Impact of host stress on the replication rate of Plasmodium: take it easy to avoid malaria recurrences
- 1Department of Ecology and Evolution, Lausanne University, Lausanne, Switzerland
- 2Laboratoire Ecologie et Biologie des Interactions (EBI), UMR CNRS 7267, Poitiers, France
- 3Musée Cantonal de Zoologie, Lausanne, Switzerland
Malaria is widespread throughout the world and affects many animal species. Although the origin of this vector-borne disease was discovered more than a century ago, several aspects of the within-host infection dynamic are still poorly understood. Among them, the factors triggering parasite recurrences – episodes of brief increase in parasite number following a period when the parasite was either absent or present at very low levels in the blood – have still not been clearly identified. Yet, recurrences may contribute significantly to overall infection prevalence in vertebrate host populations. Here, we investigated whether artificial or natural increases in stress hormone levels in chronically infected birds influence the replication rate of Plasmodium relictum and transmission to its natural vector, the mosquito Culex pipiens. Our results provide evidence that increased levels of corticosterone, either induced by oral ingestion or caused by handling stress, can trigger malaria recurrences. However, we did not observe any effect on the transmission rate of the parasite to the mosquito vector. Our study is a first fundamental step in understanding the mechanisms underlying malaria recurrences. It remains to be ascertained whether this feature extends to other malaria system and in particular to human malaria.
1 Introduction
A large diversity of parasites, ranging from viruses to eukaryote organisms, are characterized by active and latent infection stages (Hasker et al., 2013; Colangeli et al., 2020). While the first are those where the parasite actively replicates and may cause symptoms, latent infections usually do not cause any symptoms and are often hardly detectable at this stage. Latent infections may therefore play a significant epidemiological role by increasing the number (Fradejas et al., 2019) but also the mobility of the sources of infection (Angrisano and Robinson, 2022). Reactivation of latent parasite stage may be one of the factors triggering an outbreak, as a number of infected asymptomatic individuals are undetected, and may also generate sporadic cases of infection in non-endemic areas (Fradejas et al., 2019; Angrisano and Robinson, 2022). The identification of the mechanisms responsible for the recurrence of parasites, that is the transition from latent to active stages of the infection, is therefore essential to understand the dynamics of infection and to develop new strategies to attempt to control infectious diseases.
Among the parasites that exhibit cycles of latency and reactivation (i.e. susceptible–infected–latent–infected dynamics), Plasmodium is one of the most studied. This vector-borne parasite infects many vertebrate hosts, including reptiles, birds and human or non-human primates. After parasite transmission from a mosquito vector, the intensity of infection in the vertebrate host’s peripheral blood changes drastically over time. Following an initial acute phase (i.e. active infection stage), characterized by a high number of parasites in the blood, the infection is either cleared or reaches a chronic phase (i.e. latent infection stage). During this latent infection phase, the parasite burden stabilizes at a low level for several months or years (Ashley and White, 2014; Ishtiaq, 2021). Latent infection may however be interrupted by several episodes of intense, but brief, increase in parasite replication rate (i.e. malaria recurrences, (Applegate, 1971; Ishtiaq, 2021). These bouts of replication rate may be due to relapse or to recrudescence. While the former results from the activation of dormant liver-stage parasites, called hypnozoites (Zanghi and Vaughan, 2021), the latter is due to incomplete elimination of erythrocytic stages either in the blood or in extra-vascular compartments (Markus, 2018; Popovici et al., 2019). In practice, it is difficult to determine whether a significant increase in parasitemia (i.e. percentage of erythrocytes infected) during the latent stage of infection is due to a relapse or a recrudescence. In the following, the term recurrence will therefore be used to encompass both cases.
Malaria recurrences contribute significantly to overall infection rates in vertebrate host populations and is an important source of transmission (Betuela et al., 2012; Taylor et al., 2019; Commons et al., 2020). However, the periodicity of malaria recurrences is not clearly defined and seems to vary according to parasite species (Cogswell, 1992), geographical area (Battle et al., 2014) and host medical treatment (Taylor et al., 2019). Nevertheless, in temperate region, a seasonal pattern seems to emerge. In primate (human and non-human), avian and saurian malaria, recurrences are most often observed in spring or early summer, irrespective of the timing of primary infection (Applegate and Beaudoin, 1970; Schall, 1996; Cosgrove et al., 2008; White et al., 2016), which suggests that the parasite reacts to changes in its environment. These seasonal recurrences may be viewed as a strategy that allows the parasite to match the annual dynamics of vector populations (Cornet et al., 2014b). In temperate zones, mosquito vectors are only present for a few months (Lalubin et al., 2013) and parasites may benefit from using environmental cues to coincide with the occurrence of mosquitoes (Valkiunas, 2004; Cornet et al., 2014b). However, nonseasonal relapses may also occur at any time of the year, without clear connection with seasonal change in the environment (Valkiunas, 2004; Shanks, 2015).
For more than half a century, various authors have hypothesized that host hormonal systems could function as cues for parasites (Applegate and Beaudoin, 1970; Cornelius et al., 2014). The increase in the amount of sex hormones in the blood during the breeding season, which correlates with the increase in day length in spring and thus with the presence of mosquitoes, has often been advanced as a potential trigger of seasonal recurrences. To date, there is no empirical evidence confirming a role of reproductive hormones in both malaria relapses and recrudescence (Applegate and Beaudoin, 1970; Pearson, 2002; Slowinski et al., 2022). Conversely, studies, primarily in the avian malaria system, suggest that stress-related hormonal changes could increase host parasitemia during the acute and latent phases of infection (Schoenle et al., 2019; Names et al., 2021). Since the concentration of stress hormones increases during the reproductive period in many organisms (Eggermann et al., 2013; Reedy et al., 2014), it has been postulated that these hormones, possibly in combination with others, play a key role in seasonal malaria recurrences (Applegate and Beaudoin, 1970). Variations in stress-related hormones levels could also be involved in non-seasonal recurrences since it has been shown that malaria infection intensity is influenced by hosts’ exposition to stressful conditions (e.g. nutritional stress, pollution, artificial light at night; Cornet et al., 2014a; Becker et al., 2020). The mechanisms by which variation in stress hormone concentration could induce hypnozoite activation or influence Plasmodium replication rate have never been experimentally investigated. However, recent studies have highlighted that exposure to chronic or acute stress can have adverse effects on host adaptive and innate immune function (Cain and Cidlowski, 2017; Gao and Deviche, 2019).
