- 1Biology Department, Utah State University, Logan, UT, United States
- 2Pollinating Insects Research Unit, USDA-ARS, Logan, UT, United States
Introduction: The blue orchard bee, Osmia lignaria, is a widespread North American native pollinator that can be employed for commercial fruit and nut crop production. The largest supplies of these bees are collected in the wildlands ssssof Utah and Washington, United States. How O. lignaria from different geographic regions respond to current recommended management practices or translocation to novel environments is not fully understood.
Methods: Utah- and Washington-originated O. lignaria were reared in laboratory incubators under two thermal regimens: (1) constant temperatures used to manage bees through immature development, adult winter dormancy, and for spring emergence, and (2) hourly fluctuating temperatures programmed to mimic the natural daily (24 h) thermal cycles of the nearest cherry orchard growing region through their life cycles.
Results: In comparison to rearing bees at orchard temperatures, we found that rearing bees at a constant temperature increased survival and shortened egg–adult development periods. Washington bees were more adversely affected by the consistent warm rearing temperatures than were Utah bees, possibly due to their adaptations to Washington’s relatively moderate climate. At orchard temperatures, Utah bees suffered high prepupal and pupal mortality, while Washington bees suffered high pupal and adult mortality. These late life stages coincided with the hottest maximum temperatures in their respective thermal regimens. Adult females from both states naturally emerged in synchrony with local bloom time, but their emergence period overall was prolonged compared to bees in the constant thermal regimen that were induced to emerge at orchard bloom times.
Discussion: Our data support that bees originating from cool montane habitats of different U.S. states suffer from the warmer climatic conditions at lower altitudes of their respective crop-growing regions. A better understanding of optimal management temperatures for O. lignaria from different geographic regions and the effect of bee origin and temperature on survival and development timing is needed for best managing these pollinators when they are translocated or when climate change results in increased temperatures during bee development periods.
1. Introduction
Bees serve a critical ecosystem function as pollinators and are vital for the production of pollinator-dependent crops (Klein et al., 2007; Ollerton et al., 2011; Khalifa et al., 2021). Numerous studies have noted declines in health and numbers of both managed and wild bees (e.g., LeCroy et al., 2020; Osterman et al., 2021; Zattara and Aizen, 2021) due to stressors, including loss of habitat, pests and pathogens, pesticides, and climate change (Brown and Paxton, 2009). Climate change is predicted to alter the environment in both managed and natural ecosystems in ways that pose a substantial threat to solitary bees. Also, management practices used to rear, supply, and employ commercially available bees in agricultural lands can have negative consequences for managed bees (Pettis and Delaplane, 2010). Environmental and management stressors often occur in tandem or interact so that effects are additive, synergistic, or antagonistic (Meeus et al., 2018).
Bee management and commercialization vary by species. Honey bees, Apis mellifera L., and bumble bees, Bombus spp., (Hymenoptera: Apidae) are the most well-known commercially available bees for crop pollination. Commercial honey bee colonies are propagated in apiaries, and colonies are transported sometimes very long distances for migratory pollination services, honey production, and winter dormancy. Colonies of several Bombus species are reared in commercial facilities and shipped, or otherwise transported, for pollination services in field or enclosed crops, such as tomatoes, various berries, and other fruits (Velthuis and van Doorn, 2006). Typically, commercial Bombus spp. colonies are not managed for self-propagation, and, therefore, new colonies must be purchased each year. Solitary, cavity-nesting bees are also available as commercial pollinators and require unique management practices. The native blue orchard bee, Osmia lignaria Say, and the introduced alfalfa leafcutting bee, Megachile rotundata F., (Hymenoptera: Megachilidae) are pollinators for tree fruit, nut, and berry crops and for alfalfa seed and hybrid canola seed production, respectively, in North America (Torchio, 1985, 2003; Bosch et al., 2006; Pitts-Singer and Cane, 2011; Artz et al., 2013; Andrikopoulos and Cane, 2018; Horth and Campbell, 2018; Pitts-Singer et al., 2018). Osmia bicornis and O. cornuta are used as managed orchard pollinators in Europe (Sedivy and Dorn, 2014; Krunić and Stanisavljević, 2006). Osmia cornifrons is propagated in Japan (Maeta et al., 1990; Matsumoto and Maejima, 2010) and was introduced into the eastern U.S. for pollination and has been spreading across North America since the 1980s (Cane, 2003; LeCroy et al., 2020; MacIvor et al., 2022).
Ideally, managed solitary bees are propagated in provided nesting materials on-farm or in open landscapes where preferred forage is naturally available. Bee progeny contained in nesting materials can be moved to other locations for storage (under controlled temperatures as needed), processing, and preparation for future use or sale. However, solitary bee reproduction results in population increase only in certain regions or crops and is highly variable from year to year. To fulfill the demand for large numbers of pollinators over a short blooming period, cocooned bees are shipped to novel environments outside of their natal regions.
Osmia lignaria naturally occupy montane riparian forests in western North America where nesting materials (e.g., exit holes of boring beetles in trees and moist soil from winter snow melt) and preferred foraging resources (such as willow, maple, and rosaceous trees) are found (Levin, 1957; Rust, 1974; Bosch and Kemp, 2001; Tepedino et al., 2022). In the United States, much of the commercial supply of O. lignaria is trapped by placing nesting materials in wildland sites with native populations (primarily in the Rocky Mountain and Pacific Northwest regions). Sometimes, bee ranchers reintroduce a portion of their harvested bees and enhance bee forage at the native sites to prevent overharvesting and increase propagation. Harvested bees usually are removed from nests as cocooned adults. Surplus bees are sold and transported in large numbers to homeowners and orchardists.
The market for O. lignaria as pollinators has substantially increased since commercial management was initiated in the 1970s (Torchio, 2003). When O. lignaria are used to pollinate orchard crops, they are typically transported to regions of lower altitudes that have favorable growing conditions (weather, soils, accessibility, etc.) for the crops. Thus, translocated O. lignaria often experience warmer, possibly drier climatic conditions than those of their natal locations, even when used in the U.S. state of origination. Studies of the movement of solitary bee populations outside of ranges from which they are propagated are sparse and, thus, the impacts of moving bees to new climates and landscapes are unknown or poorly understood.
For our study, we were interested in the separate and interactive effects of geographic origin of O. lignaria (trapped from wildlands) and the temperatures they experience during a life cycle in local growing regions when they are used as managed pollinators. Unlike for social bees, solitary bee development, reproduction, and overwinter survival are directly influenced by ambient temperature because there is no ability to regulate temperatures via the protection of a colony. For example, populations of O. lignaria from different geographic origins have been shown to exhibit regional differences in survival and developmental biology when exposed to common, but non-natal temperatures (Sgolastra et al., 2012; Pitts-Singer et al., 2014). Phenological differences, such as emergence timing, can be assumed to have a genetic basis if responding to climate in a different manner under the same climactic conditions. These populations can undergo local selection when responding to changes in natal climatic conditions (Bosch et al., 2008). When bees are translocated to a warmer climate or used for orchard pollination earlier than their natural phenology, high mortality occurs if bees are not managed under artificial conditions (i.e., removed from the field and stored in a climate-controlled shed or refrigeration unit). If left at ambient temperatures, bees become adults so early in the summer that they deplete their fat reserves before winter (Sgolastra et al., 2011; Pitts-Singer et al., 2014). Understanding O. lignaria ecophysiology throughout its range, especially considering climate change predictions, will improve best management practices for using bees from various geographic regions according to the location and timing of crop bloom.
We chose to explore the interaction of bee origin and temperature on O. lignaria survival, development, and timing of emergence from Utah and Washington because these are the main locations from which bees are currently collected from wildlands to sell in North America. We reared bees from Utah and Washington in laboratory incubators under two thermal regimens: (1) “constant” – one temperature through immature development, one for winter dormancy, and one for adult emergence, and (2) “natural” – hourly fluctuating temperatures programmed to mimic the natural daily (24 h) thermal cycles of the nearest cherry orchard growing region for the entire bee life cycle (Supplementary Figure S1). The former treatment was a shared “common garden” in which bees from both populations experienced the same, managed temperature conditions during their spring–summer immature development, during their time as adults in cocoons in the fall and winter, and during induced adult emergence (for two temporally separate pollination events) in spring. This treatment allowed for a direct comparison of observed variables by origin only. The latter treatment was specific to bee origin and served to compare observed variables at fluctuating (natural orchard) temperatures to the same outcomes at constant temperatures for each bee population. Understanding at what life stage(s) and at what temperatures O. lignaria from geographically distinct locations differ in their survival, development, and emergence will aid in the development of best management practices for relocating and sustaining bees in novel, orchard environments.
2. Materials and methods
2.1. Bee collection
In spring 2019, suitable nesting material for O. lignaria was deployed in open landscapes to collect immature bees from two climatically distinct locations: near Logan in northern Utah (41.798, −111.650; 1,675 m) and near Leavenworth in central Washington (47.482, −120.656; 500 m) (Supplementary Figure S2; PRISM Climate Group, 2022). Bundled cardboard tubes lined with paper straws (diameter = 7.5–8 mm, length = 16.2 cm, end of tube closed with plastic plug) were sheltered in a corrugated plastic box (length by width by height = 22 × 17 × 26 cm); boxes were hung from tree branches facing south to southeast at least 1.5 m from the ground. Wild bees could construct nests in straws, and individual nests could be collected and kept intact for transport and experimentation. To increase nesting in the provided materials, a formulation (decanoic acid dissolved in ethyl acetate) of a patented chemical attractant was prepared and applied to the open end of the nesting tubes (Pitts-Singer et al., 2016).
Nests were checked periodically for bee activity and completion. Recently provisioned (≤2-week old) O. lignaria nests were collected and shipped (WA bees) or transported (UT bees) immediately to the USDA ARS Pollinating Insects Research Unit in Logan, UT. The paper straw nests were sliced longitudinally and visually inspected to select only cells with an egg, a first stadium larva inside the egg chorion, or a recently hatched second stadium larva (Figure 1 and Table 1). Bees older than the second stadium were left in their cells but not further observed or used in the study. All cells were kept within the paper straw nests and held on corrugated cardboard trays (to prevent nest movement) (Supplementary Figure S3) by treatment throughout immature development. Leaving cells in the paper straws was intended to reduce artificially-induced mortality due to moving the provision masses with eggs into rearing chambers, such as well plates, that may cause provisions to dry out or mold or may not provide suitable space for cocoon-spinning (Kopit et al., 2022).
