- 1Institute for Chemistry and Biology of the Marine Environment (ICBM-Terramare), University of Oldenburg, Wilhelmshaven, Germany
- 2Department of Marine Biology, Senckenberg am Meer, Wilhelmshaven, Germany
- 3Helmholtz Institute for Functional Marine Biodiversity (HIFMB), Oldenburg, Germany
- 4Institute of Biology and Environmental Sciences (IBU), University of Oldenburg, Oldenburg, Germany
Microphytobenthos (MPBs) are the main primary producers in shallow marine ecosystems, such as the Wadden Sea. We investigated the spatial and temporal dynamics of MPB communities across the marine-terrestrial boundary over three seasons (spring, summer, and fall) on three East Frisian Islands (Norderney, Spiekeroog, and Wangerooge) in the German Wadden Sea. Natural transects were compared with 12 experimental islands (salt marsh vegetated vs. initially bare islands) established on the tidal flats of Spiekeroog for studying dispersal-mediated community assembly. Sediment cores were taken along triplicate transects and on three elevation levels of the experimental islands, corresponding to the pioneer (pio) zone, the lower salt (LS) marsh, and the upper salt (US) marsh. On both the natural transects and the experimental islands, the highest MPB biomass was observed in the pio zone, where vegetation-driven sediment stabilization and high-mud content could have promoted MPB biomass in this marine-terrestrial transition zone. On the experimental islands, MPB biomass and diversity significantly decreased with elevation regardless of the season, indicating that the rarely submerged upper salt marsh level supported minimal MPB growth. The MPB biomass was also higher on initially vegetated than on bare islands, which was the most pronounced on the US level. On the tidal flat transects, the MPB biomass significantly increased with elevation up to the pio zone before decreasing again in the LS marsh. Temperature, sediment water content, and grain size significantly affected transect MPB biomass. MPB diversity, on the other hand, was not related to elevation but was rather determined by temperature, mean grain size, and mud content. Our study suggests that extending MPB studies into the “terrestrial” domain of salt marshes enhances our understanding of the microalgae–plant interaction in this important boundary zone.
Introduction
Tidal flats are soft bottom habitats between the mean high-tide and mean low-tide water lines found worldwide along coastlines (Dyer et al., 2000). They form the transition zone between marine and terrestrial environments, and are highly dynamic landscapes subject to variable environmental factors (e.g., irradiance, temperature, nutrients, salinity, and inundation) driven by tides, as well as by daily and seasonal fluctuations (Miththapala, 2013; Du et al., 2017). The Wadden Sea, a UNESCO Natural World Heritage Site, is the world's largest tidal flat system situated on the backwater side of low-lying barrier islands stretching for 500 km off the coast of the Netherlands to Germany and up to Denmark in the north. In addition to the extensive tidal flats in this region, coastal salt marshes with salt-tolerant plant communities border the tidal flats. European salt marshes follow a clear elevational gradient, with vegetation zonation forming the pioneer (pio), the lower salt (LS) marsh, and the upper salt (US) marsh zones (Balke et al., 2017).
In these soft bottom habitats, benthic microalgae or microphytobentho (MPB) inhabit the upper few millimeters of illuminated sediments. The MPB consists of unicellular photosynthetic eukaryotic algae (such as diatoms, dinoflagellates, euglenids, cryptophytes, chlorophytes, and chrysophytes) and cyanobacteria (MacIntyre et al., 1996; Jesus et al., 2009), often forming visible brown or greenish microbial mats on the surface of tidal flats. The primary production of MPB and biomass can exceed that of phytoplankton in the overlying water column in many shallow aquatic systems (MacIntyre et al., 1996; Underwood, 2001), and contribute up to 50% of the total primary productivity in estuarine environments (Underwood and Kromkamp, 1999). The continuous resuspension of part of the MPB community into the water column leads to transport and dispersal by waves and currents (Baillie and Welsh, 1980; MacIntyre et al., 1996; Mitbavkar and Anil, 2002), and also results in MPB contributing to part of the primary production of the phytoplankton community in the water column (MacIntyre et al., 1996; Lucas et al., 2000). In addition, the MPB play an important role in mediating nutrient fluxes between the sediment and the water column (Cabrita and Brotas, 2000; Dong et al., 2000) and provide an important food source for benthic consumers, commercially important fish and shell fish stocks, as well as migratory bird populations (Middelburg et al., 2000; Hillebrand et al., 2002; Scholz et al., 2014). Furthermore, MPB stabilize sediments through the secretion of extracellular polymeric substances (Paterson, 1989; MacIntyre et al., 1996). Aside from being the dominant primary producers in tidal flats, MPB are also present in salt marsh environments bordering the tidal flats (MacIntyre et al., 1996), being brought in during flood and ebb spring tides (Redzuan and Underwood, 2020).
Overall, a variety of abiotic and biotic factors determine the spatial and temporal dynamics of biomass and composition of MPB, resulting in a patchy distribution on small local scales driven by nutrient distribution (Thornton et al., 2002), light availability (Kühl and Jorgensen, 1994), and grazing by consumers (Kelaher et al., 2003). The North Sea and the back barrier tidal flats of the East Frisian Islands are strongly influenced by a semi-diurnal tidal system with wide tidal ranges greater than 3 m (Dittmann, 2012) inducing highly variable local environmental conditions in the tidal flat systems. However, regionally different hydrodynamic conditions resulting in differential sediment erosion and deposition may lead to large-scale habitat differences among neighboring islands, for instance, in sediment composition, grain size, and slope gradient leading up to the shoreline. These differences, in turn, may also influence MPB distribution and biomass (Chapman et al., 2010).
Algal biomass tends to be higher on the upper tidal flats due to longer emersion duration and shallower water depth during high tide, which results in higher irradiances, warmer temperatures, and higher sediment stability (low resuspension rate) (van der Wal et al., 2010, 2017; Daggers et al., 2020; Jacobs et al., 2021). Algal biomass is further strongly influenced by sediment properties, which affect water content, organic matter content, and light penetration. For instance, muddy sediments were shown to support higher biomass than sandy sediments (Brotas et al., 1995; Herman et al., 2001; Billerbeck et al., 2007). Also, seasonal succession patterns of MPB based on seasonal increases in irradiance and temperature have been demonstrated, with some systems having diatom bloom peaks in spring and/or autumn, while others have summer cyanobacteria bloom peaks (Thornton et al., 2002; Montani et al., 2003; van der Wal et al., 2010; Scholz and Liebezeit, 2012a; Brito et al., 2013). Cyanobacteria dominate MPB communities, especially in summer, when temperatures are high (Watermann et al., 1999), as they usually exhibit lower sensitivities to high temperatures compared to other algal groups like diatoms. Filamentous cyanobacteria especially dominate MPB communities on the upper shore and within salt marshes during the warmer seasons (Kaas, 1987; Tracy and South, 1989), resulting in high algal biomass, but low species richness at higher elevations. High MPB biomass often coincides with low diversity, where usually only one or few species dominated the MPB community (Colijn and Dijkema, 1981; Hillebrand and Sommer, 1997; Forster et al., 2006; Ribeiro et al., 2021).
Complementing the studies described above that investigated the effects of abiotic conditions (temperature, nutrients, and light) and sediment properties (grain size, water content) on MPB communities, numerous studies have been conducted to investigate spatial and temporal dynamics of marine MPB on tidal flats (Facca and Sfriso, 2007; Du et al., 2009; Jesus et al., 2009; Scholz and Liebezeit, 2012a,b; Vogt et al., 2018). However, most of these studies were purely observational and conducted at small local scales, while studies investigating MPB communities over larger regional scales are scarce (Sawai et al., 2016). Additionally, most of these studies only covered the marine gradient from subtidal to intertidal sites. Even in the few studies that have included the supratidal salt marsh (Brotas et al., 1995; Janousek, 2009; Redzuan and Underwood, 2020), MPB communities were compared only between selected tidal flat and salt marsh sites and not along a continuous gradient including the transitional zone across the marine-terrestrial interface.
In the present study, we contribute to the current knowledge mainly in two novel aspects: (1) by extending the analysis into the supratidal zone as we include the vegetated (salt marsh) part of the elevational gradient, and (2) we use the unique opportunity to compare natural transects with data from experimental islands. These twelve experimental islands have been installed in 2014 on the back-barrier tidal flat of Spiekeroog, each consisting of three different elevation levels mirroring the pio, LS, and US marsh, to study spatial processes determining community assembly, species sorting, dispersal, and competition (full details can be found in Balke et al., 2017). Six islands were initially bare and colonized via dispersal from source communities of the salt marsh and tidal flat, and the other six received transplanted LS marsh plant communities. We were, therefore, able to compare MPB communities in initially vegetated vs. unvegetated versions of the pio, LS, and US marsh zones.
We monitored the spatial and temporal distribution of MPB communities on the experimental islands, following a seasonal succession from spring, over summer, to autumn, and compared these patterns to natural transects across the marine-terrestrial interface of three East Frisian Islands (Norderney, Spiekeroog, and Wangerooge) differing in regional hydrodynamics and local environmental factors. We investigated chlorophyll-a (Chl-a) biomass as a proxy for MPB biomass, and also calculated MPB diversity based on pigment diversity, which is to our knowledge, a novel approach for studying MPB diversity in intertidal sediments. Previous studies have mainly used pigment composition data to analyze functional group composition of MPB (Lucas and Holligan, 1999; Méléder et al., 2005; Janousek, 2009).
We formulated our hypotheses as following: (H1) Total algal biomass is negatively correlated to pigment diversity. (H2) Total algal biomass increases with elevation and peaks toward the salt marsh, while pigment diversity decreases with elevation. (H3) Total algal biomass and pigment diversity are constrained by physical factors, such as temperature and sediment properties (water and mud content, mean grain size) that explain patterns in space and time. On the experimental islands, we additionally tested the following hypothesis: (H4) Algal biomass and pigment diversity are higher on the initially vegetated islands compared to the bare islands due to higher sediment water content as a result of vegetation cover.
Materials and methods
Study area
The study area is situated within the Lower Saxony Wadden Sea National Park in Northern Germany and focuses on the back-barrier tidal flats of the East Frisian Islands Norderney, Spiekeroog, and Wangerooge (Figures 1A,B). On each island, three transects (Figures 1A–C) were sampled across the marine-terrestrial interface, starting at the edge of the tidal flats close to the salt marsh and extending from 720 to 1,000 m in seaward direction (Figures 1C–E). Transects were spaced from 300 to 450 m apart, while sampling sites were spaced from 150 to 450 m apart. Site I was the sampling site situated furthest outside on the tidal flat of each island, and the sampling transect extended inwards down to site IV, which is the area right before where the salt marsh boundary zone starts (Figures 1C–E). Transects on Spiekeroog were extended to include the pio zone (site V) and LS marsh (site VI) of the local natural salt marsh (Figure 1C). The US marsh could not be sampled, due to the extremely dry and hard sediment, which is further made impenetrable by a tough layer of plant roots. Natural salt marshes with different vegetation zones were not present on the other two islands, which were thus only sampled up to site IV. The range of elevation (meters above the mean sea level; m AMSL) of the sampling sites differed slightly from one island to the other. Sampling sites on Norderney ranged between −0.4 and 0.4 m AMSL, while the sampled sites on Wangerooge ranged from −0.3 to 0.85 m AMSL. The transects on Spiekeroog covered elevations between 0 m and 0.9 m AMSL for sites I to IV, and up to 1.7 m AMSL for the pio and LS marsh zones. For more precise GPS locations, elevation data, and sampling dates, refer to Tables S1 and S2 in the Supplementary material.