In addition to indirect signals from the environment (i.e. host hormones), it has been suggested that malaria parasites may fine-tune their investment in replication rate by using direct cues from vectors themselves to best match their phenology and abundance. Several studies have indeed shown that Plasmodium responded plastically to mosquito blood feeding bout by increasing parasitemia (Lawaly et al., 2012; Cornet et al., 2014b; Pigeault et al., 2018). This phenomenon is particularly significant during the latent stage of infection and could be one of the mechanisms triggering recurrences. While it is not excluded that Plasmodium directly detects mosquito cues (e.g. proteins) present in the saliva injected during the blood meal (Huldén and Huldén, 2011), the response of Plasmodium to mosquito bites could, as suggested by Dhondt and Dobson (Dhondt and Dobson, 2017), be mediated by the stress response of the vertebrate host. Exposure to blood-sucking insect may generate stress (Kavaliers and Colwell, 1996; Martínez-de la Puente et al., 2011) and this physiological response, through its effect on host’s immune system (Cain and Cidlowski, 2017; Gao and Deviche, 2019), might underlie the increase in Plasmodium replication rates observed a few days after mosquito bites (Dhondt and Dobson, 2017).
In the present study, we investigated whether an acute stress triggers malaria recurrence, under both controlled and semi-natural conditions. We also tested whether the increase in parasite burden, observed in vertebrate blood a few days after mosquito bites, could be mediated by the physiological stress response of the vertebrate host. For this purpose, we increased, either artificially (i.e. orally manipulation of corticosterone levels) or naturally (i.e. handling stress), the stress hormone concentration of chronically infected host during a short period of time and monitored the impact on Plasmodium replication rate and transmission to mosquito vectors. Thereafter, we explored the association between mosquito bites and the vertebrate blood stress hormone concentrations to determine whether stress associated with mosquito exposure might be sufficient to induce recurrence. The last step of our study was to test if the results obtained under laboratory conditions were reproducible under semi-natural conditions. To do so, we experimentally infected wild vertebrate hosts and placed them in outdoor cages. In addition to documenting Plasmodium infection dynamics during one year, we studied the impact of stress induced by handling or by handling and mosquito bites on the replication rate of the malaria parasite. Avian malaria was used as a system to investigate these questions. Avian malaria is the oldest experimental system for investigating the life cycle of Plasmodium parasites and particularly to study recurrences (Rivero and Gandon, 2018). Further, avian malaria is the only currently available animal experimental system that allows working with a parasite recently isolated from the wild (Plasmodium relictum) and with its natural mosquito vector (Culex pipiens).
2 Materials and methods
2.1 Biological system
Plasmodium relictum (lineage SGS1) is the most common and prevalent parasite lineage causing avian malaria in Europe and is known to induce a long latent phase marked by sudden events of recurrences (Valkiunas, 2004). The parasite strain used in the first and second experiment was isolated from two infected House sparrows (Passer domesticus) captured in January 2019 and December 2020. The fourth experiment was carried out with a P. relictum strain isolated from a House sparrow captured in August 2018.
The first three experiments were carried out with domestic canaries (Serinus canaria) while the fourth experiment was performed with juvenile house sparrows. Both bird species are known to be naturally infected with Plasmodium relictum (lineage SGS1). Indeed, House sparrows infected by this lineage have been described all over Europe, and canaries, when kept in outdoor aviaries, can commonly acquire this infection. House sparrows used in this experiment were captured with mist nets in on the campus of the University of Lausanne, Switzerland (46°31′25.607″N 6°34′40.714″E, altitude: 380 m). Prior to the experiments, a small amount (ca. 3–5 µL) of blood was collected from the medial metatarsal vein of each bird (i.e. canary and house sparrow) to ensure that they were free from any hemosporidian infection (Hellgren et al., 2004, for further details see Supplementary Material, Section 1).
When experiments required the use of mosquitoes, these were conducted with a line of Culex pipiens collected in Lausanne (Switzerland, 46°31′25.607″N 6°34′40.714″E) in August 2017, and maintained in our insectarium since. Mosquitoes were reared using standard protocols (25°C ± 1°C, 70 ± 5% RH and with 12L:12D photoperiod). We used females 7–13 days after emergence, which had not previously access to blood meals. Mosquitoes were maintained on glucose solution (10%) since their emergence and were starved (but provided with water to prevent dehydration) for 24h before the experiments.
2.2 Experiment 1: impact of artificial increase of corticosterone concentration in vertebrate blood on Plasmodium replication rate and transmission
Eighteen canaries were infected by intraperitoneal injection of 150µL of an infected blood pool (day 0). The blood pool was made of a 1:1 mixture of PBS and mixed blood sample from eight canaries that had been similarly infected two weeks before the experiment (between 200 and 300 µL of blood were taken from each of the eight canaries from their brachial veins, Pigeault et al., 2015). After experimental infection, blood from the medial metatarsal vein (2–3 µL) was sampled in all birds to monitor parasitemia during the acute (10 dpi, i.e. day post-infection) and the latent pre-experimental stage of infection (35, 47 dpi). One individual died day 25 post-infection. The remaining were assigned to two different experimental groups: “CORT” (n=9) and “Control” (n=8). To that end, we ranked the individuals by decreasing parasitemia level [measured by quantitative PCR (qPCR) during the acute stage of infection, i.e. 10 dpi]. We randomly assigned one of the two individuals with the highest parasitemia levels to each experimental group. We repeated this for the two individuals with the next highest ranks until all individuals were assigned to a treatment group.