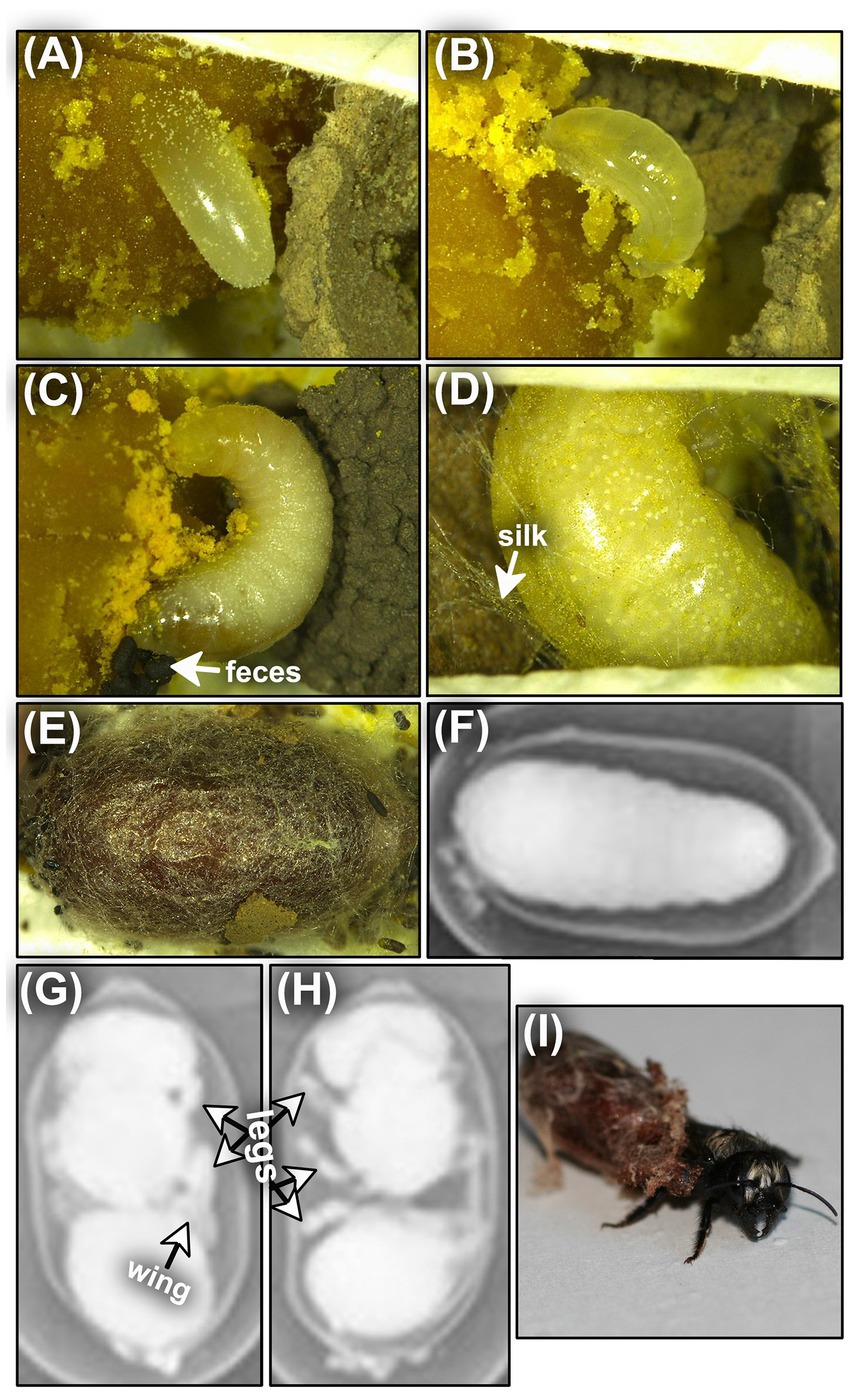
Figure 1. Optical and radiographic images of Osmia lignaria egg (A), second stadium larva (B), fifth stadium larva (C,D), cocooned prepupa (E,F), pupa (G), cocooned adult (H), and emerging adult (I). See Table 1 for details.
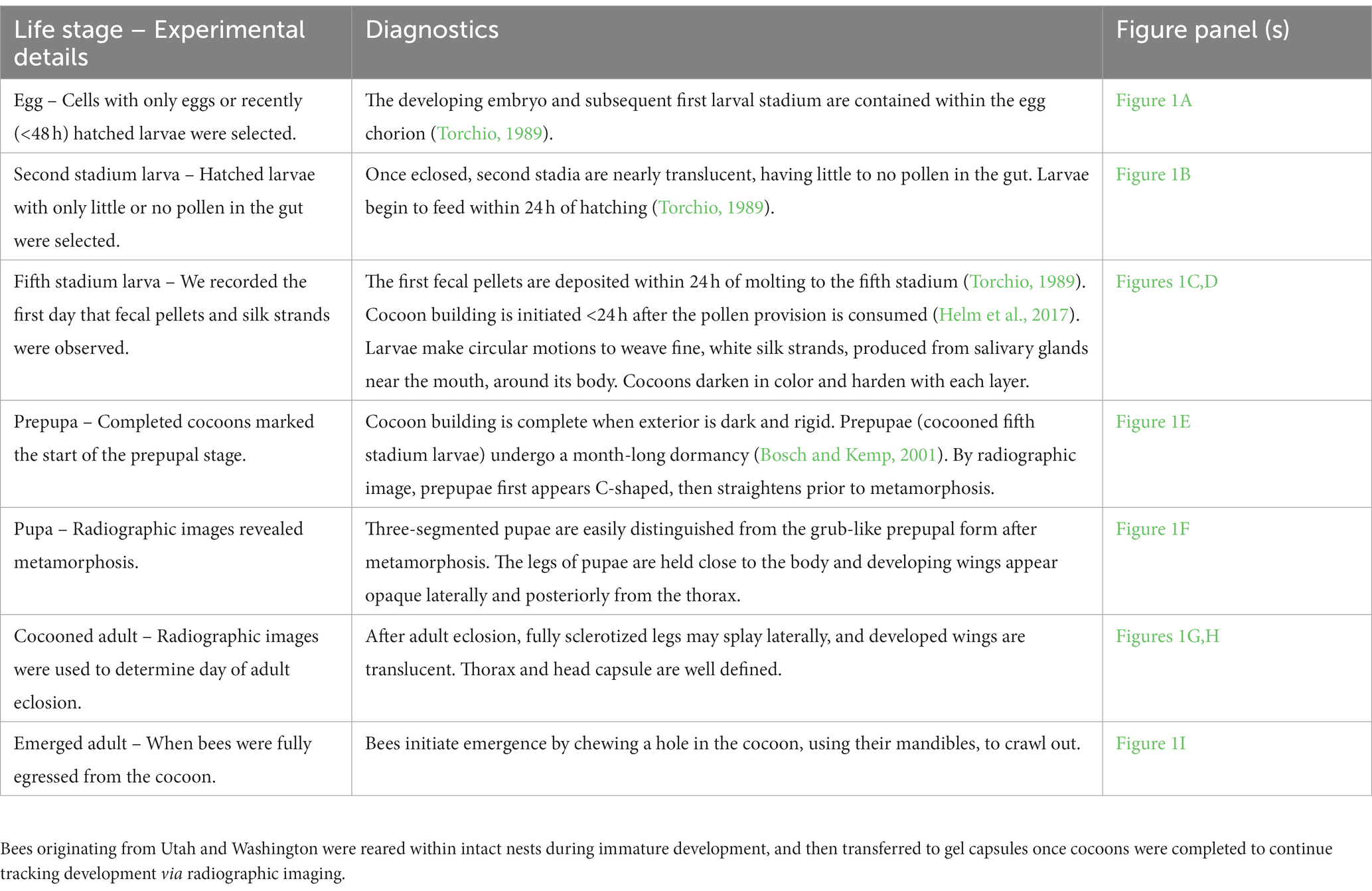
Table 1. Life stages used to track Osmia lignaria phenology, with diagnostic characteristics used for delineation.
2.2. Bee sample size and treatments
Nests contained 1–11 cells. Nests from each location were alternately assigned to one of two treatments, constant or natural thermal regimen, until at least 300 cells were available for each bee origin and temperature treatment. Therefore, all treatments contained cells that held female and male bees. In total, 1,432 O. lignaria nest cells were used in the experiment, split between four treatment groups: (1) UT bees exposed to a constant thermal regimen (UT-constant, n = 373), (2) WA bees exposed to the same constant thermal regimen as UT bees (WA-constant, n = 369), (3) UT bees exposed to a natural thermal regimen that mimics a cherry production zone near their collection site (UT-natural, n = 325), and (4) WA bees exposed to a natural thermal regimen that mimics a cherry production zone near their collection site (WA-natural, n = 365) (Supplementary Figures S1, S4). Each treatment consisted of approximately 60 nests (UT-constant = 63, WA-constant = 60, UT-natural = 62, WA-natural = 59), and nests each contained a mean of six cells (mean ± SE: UT-constant = 5.9 ± 2.5, WA-constant = 6.1 ± 1.8, UT-natural = 6.2 ± 2.2, WA-natural = 6.2 ± 2.4). Additionally, cells from all positions within nests were used (nests had up to 11 cells) with an equal number of cell positions being represented within each treatment and population.
The nests in the constant thermal regimen were held in a common environmental test chamber (Forma Scientific Dual Temperature Cabinet, Marietta, OH) set to 26°C (Bosch and Kemp, 2000, 2001; Orchard Bee Association, 2021) throughout their immature development period (Supplementary Figures S1, S4 and Supplementary Table S1). Thirty days after adult eclosion (mean date by bee origin), bees were cooled over a 2-week period by subjecting them to 19°C for 1 week and then 11°C for the next week, requiring a second incubator to accommodate differences in developmental timing (similar to Orchard Bee Association, 2021). At the end of 2 weeks, bees were placed at a winter storage temperature of 4–5°C where they remained until the following spring (Bosch and Kemp, 2000, 2001). These management steps were made to avoid an excessively long (>45 days) pre-wintering period, which can cause high winter and pre-emergence mortality (Bosch and Kemp, 2004; Bosch et al., 2008). As possible, UT and WA bees for the constant treatment remained in the same incubator.