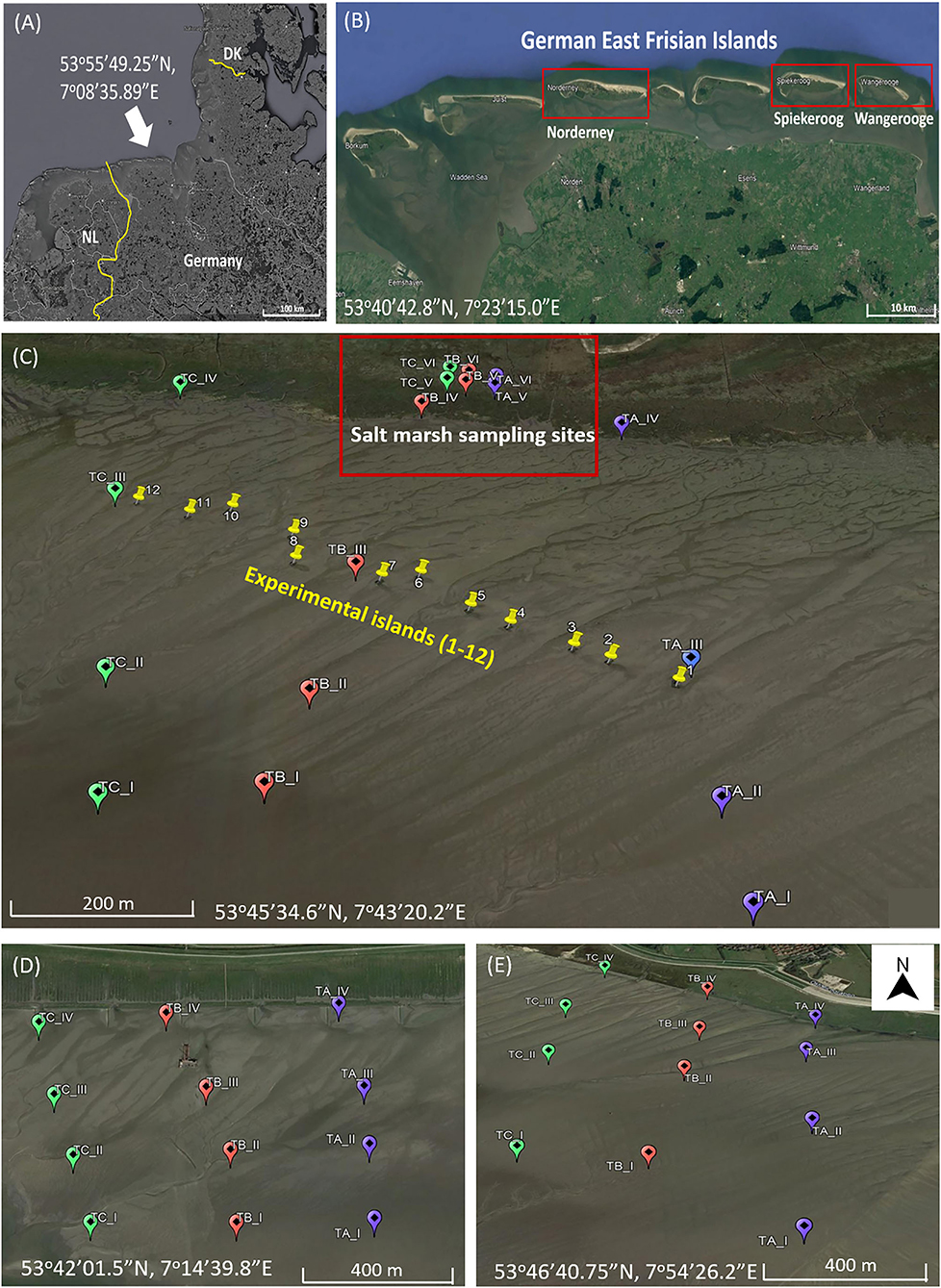
Figure 1. (A) Location of the East Frisian Islands on the north-western coast of Germany, in the state of Lower Saxony. (B) Close-up view (marked in red) of the three East Frisian Islands involved in this study (L-R) Norderney, Spiekeroog, and Wangerooge. (C) Close up image of individual sampling sites (I-VI) and transects A to C on Spiekeroog. Included also are the sites of the twelve experimental islands (shown as yellow pins). (D,E) Close up images of sampling sites on Norderney and Wangerooge, respectively. All images are modified Google Earth satellite images. GPS coordinates of individual sampling sites are listed in the Supplementary material.
Experimental islands
Twelve experimental islands made of steel cages measuring 2 m × 6 m were installed in September 2014 on the back-barrier tidal flat of Spiekeroog (for construction design details and experimental aims, refer to Balke et al., 2017). Each island consists of twelve 1 × 1 m metal cages, of which four cages were joined together to form one of the three elevation levels (70 cm, 100 cm, and 130 cm AMSL). These elevation levels correspond to the pio, LS, and US zones of the natural salt marsh on Spiekeroog. Six of these islands were initially non-vegetated (islands with even numbers in Figure 1C) and were filled with sediments from the surrounding tidal flat. The other six islands (islands with odd numbers; Figure 1C) initially received transplantations of LS marsh vegetation sods (to a depth of 30 cm) from the natural lower salt marsh on Spiekeroog (Balke et al., 2017). For the current study, samples were also taken from all the three elevational levels of the 12 experimental islands.
Sampling and sample preservation
Sampling for total algal biomass, pigment composition, and sediment properties (water and mud content, mean grain size) was performed in spring, summer, and autumn of 2019 (for exact dates, see Supplementary Table S1) during low tide. Cut-off syringes (ϕ 26 mm) were used to extract sediment cores from the tidal flats and the different elevation levels of the experimental islands. Sediment core samples were taken in triplicates per site, and the upper 2 cm of the three extracted cores, containing the majority of phototrophic cells (De Jonge and Colijn, 1994), were pooled and transferred into labeled plastic zipper bags, resulting in a total sediment volume of 31.85 cm3 per site. When sampling within the salt marsh or on experimental islands, extra care was taken to exclude any visible pieces of plant material or detritus from the sampled sediment cores. Sediment samples in the field were kept in the dark and cooled with ice packs in a cooler box. Upon return to the laboratory, sediment samples for pigment extraction were immediately transferred to −80°C, while the samples for other parameters were stored at −20°C.
Temperature
With the exception of spring temperature data from Spiekeroog, all in-situ sediment temperatures at all sampling sites were measured with a hand-held sensor (Fibox 4; PreSens Precision Sensing GmbH, Regensburg, Germany). Due to a technical malfunction with our hand-held sensor during the spring sampling campaign on Spiekeroog, in-situ sediment temperatures were recorded using four HOBO® temperature/light loggers (HOBO® UA-002-64 pendant, Onset® Corporation, Bourne, MA, USA) instead. The loggers were deployed at 2 cm sediment depth, anchored securely into the tidal flat sediment at sites I to IV of transect B, and left in the field to capture light and temperature fluctuations of at least one semidiurnal tidal cycle before retrieval (24 h). Temperature data from the pio zone (site V) and LS marsh (site VI) on Spiekeroog in spring were derived from a long-term monitoring program with permanently installed temperature loggers (Meier et al., 2020). The data were recorded with DEFI-T loggers (JFE Advantech Co., Ltd., Tokyo, Japan), which were installed at 5 cm depth in the salt marsh soil. On the experimental islands, no temperature data could be recorded in spring due to the technical malfunction.
Sediment characteristics
Sediment water content was analyzed in triplicates and determined by drying 5 g of wet sediment at 60°C for 48 h. Water content was defined as the weight ratio before and after drying. Average grain size and mud content was determined using a laser diffraction particle size analyzer (Horiba LA-950; Horiba Europe GmbH, Oberursel, Germany). Prior to analysis, the samples were suspended in a sodium metaphosphate solution (4% w/v) and coarse particles (>2 mm) were removed by sieving. Samples from the salt marsh and experimental islands were additionally treated with sodium peroxide (30% w/v) and heated to remove all organic plant material. The same treatment was not required for samples from the unvegetated tidal flat. On the experimental islands, sediment water content was the only parameter analyzed for all three elevation zones of the 12 islands.
Sediment pigment analysis
Pigment extraction and analysis were performed according to the method by Thrane et al. (2015) with slight modifications as described here: sediment samples were freeze-dried for at least 48 h. Dried samples were homogenized with a spatula and subsamples (~5 g) were directly weighed into pre-tared 15 ml centrifuge tubes. 10 ml of analytical grade 96% ethanol (Art No.: 147194.1212, PanReac AppliChem, Darmstadt, Germany) was added to each sample, followed by mixing samples thoroughly with a vortex mixer, and lastly, ultrasonication on ice for 30 min. Samples were stored at 4°C in the dark for 20–24 h, before centrifugation at 4,000 rpm, 4°C for 30 min. The supernatants (pigment extracts) were measured in triplicates of 330 μl each. Each sample replicate was pipetted into individual wells of a 96-well flat bottom clear polypropylene plate (Greiner bio one #655201). Pigment absorbances were measured using a Synergy MX plate reader (BioTek instruments, Vermont, USA). Spectral scans were performed between 300 and 800 nm with a resolution of 1 nm to determine Chl-a concentration as a proxy for total algal biomass and pigment composition to calculate pigment diversity as a proxy for MPB taxa diversity (see Section Data analysis).
Data analysis
Spectral scans from the plate reader were analyzed in R (R Core Team, 2021) using the script provided by Thrane et al. (2015), based on a modified Gauss-peak spectra (GPS) method that represents individual pigment spectra as weighted sums of Gaussian functions. The method detects biomass concentration of the following pigments (in microgram per gram dry sediment): alloxanthin, beta-carotene, 9′-cis-neoxanthin, trans-canthaxanthin, chlorophyll-a, -b, -c1, and -c2, trans-diadinoxanthin, diatoxanthin, dinoxanthin, trans-echinenone, fucoxanthin, lutein, myxoxanthophyll, peridinin, pheophytin a and b, and violaxanthin. Several of these are commonly used as diagnostic pigments for certain algal taxonomic groups (Supplementary Table S4). Pigment concentrations were then used to calculate the values of effective number of species (ENS; based on pigment and not species diversity), using the R package vegan (Oksanen et al., 2013), where ENS is derived as an inverse Simpson diversity index (1/D). In our case, D = ∑(n/N)2, where “n” represents the concentration of a particular pigment, and “N” represents the total concentration of all pigments measured in a sample. ENS is generally defined as the number of species that would be observed if all of the species in a sample were equally abundant (Jost, 2006). In other words, it is the minimum number of species that is required to achieve the same entropy (diversity) as the observed one. We provide a simple illustrative example here: if pigment A comprises 99% of the concentration and 1% is contributed by pigment B, then pigment richness would be 2, but pigment ENS would be barely larger than 1 as effectively only one pigment dominates. If pigments A and B each comprises 50% of the concentration, pigment ENS would be 2 and the richness is equal. The ENS is a robust diversity measure with regards to sampling and dominance issues, as it weighs elements by their frequency without disproportionately favoring either rare or common elements (Chase and Knight, 2013).
We chose to use the modified GPS method by Thrane et al. (2015) over other methods, because it is a fast, inexpensive, and relatively simple to operate high throughput method for analyzing algal pigment mixtures. Additionally, all aspects from model fitting to data output works well with open-source software (e.g., R). Since the method has already been successfully tested on lake phytoplankton and lake sediments in the original study, we adopted it for analyzing MPB communities in intertidal sediments.
Prior to statistical analyses, Chl-a data was logarithmically transformed and tested with a Shapiro–Wilks test to ensure normality. The hypothesis, H1 was tested using a non-parametric Spearman rank order correlation for algal biomass and pigment diversity. Hypotheses H2–H4 were tested with linear mixed-effects models (LMMs) on predictor variables, using the lmer() function from the R package lme4 (Bates et al., 2014). For H2, the effect of elevation was tested against Chl-a biomass or ENS. The model for the natural transects data set included the transect unit (A–C) nested into island location (Norderney, Spiekeroog, and Wangerooge), and season as random effects. For the experimental islands, H2 and H4 were tested with a single model that included island type (bare or vegetated) and elevation as interacting fixed effects, whereas island number and season were included as random effects. H3 was tested in a separate LMM for Chl-a biomass and ENS, which included temperature and three sediment parameters (mean grain size, sediment water, and mud content) as fixed effects. The random effects were determined as described above. Since the model output for ENS generated a singular fit caused by the lack of spatial variables-associated variance, we simplified the random effects structure down to season alone. Due to the fact that we were unable to obtain all environmental parameters from the experimental islands, the LMM for testing H3 on the experimental islands only included temperature and sediment water content as fixed effects. Additionally, since spring temperature data for the experimental islands was unavailable, only data from two seasons (summer, autumn) was used in the statistical analysis for H3. All statistical analyses were run separately for the natural transects and experimental islands data sets. Marginal and conditional R2 based on Johnson (2014) were calculated to estimate the respective model fit.
Results
In-situ environmental parameters
In-situ sediment temperatures on the tidal flats ranged between 8.8°C (in spring) and 27°C (in summer) and were within a similar range in spring and autumn. Spring temperatures in the salt marsh (sites V and VI) on Spiekeroog were considerably lower than those on the tidal flats, resulting in a high temperature variability across the marine-terrestrial gradient (Supplementary Figure S1). On the experimental islands, sediment temperatures ranged between 11.6°C (in autumn) and 22.7°C (in summer) and were on average higher and more variable on bare islands (Supplementary Figure S2).
The tidal flat on Norderney is characterized by muddy sediments, while the one on Wangerooge is predominantly sandy. On Spiekeroog, the tidal flat is characterized by a mixture of muddy and sandy zones, with sites in the middle of the transect being predominantly sandy, while sites on both the ends of the transect are predominantly muddy (Supplementary Table S3).
Overall, the mean sediment grain size was negatively correlated to increasing elevation (r = −0.26, p = 0.0061, Supplementary Figure S3A). This pattern was mainly driven by the Spiekeroog transect, which had the longest elevational gradient where the mean grain size decreased toward the salt marsh (Supplementary Figure S3B, r = −0.68, p < 0.001), and was much finer within the salt marsh than in the marine tidal flat. On the other two islands, the correlation coefficient between the mean grain size and elevation was weaker on Wangerooge (r = −0.35, p = 0.038) and positively correlated on Norderney (Supplementary Figure S3B, r = 0.37, p = 0.028).
The mean grain size and sediment water content of both the sediments exhibited the highest variation on Spiekeroog and lower variation on the other two islands (Supplementary Figures S4, S5). Results showed that the two parameters were inversely correlated to one another. Across transects, the mud content was not significantly correlated to increasing elevation (r = 0.16, p = 0.1, Supplementary Figure S6A). Individual correlation tests performed for each island indicated that the mud content was positively correlated with elevation only on Spiekeroog (r = 0.71, p < 0.001), mainly driven by the sites sampled in the salt marsh. The other two islands revealed negative and weaker correlations between elevation and mud content (Supplementary Figure S6B, r = −0.35, p = 0.047 for Norderney, r = −0.45, p = 0.0061 for Wangerooge).
Relationship between total algal biomass and pigment diversity (H1)
Algal biomass and ENS showed opposing correlations on the transects and experimental islands, which were additionally marginally non-significant. On the tidal flats, the ENS non-significantly decreased with increasing biomass (Figure 2A, r = −0.17, p = 0.082). The lowest ENS values were observed in summer especially on Wangerooge, and the highest in autumn. On the experimental islands, algal biomass and ENS were non-significantly positively correlated (Figure 2B, r = 0.17, p = 0.084).