Corticosterone is the main glucocorticoid in birds and is an essential component of their stress response (Cockrem, 2007). To study the impact of an artificial increase of this stress hormone on Plasmodium replication rate during the latent infection stage, we carried out an experiment consisting of four experimental days separated by three days interval. Due to the high number of manipulations, all experimental days were split into two blocks corresponding to two consecutive days. Eight birds were randomly selected and assigned to the first experimental block (experimental days: 52, 55, 58 and 61 dpi). The remaining nine individuals were assigned to the second experimental block (experimental days: 53, 56, 59 and 62 dpi). At 5:45 PM of each experimental day, birds ingested either 20 μL of corticosterone solution (CORT group, 20 μg of corticosterone diluted in 20 μL of dimethyl sulfoxide, DMSO, dose per body mass ≈ 1 μg/g, (Breuner et al., 1998) or 20 μL of DMSO (control group). A pipette with a 200 µL tip was used to inject the solutions directly into the birds’ beaks. The ingestion of 20 μg of corticosterone solution induced a rapid and strong increase in corticosterone concentration in birds’ blood (average increase of 46.4 ng/mL ± 16 ng/mL compared to an increase of 2.17 ng/mL ± 0.45 ng/mL in the control group, see Supplementary Material, Section 2). At 6:00 PM, birds were immobilized in PVC tubes (length 18 cm, diameter 4.5 cm) covered by a mesh at both ends and placed in individual experimental cages (L40 × W40 × H40 cm) in the dark for two hours. At 8:00 PM, blood samples from the medial metatarsal vein (ca. 2–3 µL) were taken from all individuals to quantify parasitemia by qPCR. A red lamp was used to capture the birds and collect the blood samples to avoid stressing other individuals in the room.
To explore the impact of corticosterone ingestion on Plasmodium transmission from vertebrate host to mosquitoes, on the fourth experimental day (61/62 dpi), 60 ± 5 uninfected female mosquitoes were added in each cage at 7:00 PM (i.e. one hour after corticosterone or DMSO ingestion). After the blood sample has been taken from all birds at 8:00 PM, blood-fed mosquitoes were counted and individually placed in numbered plastic tubes (30 mL) covered with a mesh and with a cotton pad soaked in a 10% glucose solution. Tubes were kept in standard insectary conditions for one week. Females were then taken out of the tubes and the amount of hematin excreted at the bottom of each tube was quantified as an estimate of the blood meal size (Vézilier et al., 2010). Females were dissected, and the number of Plasmodium oocysts in their midgut counted with the aid of a binocular microscope.
2.3 Experiment 2: impact of hosts’ stress induced by handling on Plasmodium replication rate and transmission
In addition to the effect associated with the artificial increase of host corticosterone concentration on Plasmodium replication rate, the first experiment also suggested an additional effect associated to the stress induced by host handling (see results section). In the second experiment we therefore explored this issue. For this purpose, eighteen canaries were infected and their parasitemia was monitored during the acute and latent stage of infection as described above. However, to minimize the hosts’ stress induced by handling before the experiment, only one blood sample was taken during the latent pre-experimental stage of infection (35 dpi). Individuals were then assigned to two different experimental groups: “handling” (n=9) and “control” (n=9) using the same procedure as described above.
While control individuals were not manipulated, birds belonging to the “handling” group were captured at days 52 or 53, 55 or 56 and 58 or 59 post-infection (experimental days were split into two blocks as described above) and handled and restrained in the same way as in Experiment 1 to generate stress. To avoid a confounding effect between handling stress and blood loss, no blood sample was taken during this period. Then, to explore the impact of handling stress on the dynamics of Plasmodium replication rate and transmission to mosquitoes, on day 61 or 62 dpi, all birds were captured, immobilized in PVC tubes and placed, at 6:00 PM, in the dark in individual experimental cages. One hour later, all individuals were exposed for one hour to 60 ± 5 uninfected female mosquitoes. At the end of the exposure session, a blood sample (ca. 2–3 µL) was taken from the medial metatarsal vein of all individuals to quantify parasitemia by qPCR. Blood-fed female mosquitoes were counted, isolated, and dissected as described above.
Finally, for both corticosterone and handling experiments, blood samples were taken on all birds at day 65 and 75 post-infection to measure their parasitemia by qPCR. The latent infection period was therefore divided into three different stages: the latent pre-experimental stage (35–47 dpi), the latent experimental stage (52–62 dpi) and the latent post-experimental stage (65–75 dpi).
2.4 Experiment 3: effect of mosquito bites on corticosterone concentration in birds’ blood
Eighteen uninfected canaries were used for this experiment, from which nine were exposed to uninfected mosquito bites (“exposed”) and the remaining nine were used as “control”. To obtain baseline or near-baseline corticosterone levels prior to mosquito exposure, 90–110 µL of blood was taken from the brachial vein of all birds within three minutes of capture (Wingfield and Romero, 2011). Immediately after the blood sampling, birds were immobilized in PVC tube covered by a mesh at both ends and placed in individual experimental cages in the dark at 6:00 PM. After one hour of acclimatization, 60 ± 5 mosquitoes were released in each cage containing an “exposed” bird. One hour later (i.e. 8:00 PM), a new blood sample was taken from the brachial vein of all birds to quantify corticosterone concentrations. Total corticosterone from plasma samples was quantified using enzyme immunoassay kit from Enzo Life Sciences (Corticosterone ELISA Kit, cat. No. ADI-900-097, Lausen, Switzerland) following the manufacturer’s instructions. Plasma samples were obtained by centrifugation of bird blood, which was kept on ice. After centrifugation, plasma was collected and stored at −20 °C. Samples were run in duplicate and randomized across plates. Concentration values were extrapolated from standard curves and all samples felt within the standard curves. Inter-plate variation was 1.3%.
2.5 Experiment 4: impact of hosts’ stress on Plasmodium replication rate in semi-natural conditions
On October 11, 2018, seven uninfected juvenile House sparrows were infected by intraperitoneal injection of an infected blood pool (day 0) and placed together in an outdoor aviary (4.6 m × 4.6 m, 2.7 m high (complete material and methods are provided in the Supplementary Material, Section 3). Blood samples were taken regularly – i.e. between monthly and weekly, depending on the stage of infection, see Figure S3 – during one year from the medial metatarsal vein (ca. 2–3 µL) of all individuals to quantify parasitemia by qPCR. One bird died for unknown reason day 104 post-infection.