The natural thermal regimen reflected the use of “local” bees as pollinators in their regional orchard environments. Nest cells collected from a UT mountain site were placed in an incubator programmed for temperatures in a UT cherry orchard as the UT-natural regimen. Another incubator held nest cells from a WA mountain site and was programmed for temperatures in a WA cherry orchard as the WA-natural treatment (Percival Intellus Control System, Percival Scientific, Inc., Perry, IA, United States) (Supplementary Figure S4). For each bee origin, the natural thermal regimen mimicked daily (24 h) temperature cycles of the nearest orchard growing region for which data were available, starting at the time of cherry bloom. The programmed diel temperature cycles were updated weekly to match average hourly temperatures from a previous 6-year period (2012–2017) from weather stations in Provo, Utah (40.216, −111.716; 1,370 m; approx. 170 km from the bee collection location) and Wenatchee, Washington (47.397, −120.201; 378 m; approx. 35 km from the bee collection location) (Supplementary Figure S1; MesoWest, 2021). Bees remained at these natural temperature cycles throughout immature development, winter dormancy, and adult emergence the following spring (or until death) (Supplementary Figure S1).
The timing of tart cherry bloom in central Utah and sweet cherry bloom in the Columbia Basin of Washington is mid-April (United States Department of Agriculture National Agricultural Statistics Service, 2006). For the UT-natural and WA-natural treatments, the programmed diel temperature cycle for Week 1 of our experiment corresponded to mean hourly temperatures during 16 April (Day 1) to 22 April (Day 7) in each location (Supplementary Table S1). Week 2 of the experiment corresponded to the following seven dates, and so on. Since O. lignaria were collected from higher altitude wildlands than orchard landscapes and are naturally active in collection locations after cherry bloom, nests used in this study were made and collected after 16 April. UT bees (eggs or second stadium larvae) were obtained and assigned to experimental treatments between actual calendar dates of 14–21 May, and WA bees between actual dates of 07–14 May. For ease and clarity, we have reported dates in terms of weeks and days since the start of the experiment or dates which correspond to the timing of cherry bloom (i.e., the artificial timeline).
2.3. Mortality and development
Each bee cell was visually inspected (with the aid of a compound microscope, when necessary) three times per week (on Monday, Wednesday, and Friday) to document mortality and timing of immature life stages until larvae completed cocoon spinning (similar to Pitts-Singer et al., 2014). Because the date of oviposition was unknown, the first life stage date recorded for all study specimens was the second stadium larva, after eclosion from the egg chorion; this served as a reliable starting point for comparing treatment effects on development periods for each immature life stage (Figure 1 and Table 1). Once cocooned, bees were considered prepupae and transferred to gelatin capsules. Digital radiographic images (12 s exposure at 24 kVp; computed radiography high-resolution system by Faxitron X-Ray LLC, Linconshire, IL) were taken three times per week (Monday, Wednesday, Friday) to determine the development periods for the prepupal, pupal, and adult stages and to record mortality at any cocooned life stage. Mortality was indicated by the failure to develop to the subsequent life stage (e.g., a bee died in the egg stage if a larva did not eclose from the egg chorion, or died in the prepupal stage if metamorphosis was incomplete).
2.4. Spring emergence
2.4.1. Incubation for adult emergence for constant regimen treatment
The UT- and WA-constant treatments were each further subdivided for two post-winter incubation events (Supplementary Figure S4). One subset was for incubating cocooned UT and WA bees in mid-March to simulate management for pollinating California cherry orchards; this treatment reflects a common real-world scenario in which bees are translocated because pollination demand in California is high and the natural abundance of O. lignaria is low or absent. The other subset was for incubating bees on two dates in mid-April to simulate pollinating cherry orchards in their natal regions so that UT bees were timed for cherry bloom in UT, and WA bees were timed for cherry bloom in WA.
2.4.2. Adult emergence for natural regimen treatment
Prior to incubation, the test chamber containing UT-constant and WA-constant bees was raised from 4 to 7°C for 5 days to prime bees for subsequent rapid emergence, which is a practice used by some bee managers. However, because some males emerged at 7°C (see below), the temperature was cooled back down to 5°C; males that emerged prematurely were excluded from further statistical analyses. Cocooned bees were moved to a 24°C incubator to induce emergence as if used for pollination in cherry orchards at the assigned times (Supplementary Figures S1, S4 and Supplementary Table S1), and checked daily for emergence, i.e., when the adult bee had chewed out of its cocoon but remained in the gelatin capsule.
Bees in the natural thermal regimens remained at mean daily temperature cycles of their region of origin to reveal emergence timing in the absence of temperature management. Bees were checked daily once natural temperatures reached 10°C to document the timing of adult emergence.
2.5. Statistical analysis
Prior to formal analysis, all Generalized Linear Mixed Models (GLMM) underwent an Akaike Information Criterion (AIC) model selection process, where treatment, bee origin, nest number, cell position (categorical) and sex (when appropriate) were analyzed. Nest number was always treated as a random effect, while all other factors were treated as fixed effects. The AIC model selection showed best fit for models using bee origin and treatment type as predictor variables and with nest as a random factor. All analyses were performed using R.3.1.2 (R core team) and R packages lme4 (Bates et al., 2015) and arm (Gelman and Su, 2022). Post hoc tests were performed using the lsmeans – Tukey analysis (Lenth, 2016).
2.5.1. For mortality and development
To compare mortality during immature development with respect to bee origin as a binary output (i.e., dead or alive), we performed a generalized linear mixed effects model (GLMM). We examined mortality as a response variable and bee origin and treatment as our predictor variables; we treated nest as a random effect. We performed this model with a binomial distribution.
To investigate immature (larva, prepupa, pupa) and mature (cocooned adult) mortality with respect to bee origin, we performed two GLMMs using a Poisson distribution, one for each treatment type (managed and unmanaged). Prior to analyses, data was checked for over-dispersal using a Goodness-of-fit model. In these models, the sum of dead individuals at each life stage per nest were used as our response variable. Life stage and bee origin was the predictor variables, and nest was a random effect.
To look for effects of thermal regimen and bee origin on duration of immature development, we performed two GLMMs separated by sex (i.e., a male model and a female model) comparing each life stage development. Female and male bees were analyzed separately because life stage duration has previously been shown to vary by sex (Bosch et al., 2000; Sgolastra et al., 2012; Pitts-Singer et al., 2014). To determine sex, we visually inspected bees after emergence. Cocoons containing dead pupae and un-emerged adults were also dissected to determine the sex, when possible. Sex could not be determined for prepupae and some pale (unsclerotized) pupae, nor for bees that died in the egg or larval stages.
2.5.2. For spring emergence
To reveal any effects of bee origin on (temperature-induced) timing of spring emergence, we performed two GLMMs (one for each treatment). For the managed treatment, we examined the number of days it took O. lignaria adults to emerge in spring of year 2 as a response variable and bee origin as our predictor variable; we treated nest number as a random effect. For the unmanaged treatment, we analyzed the number of days between adult eclosion and adult emergence in spring of year 2 as a response variable and bee origin as our predictor variable, with nest as a random effect.
3. Results
3.1. Mortality and development
Mortality was higher in the unmanaged treatments than in the managed treatments (Z = 6.33, df = 3, p < 0.001). When reared at constant 26°C, significantly fewer UT bees died (16%) prior to adult eclosion than did WA bees (25%; Z = 2.91, df = 3, p = 0.022). Mortality was statistically similar between UT and WA bees at the egg (Z = −1.141, p = 1.000), prepupal (Z = 2.064, p = 0.467), and pupal (Z = 1.147, p = 1.0000) life stages, but was significantly higher for WA bees at the larval stage (Z = −3.746, p = 0.002) (Figure 2).
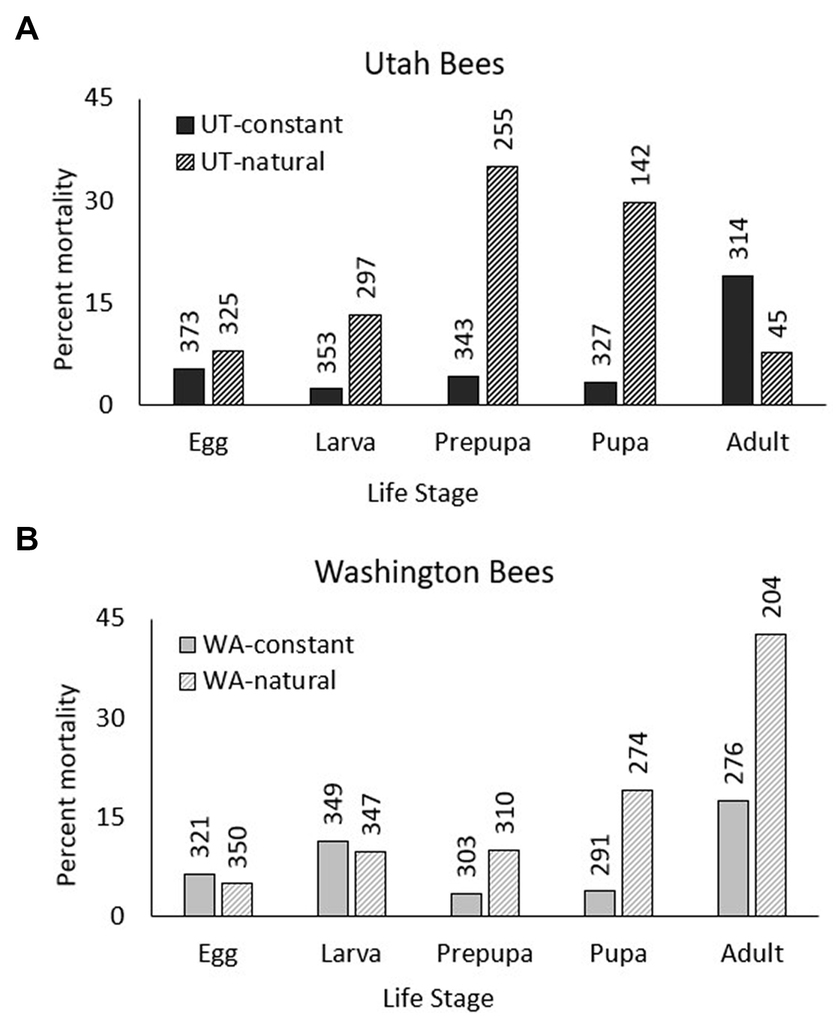
Figure 2. For Utah- (A) and Washington-sourced (B) Osmia lignaria by thermal regimen, the percent of reared bees that died at each immature (egg, larval, prepupa, pupa) and mature (cocooned adult) life stage. The number of bees in each sample is noted at the top of each bar.