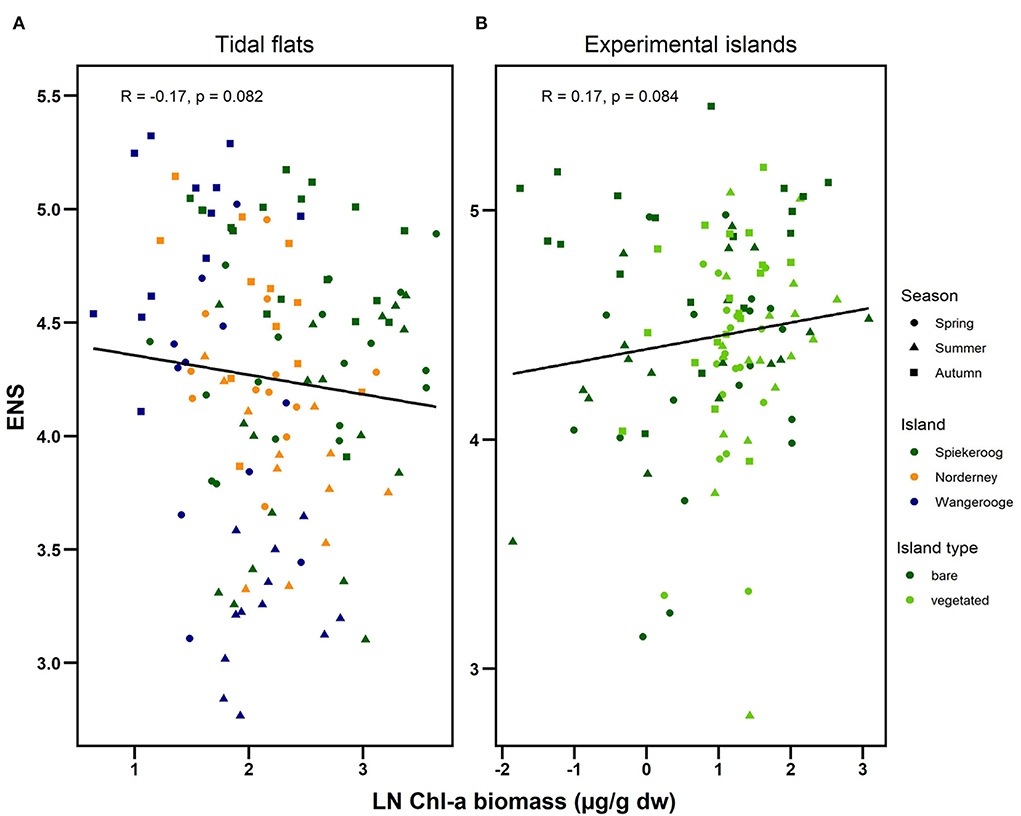
Figure 2. Correlation test results between logarithmic-transformed Chl-a biomass in micrograms per gram dry weight sediment (μg/g dw) and pigment diversity [effective number of species (ENS)] on natural tidal flat transects (A) and on experimental islands (B). Shapes represent seasons and colored symbols represent island location or island type (bare or vegetated experimental islands).
Effect of elevation on total algal biomass and pigment diversity on the natural transects (H2)
Overall, the MPB biomass significantly increased with the increasing elevation (Figure 3A, Table 1), but elevation alone explained only 9.2% of the variance in the biomass, whereas a substantial variation was captured by seasonal and spatial random effects. The elevational effect was mainly driven by high Chl-a concentrations up to 1.25 m on Spiekeroog, but we also observed a decline at the highest elevation (Figure 3A) which matches the decline at the corresponding elevation on the experimental islands (Figure 3B, see below for details).
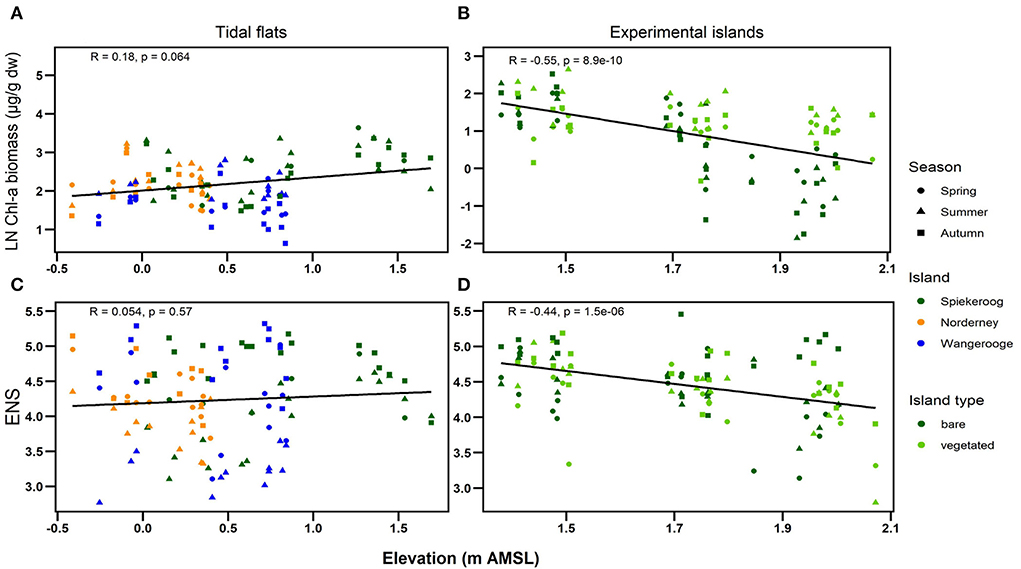
Figure 3. Relationship between logarithmic-transformed Chl-a biomass in micrograms per gram dry weight sediment (μg/g dw) and pigment diversity (ENS) against elevation (meters above mean sea level; m AMSL) on natural tidal flats [left panel; (A,C)] and on experimental islands (bare and vegetated) [right panel; (B,D)].
We found no significant effect of elevation on pigment diversity, but strong variation between seasons (Table 1, Figure 3C). Seasons stood as the reason for the majority of the random effects on ENS, which was the lowest in summer and the highest in autumn.
Relationship of algal biomass and pigment diversity to temperature and sediment characteristics on the natural transects (H3)
Sediment Chl-a significantly increased with temperature (Figure 4A, p = 0.011) and sediment water content (Figure 4E, p = 0.017). The mean grain size had a negative, but only marginally significant effect (Figure 4C, p = 0.07), while the mud content had a positive but non-significant effect (Figure 4G, p = 0.808) on sediment Chl-a biomass. These fixed effects explained 48.8% of the variance in biomass (Table 2). ENS, on the other hand, significantly decreased with temperature (Figure 4B, p < 0.001) and the mean grain size (Figure 4D, p = 0.019), but increased with the sediment mud content (Figure 4H, p = 0.003). The increase in ENS with increasing water content was marginally non-significant (Figure 4F, p = 0.057). These fixed effects accounted for 27.5% of the variance in ENS (Table 2).
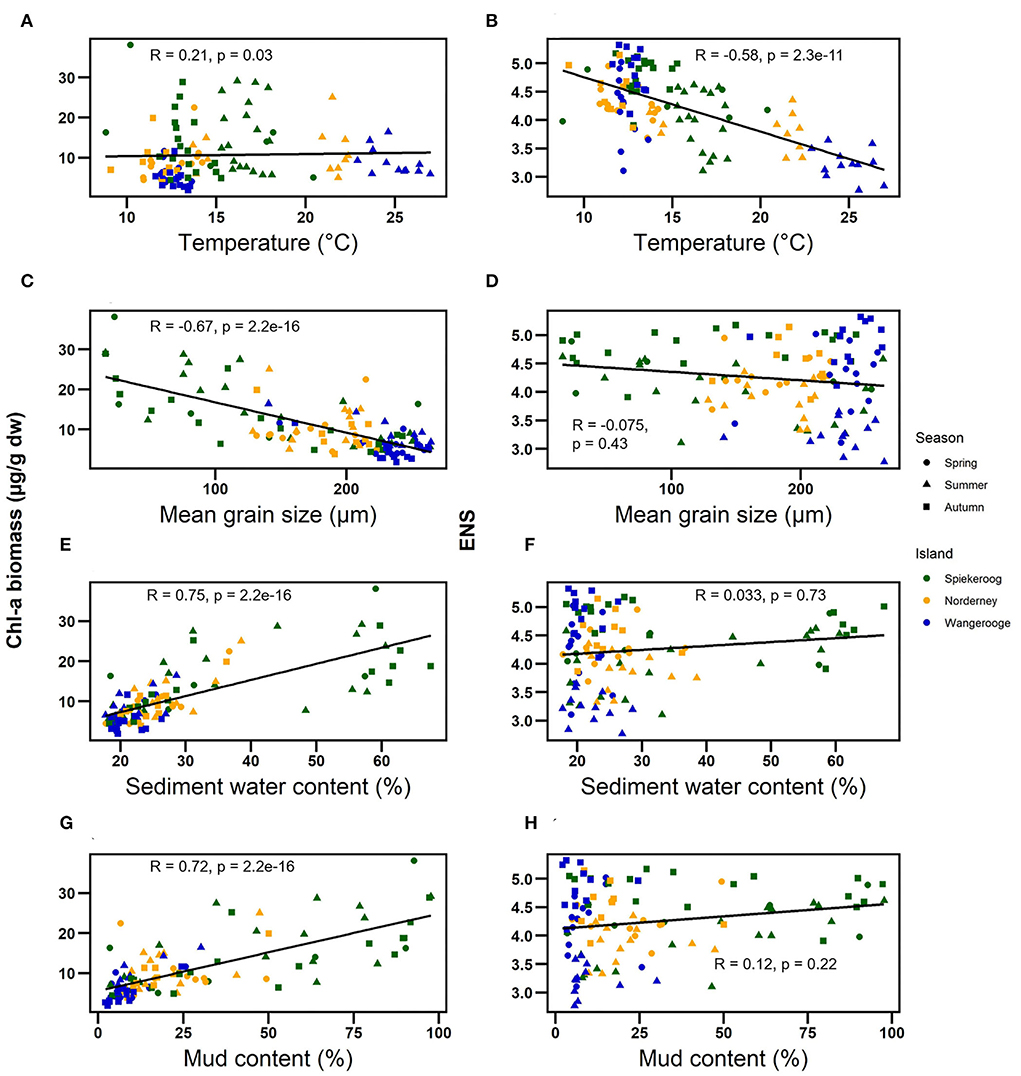
Figure 4. Relationship between Chl-a biomass in micrograms per gram dry weight sediment (μg /g dw) (left panel) and pigment diversity (ENS, right panel) with physical abiotic parameters: temperature (A,B), sediment mean grain size (C,D), sediment water content (E,F) and sediment mud content (G,H) on natural tidal flat transects.
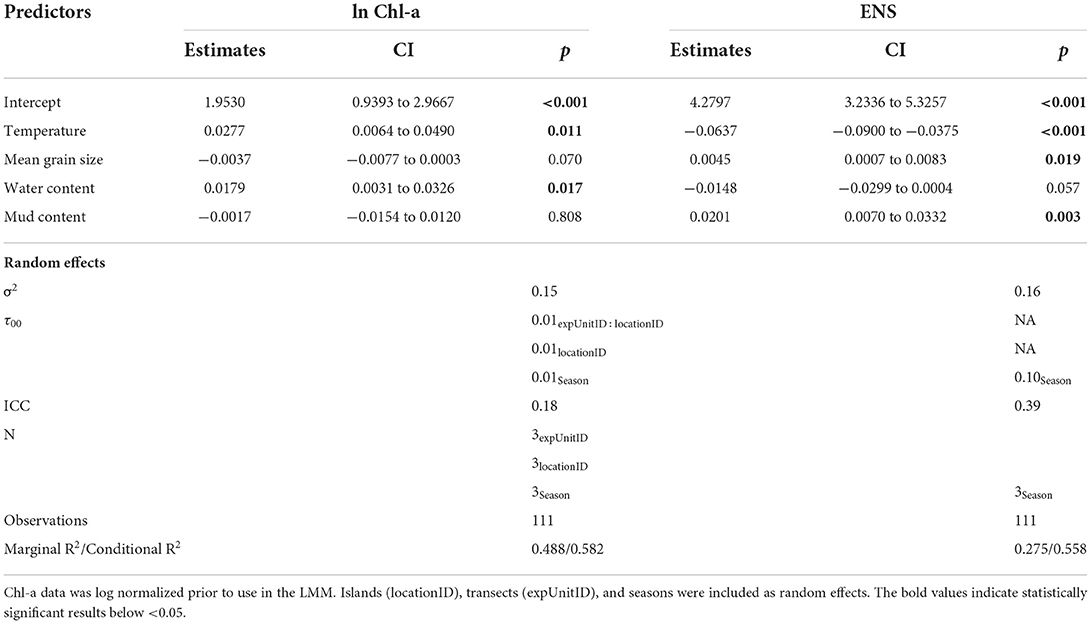
Table 2. Linear mixed effects model output from the analysis of the response variables chlorophyll-a and pigment diversity (ENS) against the predictor variables: temperature, mean grain size, water content, and mud content on natural transects.