Day 295 post-infection birds were captured and housed in an individual cage (100 cm × 30 cm × 40 cm high) that was placed in the outdoor aviary. They were isolated to facilitate their capture for the rest of the experiment and thus minimize their stress. Thirteen days later (i.e. 308 dpi) each bird was captured and transported in holding bags to the laboratory, 250 m apart. A t 6:00 PM, individuals were randomly assigned to the “exposed” (n=3) or to the “control” group (n=3), immobilized in PVC tubes covered by a mesh at both extremities and placed in individual experimental cages for two hours. After one hour, 60 ± 5 mosquitoes were released in each cage containing an “exposed” bird. One hour later (i.e. 8:00 PM), a blood sample was taken from the medial metatarsal vein of all individuals to quantify parasitemia by qPCR and birds were release outside in their individual cage. Parasitemia was then monitored every 2–3 days during two weeks and birds were then released in the large aviary. Thereafter, birds’ parasitemia was monitored regularly until the end of the experiment (i.e. 365 dpi). At the end of the experiment all House sparrows were treated to clear infection and kept in the outdoor aviaries.
2.6 Molecular analyses
The quantification of blood parasitemia was carried out using qPCR with a protocol adapted from Christe et al., 2011. Briefly, DNA was extracted from the blood using a standard protocol (Qiagen DNeasy 96 blood and tissue kit). For each individual, we conducted a multiplex qPCR. We targeted the nuclear cyt b gene of Plasmodium (Primers: L4050Plasmo: 5′-GCTTTATGTATTGTATTTATAC-3′, H4121Plasmo: 5′-GACTTAAAAGATTTGGATAG-3′, TaqMan probe: CY3-CYTb-BHQ2: 5′-CCTTTAGGGTATGATACAGC-3′) and the 18s rRNA gene of the bird (Primers: Plasmo18S-f: 5′-GGCAGCTTTGGTGACTCTAGA-3′, Plasmo18S-r: 5′-AGTTGATAGGGCAGACATTCG-3′, TaqMan probe: FAM-18S-BHQ1: 5′-AACCTCGAGCCGATCGCACG-3′). All samples were run in triplicate (Bio-Rad CFX96TM Real-Time System). Parasite number was calculated with relative quantification values (RQ). RQ can be interpreted as the fold‐amount of target gene (Plasmodium 18s rDNA) with respect to the amount of the reference gene (Bird18s rDNA) and are calculated as 2−(Ct18s Plasmodium – Ct18s Bird). For convenience, RQ values were standardized by ×104 factor and log-transformed (log(x, base = exp(1))).
2.7 Statistical analysis
Analyses were carried out using the R statistical package (v. 4.0.2). Data were analyzed separately for each experiment. The different statistical models built to analyse the data are described in the Supplementary Material (Table S1). Briefly, analyses where a same individual bird was sampled repeatedly, such as corticosterone quantification or fluctuation of blood parasitemia throughout time, were analysed fitting bird as a random factor into the models (to account for the temporal pseudoreplication), using a mixed model procedure (lmer, package: lme4, Bates et al., 2015). When experimental days were split into two blocks, bird random factor was nested in experimental blocks. Bird treatment and day post-infection (experiment 1, 2 & 4) or the time of blood sampling (experiment 3) were used as fixed factors. When only one parasitemia measurement was taken per bird and experimental days were not split into two blocks, the analysis was performed using Mann-Whitney-Wilcoxon Test (i.e. comparison of bird latent pre-experimental parasitemia according to bird treatment in the handling experiment). Mosquito-centred traits, such as blood meal size (hematin quantity, ng), infection prevalence (proportion of mosquitoes containing at least one oocyst) and oocyst burden (taking account of mosquitoes with one or more oocysts in the midgut), which may depend on which bird mosquitoes fed on, were analysed fitting bird as a random factor into the models, using lmer, glmer.nb or glmer (package: lme4) according to whether the errors were normally (hematin quantity), binomially (infection prevalence) or negative binomially distributed (oocyst burden). Bird treatment and, when necessary, blood meal size (haematin) were used as fixed factors.
Maximal models, including all higher-order interactions, were simplified by sequentially eliminating non-significant terms and interactions to establish a minimal model (Crawley, 2012). The significance of the explanatory variables was established using a likelihood ratio test (which is approximately distributed as a Chi-square distribution or an F test (Bolker, 2008). The significant Chi-square or F values given in the text are for the minimal model, whereas non-significant values correspond to those obtained before the deletion of the variable from the model. A posteriori contrasts were carried out by aggregating factor levels together and by testing the fit of the simplified model using a likelihood ratio test.
3 Results
Table 1 summarizes the objectives and the biological models used in each of the experiments.
3.1 Experiment 1: effect of artificial increase of corticosterone concentration in vertebrate blood on Plasmodium replication rate and transmission
Bird parasitemia decreased drastically between the acute infection stage (10 dpi) and the first blood sample of the latent infection stage (35 dpi, model 1: χ² = 61.09 p < 0.0001). Then, a significant increase in host parasitemia over time was observed during the latent infection period (from 35 to 75 dpi, model 2: χ² = 42.13 p < 0.0001, Figure 1A).