The percent mortality during immature development was significantly higher for UT (86%) and WA (43%) bees reared at the natural thermal regimen compared to the constant 26°C treatment (UT bees: Z = 12.24, df = 1, p < 0.001; WA bees: Z = 10.23, df = 1, p < 0.001), and tended to increase from early to late immature life stages (Figures 2, 3). Compared to the constant treatment, mortality was eight times higher for UT-natural prepupae and pupae and was five times higher for WA-natural pupae. Due to high mortality of UT-natural bees prior to reaching the adult stage, their sex was unknown for later comparisons of life stage duration (Supplementary Figure S5).
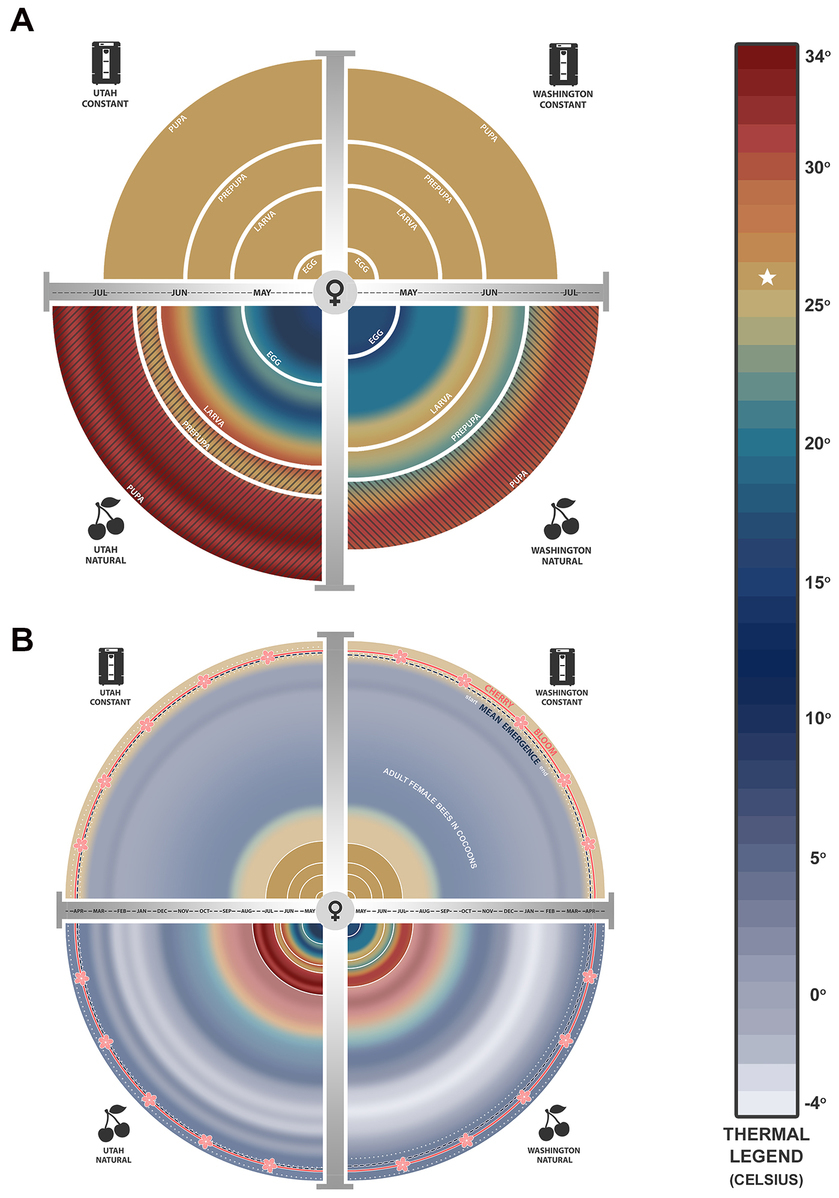
Figure 3. For a study of development and adult emergence of Osmia lignaria, Utah (left) and Washington (right) bees were reared using: (1) a constant thermal regimen in incubators (Top), and (2) a regimen of mean annual temperatures from a cherry orchard near their natal origins (UT-natural and WA-natural) (Bottom). (A) Mean life stage durations (width of band) at constant and maximum natural temperatures (color-coded) across months since the timing of Utah and Washington orchard bloom (mid-April) for female bees from egg (date of collection) through adult eclosion. Hatched lines indicate life stages where mortality was significantly high. (B) Thermal conditions for adult female bees in cocoons after eclosion (inner disc) until females chewed out of cocoons (emergence = dashed lines; duration of emergence = between dotted lines). Mean emergence day is weighted for when most bees emerged. For the constant regimen (Top), the temperature was lowered 30 days after adult eclosion to provide a moderate pre-wintering period and lowered further for a long overwintering period. Constant temperature was increased to induce adult emergence in time for mid-April orchard bloom. No modifications were made to natural orchard temperatures. Average bloom date is indicated by the outer “blossom” circle. (Graphic created by Erica J. Brus).
Mortality during the cocooned adult life stage was similar for both populations (Z = 0.247, df = 1, p = 0.618; Figures 2, 3) in the constant thermal regimen (i.e., when bees were managed during the pre-wintering, wintering, and emergence periods). For UT bees, adult mortality was significantly higher in the managed constant thermal regimen than the natural thermal regimen (Z = 19.81, df = 1, p < 0.001); however, as noted previously, immature mortality for UT-natural bees was high, and few bees survived to the cocooned adult life stage for statistical comparisons. For WA bees, adult mortality was significantly lower in the constant compared to the natural thermal regimen (Z = 54.91, df = 1, p < 0.001).When bees from both regions were reared at constant 26°C, immature development (from the second stadium to adult eclosion) was shorter for WA bees than for UT bees; this difference was significant for females (p < 0.001, Δ = 4.42 days) but not for males (p = 0.09, Δ = 1.82 days) (Figures 3, 4 and Supplementary Tables S2, S3). For both bee populations and sexes, immature development was completed in 68–74 days. The duration of larval and prepupal stages were not statistically different by bee origin; however, the pupal stage was significantly shorter for WA bees compared to UT bees.
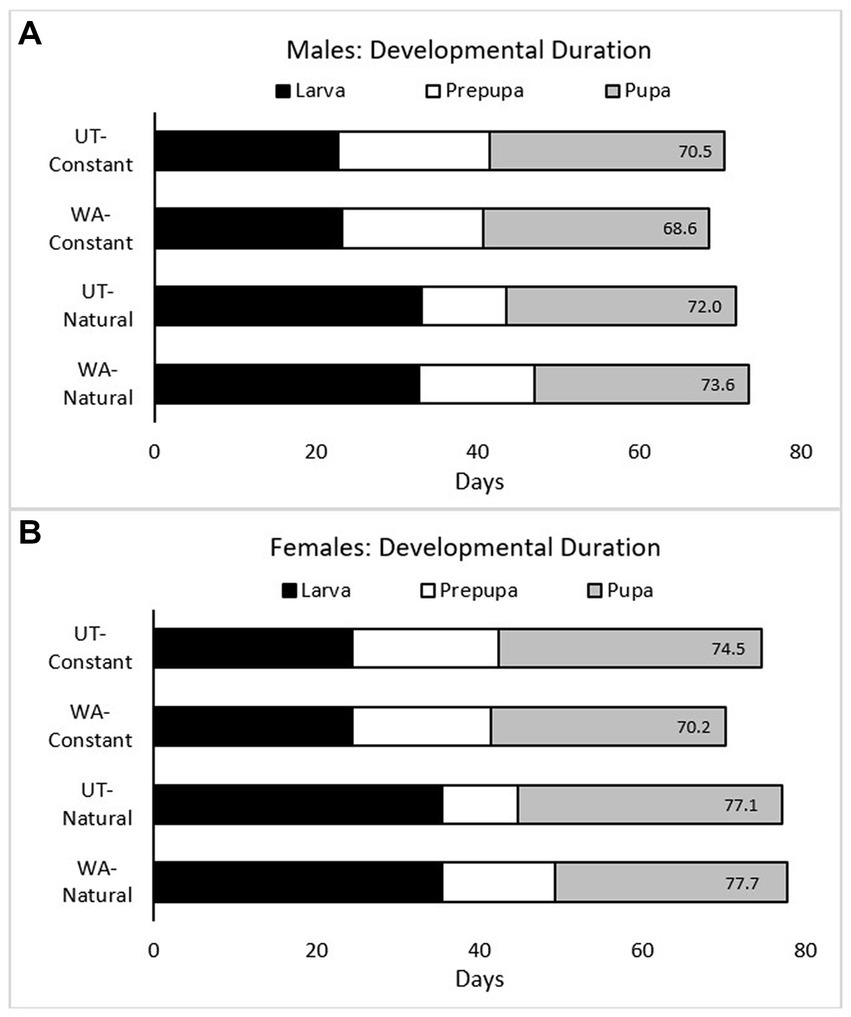
Figure 4. For male (A) and female (B) Osmia lignaria by treatment (bee origin–thermal regimen), the mean number of days spent in each immature life stage (larva, prepupa, and pupa). The mean number of days from the larval second stadium to adult eclosion is noted at the inner end of each bar.
With respect to thermal regimen, development was shortened for both UT and WA bees when reared at the warmer constant temperature compared to their respective natural thermal regimens (Figures 3, 4 and Supplementary Tables S2, S3). This difference was significant for WA females (Δ = 7.56 days) and males (Δ = 4.91 days) but not for UT females (Δ = 2.52 days) nor males (Δ = 1.54 days). For both bee populations and sexes in the natural thermal regimens, immature development was completed in 72–78 days. The larval period prior to defecation at the fifth stadium was significantly reduced in bees reared at constant 26°C by about 13 days for UT females and 11 days for WA females; similar results were found for males. By contrast, we found that the prepupal stage was significantly prolonged for bees reared at the constant compared to the natural thermal regimens, for both UT females (Δ = 7.71 days) and WA females (Δ = 2.70 days); similar results were found for males. Overall, thermal regimen had little effect on the duration of the pupal stage for both bee origins. Similar trends in the durations of immature stages were observed from analyses of all data including individuals whose sex was undetermined (Supplementary Figure S3).