Effect of elevation and vegetation on the total algal biomass and pigment diversity on experimental islands (H2, H4)
On the experimental islands, sediment Chl-a biomass was more variable than on the tidal flats and was significantly affected by elevation, island type (vegetated vs. bare), and an interaction of both (p < 0.001, Table 1, marginal R2 = 57.5 %). Biomass significantly decreased with the increasing elevation, being the highest in the pioneer zone and the lowest in the US level (Figure 3B). Comparison of the values between transects (with a maximum elevation of 1.69 m AMSL) and the islands (with a minimum elevation of 1.38 m AMSL) revealed similar total biomass for this section of the elevation gradient present in both data sets (Figures 3A,B). Additionally, the experimental islands continued the negative trend of biomass with elevation that appeared at the upward end of the transects as well. The significant interaction between island type and elevation reflected that the decline in the elevation was much stronger for initially bare islands than the vegetated, which led to overall lower biomass in the US zone of bare islands than the vegetated ones (Figure 3B).
Similarly, ENS on the islands was also in the same range as on the transects, and significantly decreased with the elevation on the experimental islands (Figure 3D, p = 0.002, Table 1). However, this pattern was not affected by interaction with the island type, which was not significantly different (Table 1, marginal R2 = 16.8%).
Relationship of algal biomass and pigment diversity to temperature and sediment water content on experimental islands (H3)
On the experimental islands, Chl-a biomass significantly increased with sediment water content (Figure 5C, p = <0.001) while temperature did not have a significant effect (Figure 5A, p = 0.366). The fixed effects accounted for 39.4% of the variance in biomass and together with the random effect season accounted for 60.2% of the variance (Table 3). Though non-significant, ENS tended to decrease with the increasing temperature (Figure 5B) but increased with the increasing sediment water content (Figure 5D). However, the random effect season contributed substantially to the conditional R2 covering 32.5% of the variance in ENS (Table 3).
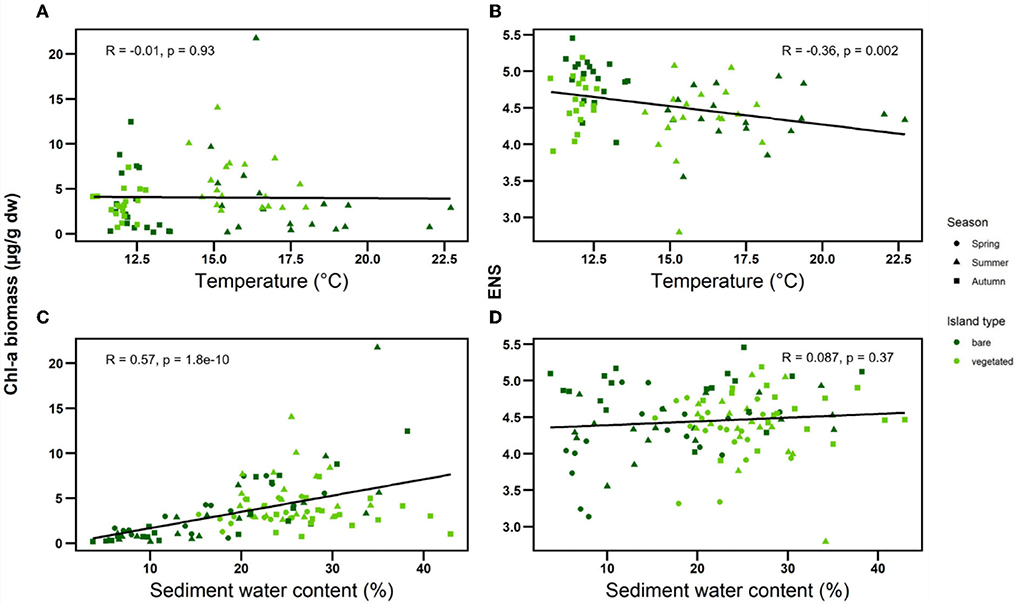
Figure 5. Relationship between Chl-a biomass in micrograms per gram dry weight sediment (μg /g dw) (left panel) and pigment diversity (ENS, right panel) with physical abiotic parameters: temperature (A,B) and sediment water content (C,D) on experimental islands.
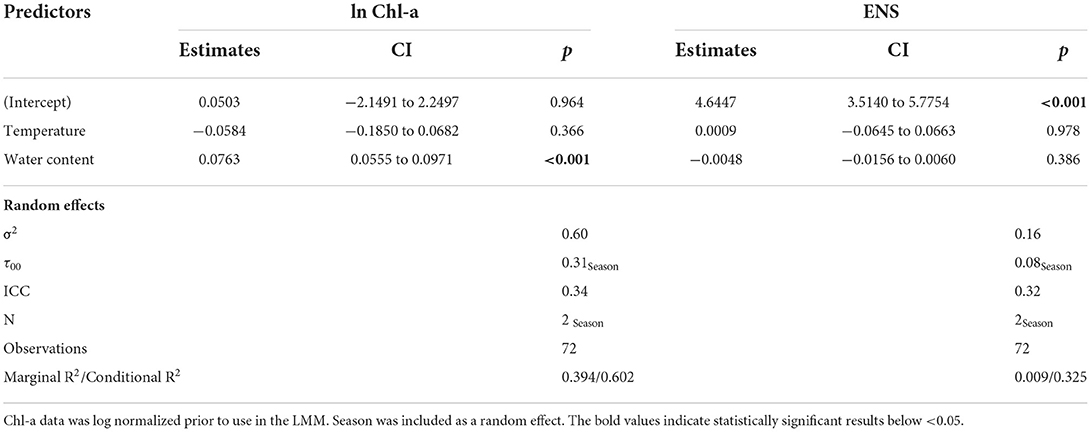
Table 3. Linear mixed effects model output from the analysis of the response variables chlorophyll-a and pigment diversity (ENS) against the predictor variables: temperature and sediment water content on experimental islands.
Pigment composition on natural transects and experimental islands
On the natural transects, the most commonly detected pigments were alloxanthin, beta-carotene, Chl-b, canthaxanthin, diatoxanthin, echinenone, fucoxanthin, peridinin, and the chlorophyll degradation products, pheophytin a and b (Figure 6). Pheophytin a and b were ubiquitous and abundant during all three seasons and at all sites, as were Chl-b, diatoxanthin, and echinenone. The algal biomarker pigments, such as alloxanthin, fucoxanthin, and peridinin displayed seasonal distribution patterns. Alloxanthin was detected in summer and autumn on Spiekeroog and Wangerooge. Fucoxanthin was present in varying percentages on all islands and sites in summer and was only present at certain sites during other seasons. Peridinin was present on Wangerooge at sites II to IV in summer, and at site IV in autumn. A small percentage was only present at site IV in summer on Spiekeroog. Canthaxanthin exhibited a spatial distribution pattern and was found only in the salt marsh (sites V and VI) on Spiekeroog, as well as at site IV in summer. Additionally, low concentrations of canthaxanthin were also detected at sites III and IV on Wangerooge in autumn.
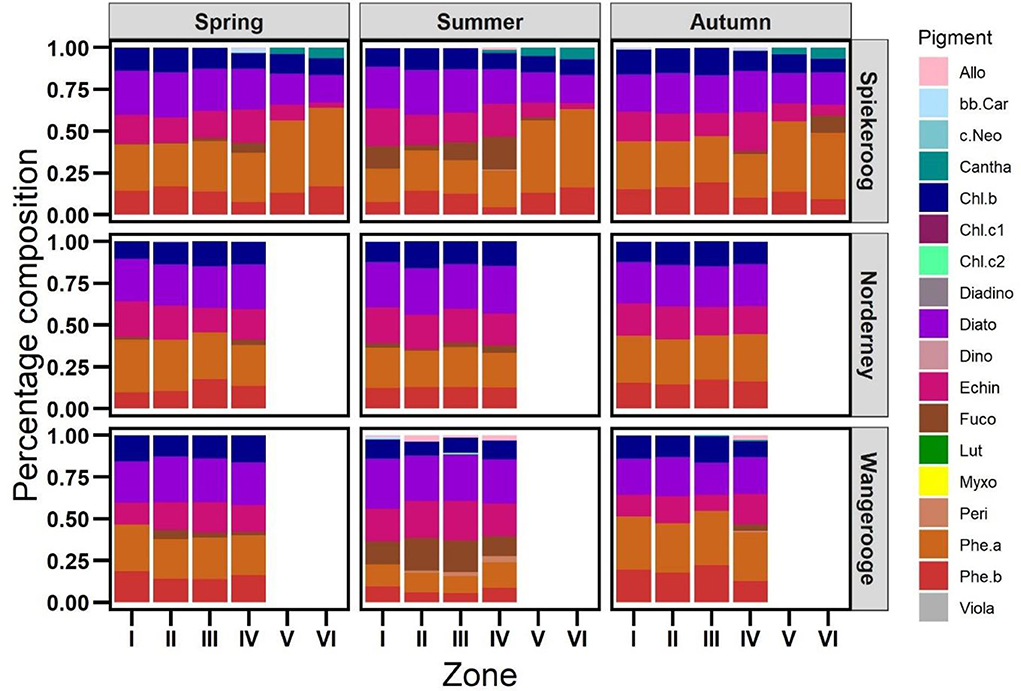
Figure 6. Seasonal pigment:Chl-a biomass ratios (expressed as percentage) calculated for 18 pigments (based on Thrane et al., 2015) from samples sampled from Norderney, Spiekeroog, and Wangerooge (Pigment abbreviations: Allo, Alloxanthin; bb.Car, beta-carotene; c.Neo, 9'-cis-neoxanthin; Cantha, trans-canthaxanthin; Chl.b, chlorophyll-b; Chl.c1, chlorophyll-c1; Chl.c2, chlorophyll-c2; Diadino, trans-diadinoanthin; Diato, Diatoxanthin; Dino, Dinoxanthin; Echin, trans-echinenone; Fuco, Fucoxanthin; Lut, lutein; Myxo, myxoxanthophyll; Peri, Peridinin; Phe.a, pheophytin a; Phe.b, pheophytin b; Viola, violaxanthin).
On the experimental islands, pigment richness was lower than on the natural transects. The chlorophyll degradation products, pheophytin a and b were also ubiquitous across all seasons and elevation levels, accounting for up to 60% of the total pigment biomass (Figure 7). Similar to the transects, Chl-b, diatoxanthin, and echinenone were also ubiquitous. Alloxanthin and peridinin were not detected on the experimental islands. Fucoxanthin represented only a very small percentage of the detected algal pigments, mainly in the LS and US zones in spring and autumn. Canthaxanthin was present in all the three seasons mainly in the US zone of vegetated islands, and in small percentages in the pio and LS zones of vegetated islands in autumn.
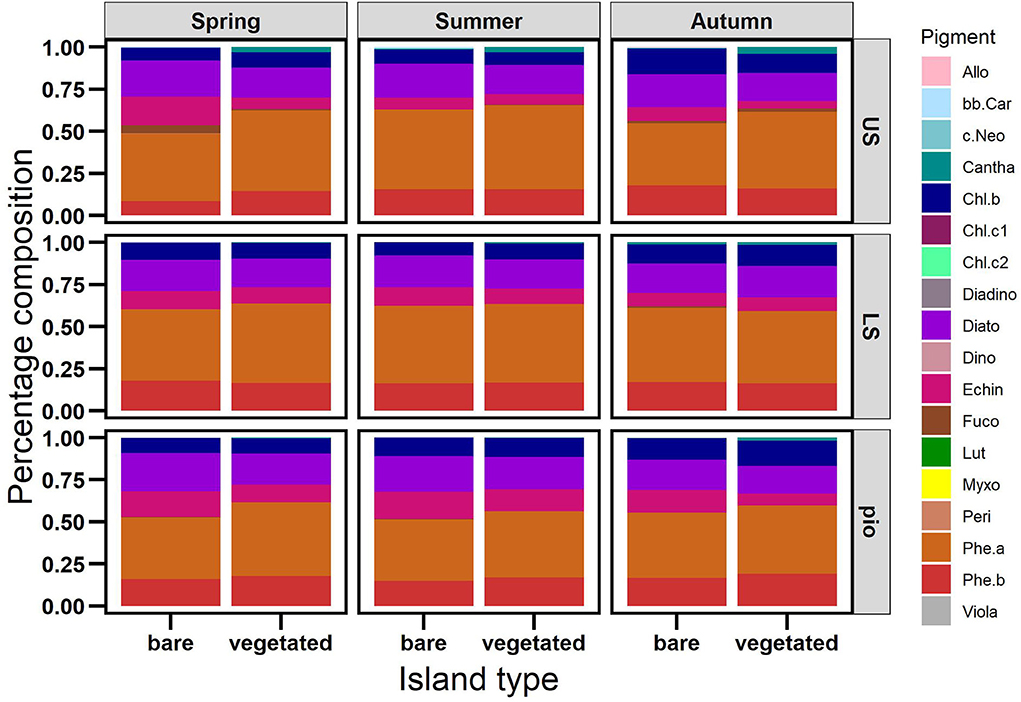
Figure 7. Seasonal pigment:Chl-a ratios (expressed as percentage) on all three elevational levels: pioneer (pio), lower salt marsh (LS), and upper salt marsh (US) of the experimental islands of Spiekeroog (Pigment abbreviations: Allo, Alloxanthin; bb.Car, beta-carotene; c.Neo, 9'-cis-neoxanthin; Cantha, trans-canthaxanthin; Chl.b, chlorophyll-b; Chl.c1, chlorophyll-c1; Chl.c2, chlorophyll-c2; Diadino, trans-diadinoanthin; Diato, Diatoxanthin; Dino, Dinoxanthin; Echin, trans-echinenone; Fuco, Fucoxanthin; Lut, lutein; Myxo, myxoxanthophyll; Peri, Peridinin; Phe.a, pheophytin a; Phe.b, pheophytin b; Viola, violaxanthin).