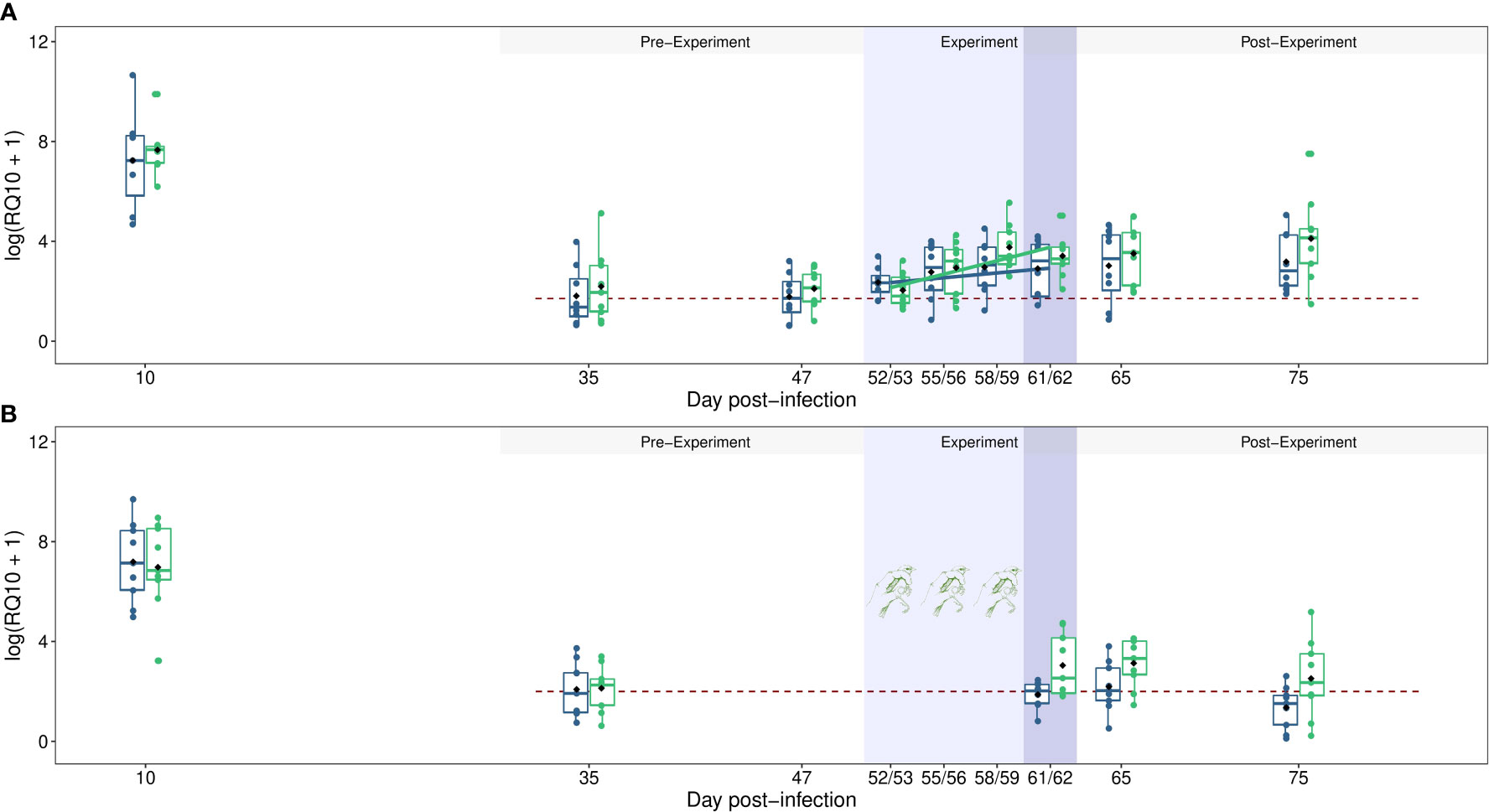
Figure 1 Dynamics of blood parasitemia (Log(RQ+1)) of Plasmodium relictum in vertebrate hosts (experiment 1 & 2). (A) Dynamics of parasitemia in control individuals (blue) or in individuals that ingested corticosterone (green) during the latent experiment stage (light blue areas, refer to Materials & Methods, 2.2 experiment 1 for details). (B) Dynamics of parasitemia in unstressed individuals (i.e. control, blue) or in individuals that were stressed by handling (green) during the latent experiment stage (light blue area, refer to Materials & Methods, 2.3 experiment 2 for details). The impact of bird treatment on the transmission rate of Plasmodium from vertebrate hosts to mosquitoes was explored on day 61/62 post-infection (dark blue area). The dashed red line indicates the average parasitemia measured during the chronic pre-experimentation stage. The colored dots correspond to the raw data and the black points represent the means.
We further divided the latent stage of infection into three different stages: the latent pre-experimental stage (35–47 dpi), the latent experimental stage (52–62 dpi) and the latent post-experimental stage (65–75 dpi). During the latent pre-experimental stage, parasitemia did not vary between the different blood sampling days (model 3, 35 and 47 dpi, χ² = 1.29, p = 0.255, Figure 1A) and was not different between control individuals and birds that would later ingest corticosterone (model 3, χ² = 0.65, p = 0.422). During the latent experimental stage, an interaction between dpi and bird treatment was observed (model 4, χ² = 3.95, p = 0.046, Figure 1A). While the parasitemia of the control individuals did not vary significantly over time (model 5, χ² = 1.98, p = 0.158), the parasite burden of CORT birds increased (model 6, χ² = 16.40, p < 0.0001). Then, during the post-experimental stage, parasitemia did not vary between the different blood sampling days (65 and 75 dpi, model 7, χ² = 0.96, p = 0. 326, Figure 1A) and was not different between control and CORT birds (model 7, χ² = 1.70, p = 0.191).
On the last day of the latent experimental stage (61/62 dpi), we evaluated the impact of the oral hormone treatment on Plasmodium transmission rate to mosquito vector. No effect of bird treatment was observed on mosquito blood meal size (mean ± s.e., CORT: 23.15 ng ± 8.26, control: 23.94 ng ± 8.07, model 8, χ²1 = 0.029 p = 0.865), mosquito infection prevalence (CORT: 0.66, 95% CI: 0.06, control: 0.53, 95% CI: 0.06, model 9, χ²1 = 1.140 p = 0.286) and oocyst burden (geometric mean ± s.d., CORT: 2.74 ± 2.40, control: 4.07 ± 3.11, model 10, χ²1 = 0.033 p = 0.857).