Mean rearing temperatures during immature development were higher in the constant thermal regimen (26°C) compared to the natural thermal regimen for both bee sources (Figures 3, 5). Since temperatures in the natural thermal regimens slowly increased through spring and summer, the effect of temperature was greater during the larval period than for the prepupal and prepupal period. For the UT-natural regimen, mean temperatures were 12–22°C during larval development, 19–22°C during prepupal development, and 19–26°C during pupal development. For WA-natural bees, mean rearing temperatures were cooler than UT-natural temperatures during larval development (12–18°C) and similar during prepupal and pupal development. However, maximum temperatures exceeded 26°C by the end of the larval period in the UT-natural treatment and during the prepupal period for the WA-natural treatment.
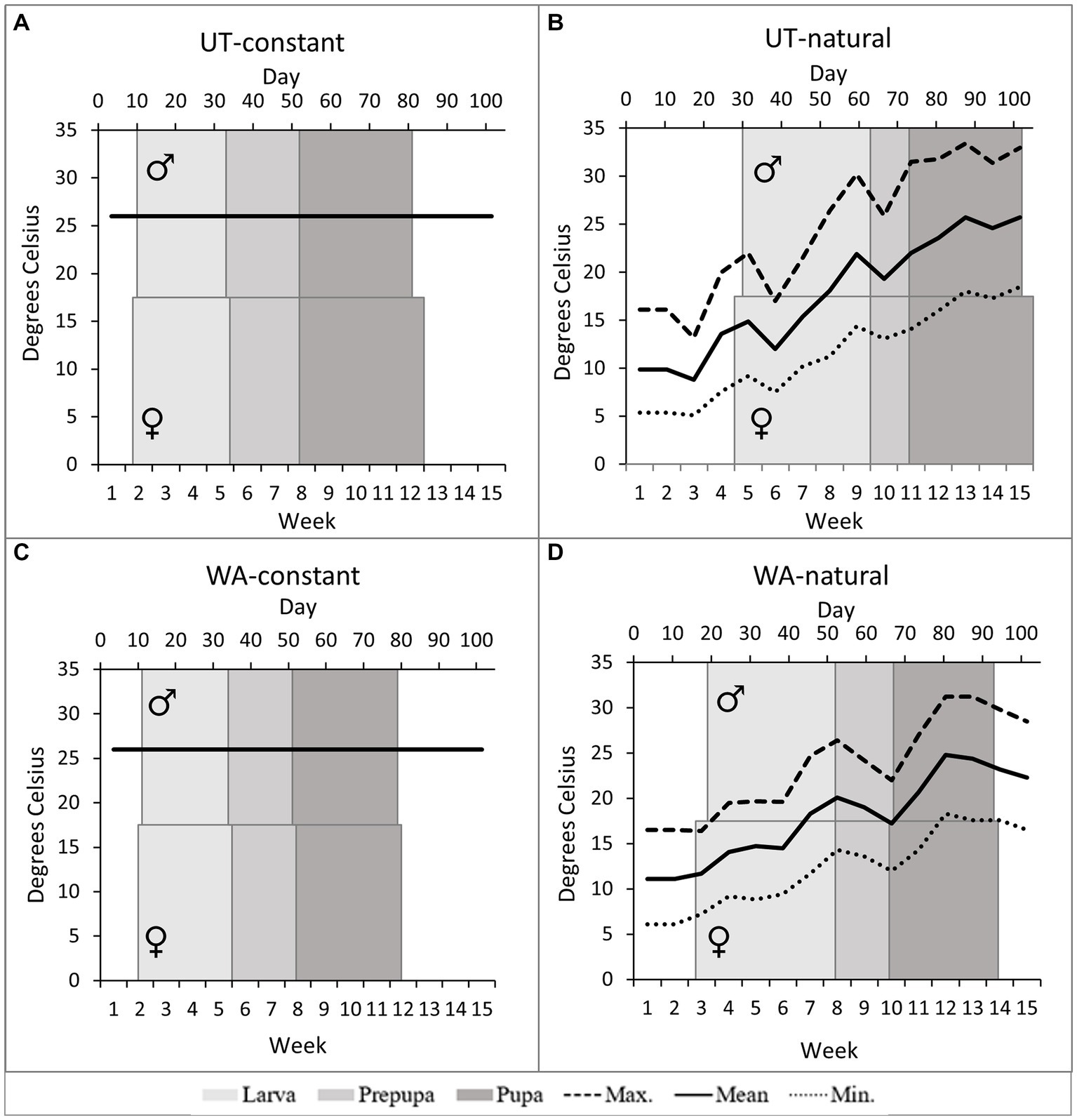
Figure 5. The programmed temperatures and mean duration of male and female Osmia lignaria immature developmental stages by treatment (A) UT-constant, (B) UT-natural (C) WA-constant, & (D) WA-natural.
3.2. Spring emergence
3.2.1. Incubation for adult emergence for constant regimen treatment
For both bee populations and sexes in the constant thermal regimen, the mean day of adult eclosion since the time nests were placed in incubators was during Week 12 (Days 79–84); the number of development days observed here was longer than the number of days in Figure 4 because it includes the time needed for eggs (of unknown age when collected) to hatch to the second stadium. Development from egg to second stadium was greatly affected by temperature (Figures 3, 5). For the UT-natural and WA-natural treatments, the mean day of adult eclosion was during Week 16 (Day 106) and Week 14 (Day 93), respectively. Unlike the constant thermal regimen in which bees were gradually cooled to wintering temperatures (4°C) 30 days after adult eclosion (Week 16), bees in the natural thermal regimen experienced warm pre-wintering and wintering temperatures for a longer period. Mean temperatures reached 4°C during Week 33 (Day 225) for the UT-natural treatment and during Week 31 (Day 211) for the WA-natural treatment.
For the cocooned bees in the constant thermal regimen placed at 7°C (from 4°C) prior to incubation, 3 female and 90 male UT bees and 1 female and 31 male WA bees emerged prematurely and were excluded from data analyses on adult emergence timing (Supplementary Table S1). When one subsample of bees was managed (incubated at 24°C) for March orchard bloom (UT bees on Day 339 and WA bees on Day 333), over 90% of UT and WA bees to emerge did so within the first and second day after incubation (Figure 6 and Table 2). Therefore, the duration of the cocooned adult stage was 257 days for UT males and 255 days for UT females; for WA bees, males were cocooned adults for 252 days and females for 253 days.
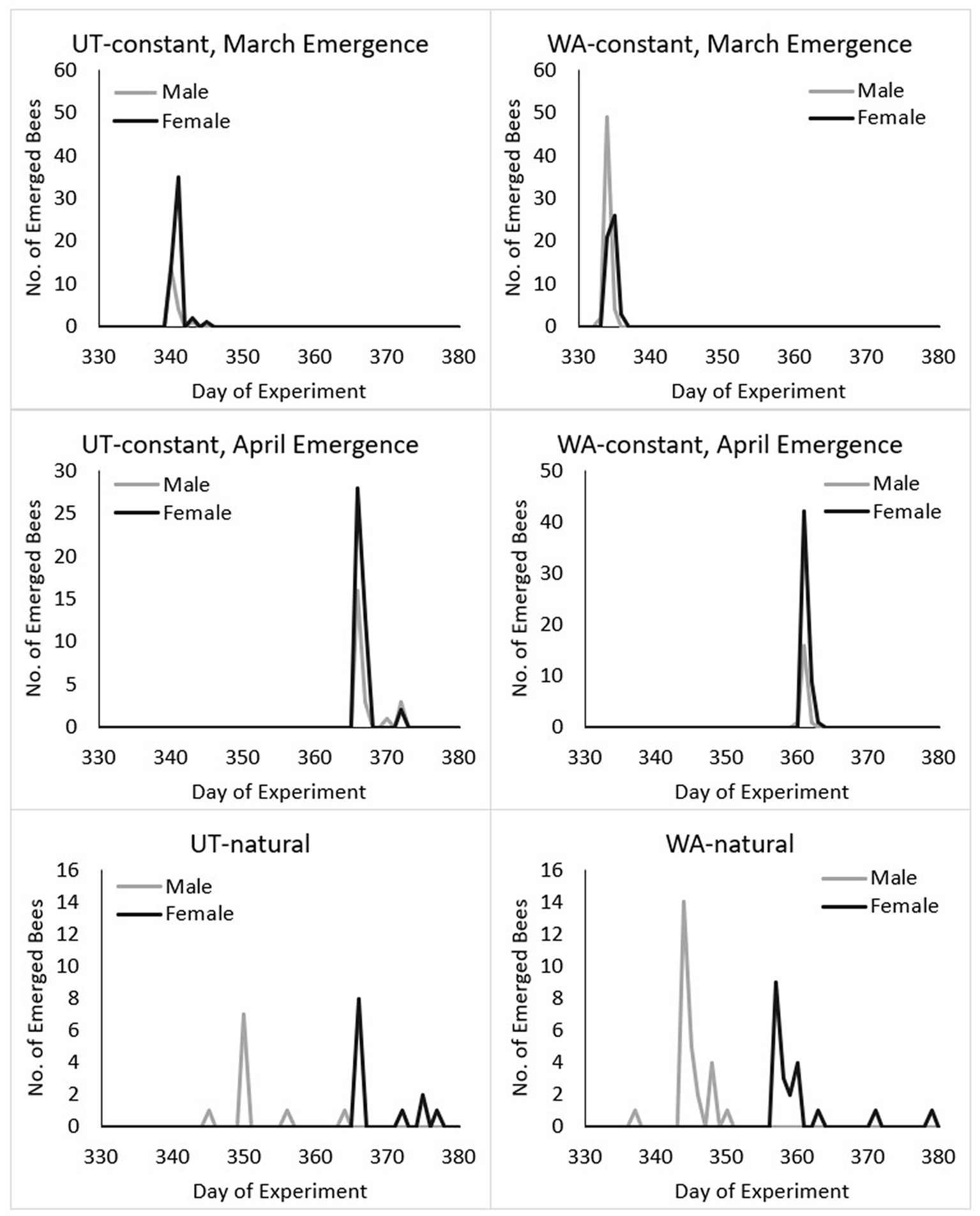
Figure 6. By treatment, emergence of Osmia lignaria females and males in spring of year 2. For the constant thermal regimen, bees were incubated (at 24°C) to induce emergence in mid-March (UT bees on Day 339 and WA bees on Day 333) approximately 1 month earlier than the pollination season in which they were born the previous year, and in mid-April (UT bees on Day 365 and WA bees on Day 360) on the same pollination timeline of their natal orchard. Bees in the natural thermal regimens were not induced to emerge via artificial warm temperatures but remained at simulated outdoor orchard temperatures; if kept for pollination in their natal orchard locations, target emergence period would be around Day 365 given similar timing of crop bloom. Prior to incubation, 3 female and 90 male UT bees emerged prematurely (at 7°C), and 1 female and 31 male WA bees emerged prematurely and are not included here.