Discussion
The current study found a weak inverse relationship between the algal biomass and ENS on the natural transects, and an opposing weak trend on the experimental islands. The opposing signs of marginally non-significant trends falsifies hypothesis H1. We found significant effects of elevation on MPB biomass on both the natural transects and experimental islands. The correlations were different (positive on transects, negative on islands, and partly refuting hypothesis H2), but since both covered different parts of the elevational gradient, they jointly converged with an increasing biomass trend up to the pio zone, and then declined toward the upper salt marsh zones. ENS, on the other hand, did not vary with the elevation on the natural transects, but decreased significantly with elevation on the experimental islands (partly refuting hypothesis H2). Environmental factors affected Chl-a biomass and ENS on the natural transects (accepting hypothesis H3). Biomass was higher at higher temperature and in water content and lower grain size (marginally non-significant).ENS significantly decreased with increasing temperature and mean grain size but increased with increasing mud and water content (marginally non-significant). On the experimental islands, vegetation history played a role in biomass (partly supporting hypothesis H4) but not in the ENS (partly refuting hypothesis H4). Chl-a biomass declined with elevation more on bare islands than on initially vegetated ones, leading to lower overall biomass on the former in the US zone (partly supporting hypothesis H4). Pigment composition results showed some spatial and temporal distribution of certain algal pigments on the natural transects, while both spatial and seasonal variations were minimal on the experimental islands.
MPB biomass and pigment diversity patterns
While the inverse relationship of MPB Chl-a biomass and pigment (community) diversity was weak in our study, the general pattern has been observed before in other microphytobenthos communities (Hillebrand and Sommer, 1997; Forster et al., 2006; Ribeiro et al., 2021). It should be noted, though, that the diversity estimates in the cited studies were based mainly on microscopic analyses of diatom assemblages in contrast to the current study using the overall pigment diversity and encompassing all MPB taxa, but at a much lower resolution. Both Colijn and Dijkema (1981) and Forster et al. (2006) demonstrated that high MPB biomass at certain sites was usually associated with single or few dominant (>50% of population) species. However, in our study this relationship was not significant and inconsistent, especially in the pio and LS zones on Spiekeroog, where high Chl-a biomass and high ENS were observed. MPB communities in this transitional zone across the marine-terrestrial interface are more protected from both marine stressors on the tidal flats (inundation, high irradiance, temperature stress, resuspension, turbidity, etc.) and from terrestrial stressors in the salt marsh (mainly from desiccation and high salinity stress) (Pomeroy, 1959; Janousek, 2009). Hence, such transition zones are considered hotspots for MPB biomass and diversity as they combine high nutrient/water retention properties and high organic matter content (Traut, 2005; Janousek, 2009; Ferro and Morrone, 2014). On the experimental islands, terrestrial stressors increase at higher elevational levels, causing a deterioration in conditions for MPB growth and allowing for only highly adapted species to thrive in this environment. We acknowledge that the use of Chl-a as a proxy for algal biomass in sediments has its pitfalls (Baulch et al., 2009; Ramaraj et al., 2013). Sediment Chl-a may not be completely of in-situ MPB origin but it can be derived from allochthonous sources, such as macroalgae, detritus from macrophytes, sedimenting tycho-/phytoplankton, and terrestrial inputs (Lucas and Holligan, 1999; Steinman et al., 2017; Daggers et al., 2020). This can lead to an overestimation of MPB biomass, as might have been the case in the salt marsh samples due to plant debris. Furthermore, degradation products of Chl-a, such as pheophytin a and pheophorbide a can also absorb light under very similar wavelengths as Chl-a (Steinman et al., 2017), and contribute up to 60% of the measured Chl-a content in freshwater samples (Marker, 1980). Additionally, the amount of Chl-a within cells may vary from species to species, and this is further affected by cell physiology, temperature, light, and nutrient supply, as well as other changes in environmental conditions (Schlüter et al., 2006; Barlow et al., 2008; Steinman et al., 2017). The same applies for other accessory pigments, whose ratios within cells may change not only with changing environmental conditions, but also with senescence and grazing (Wright and Jeffrey, 2006; Chao et al., 2016; Lima et al., 2019), leading to changes in pigment composition and diversity without actual changes in cell abundances within the community. Despite these intricacies, Chl-a remains a simple biomass proxy and pigment composition has successfully been used as a proxy for MPB community structure (Lucas and Holligan, 1999; Méléder et al., 2005; Steinman et al., 2017) and diversity (Janousek, 2009).
Currently, three methods are commonly used for determining algal pigment concentrations: spectrophotometry, fluorometry, and high performance liquid chromatography (HPLC) (Steinman et al., 2017). The latter is considered the “golden standard” for determining pigment concentrations at a high resolution in plants and algae, resolving most chlorophylls, carotenoids, and their degradation products. However, this method is costly in terms of time and the equipment required (Mantoura and Llewellyn, 1983; Wright et al., 1991). The original GPS method by Küpper et al. (2000) is conceptually sound, but relies on the use of single-cuvette spectrophotometry and a separate compilation of data and pigment concentration determination, making the method cumbersome and time-consuming (Thrane et al., 2015). The modified GPS method of Thrane et al. (2015) is a faster and relatively simple to operate high throughput method that has been shown to accurately estimate concentrations of total chlorophylls and total carotenoids in both plankton and sediment lake samples. However, individual pigment concentrations (especially carotenoids) can be difficult to resolve due to similarity between their absorbance spectra, also known as aliasing. To alleviate this problem, the authors assessed aliasing by Monte-Carlo simulation of the identification error rate of each pigment and used the information to come up with a “core set” of correctly identifiable pigments that are common in natural samples and less prone to aliasing. However, the potential for aliasing between certain pigments still exists when using any GPS method, so that the interpretation of the estimated pigment concentrations should be regarded with care, also in our study.
HPLC-based methods, such as CHEMTAX, which enable the calculation of the contribution from different taxa to total Chl-a based on pigment:Chl-a ratios, are more comprehensive and sensitive compared to the modified GPS method used in our study. However, the latter is still able to accurately estimate the total Chi-a concentration in samples and the concentrations of eighteen other pigments (with lower accuracy). Pigment:Chl-a mass ratios could still be generated, which we used to gain an insight into the MPB community structure. We understand that the absorption spectra of some phytopigments may overlap and many pigments are not unique to just a single taxon (Wright and Jeffrey, 2006). Therefore, MPB community composition was not a main focus in our study and pigment-based MPB diversity was likely underestimated in our samples. Another aspect to consider is the fact that a large portion (sometimes >50%) of the pigments measured consisted of chlorophyll degradation products (pheophytin a and b) that were also included into the calculations of ENS. However, these two pigments were ubiquitous in all our samples, increasing the pigment diversity uniformly in the entire data set. Despite its pitfalls, we believe that the modified GPS method was suitable to detect the spatial and seasonal diversity changes in major taxonomic groups within MPB communities. However, we would like to stress that a deeper insight into MPB community structure at a higher taxonomic resolution requires other methods, such as microscopic examinations and molecular barcoding to complement any chemotaxonomic method (Paerl et al., 2003; Wright and Jeffrey, 2006).
The use of pigment concentrations for estimating pigment diversity and relating it to the diversity of taxa within a community is an idea adopted from the field of ecology where ENS is used as a diversity measure. ENS is regarded a robust measure for comparing within-community and among-community diversity, as it overcomes several limitations in terms of both diversity indices and species richness (S) (Jost, 2006; Cao and Hawkins, 2019). The use of ENS as a diversity measure allows us to substitute species abundances with concentrations of detected pigments, and the sum of all species abundances for total pigment concentrations. This method seems relevant in all cases where a microscopic analysis of the MPB communities is not included into the scope of the study. To our knowledge, only one study was based on diversity metrics on pigments and the inverse Simpson index (Janousek, 2009), but with lower number of pigments.
Elevation, MPB biomass, and pigment diversity patterns on natural transects
The significant increase in MPB biomass with elevation in the tidal flats matched the findings of previous studies of other European tidal flats (Brotas et al., 1995; van der Wal et al., 2010; Daggers et al., 2020). Upper tidal flats fall dry for a longer period of time during low tide, resulting in higher stability (low resuspension rate) of the sediment, and longer exposure to sunlight, which therefore increases the photosynthetic period (van der Wal et al., 2017; Jacobs et al., 2021). We did not find a significant relationship between ENS and elevation, which is in contrast to previous studies that observed a decrease in MPB diversity with increasing elevation, driven by local in-situ factors, such as sediment grain size and mud content (Scholz and Liebezeit, 2012b; Ribeiro et al., 2021). Other studies related the opposite pattern (increasing diversity with elevation) to stronger wave action and hydrodynamic conditions at the seaward side of the elevation gradient, potentially causing resuspension of MPB, and the mixing of sediments and thus burying MPB cells into deeper sediment layers (Underwood and Kromkamp, 1999; van der Wal et al., 2010; Jacobs et al., 2021). Janousek (2009) also reported higher pigment diversity in the salt marsh compared to the adjacent bare tidal flats, due to the various conditions provided by salt marsh vegetation already described earlier. These studies and our results indicate that elevation patterns across marine-terrestrial transects cannot straightforwardly be generalized for different systems, as these gradients are strongly associated with in-situ environmental and hydrodynamic factors. Therefore, elevation itself is still likely to be a strong determinant in many systems (including our study system) because hydrodynamics and the gradients of many in-situ environmental factors, such as sediment grain size (and in turn water content), organic matter content (not assessed in this study), and temperature, are closely coupled to the elevational gradient.
Environmental factors, MPB biomass, and pigment diversity patterns on natural transects
Positive relationships between MPB biomass with temperature, and water content have frequently been observed beyond our study system (Colijn and Dijkema, 1981; Lucas and Holligan, 1999; Scholz and Liebezeit, 2012b; Ribeiro et al., 2021). Seasonal variability in MPB biomass and productivity has been related to changes in irradiance and temperature, nutrient availability, and sediment composition in other systems (Colijn and Van Buurt, 1975; De Jonge and Colijn, 1994; Montani et al., 2003; van der Wal et al., 2010), indicating that the determinants for MPB biomass are highly system-specific. Since some of the sediment characteristics vary in a multicollinear way, we suggest some caution in interpreting the LMM results (Gareth et al., 2013). Comparing the LMM results (Table 2) to the bivariate patterns (Figure 4) reveals that the apparent positive association between biomass and mud content did not appear in the LMM, potentially reflecting a similar relationship for water content that is correlated with mud content.
A combination of several forces, such as tidal currents, waves and associated winds, and drainage influences the hydrodynamics on intertidal flats (Le Hir et al., 2000). Hydrodynamics can influence sediment erosion and deposition processes, the distribution of sediment grain sizes and types, and organic matter accumulation. Additionally, factors, such as sediment input and bathymetry can also impact sedimentation rates (Morris et al., 2002; Fagherazzi et al., 2014). In general, sediment grain size decreases with increasing elevation due to reduced wave action, which allows for fine particles like silt and clay to settle close to the salt marshes (Brotas et al., 1995; van der Wal et al., 2017; Jacobs et al., 2021). Finer muddier sediments are associated with higher organic matter, water, and nutrient content, which support higher MPB biomass (Pomeroy, 1959; Brotas et al., 1995; Barranguet et al., 1998; Thornton et al., 2002). Coarser sediments, in contrast, tend to be deposited in areas characterized by higher tidal and wave action. The stronger hydrodynamic forces result in higher instability, export and resuspension of sediment and MPB material, and lower organic matter content (Barranguet et al., 1998). Consequently, Chl-a biomass is often lower at high energy sites.
Tidal currents resuspend and transport sediments to the salt marsh during high slack water, where fine cohesive particles get deposited due to the strong friction of the salt marsh vegetation canopy (Zhang et al., 2019). Salt marsh plants maintain the salt marsh elevation not only by trapping sediment during periods of inundation, but also by building biomass and accumulating organic matter, constantly modifying the elevation in response to the mean sea level (Morris et al., 2002). Climate change induced increases in flooding and storm events will likely lead to longer inundation duration in salt marshes, resulting in either positive or negative responses in vegetation biomass and organic matter accretion, depending on marsh height and associated sediment loads. Hence, future salt marshes will either be able to keep up with the sea level rise, or ultimately drown and be lost to the sea (Kirwan and Guntenspergen, 2012). Future changes in hydrodynamics, potentially resulting in altered elevation gradients and salt marsh heights will also likely impact MPB community dynamics in salt marsh-mudflat systems.
In addition to the conditions associated with finer muddier sediments, the presence of plants in the salt marshes also creates a favorable environment for MPB growth. Sediment is stabilized by the root systems of these plants and is therefore not as erosion-prone as on the open tidal flats (Chen et al., 2012). This positive relationship between MPB biomass and vegetated habitats in contrast to unvegetated tidal flats has also been found before (Pomeroy, 1959; Facca and Sfriso, 2007; Janousek, 2009). Whitcraft and Levin (2007) showed that experimental plots with salt marsh vegetation exhibited lower sediment temperature, as well as higher water content due to shading. Since the US marsh is rarely inundated, and if it occurs, only briefly during the highest spring tides or storm events; the US marsh sediments can become very dry and subject to high salinity stress. Thus, the US marsh becomes unsuitable for algal growth, especially during the hotter and drier summer months (Zedler, 1980).