3.2 Experiment 2: effect of hosts’ stress induced by handling on Plasmodium replication rate and transmission
Bird parasitemia decreased drastically between the acute infection stage (10 dpi) and the first blood sample of the latent infection stage (35 dpi, model 11: χ² = 54.39 p < 0.0001). Then, a significant interaction between bird treatment and dpi was observed during the latent infection period (model 12: χ² = 4.92 p = 0.026, Figure 1B). While the parasitemia of control birds did not vary, the parasite burden of handling individuals increased over time (Figure 1B).
During the latent pre-experimental stage, parasitemia was not different between control individuals and birds in the “handling” group (model 13, W = 40, p = 0.99, Figure 1B). After the four days of bird manipulation (i.e. latent experimental stage), the parasitemia of handled individuals, measured day 61 or 62 post-infection, was significantly higher than the parasitemia of control birds (mean RQ10 ± s.e., control = 12.65 ± 4.62, handling = 39.54 ± 14.95, model 14, χ² = 5.32 p = 0.021, Figure 1B). This significant difference was maintained throughout the latent post-experiment stage (control = 8.4 ± 2.55, handling = 32.32 ± 9.82, model 15, χ² = 4.91 p = 0.027, Figure 1B), but a slight decrease of host parasitemia was observed according to blood sampling days (65 versus 75 dpi, model 15, χ² = 6.206, p = 0.013, Figure 1B).
As in the first experiment, on the last day of the latent experimental stage (61/62 dpi), no effect of bird treatment was observed on mosquito blood meal size (mean ± s.e., Control: 31.83 ng ± 9.04, Handling: 28.86 ng ± 9.98, model 16, χ²1 = 1.97 p = 0.160), infection prevalence (Control: 0.53, CI: 0.06, Handling: 0.55, CI: 0.07, model 17, χ²1 = 0.08 p = 0.772) and oocyst burden (geometric mean ± s.d., control: 3.87 ± 2.70, handling: 5.38 ± 3.24, model 18, χ²1 = 0.69 p = 0.407).
3.3 Experiment 3: effect of mosquito bites on host corticosterone concentration
The mean baseline corticosterone of birds was 2.50 ± 0.66 ng.mL−1 (mean ± s.e.). Corticosterone level increased significantly after the handling and isolation period in both “control” and “exposed” groups (model 19, time of blood sampling: χ² = 19.21, p < 0.0001, contrast analysis: unexposed χ² = 4.61, p = 0.032, exposed χ² = 21.41, p < 0.0001, Figure 2). The increase was however significantly higher in birds exposed to mosquito bites (significant interaction between bird treatment and time of blood sampling, model 19, χ² = 4.56, p = 0.032). On average, the corticosterone level increased by more than 7.5 times in the “exposed” group (first blood sample: 1.79 ng.mL−1 ± 0.31, second blood sample: 13.57 ng.mL−1 ± 2.57) and by 2.5 times in the “control” group (first blood sample: 3.30 ng.mL−1 ± 1.35, second blood sample: 8.28 ng.mL−1 ± 1.90).
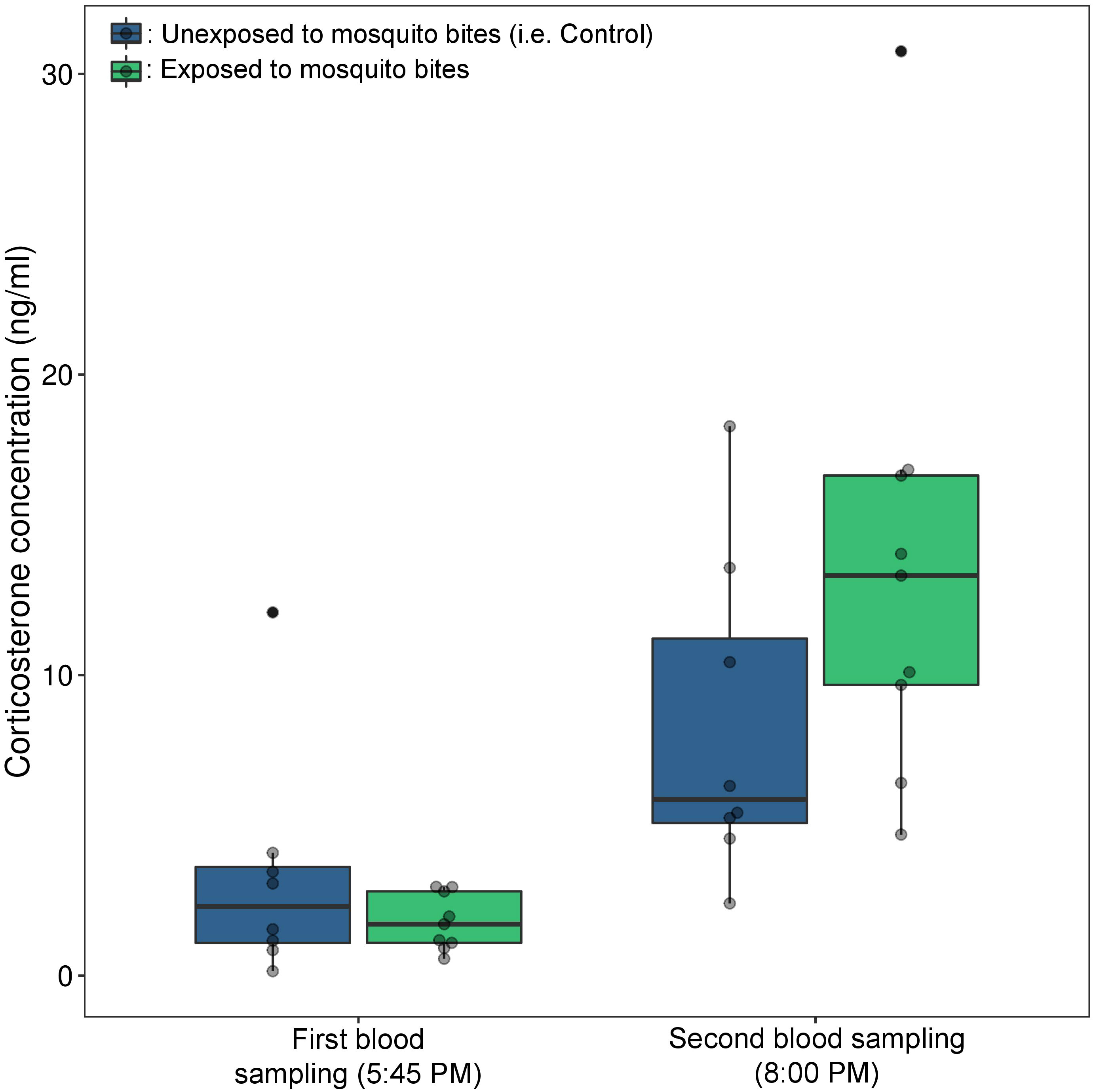
Figure 2 Baseline and stress induced corticosterone (ng/mL) in birds exposed or not to mosquito bites (experiment 3). Baseline corticosterone was measured from blood collected within 3 min of disturbance. Immediately after the blood sampling, all birds were immobilized and placed in individual cages. After one hour of acclimatization, mosquitoes were released in each cage containing an “exposed” bird (green boxplot). One hour later, a new blood samples were taken from the brachial vein of all birds to quantify corticosterone concentrations. The grey dots correspond to the raw data.