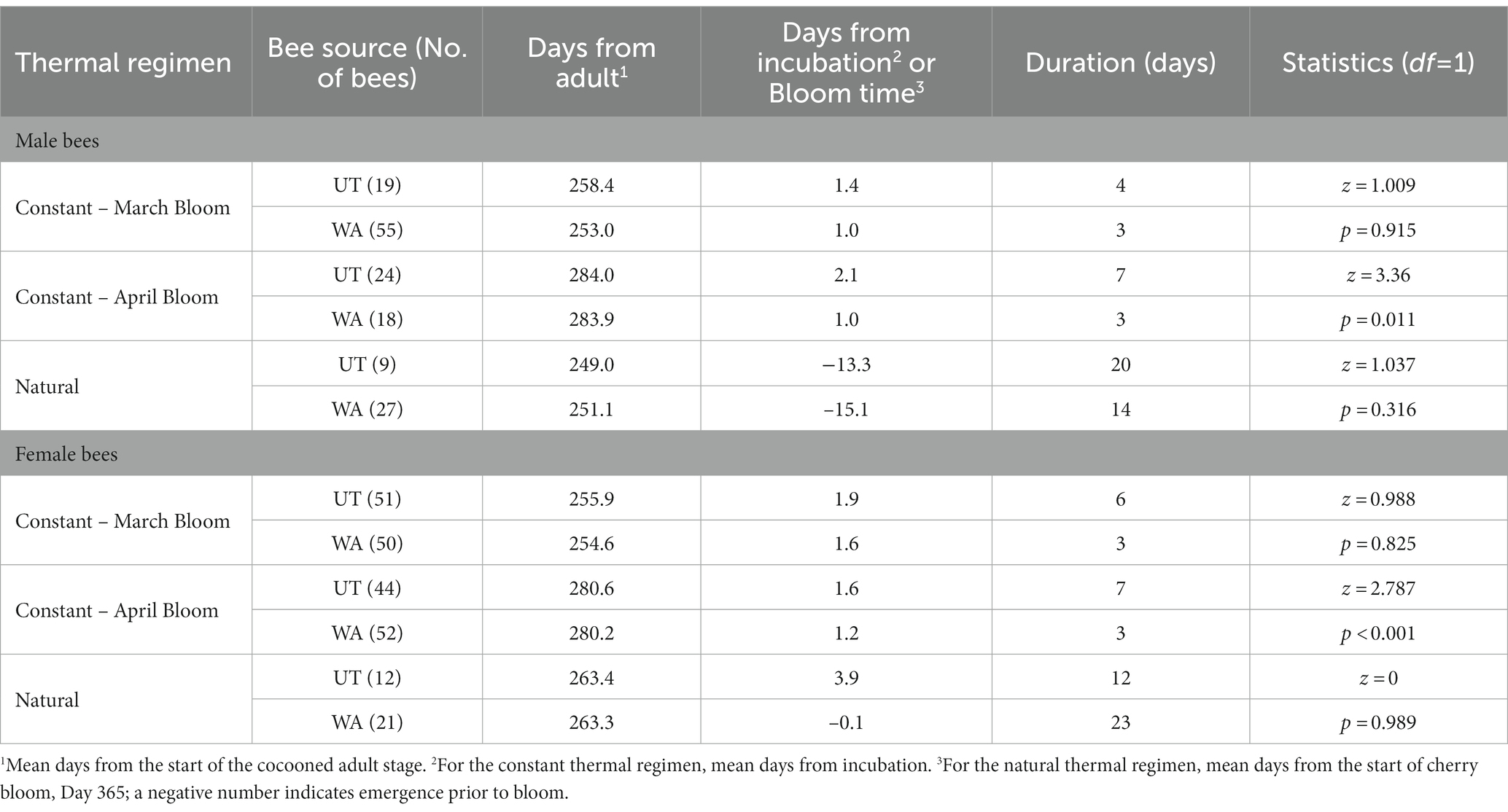
Table 2. Summary data and statistics for ANOVA comparisons of the number of days for Osmia lignaria to emerge from the cocooned adult life stage.
When the other subsample of bees was incubated in April (UT bees on Day 365 and WA bees on Day 360) to mimic the same timing of orchard bloom in their respective regions, 88 and 98% of UT and WA bees, respectively, emerged within 1–2 days. Therefore, the duration of the cocooned adult life stage was 284 days for UT males and 281 days for UT females; for WA bees, males were cocooned adults for 272 days and females for 271 days.
3.2.2. Adult emergence for natural regimen treatment
When UT and WA bees were reared at natural orchard temperatures of their respective regions, they emerged over a much longer period than bees in the constant regimen treatment (Figures 3, 5B and Table 2). Bees in the UT-natural group emerged over a 32-day period, 85% of which emerged between Day 350 (peak male emergence) and Day 366 (peak female emergence). Bees in the WA-natural group emerged over a 26-day period, 95% of them emerged between Day 344 (peak male emergence) and Day 360 (peak female emergence).
4. Discussion
Our study revealed the effects of O. lignaria origin and rearing temperature on development and survival. Use of the common, constant temperature regimen for UT- and WA-sourced bees allowed for isolating the differences between the populations in the absence of temperature variation. Use of the natural orchard temperatures allowed a comparison of development and survival for each population at both the constant and natural temperatures to reveal impacts of realistic uses of bees as pollinators in their respective localities. Insect development, activity, reproduction, and survival are strongly influenced by ambient temperature. Increased temperatures have been shown to shorten developmental periods, increase activity, and result in high survival and reproduction in Osmia (Bosch and Kemp, 2003, 2004), but of course extreme temperatures, or those outside a species’ thermal limits, are harmful (Martin and Huey, 2008; McCabe et al., 2019). However, responses to ambient temperature can vary by species and by populations within species (Colinet et al., 2015; Jarimi et al., 2020; Orr et al., 2021; Porter, 1988; Rebaudo and Rabhi, 2018; Wilson, 2019). Our work corroborates these two former statements. Understanding how temperature affects solitary bees managed to provide pollination services can help to not only assure population survival, but also inform how to use temperature management for timing adult bee emergence with crop bloom, especially when the crop is located where climate differs from that of the bees’ origin (Bosch et al., 2000; Sgolastra et al., 2011; Pitts-Singer et al., 2014).
At the constant warm temperature, young UT and WA larvae fed and grew very quickly. The orchard temperatures of both states during larval development times fluctuated but were much lower than 26°C, meaning that larval growth was slowed under cool conditions. Conversely, the prepupal period was prolonged at constant 26°C compared to those at orchard temperatures. During the prepupal and pupal stages, the mean orchard temperature never reached 26°C, but maximum orchard temperatures exceeded 30°C and most likely shortened these life stage durations. Warm temperatures have been known to speed up development and/or increase mortality; both are consistent with our results (Bosch and Kemp, 2000).
Overall bee mortality from egg to adult emergence under the constant thermal regimen was low. The level of larval mortality in this study (<20% total) was similar to a previous laboratory study with UT bees held at 22 and 26°C (Bosch and Kemp, 2000). Interestingly, we found mortality was 11% for larval WA bees, but less than 3% for larval UT bees. We suggest that WA bees may be more adversely affected by warmer than natural spring and early summer conditions than are UT bees. Pitts-Singer et al. (2014) exposed offspring of UT and WA populations to temperatures simulating a California almond orchard that included hotter maximum temperatures than either of the natural temperature regimens used in this study. That study also found a difference in larval mortality according to parental bee origin with mortality for WA male larvae being lower than for UT male larvae, but mortality for WA female larvae being higher than for UT female larvae.
At natural orchard temperatures, mortality was higher overall than when bees were raised at a constant temperature. The greatest mortality was in the life stages that experienced the highest maximum temperatures, which were the prepupal and pupal stages for UT bees and the pupal and adult stages for the WA bees. Bosch and Kemp (2000) found that some bees from northern UT failed to complete prepupal dormancy only at the lowest temperatures tested (constant 18°C). However, our UT-natural bees experienced a range of temperatures that were well above and just below 18°C. Although there was a decline in UT temperatures during the prepupal stage, it was only about as cool as the hottest temperature during the WA prepupal stage. McCabe et al. (2022) also found that maximum temperatures were likely to predict prepupal and pupal mortality in megachilids in natural montane environments.
Differences by bee parental origin in timing of development under fluctuating temperatures also resemble the results found by Pitts-Singer et al. (2014). In this study and the previous one, WA bees took slightly longer to reach adulthood than UT bees, and the duration of the UT prepupal period was much shorter and pupal period longer than the durations of those life stages for WA bees. Potentially, this quicker development to adulthood for WA bees could lead to lethal or sublethal effects if care is not taken to properly manage bees from different origins. Bosch et al. (2010) found that when bees remained at warm temperatures in their adult stage for greater than 30 days, their fat bodies were depleted, and adults were less likely to emerge. There has also been evidence that bees with smaller body sizes (perhaps due to quick development) are poor pollinators (Jauker et al., 2016). Therefore, by not understanding the development timing of these bees, farmers and bee managers could be releasing suboptimal pollinators in their orchards.
Managing bees via artificial conditions to time their release for commercial pollination is thought to be more effective than allowing for natural emergence (Bosch and Blas, 1994; Bosch et al., 2000, 2008; Bosch and Kemp, 2000). In our study, the use of a constant thermal regimen for both an early and late cherry bloom event resulted in synchronous and quick bee emergence from cocoons. Management recommendations for commercial populations are to allow for at least 180 days of winter temperatures to obtain narrow peaks of bee emergence (Bosch and Kemp, 2001); the cocooned adults in this study received >240 days of winter temperatures (4°C). The natural emergence of bees from both UT and WA occurred very close to 1 year since they were collected as eggs, which was around the time of natural orchard bloom. These bees did not experience temperatures at or below 4°C of wintering until approximately 150 days before they emerged in spring. This short wintering period may be the reason for the window of natural adult emergence being wider than for the managed bees. Males in the natural thermal regimen emerged slightly ahead of cherry bloom, and females emerged more or less in synchrony with the timing of cherry bloom. Naturally emerging bees would benefit from supplemental, early blooming floral resources to sustain early emerging male bees prior to female emergence. After crop bloom has ceased, females (some just recently out of cocoons) could continue nesting if floral resources are available (Boyle et al., 2020).