Effective number of species was also significantly influenced by temperature and sediment characteristics, i.e., mean grain size and mud content. The negative relationship with temperature supported previous findings that show that warmer temperatures often favor dominance of certain MPB groups (Sin et al., 2009; Cartaxana et al., 2015; Ribeiro et al., 2021), whereas diatoms thrive only at lower temperatures (Watermann et al., 1999; Underwood, 2001; Scholz and Liebezeit, 2012b). Janousek (2009) and Semcheski et al. (2016) reported higher MPB diversity in muddy sediments than in sandy sediments, a result of the depository nature of more protected muddy sites, and higher resistance of MPB biofilms on muddy sediments against erosion (Cahoon et al., 1999; Herman et al., 2001). Likewise, Scholz and Liebezeit (2012b) demonstrated that diversity decreases in coarser sediments, while other studies found higher diversity in sandbars than in mudflats from mixed assemblages (Paterson and Hagerthey, 2001; Underwood and Barnett, 2006; Jesus et al., 2009; Rivera-Garcia et al., 2018). These studies indicate that diversity patterns in relation to sediment types may differ, depending on how diversity was defined in the respective study (species or pigments), what communities were targeted (diatoms vs. mixed communities), and how samples were analyzed (chemotaxonomy, microscopy, and counting methods). Janousek (2009) dealt with pigment composition, whilst Semcheski et al. (2016) used the Utermöhl method on sediment samples and Scholz and Liebezeit (2012b) focused on the non-diatom MPB fractions. Sediment type and grain size can also greatly affect light availability for MPB cells within sediments, as well as porewater nutrient content and water retention capabilities during low tide, which in turn determine the MPB composition and diversity (Barranguet et al., 1998; Watermann et al., 1999; Billerbeck et al., 2007).
Aside from abiotic conditions, benthic herbivore grazing may largely control MPB biomass and diversity in tidal flat habitats, especially in summer (Admiraal and Peletier, 1980; Colijn and Dijkema, 1981; MacIntyre et al., 1996; Daggers et al., 2020). Grazing pressure resulted in higher MPB diversity in some studies (Sommer, 1999; Hagerthey et al., 2002; Balvanera et al., 2006) and was shown to be higher in sandy sediments than in muddier sediments (Underwood and Kromkamp, 1999; Herman et al., 2001), indicating the interactive effects of sediment structure and grazing pressure on MPB.
Environmental factors, MPB biomass, and pigment diversity patterns on the experimental islands
Both MPB biomass and ENS significantly decreased with increasing elevation on the experimental islands. This decline in MPB biomass from the pio zone to the US marsh has been well-documented in other studies (Pomeroy, 1959; Zedler, 1980; Thornton and Visser, 2009). The experimental islands support these results and the decrease in biomass toward the US zone is in line with observations from the upper end of the natural transect. When comparing MPB biomass patterns on natural transects and experimental islands, the pio zone promotes the highest MPB biomass. In the seaward direction, marine stressors increase (leading to the positive relationship between MPB biomass and elevation), and toward the salt marsh, the terrestrial stressors increase (leading to the negative relationship between elevation and MPB biomass on the experimental islands). Additionally, the experimental islands show a clear interaction between vegetation cover history and the biomass-elevation correlation, reflected by a significant interaction term in the LMM. We postulate that the initial vegetation buffered some of the negative impacts of high elevation described above (desiccation and salinity stress), leading to higher biomass in vegetated than bare islands on the highest elevational US zone. The lack of a significant effect of temperature on Chl-a biomass suggests that in addition to the other benefits already discussed, vegetation cover also has a buffering effect on temperature conditions on the islands. In addition, the positive and strong correlation between sediment water content and MPB biomass, supported the patterns on the natural transects. Though non-significant, the relationship trends of temperature and sediment water content with ENS were also similar to those on the natural transects. Hence, these results indicate that conditions on the experimental islands were comparable to those on the natural transects. Furthermore, we saw that there was no significant difference in the ENS between vegetated and bare islands, which also hints toward the buffering effect of vegetation on abiotic conditions on the experimental islands. Nevertheless, we acknowledge that the environmental parameters data set for the experimental islands was incomplete and consider the conclusions to be preliminary as the LMMs can change with the increasing number of observations and variables.
Pigment composition patterns on natural transects and experimental islands
Pigment composition revealed some distinct spatial and temporal patterns, which were more pronounced on the natural transects than on the experimental islands. Our results showed that canthaxanthin, which has been used as a marker for heterocystous cyanobacteria (Charette and Prepas, 2003; Krajewska et al., 2019), was only detected in the salt marsh (sites V and VI) and at the marine-terrestrial boundary zone (site IV). This indicates that heterocystous cyanobacteria were more distributed in salt marsh MPB communities, an observation also commonly reported for other temperate salt marsh systems (Tracy and South, 1989; Calvo et al., 1999; Janousek, 2009). However, higher plants can also be a source of canthaxanthin (Naz et al., 2021); therefore, our results should be regarded with care despite our efforts to remove all visible plant material from sampled cores. The presence of canthaxanthin only on Spiekeroog was likely due to the fact that only the Spiekeroog transects incorporated saltmarsh sites in contrast to the other two islands comprising only tidal flat samples. Alloxanthin and peridinin were present only in summer and autumn and suggest the succession of cryptophytes and dinoflagellates in the MPB communities. The succession of these algal groups in the Wadden Sea MPB communities during the warmer months was also reported by Scholz and Liebezeit (2012b), using microscopic analyses. The prevalence of fucoxanthin in our samples indicating the presence of diatoms mostly in summer did not match the results of other studies focusing on MPB pigment composition, where fucoxanthin dominated the MPB community all year round (Méléder et al., 2005; Ribeiro et al., 2021). Other microscopy-based studies found diatoms to dominate MPB communities in spring and autumn (Barranguet et al., 1997; Scholz and Liebezeit, 2012b), or in spring and summer (Semcheski et al., 2016) (together with other algae). However, the carotenoid diatoxanthin formed a substantial portion of the pigment mixture across all seasons and locations in our study, indicating the presence of diatoms, dinoflagellates, and/or haptophytes. Since diatoms are the dominant group in the Wadden Sea MPB communities, it is feasible that the diatoxanthin measured in our samples was mostly of diatom origin. In that case, diatoms indeed formed a large percentage of our MPB communities during all three seasons and at most sites, similar to the studies mentioned above. A few studies (Lucas and Holligan, 1999; Méléder et al., 2005; Jesus et al., 2009) found an increase in cyanobacteria pigments in the tidal flat sediments during summer; however, this was not observed in our study, as echinenone was present in all the three seasons. Overall, our pigment composition results showed that MPB taxonomic groups varied not just seasonally and among islands, but also along transects, which was also observed in studies by Colijn and Nienhuis (1977) and Scholz and Liebezeit (2012b).
On the experimental islands, seasonal variations in pigment composition for alloxanthin, fucoxanthin, and peridinin were not observed as on the natural transects. This suggests that MPB communities on the experimental islands were likely not of the same composition as on the transects and also not much influenced by seasons, although the LMM results showed that seasons had a substantial (accounted for >30% of the variance) effect on ENS. Pigment composition results here were non-conclusive and offered little information about the seasonal and elevation differences in MPB communities.
Overall, we would like to emphasize that the pigment composition results presented here provide only a rough insight into the community structure and are not intended to serve as a definitive species inventory. Most MPB pigment composition studies use the HPLC method and thus hampers their comparison with our data. As we can draw only limited conclusions regarding algal group composition from pigment composition using the modified GPS method, we explicitly did not include MPB community structure in our analysis, but only ENS.
Outlook
The current study enabled us to compare spatial and temporal MPB patterns in natural tidal flat transects and the salt marsh with artificial islands, and their specific manipulation of initial plant cover. Our major findings comprised of the decline in algal biomass with more stressful marine and terrestrial conditions, leading to a convergence of peak biomass in the pioneer zone of the salt marsh, right at the marine-terrestrial transition zone. We further showed that environmental stress in the terrestrial environment can be mediated by vegetation cover. Nevertheless, our study can only be a first survey generating new hypotheses that need to be tested with more detailed analyses of species composition and their traits in relation to the environmental variables. Despite some pitfalls in the modified GPS method, we believe that this method was sufficient and suitable for detecting spatial and seasonal changes in MPB biomass and the diversity of major taxonomic groups within MPB communities.
Data availability statement
The raw data supporting the conclusions of this article will be made available by the authors, without undue reservation.
Author contributions
SM, HH, SR, and IK contributed to conception and design of the study. JY and HH performed the statistical analysis. JD performed the sediment characteristics analysis. JY, MM, JD, KL, and DP collected data for the manuscript. JY wrote the first draft of the manuscript. SM, HH, and MM wrote sections of the manuscript. All authors contributed to manuscript revision, read, and approved the submitted version.
Funding
The DynaCom Research Unit was funded by German Research Foundation (DFG FOR 2715), the microphytobenthos subproject under the funding number MO 1931/5-1, and Bibliotheks- und Informationssystem (BIS) of the University of Oldenburg partially funded the publishing costs for this publication.
Acknowledgments
We would like to express our gratitude to the workshop staff of the Institute for Chemistry and Biology of the Marine Environment (ICBM), especially Helmo Nicolai, Gerrit Behrens, and Waldemar Siewert for their assistance in the logistics, provision of specially modified equipment and materials during our field sampling campaigns. In addition, special thanks to the staff from the Lower Saxony Wadden Sea National Park, Nationalpark-Haus Wittbülten am Internat Hermann Lietz-Schule on Spiekeroog, and the NLWKN Betriebstelle Norderney for granting us permission and access to the field, and for their logistic support and advice.
Conflict of interest
The authors declare that the research was conducted in the absence of any commercial or financial relationships that could be construed as a potential conflict of interest.
Publisher's note
All claims expressed in this article are solely those of the authors and do not necessarily represent those of their affiliated organizations, or those of the publisher, the editors and the reviewers. Any product that may be evaluated in this article, or claim that may be made by its manufacturer, is not guaranteed or endorsed by the publisher.
Supplementary material
The Supplementary Material for this article can be found online at: https://www.frontiersin.org/articles/10.3389/fevo.2022.956092/full#supplementary-material
Supplementary Figure S1. In-situ sediment temperatures measured along sampling transects (zone I to IV on Norderney and Wangerooge, and zone I to VI on Spiekeroog). Values were averaged values from three transects. *Due to a technical fault with the temperature probe, temperatures for spring on Spiekeroog could not be measured in triplicates. Data shown were derived from backup HOBO temperature loggers.
Supplementary Figure S2. In-situ sediment temperatures measured on the experimental islands of Spiekeroog, on all three elevational levels [pioneer (pio), lower salt marsh (LS) marsh, upper salt marsh (US) marsh], during the summer and autumn of 2019. Temperature data are not available for spring due to a technical fault in our measurement device.
Supplementary Figure S3. (A) General relationship between sediment mean grain size (in micrometers) and elevation (meters above mean sea level) on Spiekeroog, Norderney, and Wangerooge. (B) Correlations between elevation (meters above mean sea level) and sediment mean grain size (in micrometers) correlated individually for each of the sampled East Frisian Islands.
Supplementary Figure S4. Overview of sediment mean grain size (averaged between three transects) along the sampling sites (zones I–IV) on Norderney and Wangerooge, and along sampling sites (zones I–VI) on Spiekeroog.
Supplementary Figure S5. Overview of sediment water content (averaged between three transects) along the sampling sites (zones I–IV) on Norderney and Wangerooge, and along sampling sites (zones I-VI) on Spiekeroog.
Supplementary Figure S6. (A) General relationship between mud content (%) and elevation (meters above mean sea level) on Spiekeroog, Norderney. and Wangerooge. (B) Correlations between elevation (meters above mean sea level; m AMSL) and mud content (%) correlated individually for each of the sampled East Frisian Islands.
Supplementary Table S1. Sampling dates of the sampling campaigns in 2019.
Supplementary Table S2. List of all sampling sites on the three East Frisian Islands and corresponding GPS coordinates (in decimal degrees). Exp. Isl, experimental island; pio, pioneer zone; LS, lower saltmarsh; US, upper saltmarsh.
Supplementary Table S3. Mean and standard deviation values for abiotic environmental parameters measured and analyzed on all three islands and seasons. Standard deviation values for spring temperature data from Spiekeroog, and autumn temperature data from Norderney are unavailable, due to a technical fault in our measurement device in the field. pio, pioneer zone; LS, lower saltmarsh.