3.4 Experiment 4: effect of hosts’ stress on Plasmodium replication rate in semi-natural conditions
Three hundred and eight days after their infections (i.e., dpi), we exposed latently infected birds to a stressful event. Handling stress alone and handling stress following by exposure to mosquito bites influenced Plasmodium replication rate in house sparrows. We recorded a significant variation in parasitemia overtime after bird exposure to the stressful events (model 20, χ² = 4.29, p = 0.038, Figure 3). Peak parasitemia was reached approximately one week after bird manipulation and then decreased thereafter. Parasitemia in birds exposed to mosquito bites appeared to increase more strongly over time than parasitemia of birds exposed to handling stress only. However, we did not observe a significant interaction between bird treatment and dpi (model 20, χ² = 0.01, p = 0.927, Figure 3). The description and analysis of the overall infection dynamics is presented in Supplementary Material (Section 3 & Figure S2).
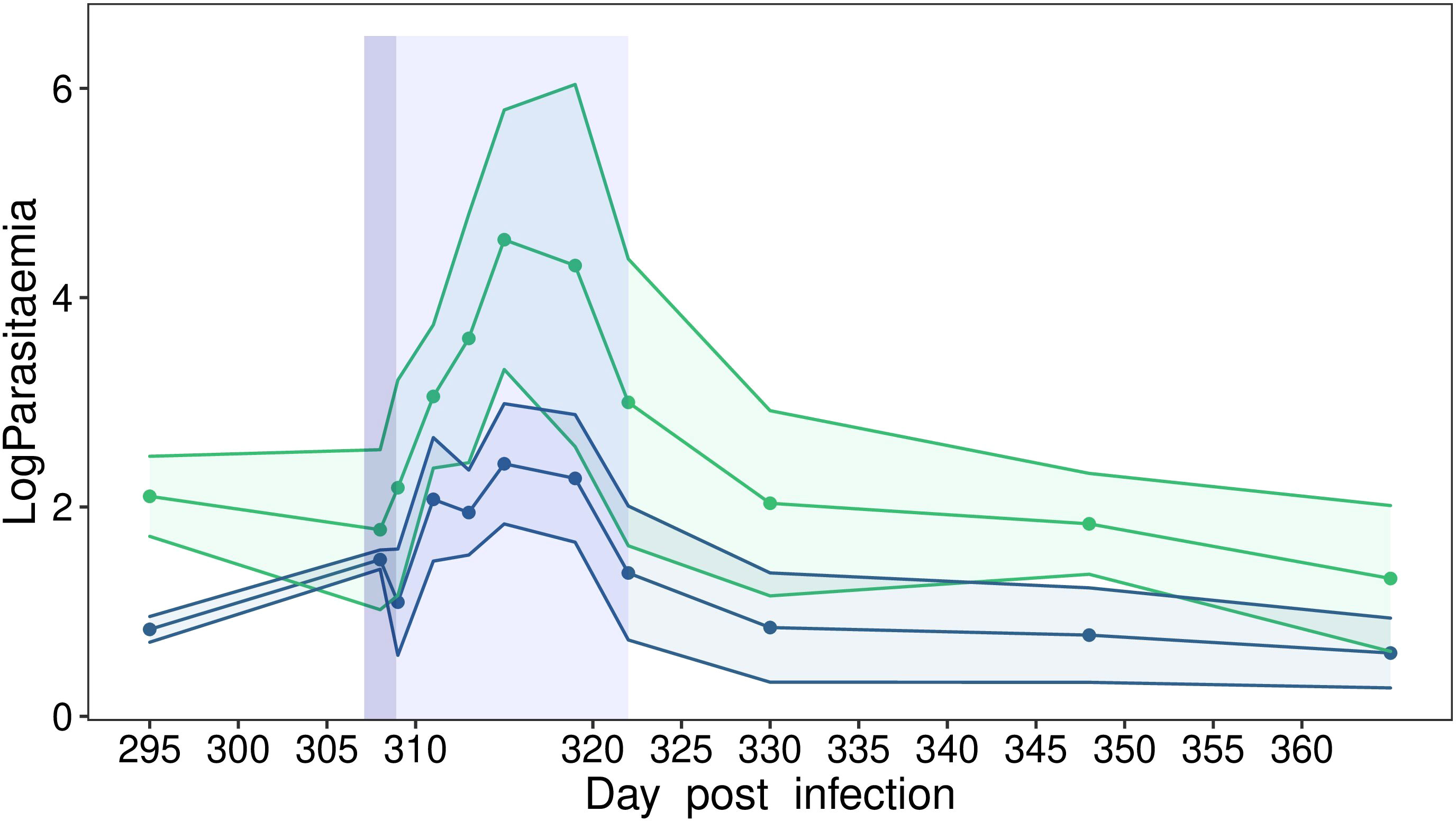
Figure 3 Dynamics of blood parasitemia (Log(RQ+1)) of Plasmodium relictum in wild house sparrows held in semi-natural conditions (experiment 4). Dynamics of parasitemia in chronically infected control individuals (i.e., stressed by handling, blue) or in chronically infected individuals that were stressed by handling and then exposed to mosquito bites (green) during the latent experiment stage. The dark blue area corresponds to the day when the birds were handled, isolated and, for some, exposed to mosquito bites. The light blue area corresponds to the period when the birds’ parasitemia was monitored every 2–3 days following their exposure to a stressful event.