Overall, the effect of the various timings of higher-than-average temperatures implies that using bees in climates with temperatures warmer than, or at the extreme ends of, their native temperature ranges can be detrimental for developing bees. Scenarios where temperature extremes are experienced by O. lignaria are realistic, as in California for almond pollination when bees are left to develop there. If bees from Utah and Washington produce offspring in California during February almond bloom, then many of those progenies are unlikely to survive if not managed for much of the year under controlled temperatures. Proper bee management strategies will also be needed if climate change predictions of a 3–8°C increase in Earth’s temperature over the next 80 years are realized (Allen et al., 2018). Therefore, this current baseline understanding of temperature effects on O. lignaria and other pollinators and how different populations/species respond to new and warming conditions is needed for future management and decision making.
These results provide baseline expectations for the performance of bees as pollinators in areas where they presumably are regionally adapted to climatic conditions. Practical management implications are that bees used for orchard (or other crop) pollination should be moved during larval development periods (>7–9 weeks after nesting, before larvae make cocoons and enter the prepupal stage) to a location where they can avoid high temperatures such as the maximum 30°C that bees experienced in this study. Maintaining bees at a constant temperature, such as 26°C, prior to reaching adulthood might also better synchronize adult eclosion so that the timing for winter storage is easy to predict and accommodate, especially when the target, pollinator-dependent crop blooms in early spring.
The differences in development between the UT and WA populations at constant and natural thermal temperatures may indicate a genes × environment (G × E) interaction. Our work indicates that each population genotype is responding to environmental conditions (in this case, temperature) in a different way. This G × E interaction is important to understand especially when translocating managed bees to novel climates. Honey bee populations have been shown to have different behavioral responses when moved to a common environment (Costa et al., 2012). As the climate continues to warm, different O. lignaria population genotypes may be better adapted to tolerating increased temperatures, making some populations more optimal than others to serve as pollinators. Further research is needed to understand whether geographically distinct bee populations present different physiological thermal tolerances, differences in developmental timing of specific life stages, or differences in ability to adapt to novel environments over generations, when extreme climatic conditions are experienced, such as prolonged or shortened summer quiescence as prepupae and earlier or later spring adult emergence.
Data availability statement
The raw data supporting the conclusions of this article will be made available by the authors, without undue reservation.
Author contributions
MS, TP-S, and DA: conceptualization. MS: data curation and investigation. MS and LM: formal analysis. TP-S and DA: funding acquisition and supervision. MS and TP-S: methodology and project administration. TP-S: resources. MS, TP-S, and LM: visualization and writing—original draft. MS, TP-S, LM, and DA: writing—reviewing and editing. All authors contributed to the article and approved the submitted version.
Funding
For funding this project, we thank USDA National Institute of Food and Agriculture, Western Sustainable Research and Education, award number SW17-077.
Acknowledgments
For the collection of bees, we thank Natalie Boyle and Mary-Kate Williams. For technical support, we gratefully acknowledge Jenna Frost-Wake and Ellen Klomps. For graphic design of Figure 3, we thank Erica J. Brus.
Conflict of interest
The authors declare that the research was conducted in the absence of any commercial or financial relationships that could be construed as a potential conflict of interest.
Publisher’s note
All claims expressed in this article are solely those of the authors and do not necessarily represent those of their affiliated organizations, or those of the publisher, the editors and the reviewers. Any product that may be evaluated in this article, or claim that may be made by its manufacturer, is not guaranteed or endorsed by the publisher.
Supplementary material
The Supplementary material for this article can be found online at: https://www.frontiersin.org/articles/10.3389/fevo.2023.1083448/full#supplementary-material
References
Allen, M. R., Babiker, M., Chen, Y., de Coninck, H., Connors, S., van Diemen, R., et al. (2018). “Summary for policymakers” in Global warming of 1.5: An IPCC special report on the impacts of global warming of 1.5°C above pre-industrial levels and related global greenhouse gas emission pathways, in the context of strengthening the global response to the threat of climate change, sustainable development, and efforts to eradicate poverty. eds. V. Masson-Delmotte, P. Zhai, H.-O. Pörtner, D. Roberts, J. Skea, and P. R. Shukla, et al. (Geneva: World Meteorological Organization).
Andrikopoulos, C. J., and Cane, J. H. (2018). Comparative pollination efficacies of five bee species on raspberry. J. Econ. Entomol. 111, 2513–2519. doi: 10.1093/jee/toy226
Artz, D. R., Allan, M. J., Wardell, G. I., and Pitts-Singer, T. L. (2013). Nesting site density and distribution affect Osmia lignaria (hymenoptera: Megachilidae) reproductive success and almond yield in a commercial orchard. Insect Conserv. Divers. 6, 715–724. doi: 10.1111/icad.12026
Bates, D., Maechler, M., Bolker, B., and Walker, S. (2015). Fitting Linear Mixed-Effects Models Using lme4. J. Statistical Software. 67, 1–48. doi: 10.18637/jss.v067.i01
Bosch, J., and Blas, M. (1994). Effect of over-wintering and incubation temperatures on adult emergence in Osmia cornuta Latr (hymenoptera, Megachilidae). Apidologie 25, 265–277. doi: 10.1051/apido:19940301
Bosch, J., and Kemp, W. P. (2000). Development and emergence of the orchard pollinator Osmia lignaria (hymenoptera: Megachilidae). Environ. Entomol. 29, 8–13. doi: 10.1603/0046-225X-29.1.8
Bosch, J., and Kemp, W. P. (2001). How to manage the blue orchard bee as an orchard pollinator. Sustainable Agricultural Network: Beltsville, MD 98.
Bosch, J., and Kemp, W. P. (2003). Effect of wintering duration and temperature on survival and emergence time in males of the orchard pollinator Osmia lignaria (hymenoptera: Megachilidae). Environ. Entomol. 32, 711–716. doi: 10.1603/0046-225X-32.4.711
Bosch, J., and Kemp, W. P. (2004). Effect of prewintering and wintering temperatures on weight loss, survival, and emergence time in the mason bee Osmia cornuta (hymenoptera: Megachillidae). Apidologie 35, 469–479. doi: 10.1051/apido:2004035
Bosch, J., Kemp, W. P., and Peterson, S. S. (2000). Management of Osmia lignaria (hymenoptera: Megachilidae) populations for almond pollination: methods to advance bee emergence. Environ. Entomol. 29, 874–883. doi: 10.1603/0046-225X-29.5.874
Bosch, J., Kemp, W. P., and Trostle, G. E. (2006). Bee population returns and cherry yields in an orchard pollinated with Osmia lignaria (hymenoptera: Megachillidae). J. Econ. Entomol. 99, 408–413. doi: 10.1093/jee/99.2.408
Bosch, J., Sgolastra, F., and Kemp, W. P. (2008). “Life cycle ecophysiology of Osmia mason bees used as crop pollinators” in Bee pollination in agricultural ecosystems. eds. R. R. James and T. L. Pitts-Singer (Oxford, NY: Oxford University Press), 83–104.
Bosch, J., Sgolastra, F., and Kemp, W. P. (2010). Timing of eclosion affects diapause development, fat body consumption and longevity in Osmia lignaria, a univoltine, adult-wintering solitary bee. J. Insect Physiol. 56, 1949–1957. doi: 10.1016/j.jinsphys.2010.08.017
Boyle, N. K., Artz, D. R., Lundin, O., Ward, K. L., Picklum, D., Wardell, G. I., et al. (2020). Wildflower plantings promote blue orchard bee, Osmia lignaria (hymenoptera: Megachilidae), reproduction in California almond orchards. Ecol. Evol. 10, 10, 3189–3199. doi: 10.1002/ece3.5952
Brown, M. J. F., and Paxton, R. J. (2009). The conservation of bees: a global perspective. Apidologie 40, 410–416. doi: 10.1051/apido/2009019
Cane, J. H. (2003). “Exotic nonsocial bees (hymenoptera: Apiformes)in North America: ecological implications” in For nonnative crops, whence pollinators of the future. eds. K. Strickler and J. H. Cane (Lanham, MD: Entomological Society of America), 113–126.