References
Admiraal, W., and Peletier, H. (1980). Influence of seasonal variations of temperature and light on the growth rate of cultures and natural populations of intertidal diatoms. Mar. Ecol. Prog. Ser. 2, 35–43. doi: 10.3354/meps002035
Baillie, P. W., and Welsh, B. L. (1980). The effect of tidal resuspension on the distribution of intertidal epipelic algae in an estuary. Estuar. Coast. Mar. Sci. 10, 165–180. doi: 10.1016/S0302-3524(80)80056-9
Balke, T., Lõhmus, K., Hillebrand, H., Zielinski, O., Haynert, K., Meier, D., et al. (2017). Experimental salt marsh islands: a model system for novel metacommunity experiments. Estuar. Coast. Shelf Sci. 198, 288–298. doi: 10.1016/j.ecss.2017.09.021
Balvanera, P., Pfisterer, A. B., Buchmann, N., He, J.-S., Nakashizuka, T., Raffaelli, D., et al. (2006). Quantifying the evidence for biodiversity effects on ecosystem functioning and services. Ecol. Lett. 9, 1146–1156. doi: 10.1111/j.1461-0248.2006.00963.x
Barlow, R., Kyewalyanga, M., Sessions, H., van den Berg, M., and Morris, T. (2008). Phytoplankton pigments, functional types, and absorption properties in the Delagoa and Natal Bights of the Agulhas ecosystem. Estuar. Coast. Shelf Sci. 80, 201–211. doi: 10.1016/j.ecss.2008.07.022
Barranguet, C., Herman, P., and Sinke, J. (1997). Microphytobenthos biomass and community composition studied by pigment biomarkers: importance and fate in the carbon cycle of a tidal flat. J. Sea Res. 38, 59–70. doi: 10.1016/S1385-1101(97)00032-4
Barranguet, C., Kromkamp, J., and Peene, J. (1998). Factors controlling primary production and photosynthetic characteristics of intertidal microphytobenthos. Mar. Ecol. Prog. Ser. 173, 117–126. doi: 10.3354/meps173117
Bates, D., Mächler, M., Bolker, B., and Walker, S. (2014). Fitting linear mixed-effects models using lme4. arXiv preprint arXiv:1406.5823. doi: 10.18637/jss.v067.i01
Baulch, H. M., Turner, M. A., Findlay, D. L., Vinebrooke, R. D., and Donahue, W. F. (2009). Benthic algal biomass—measurement and errors. Can. J. Fish. Aquat. Sci. 66, 1989–2001. doi: 10.1139/F09-122
Billerbeck, M., Røy, H., Bosselmann, K., and Huettel, M. (2007). Benthic photosynthesis in submerged Wadden Sea intertidal flats. Estuar. Coast. Shelf Sci. 71, 704–716. doi: 10.1016/j.ecss.2006.09.019
Brito, A. C., Benyoucef, I., Jesus, B., Brotas, V., Gernez, P., Mendes, C. R., et al. (2013). Seasonality of microphytobenthos revealed by remote-sensing in a South European estuary. Cont. Shelf Res. 66, 83–91. doi: 10.1016/j.csr.2013.07.004
Brotas, V., Cabrita, T., Portugal, A., Serôdio, J., and Catarino, F. (1995). “Spatio-temporal distribution of the microphytobenthic biomass in intertidal flats of Tagus Estuary (Portugal),” in Space Partition Within Aquatic Ecosystems (Berlin: Springer), 93–104. doi: 10.1007/978-94-011-0293-3_8
Cabrita, M. T., and Brotas, V. (2000). Seasonal variation in denitrification and dissolved nitrogen fluxes in intertidal sediments of the Tagus estuary, Portugal. Mar. Ecol. Prog. Ser. 202, 51–65. doi: 10.3354/meps202051
Cahoon, L. B., Nearhoof, J., and Tilton, C. (1999). Sediment grain size effect on benthic microalgal biomass in shallow aquatic ecosystems. Estuaries 22, 735–741. doi: 10.2307/1353106
Calvo, S., Bárbara, I., and Cremades, J. (1999). Benthic algae of salt-marshes (Corrubedo Natural Park, NW Spain): the Flora. Bot Marina 42, 343–53. doi: 10.1515/BOT.1999.039
Cao, Y., and Hawkins, C. P. (2019). Weighting effective number of species measures by abundance weakens detection of diversity responses. J. Appl. Ecol. 56, 1200–1209. doi: 10.1111/1365-2664.13345
Cartaxana, P., Vieira, S., Ribeiro, L., Rocha, R. J., Cruz, S., Calado, R., et al. (2015). Effects of elevated temperature and CO2 on intertidal microphytobenthos. BMC Ecol. 15, 1–10. doi: 10.1186/s12898-015-0043-y
Chao, C., Tao, J., Cen, J., Wei, G., and Lu, S. (2016). Phytoplankton pigments and functional community structure in relation to environmental factors in the Pearl River Estuary. Oceanologia 58, 201–211. doi: 10.1016/j.oceano.2016.03.001
Chapman, M., Tolhurst, T., Murphy, R., and Underwood, A. (2010). Complex and inconsistent patterns of variation in benthos, micro-algae and sediment over multiple spatial scales. Mar. Ecol. Prog. Ser. 398, 33–47. doi: 10.3354/meps08328
Charette, T., and Prepas, E. E. (2003). Wildfire impacts on phytoplankton communities of three small lakes on the Boreal Plain, Alberta, Canada: a paleolimnological study. Can. J. Fish. Aquat. Sci. 60, 584–593. doi: 10.1139/f03-049
Chase, J. M., and Knight, T. M. (2013). Scale-dependent effect sizes of ecological drivers on biodiversity: why standardised sampling is not enough. Ecol. Lett., 16, 17–26. doi: 10.1111/ele.12112
Chen, Y., Thompson, C., and Collins, M. (2012). Saltmarsh creek bank stability: biostabilisation and consolidation with depth. Cont. Shelf Res. 35, 64–74. doi: 10.1016/j.csr.2011.12.009
Colijn, F., and Dijkema, K. S. (1981). Species composition of benthic diatoms and distribution of chlorophyll a on an intertidal flat in the Dutch Wadden Sea. Mar. Ecol. Prog. Ser. 4, 9–21. doi: 10.3354/meps004009
Colijn, F., and Nienhuis, H. (1977). The intertidal microphytobenthos of the 'Hohe Weg' shallows in the German Wadden Sea. Forschung. Nord. Jahresb. 29, 149–174.
Colijn, F., and Van Buurt, G. (1975). Influence of light and temperature on the photosynthetic rate of marine benthic diatoms. Mar. Biol. 31, 209–214. doi: 10.1007/BF00387148
Daggers, T. D., van Oevelen, D., Herman, P. M., Boschker, H. T., and van der Wal, D. (2020). Spatial variability in macrofaunal diet composition and grazing pressure on microphytobenthos in intertidal areas. Limnol. Oceanogr. 65, 2819–2834. doi: 10.1002/lno.11554
De Jonge, V., and Colijn, F. (1994). Dynamics of microphytobenthos biomass in the Ems estuary. Mar. Ecol. Prog. Ser. 104, 185–196. doi: 10.3354/meps104185
Dittmann, S. (2012). The Wadden Sea Ecosystem: Stability Properties and Mechanisms. Berlin: Springer Science and Business Media.
Dong, L., Thornton, D., Nedwell, D., and Underwood, G. (2000). Denitrification in sediments of the River Colne estuary, England. Mar. Ecol. Prog. Ser. 203, 109–122. doi: 10.3354/meps203109
Du, G., Son, M., Yun, M., An, S., and Chung, I. K. (2009). Microphytobenthic biomass and species composition in intertidal flats of the Nakdong River estuary, Korea. Estuar. Coast. Shelf Sci. 82, 663–672. doi: 10.1016/j.ecss.2009.03.004
Du, G., Yan, H., and Dupuy, C. (2017). Microphytobenthos as an indicator of environmental quality status in intertidal flats: case study of coastal ecosystem in Pertuis Charentais, France. Estuar. Coast. Shelf Sci. 196, 217–226. doi: 10.1016/j.ecss.2017.06.031
Dyer, K., Christie, M., and Wright, E. (2000). The classification of intertidal mudflats. Cont. Shelf Res. 20, 1039–1060. doi: 10.1016/S0278-4343(00)00011-X
Facca, C., and Sfriso, A. (2007). Epipelic diatom spatial and temporal distribution and relationship with the main environmental parameters in coastal waters. Estuar. Coast. Shelf Sci. 75, 35–49. doi: 10.1016/j.ecss.2007.03.033
Fagherazzi, S., Mariotti, G., Banks, A. T., Morgan, E. J., and Fulweiler, R. W. (2014). The relationships among hydrodynamics, sediment distribution, and chlorophyll in a mesotidal estuary. Estuar. Coast. Shelf Sci. 144, 54–64. doi: 10.1016/j.ecss.2014.04.003
Ferro, I., and Morrone, J. J. (2014). Biogeographical transition zones: a search for conceptual synthesis. Biol. J. Linn. Soc. 113, 1–12. doi: 10.1111/bij.12333
Forster, R., Créach, V., Sabbe, K., Vyverman, W., and Stal, L. (2006). Biodiversity-ecosystem function relationship in microphytobenthic diatoms of the Westerschelde estuary. Mar. Ecol. Prog. Ser. 311, 191–201. doi: 10.3354/meps311191
Gareth, J., Witten, D., Hastie, T., and Tibshirani, R. (2013). An Introduction to Statistical Learning: With Applications in R (1st ed.). New York, NY: Springer Publishing Company.
Hagerthey, S. E., Defew, E. C., and Paterson, D. M. (2002). Influence of Corophium volutator and Hydrobia ulvae on intertidal benthic diatom assemblages under different nutrient and temperature regimes. Mar. Ecol. Prog. Ser. 245, 47–59. doi: 10.3354/meps245047
Herman, P. M., Middelburg, J. J., and Heip, C. H. (2001). Benthic community structure and sediment processes on an intertidal flat: results from the ECOFLAT project. Cont. Shelf Res. 21, 2055–2071. doi: 10.1016/S0278-4343(01)00042-5
Hillebrand, H., Kahlert, M., Haglund, A.-L., Berninger, U.-G., Nagel, S., and Wickham, S. (2002). Control of microbenthic communities by grazing and nutrient supply. Ecology 83, 2205–2219. doi: 10.1890/0012-9658(2002)083[2205:COMCBG]2.0.CO;2
Hillebrand, H., and Sommer, U. (1997). Response of epilithic microphytobenthos of the Western Baltic Sea to in situ experiments with nutrient enrichment. Mar. Ecol. Prog. Ser. 160, 35-46. doi: 10.3354/meps160035
Jacobs, P., Pitarch, J., Kromkamp, J. C., and Philippart, C. J. (2021). Assessing biomass and primary production of microphytobenthos in depositional coastal systems using spectral information. PLoS ONE 16, e0246012. doi: 10.1371/journal.pone.0246012
Janousek, C. N. (2009). Taxonomic composition and diversity of microphytobenthos in southern California marine wetland habitats. Wetlands 29, 163–175. doi: 10.1672/08-06.1
Jesus, B., Brotas, V., Ribeiro, L., Mendes, C., Cartaxana, P., and Paterson, D. (2009). Adaptations of microphytobenthos assemblages to sediment type and tidal position. Cont. Shelf Res. 29, 1624–1634. doi: 10.1016/j.csr.2009.05.006
Johnson, P. C. (2014). Extension of Nakagawa and Schielzeth's R2GLMM to random slopes models. Methods Ecol. Evol. 5, 944–946. doi: 10.1111/2041-210X.12225
Kaas, H. (1987). Algal studies of the Danish Wadden Sea. V. Blue-green algae in higher salt march areas-their seasonal and spatial distribution. Nord. J. Bot. 7, 735–749. doi: 10.1111/j.1756-1051.1987.tb02041.x
Kelaher, B. P., Levinton, J. S., and Hoch, J. M. (2003). Foraging by the mud snail, Ilyanassa obsoleta (Say), modulates spatial variation in benthic community structure. J. Exp. Mar. Biol. Ecol. 292, 139–157. doi: 10.1016/S0022-0981(03)00183-7
Kirwan, M. L., and Guntenspergen, G. R. (2012). Feedbacks between inundation, root production, and shoot growth in a rapidly submerging brackish marsh. J.Ecol. 100, 764–770. doi: 10.1111/j.1365-2745.2012.01957.x
Krajewska, M., Szymczak-Zyła, M., Kobos, J., Witak, M., and Kowalewska, G. (2019). Canthaxanthin in recent sediments as an indicator of heterocystous cyanobacteria in coastal waters. Oceanologia 61, 78–88. doi: 10.1016/j.oceano.2018.07.002
Kühl, M., and Jorgensen, B. B. (1994). The light field of microbenthic communities: radiance distribution and microscale optics of sandy coastal sediments. Limnol. Oceanogr. 39, 1368–1398. doi: 10.4319/lo.1994.39.6.1368
Küpper, H., Spiller, M., and Küpper, F. C. (2000). Photometric method for the quantification of chlorophylls and their derivatives in complex mixtures: fitting with gauss-peak spectra. Anal. Biochem., 286, 247–256. doi: 10.1006/abio.2000.4794
Le Hir, P., Roberts, W., Cazaillet, O., Christie, M., Bassoullet, P., and Bacher, C. (2000). Characterization of intertidal flat hydrodynamics. Cont. Shelf Res. 20, 1433–1459. doi: 10.1016/S0278-4343(00)00031-5
Lima, C. R., Mendes, C. R. B., Tavano, V. M., Detoni, A. M. S., and Secchi, E. R. (2019). Chemotaxonomy-based mapping of phytoplankton communities in the subtropical Southwestern Atlantic Ocean, with emphasis on the marine cyanobacterium Trichodesmium. Prog. Oceanogr. 172, 77–88. doi: 10.1016/j.pocean.2019.01.008
Lucas, C. H., and Holligan, P. M. (1999). Nature and ecological implications of algal pigment diversity on the Molenplaat tidal flat (Westerschelde estuary, SW Netherlands). Mar. Ecol. Prog. Ser. 180, 51–64. doi: 10.3354/meps180051
Lucas, C. H., Widdows, J., Brinsley, M. D., Salkeld, P. N., and Herman, P. M. (2000). Benthic-pelagic exchange of microalgae at a tidal flat. 1. Pigment analysis. Mar. Ecol. Prog. Ser. 196, 59–73. doi: 10.3354/meps196059
MacIntyre, H. L., Geider, R. J., and Miller, D. C. (1996). Microphytobenthos: the ecological role of the “secret garden” of unvegetated, shallow-water marine habitats. I. Distribution, abundance and primary production. Estuaries 19, 186–201. doi: 10.2307/1352224
Mantoura, R. F. C., and Llewellyn, C. A. (1983). The rapid determination of algal chlorophyll and carotenoid pigments and their breakdown products in natural waters by reverse-phase high-performance liquid chromatography. Anal. Chim. Acta 151, 297–314. doi: 10.1016/S0003-2670(00)80092-6
Marker, A. (1980). The measurement of photosynthetic pigments in freshwaters and standardization of methods: conclusions and recommendations. Arch. Hydrobiol. Beih 14, 91–106.
