proceedings of the area-wide control of fruit flies and other insect pests. International conference on area-wide control of insect pests, and the 5th international symposium on fruit flies of economic importance, 28 May 5 June 1998, ed. K. H. Tan (Pulau Pinang: Penerbit Universiti Sains Malaysia), 377–380.
Rosenberg, N. A. (2004). Distruct: a program for the graphical display of population structure. Mol. Ecol. Not. 4, 137–138. doi: 10.1046/j.1471-8286.2003.00566.x
Rousset, F. (2008). genepop’007: a complete re-implementation of the genepop software for windows and linux. Mol. Ecol. Resour. 8, 103–106. doi: 10.1111/j.1471-8286.2007.01931.x
Ruiz-Arce, R., Islam, M. S., Aluja, M., and McPheron, B. A. (2019). Genetic variation in Anastrepha obliqua (Diptera: Tephritidae) in a highly diverse tropical environment in the Mexican state of veracruz. J. Econ. Entomol. 112, 2952–2965. doi: 10.1093/jee/toz223
Ruiz-Arce, R., Owen, C. L., Thomas, D. B., Barr, N. B., and McPheron, B. A. (2015). Phylogeographic structure in Anastrepha ludens (Diptera: Tephritidae) populations inferred with mtDNA sequencing. J. Econ. Entomol. 108, 1324–1336. doi: 10.1093/jee/tov082
Ruiz-Montoya, L., Vallejo, R. V., Haymer, D., and Liedo, P. (2020). Genetic and ecological relationships of Anastrepha ludens (Diptera: Tephritidae) populations in Southern Mexico. Insects 11:E815. doi: 10.3390/insects11110815
Rull, J., and Barreda-Landa, A. (2007). Colonization of a hybrid strain to restore male Anastrepha ludens (Diptera: Tephritidae) mating competitiveness for sterile insect technique programs. J. Econ. Entomol. 100, 752–758. doi: 10.1603/0022-0493(2007)100[752:coahst]2.0.co;2
Rull, J., Brunel, O., and Mendez, M. E. (2005). Mass rearing history negatively affects mating success of male Anastrepha ludens (Diptera: Tephritidae) reared for sterile insect technique programs. J. Econ. Entomol. 98, 1510–1516. doi: 10.1093/jee/98.5.1510
SAGARPA (2022). Secretaría de Agricultura y Desarrollo Rural, 2022. Available online at: https://www.gob.mx/agricultura#335 (Accessed July 7, 2022).
Santos, R. P. D., Silva, J. G., and Miranda, E. A. (2020). The past and current potential distribution of the fruit fly Anastrepha obliqua (Diptera: Tephritidae) in South America. Neotrop. Entomol. 49, 284–291. doi: 10.1007/s13744-019-00741-1
Schug, M. D., Mackay, T. F., and Aquadro, C. F. (1997). Low mutation rates of microsatellite loci in Drosophila melanogaster. Nat. Genet. 15, 99–102.
Selkoe, K. A., and Toonen, R. J. (2006). Microsatellites for ecologists: a practical guide to using and evaluating microsatellite markers. Ecol. Lett. 9, 615–629. doi: 10.1111/j.1461-0248.2006.00889.x
Shi, W., Kerdelhue, C., and Ye, H. (2005). Population genetics of the oriental fruit fly, Bactrocera dorsalis (Diptera: Tephritidae), in Yunnan (China) based on mitochondrial dna sequences. Environ. Entomol. 34, 977–983. doi: 10.1603/0046-225X-34.4.977
Slatkin, M. (1995). A measure of population subdivision based on microsatellite allele frequencies. Genetics 139, 457–462. doi: 10.1093/genetics/139.1.457
Thomas, D. B. (2003). Reproductive phenology of the Mexican fruit fly, Anastrepha ludens (Loew) (Diptera: Tephritidae) in the sierra madre oriental, Northern Mexico. Neotrop. Entomol. 32, 385–397. doi: 10.1590/S1519-566X2003000300002
Thomas, D. B. (2004). Hot peppers as a host for the Mexican fruit fly Anastrepha ludens (Diptera: Tephritidae). Fla. Entomol. 87, 603–608.
Vreysen, M. J. B. (2005). “Monitoring sterile and wild insects in area-wide integrated pest management programmes,” in Sterile Insect Technique: Principles and Practice in Area-Wide Integrated Pest Management, eds V. A. Dyck, J. Hendrichs, and A. S. Robinson (Dordrecht: Springer Netherlands), 325–361. doi: 10.1007/1-4020-4051-2_12
Walder, J. M. M., and Calkins, C. O. (1993). Effects of gamma radiation on the sterility and behavioral quality of the Caribbean fruit fly, Anastrepha suspensa (Loew)(Diptera: Tephritidae). Scientia Agrícola 50, 157–165.
Wegier, A., Piñeyro-Nelson, A., Alarcón, J., Gálvez-Mariscal, A., Álvarez-Buylla, E. R., and Piñero, D. (2011). Recent long-distance transgene flow into wild populations conforms to historical patterns of gene flow in cotton (Gossypium hirsutum) at its centre of origin. Mol. Ecol. 20, 4182–4194. doi: 10.1111/j.1365-294X.2011.05258.x
Keywords: variability, structure, migration, biogeographic zone, nuclear, microsatellites
Citation: Gálvez-Reyes N, Salvador-Figueroa M, Santini NS, Mastretta-Yanes A, Núñez-Farfán J and Piñero D (2022) Nuclear genetic diversity and structure of Anastrepha ludens wild populations evidenced by microsatellite markers. Front. Ecol. Evol. 10:948640. doi: 10.3389/fevo.2022.948640
Received: 20 May 2022; Accepted: 18 July 2022;
Published: 15 August 2022.
Edited by:
Nikica Šprem, University of Zagreb, CroatiaReviewed by:
Raul Ruiz, United States Department of Agriculture (USDA), United StatesEugenia Zarza, El Colegio de la Frontera Sur, Mexico
Copyright © 2022 Gálvez-Reyes, Salvador-Figueroa, Santini, Mastretta-Yanes, Núñez-Farfán and Piñero. This is an open-access article distributed under the terms of the Creative Commons Attribution License (CC BY). The use, distribution or reproduction in other forums is permitted, provided the original author(s) and the copyright owner(s) are credited and that the original publication in this journal is cited, in accordance with accepted academic practice. No use, distribution or reproduction is permitted which does not comply with these terms.
*Correspondence: Nancy Gálvez-Reyes, bmFuY3lnYWx2ZXpAZWNvbG9naWEudW5hbS5teA==; Daniel Piñero, cGluZXJvQHVuYW0ubXg=