Colinet, H., Sinclair, B. J., Vernon, P., and Renault, D. (2015). Insects in fluctuating thermal environments. Annu. Rev. Entomol. 60, 123–140. doi: 10.1146/annurev-ento-010814-021017
Costa, C., Lodesani, M., and Bienefeld, K. (2012). Differences in colony phenotypes across different origins and locations: evidence for genotype by environment interactions in the Italian honeybee (Apis mellifera ligustica)? Apidologie 43, 634–642. doi: 10.1007/s13592-012-0138-9
Gelman, A., and Su, Y.S. (2022). arm: Data Analysis Using Regression and Multilevel/Hierarchical Models. R package version. 1, 13–1. Available at: https://CRAN.R-project.org/package=arm
Helm, B. R., Rinehart, J. P., Yocum, G. D., Greenlee, K. J., and Bowsher, J. H. (2017). Metamorphosis is induced by food absence rather than a critical weight in the solitary bee, Osmia lignaria. PNAS 114, 10924–10929. doi: 10.1073/pnas.1703008114
Horth, L., and Campbell, L. A. (2018). Supplementing small farms with native mason bees increases strawberry size and growth rate. J. Appl. Ecol. 55, 591–599. doi: 10.1111/1365-2664.12988
Jarimi, H., Tapia-Brito, E., and Riffat, S. (2020). A review on thermoregulation techniques in honey bees’(Apis mellifera) beehive microclimate and its similarities to the heating and cooling management in buildings. Future Cities Environ. 6:7. doi: 10.5334/fce.81
Jauker, F., Speckmann, M., and Wolters, V. (2016). Intra-specific body size determines pollination effectiveness. Basic Appl. Ecol. 17, 714–719. doi: 10.1016/j.baae.2016.07.004
Khalifa, S. A. M., Elshafiey, E. H., Shetaia, A. A., Abd El-Wahed, A. A., Algethami, A. F., Musharraf, S. F., et al. (2021). Overview of bee pollination and its economic value for crop production. Insects 12:688. doi: 10.3390/insects12080688
Krunić, M. D., and Stanisavljević, L. Z. (2006). Augmentation of managed populations of Osmia cornuta and O. rufa (Hymenoptera: Megachilidae) in Southeastern Europe. Eur. J. Entomol. 103, 695–697. doi: 10.14411/eje.2006.091
Klein, A.-M., Vaissière, B. E., Cane, J. H., Steffan-Dewenter, I., Cunningham, S. A., Kremen, C., et al. (2007). Importance of pollinators in changing landscapes for world crops. Proc. Royal Soc. B 274, 303–313. doi: 10.1098/rspb.2006.3721
Kopit, A. M., Klinger, E. G., Cox-Foster, D. L., Ramirez, R. A., and Pitts-Singer, T. L. (2022). Effects of provision types and pesticide exposure on the larval development of Osmia lignaria (hymenoptera: Megachilidae). Environ. Entomol. 51, 240–251. doi: 10.1093/ee/nvab119
LeCroy, K. A., Savoy-Burke, G., Carr, D. E., and Delaney, D. A. (2020). Decline of six native mason bee species following the arrival of an exotic congener. Sci. Rep. 10:18745. doi: 10.1038/s41598-020-75566-9
Lenth, R. V. (2016). Least-squares means: the R package lsmeans. J. Stat. Softw. 69, 1–33. doi: 10.18637/jss.v069.i01s
Levin, M. D. (1957). Artificial nesting burrows for Osmia lignaria say. J. Econ. Entomol. 50, 506–507. doi: 10.1093/jee/50.4.506
Martin, T. L., and Huey, R. B. (2008). Why “suboptimal” is optimal: Jensen’s inequality and ectotherm thermal preferences. Am. Nat. 171, E102–E118. doi: 10.1086/527502
MacIvor, J. S., de Keyer, C. W., Marshall, M. S., Thurston, G. S., and Onuferko, T. M. (2022). Establishment of the non-native horn-face bee Osmia cornifrons and the taurus mason bee Osmia taurus (Hymenoptera: Megachilidae) [in Canada] PeerJ. 10:e14216. doi: 10.7717/peerj.14216
Maeta, Y., Okamura, S., and Ueda, H. (1990). Use of the Mamekobachi, Osmia cornifrons (Radoszkowski), as a pollinator of blueberries (Hymenoptera, Megachilidae). Report of Chugoku Branch. Odokou. 32, 33–42.
Matsumoto, S., and Maejima, T. (2010). Several new aspects of the foraging behavior of Osmia cornifrons in an apple orchard. Psyche 2010, 1–6. doi: 10.1155/2010/384371
McCabe, L. M., Aslan, C. E., and Cobb, N. S. (2022). Decreased bee emergence along an elevation gradient: implications for climate change revealed by a transplant experiment. Ecology 103:e03598. doi: 10.1002/ecy.3598
McCabe, L. M., Cobb, N. S., and Butterfield, B. J. (2019). Environmental filtering of body size and darker coloration in pollinator communities indicate thermal restrictions on bees, but not flies, at high elevations. PeerJ. 7:e7867. doi: 10.7717/peerj.7867
Meeus, I., Pisman, M., Smagghe, G., and Piot, N. (2018). Interaction effects of different drivers of wild bee decline and their influence on host–pathogen dynamics. Curr. Opin. Insect Sci. 26, 136–141. doi: 10.1016/j.cois.2018.02.007
MesoWest (2021). University of Utah, Department of Atmospheric Sciences. Available at: https://mesowest.utah.edu (Accessed January 16, 2019).
Ollerton, J., Winfree, R., and Tarrant, S. (2011). How many flowering plants are pollinated by animals? Oikos 120, 321–326. doi: 10.1111/j.1600-0706.2010.18644.x
Orchard Bee Association. (2021). Guidelines for sustainably sourcing and managing mason bees: Management during bee development. Available at: https://www.orchardbee.org/guidelines
Orr, M. C., Hughes, A. C., Chesters, D., Pickering, J., Zhu, C. D., and Ascher, J. S. (2021). Global patterns and drivers of bee distribution. Curr. Biol. 31, 451–458.e4. doi: 10.1016/j.cub.2020.10.053
Osterman, J., Aizen, M. A., Biesmeijer, J. C., Bosch, J., Howlett, B. G., Inouye, D. W., et al. (2021). Global trends in the number and diversity of managed pollinator species. Agric. Ecosyst. Environ. 322:107653. doi: 10.1016/j.agee.2021.107653
Pettis, J. S., and Delaplane, K. S. (2010). Coordinated responses to honey bee decline in the USA. Apidologie 41, 256–263. doi: 10.1051/apido/2010013
Pitts-Singer, T. L., Artz, D. R., Peterson, S. S., Boyle, N. K., and Wardell, G. I. (2018). Examination of a managed pollinator strategy for almond production using Apis mellifera (hymenoptera: Apidae) and Osmia lignaria (hymenoptera: Megachilidae). Environ. Entomol. 47, 364–377. doi: 10.1093/ee/nvy009
Pitts-Singer, T. L., and Cane,. (2011). The alfalfa leafcutting bee, Megachile rotundata: the world’s most intensively managed solitary bee. Annual review of entomology, 56, 221–237.
Pitts-Singer, T. L., Cane, J. H., and Trostle, G. (2014). Progeny of Osmia lignaria from distinct regions differ in developmental phenology and survival under a common thermal regime. J. Insect Physiol. 67, 9–19. doi: 10.1016/j.jinsphys.2014.05.018
Pitts-Singer, T. L., Kemp, W. P., Moreland, D., Peterson, S., Buckner, J. S., and Hagen, M. (2016). Bee attractants. U.S. patent no. 9, 301, 501 B2. Washington, DC: U.S. Patent and Trademark Office.
Porter, S. D. (1988). Impact of temperature on colony growth and developmental rates of the ant, Solenopsis invicta. J. Insect Physiol. 34, 1127–1133. doi: 10.1016/0022-1910(88)90215-6
PRISM Climate Group. (2022) Oregon State University. Available at: https://prism.oregonstate.edu (Accessed May 24, 2022).
Rebaudo, F., and Rabhi, V.-B. (2018). Temperature-dependent development rate and phenology in insects: review of major developments, challenges, and future directions. Entomol. Exp. Appl. 166, 607–617. doi: 10.1111/eea.12693
Rust, R. W. (1974). The systematics and biology of the genus Osmia, subgenera Osmia, Chalcosmia, and Cephalosmia (hymenoptera: Megachilidae). Wasmann J. Biol. 32:1.
Sedivy, C., and Dorn, S. (2014). Towards a sustainable management of bees of the subgenus Osmia (Megachilidae; Osmia) as fruit tree pollinators. Apidologie, 45, 88–105.
Sgolastra, F., Kemp, W. P., Buckner, J. S., Pitts-Singer, T. L., Maini, S., and Bosch, J. (2011). The long summer: pre-wintering temperatures affect metabolic expenditure and winter survival in a solitary bee. J. Insect Physiol. 57, 1651–1659. doi: 10.1016/j.jinsphys.2011.08.017
Sgolastra, F., Kemp, W. P., Maini, S., and Bosch, J. (2012). Duration of prepupal summer dormancy regulates synchronization of adult diapause with winter temperatures in bees of the genus Osmia. J. Insect Physiol. 58, 924–933. doi: 10.1016/j.jinsphys.2012.04.008
Tepedino, V. J., Parker, F. D., and Durham, S. L. (2022). Mixed diapause duration in cohorts of four species of Osmia bees (Megachilidae) along an elevation and temperature gradient in northern Utah (USA). J. Apic. Res. 61, 481–491. doi: 10.1080/00218839.2022.2042049
Torchio, P. F. (1985). Field experiments with the pollinator species, Osmia lignaria propinqua Cresson, in apple orchards: V (1979-1980), methods of introducing bees, nesting success, seed counts, fruit yields (Hymenoptera: Megachilidae). Journal of the Kansas Entomological Society, 448–464.
Torchio, P. F. (1989). In-nest biologies and development of immature stages of three Osmia species (hymenoptera: Megachilidae). Ann. Entomol. Soc. Am. 82, 599–615. doi: 10.1093/aesa/82.5.599
Torchio, P. F. (2003). “The development of Osmia lignaria (hymenoptera: Megachilidae) as a managed pollinator of apple and almond crops: a case history” in For nonnative crops, whence pollinators of the future? eds. K. Strickler and J. H. Cane (Lanham, MD: Entomological Society of America), 67–84.
United States Department of Agriculture National Agricultural Statistics Service. (2006). Fruit and tree nuts: blooming, harvesting and marketing dates. Agriculture handbook number 729. Available at: https://usda.library.cornell.edu/concern/publications/qr46r081g
Velthuis, H. H. W., and van Doorn, A. (2006). A century of advances in bumblebee domestication and the economic and environmental aspects of its commercialization for pollination. Apidologie 37, 421–451. doi: 10.1051/apido:2006019
Wilson, E. S. (2019). Nest cavity temperature impacts nesting choice and development of the alfalfa leafcutting bee (Megachile rotundata). master’s thesis. Fargo, ND: North Dakota State University
Keywords: blue orchard bee, solitary, Megachilidae, phenology, environmental stressors, genes × environment, ecophysiology
Citation: Scalici MB, McCabe LM, Alston DG and Pitts-Singer TL (2023) Effects of geographic origin and temperature on survival, development, and emergence of the managed pollinator Osmia lignaria. Front. Ecol. Evol. 11:1083448. doi: 10.3389/fevo.2023.1083448
Edited by:
Lars Straub, University of Bern, SwitzerlandReviewed by:
Jessica Forrest, University of Ottawa, CanadaJulia Osterman, University of Freiburg, Germany
Blair Sampson, Southern Horticultural Research Laboratory, Agricultural Research Service (USDA), United States
Copyright © 2023 Scalici, McCabe, Alston and Pitts-Singer. This is an open-access article distributed under the terms of the Creative Commons Attribution License (CC BY). The use, distribution or reproduction in other forums is permitted, provided the original author(s) and the copyright owner(s) are credited and that the original publication in this journal is cited, in accordance with accepted academic practice. No use, distribution or reproduction is permitted which does not comply with these terms.
*Correspondence: Morgan B. Scalici, bWJzY2FsaWNpQGdtYWlsLmNvbQ==