Meier, D., Thölen, C., Hillebrand, H., Kleyer, M., Lõhmus, K., and Zielinski, O. (2020). Continuous Temperature Observations in Surface Sediment Within DynaCom Experimental Islands And Saltmarsh Enclosed Plots at Different Elevation Levels. Spiekeroog: PANGAEA.
Méléder, V., Barillé, L., Rincé, Y., Morançais, M., Rosa, P., and Gaudin, P. (2005). Spatio-temporal changes in microphytobenthos structure analysed by pigment composition in a macrotidal flat (Bourgneuf Bay, France). Mar. Ecol. Prog. Ser. 297, 83–99. doi: 10.3354/meps297083
Middelburg, J. J., Barranguet, C., Boschker, H. T., Herman, P. M., Moens, T., and Heip, C. H. (2000). The fate of intertidal microphytobenthos carbon: An in situ 13C-labeling study. Limnol. Oceanogr. 45, 1224–1234. doi: 10.4319/lo.2000.45.6.1224
Mitbavkar, S., and Anil, A. (2002). Diatoms of the microphytobenthic community: population structure in a tropical intertidal sand flat. Mar. Biol. 140, 41–57. doi: 10.1007/s002270100686
Montani, S., Magni, P., and Abe, N. (2003). Seasonal and interannual patterns of intertidal microphytobenthos in combination with laboratory and areal production estimates. Mar. Ecol. Prog. Ser. 249, 79–91. doi: 10.3354/meps249079
Morris, J. T., Sundareshwar, P., Nietch, C. T., Kjerfve, B., and Cahoon, D. R. (2002). Responses of coastal wetlands to rising sea level. Ecology 83, 2869–2877. doi: 10.1890/0012-9658(2002)083[2869:ROCWTR]2.0.CO;2
Naz, T., Yang, J., Nosheen, S., Sun, C., Nazir, Y., Mohamed, H., et al. (2021). Genetic modification of mucor circinelloides for canthaxanthin production by heterologous expression of β-carotene ketolase gene. Front. Nutr. 8, 756218. doi: 10.3389/fnut.2021.756218
Oksanen, J., Blanchet, F. G., Kindt, R., Legendre, P., Minchin, P. R., O'hara, R., et al. (2013). Package 'vegan'. Community Ecology Package, Version 2, 1–295.
Paerl, H. W., Valdes, L. M., Pinckney, J. L., Piehler, M. F., Dyble, J., and Moisander, P. H. (2003). Phytoplankton photopigments as indicators of estuarine and coastal eutrophication. Bioscience 53, 953–964. doi: 10.1641/0006-3568(2003)053[0953:PPAIOE]2.0.CO;2
Paterson, D. M. (1989). Short-term changes in the erodibility of intertidal cohesive sediments related to the migratory behavior of epipelic diatoms. Limnol. Oceanogr. 34, 223–234. doi: 10.4319/lo.1989.34.1.0223
Paterson, D. M., and Hagerthey, S. E. (2001). “Microphytobenthos in contrasting coastal ecosystems: biology and dynamics,” in Ecological Comparisons of Sedimentary Shores, Ecological Studies. Vol. 150 (Berlin; Heidelberg: Springer). doi: 10.1007/978-3-642-56557-1_6
Pomeroy, L. R. (1959). Algal productivity in salt marshes of Georgia 1. Limnol. Oceanogr. 4, 386–397. doi: 10.4319/lo.1959.4.4.0386
R Core Team (2021). R: A Language and Environment for Statistical Computing. Vienna: R Foundation for Statistical Computing. Available online at: https://www.R-project.org/
Ramaraj, R., Tsai, D. D., and Chen, P. H. (2013). Chlorophyll is not accurate measurement for algal biomass. Chiang Mai J. Sci. 40, 547–555. Available online at: http://it.science.cmu.ac.th/ejournal/ (accessed May 13, 2022).
Redzuan, N. S., and Underwood, G. J. (2020). Movement of microphytobenthos and sediment between mudflats and salt marsh during spring tides. Front. Mar. Sci. 7, 496. doi: 10.3389/fmars.2020.00496
Ribeiro, L., Benyoucef, I., Poulin, M., Jesus, B., Rosa, P., Méléder, V., et al. (2021). Spatio-temporal variation of microphytobenthos biomass, diversity and assemblage structure in the Loire Estuary, France. Aquat. Microb. Ecol. 87, 61–77. doi: 10.3354/ame01971
Rivera-Garcia, L. G., Hill-Spanik, K. M., Berthrong, S. T., and Plante, C. J. (2018). Tidal stage changes in structure and diversity of intertidal benthic diatom assemblages: a case study from two contrasting Charleston harbor flats. Estuar. Coasts 41, 772–783. doi: 10.1007/s12237-017-0312-4
Sawai, Y., Horton, B. P., Kemp, A. C., Hawkes, A. D., Nagumo, T., and Nelson, A. R. (2016). Relationships between diatoms and tidal environments in Oregon and Washington, USA. Diatom Res. 31, 17–38. doi: 10.1080/0269249X.2015.1126359
Schlüter, L., Lauridsen, T. L., Krogh, G., and Jørgensen, T. (2006). Identification and quantification of phytoplankton groups in lakes using new pigment ratios-a comparison between pigment analysis by HPLC and microscopy. Freshw. Biol. 51, 1474–1485. doi: 10.1111/j.1365-2427.2006.01582.x
Scholz, B., Küpper, F. C., Vyverman, W., and Karsten, U. (2014). Eukaryotic pathogens (Chytridiomycota and Oomycota) infecting marine microphytobenthic diatoms—a methodological comparison. J. Phycol., 50, 1009–1019. doi: 10.1111/jpy.12230
Scholz, B., and Liebezeit, G. (2012a). Microphytobenthic dynamics in a Wadden Sea intertidal flat-Part I: Seasonal and spatial variation of diatom communities in relation to macronutrient supply. Eur. J. Phycol. 47, 105–119. doi: 10.1080/09670262.2012.663793
Scholz, B., and Liebezeit, G. (2012b). Microphytobenthic dynamics in a Wadden Sea intertidal flat-Part II: seasonal and spatial variability of non-diatom community components in relation to abiotic parameters. Eur. J. Phycol. 47, 120–137. doi: 10.1080/09670262.2012.665251
Semcheski, M. R., Egerton, T. A., and Marshall, H. G. (2016). Composition and diversity of intertidal microphytobenthos and phytoplankton in Chesapeake Bay. Wetlands 36, 483–496. doi: 10.1007/s13157-016-0756-5
Sin, Y.-S., Ryu, S.-O., and Song, E.-S. (2009). Characteristics of benthic chlorophyll a and sediment properties in the tidal flats of Kwangyang Bay, Korea. Algae 24, 149–161. doi: 10.4490/ALGAE.2009.24.3.149
Sommer, U. (1999). The impact of herbivore type and grazing pressure on benthic microalgal diversity. Ecol. Lett. 2, 65–69. doi: 10.1046/j.1461-0248.1999.22052.x
Steinman, A. D., Lamberti, G. A., Leavitt, P. R., and Uzarski, D. G. (2017). “Biomass and pigments of benthic algae,” in Methods in Stream Ecology, Volume 1 (Amsterdam: Elsevier), 223–241. doi: 10.1016/B978-0-12-416558-8.00012-3
Thornton, D. C., Dong, L. F., Underwood, G. J., and Nedwell, D. B. (2002). Factors affecting microphytobenthic biomass, species composition and production in the Colne Estuary (UK). Aquat. Microb. Ecol. 27, 285–300. doi: 10.3354/ame027285
Thornton, D. C., and Visser, L. A. (2009). Measurement of acid polysaccharides (APS) associated with microphytobenthos in salt marsh sediments. Aquat. Microb. Ecol. 54, 185–198. doi: 10.3354/ame01265
Thrane, J.-E., Kyle, M., Striebel, M., Haande, S., Grung, M., Rohrlack, T., et al. (2015). Spectrophotometric analysis of pigments: a critical assessment of a high-throughput method for analysis of algal pigment mixtures by spectral deconvolution. PLoS ONE 10, e0137645. doi: 10.1371/journal.pone.0137645
Tracy, E. J., and South, G. R. (1989). Composition and seasonality of micro-algal mats on a salt marsh in New Brunswick, Canada. Br. Phycol. J. 24, 285–291. doi: 10.1080/00071618900650301
Traut, B. H. (2005). The role of coastal ecotones: a case study of the salt marsh/upland transition zone in California. J.Ecol., 279–290. doi: 10.1111/j.1365-2745.2005.00969.x
Underwood, G. J., and Barnett, M. (2006). “What determines species composition in microphytobenthic biofilms,” in Functioning of Microphytobenthos in Estuaries. Kromkamp J, editor. Microphytobenthos Symposium. Amsterdam, The Netherlands: Royal Netherlands Academy of Arts and Sciences.
Underwood, G. J. C. (2001). “Microphytobenthos,” in Encyclopedia of Ocean Sciences (Second Edition), ed J. H. Steele (New York, NY: Academic Press), 807–814. doi: 10.1016/B978-012374473-9.00213-7
Underwood, G. J. C., and Kromkamp, J. (1999). “Primary production by phytoplankton and microphytobenthos in estuaries,” in Advances in Ecological Research (Vol. 29, pp. 93-153), eds D. B. Nedwell and D. G. Raffaelli (New York, NY: Academic Press). doi: 10.1016/S0065-2504(08)60192-0
van der Wal, D., Lambert, G. I., Ysebaert, T., Plancke, Y. M., and Herman, P. M. (2017). Hydrodynamic conditioning of diversity and functional traits in subtidal estuarine macrozoobenthic communities. Estuar. Coast. Shelf Sci. 197, 80–92. doi: 10.1016/j.ecss.2017.08.012
van der Wal, D., Wielemaker-van den Dool, A., and Herman, P. M. (2010). Spatial synchrony in intertidal benthic algal biomass in temperate coastal and estuarine ecosystems. Ecosystems 13, 338–351. doi: 10.1007/s10021-010-9322-9
Vogt, J. C., Albach, D. C., and Palinska, K. A. (2018). Cyanobacteria of the Wadden Sea: seasonality and sediment influence on community composition. Hydrobiologia 811, 103–117. doi: 10.1007/s10750-017-3287-z
Watermann, F., Hillebrand, H., Gerdes, G., Krumbein, W. E., and Sommer, U. (1999). Competition between benthic cyanobacteria and diatoms as influenced by different grain sizes and temperatures. Mar. Ecol. Prog. Ser. 187, 77–87. doi: 10.3354/meps187077
Whitcraft, C. R., and Levin, L. A. (2007). Regulation of benthic algal and animal communities by salt marsh plants: impact of shading. Ecology 88, 904–917. doi: 10.1890/05-2074
Wright, S., Jeffrey, S., Mantoura, R., Llewellyn, C., Bjørnland, T., Repeta, D., et al. (1991). Improved HPLC method for the analysis of chlorophylls and carotenoids from marine phytoplankton. Mar. Ecol. Prog. Ser. 183–196. doi: 10.3354/meps077183
Wright, S. W., and Jeffrey, S. (2006). Pigment Markers for Phytoplankton Production. Marine Organic Matter: Biomarkers, Isotopes and DNA, 71–104. doi: 10.1007/698_2_003
Zedler, J. B. (1980). Algal mat productivity: comparisons in a salt marsh. Estuaries 3, 122–131. doi: 10.2307/1351556
Keywords: East Frisian Islands, benthic microalgae, tidal flat, salt marsh, marine-terrestrial boundary, experimental islands, pigment diversity
Citation: Yong J, Moick M, Dewenter J, Hillebrand H, Kröncke I, Lõhmus K, Pieck D, Rohde S and Moorthi S (2022) Spatial and temporal patterns of microphytobenthos communities along the marine-terrestrial boundary in the German Wadden Sea. Front. Ecol. Evol. 10:956092. doi: 10.3389/fevo.2022.956092
Received: 29 May 2022; Accepted: 15 August 2022;
Published: 14 September 2022.
Edited by:
Björn Kristian Klatt, Faculty of Science, Lund University, SwedenReviewed by:
Xiaohe Zhang, Boston University, United StatesLourenço Ribeiro, Center for Marine and Environmental Sciences (MARE), Portugal
Copyright © 2022 Yong, Moick, Dewenter, Hillebrand, Kröncke, Lõhmus, Pieck, Rohde and Moorthi. This is an open-access article distributed under the terms of the Creative Commons Attribution License (CC BY). The use, distribution or reproduction in other forums is permitted, provided the original author(s) and the copyright owner(s) are credited and that the original publication in this journal is cited, in accordance with accepted academic practice. No use, distribution or reproduction is permitted which does not comply with these terms.
*Correspondence: Joanne Yong, am9hbm5lLnlvbmcueWEubGluQHVuaS1vbGRlbmJ1cmcuZGU=