4 Discussion
Understanding the mechanism(s) underlying the increase of Plasmodium replication rate in the host blood compartment during latent infection stage is essential for targeting and controlling malaria recurrences. Here we provide evidence that acute increased levels of stress-related hormones can trigger malaria recurrences. Our study also supports the hypothesis put forward by Dhondt and Dobson (2017) that the increase in parasitemia observed in hosts exposed to mosquitoes (Lawaly et al., 2012; Cornet et al., 2014b; Pigeault et al., 2018) could be partially mediated by mosquito bite-induced stress. However, while stress caused a long-lasting increase in parasite burden in the peripheral blood of chronically infected hosts, we surprisingly did not observe any effect on the transmission rate of the parasite.
Glucocorticoid hormones, such as cortisol or corticosterone, orchestrate numerous physiological and behavioral changes that allow animals to cope with acute stress events. The preeminent effects of short-term glucocorticoid augmentation are a rapid increase in circulating glucose concentrations in bloodstream (Kuo et al., 2015), a reinforcement of escape or avoidance behavior (Thaker et al., 2009; Wang et al., 2022) and a facilitation of classical conditioning and associative learning (Thaker et al., 2010). In the last decades, several studies have also examined the relationship between glucocorticoid concentration and immunity (Martin, 2009; Gao and Deviche, 2019). It has been generally shown that short-term increases in stress hormone levels influence some aspects of immunity with positive, but mostly negative effects depending on both the nature of the immune parameters and the animal species studied (e.g., Matson et al., 2006; Gao and Deviche, 2019; Tang et al., 2022). The impact of acute stress on the immune response of organisms can therefore ultimately affect the susceptibility of hosts to parasitic infection (Belden and Kiesecker, 2005; Gervasi et al., 2017; Martin et al., 2019). One approach to demonstrate the relationship between acute stress and susceptibility to pathogen infection is to artificially manipulate the concentration of corticosterone in the blood of hosts exposed to or infected by parasites. It has been shown that zebra finches infected by West Nile Virus and implanted with silastic tubules filled with corticosterone showed higher viremia than those observed in control birds (Gervasi et al., 2017; Martin et al., 2019). Tree frog tadpoles treated with exogenous corticosterone and infected by trematode (Alaria sp.) developed higher parasite loads than control tadpoles (Belden and Kiesecker, 2005). Here, our oral hormone treatment led to a significant increase in malaria parasite blood stage number over time compared to infection dynamics recorded in control individuals. This result is consistent with that obtained in an early study on house sparrows chronically infected by P. relictum, where intramuscular injection of corticosterone led to a higher proportion of infected erythrocytes than in the control group (40–200 times higher parasitemia, see Figure 3 in Applegate, 1970). The difference in effect size between this study and our result could be explained by the mode of corticosterone administration (oral versus intramuscular) or, more probably, by the amount of corticosterone injected. The dose per body mass used by Appelgate (10 µg/g) was indeed 10 time higher than that used here (1 µg/g).
During the latent experiment and post-experiment stages, birds which have ingested corticosterone but also control individuals showed a higher parasite burden compared to that measured during the latent pre-experimental stage (i.e. before exposure to the stressful event). This result strongly suggests that the stress induced by handling the birds was sufficient to underlie an increase of parasite replication rate. Indeed, all birds (i.e. control and CORT) were manipulated during the latent experimental stage and we demonstrated that handling and restraint induced a significant increase in bird plasma corticosterone levels. Our second experiment confirmed this observation by demonstrating that, unlike unhandled and unrestrained birds (i.e., unstressed), for which parasitemia remained constant throughout the latent infection stage, parasitemia of handled individuals increased significantly and durably over time. The experiment conducted under semi-natural conditions reinforce this observation. It is indeed possible to induce malaria recurrence by exposing host infected for over than 300 days to an acute stress. Bouts of Plasmodium replication rate were observed in both birds exposed to handling stress and to mosquito bites or to handling stress alone. However, contrary to our expectation we did not observe an additive effect of mosquito bite (Cornet et al., 2014b). Yet, we have shown that handling stress followed by exposure to mosquito bites induces a stronger stress response than handling stress alone and the result of our first experiment suggest that the intensity of malaria recurrence is related to the concentration of circulating corticosterone. The lack of significant difference between the two groups of house sparrows is probably due to the small sample size.
While natural and artificial increases in stress hormones impacted the replication rate of Plasmodium, we did not observe any effect on the transmission rate of the parasite to the mosquitoes. The parasite burden of birds that ingested corticosterone in the first experiment was relatively close to that measured in control birds on the day of mosquito exposure (i.e. 61/62 dpi, CORT: 41.39 ± 14.39, control: 29.02 ± 8.98), which can explain this result. However, in the second experiment, the parasitemia of the handled birds was on average more than six times higher than that of the control individuals. Considering the positive relationship reported between vertebrate host parasitemia and the transmission rate of Plasmodium relictum to Culex pipiens mosquitoes (Pigeault et al., 2015), we would expect to observe a higher oocyst burden in mosquitoes fed on stressed hosts. Nonetheless, we cannot exclude that, despite a large difference in parasitemia, the gametocyte (sexual transmissible stage of Plasmodium) burden was similar between the two groups of individuals. Unfortunately, we do not currently have the molecular tools to quantify the gametocyte burden in the blood of chronically infected hosts and thus test this prediction. Another possible explanation could be that the birds might have been exposed to the mosquitoes too late after the beginning of the stress period, and thus of the increase in Plasmodium’s investment in the production of sexual stages. Indeed, exposure to acute stress may initially negatively impact the innate immune response of vertebrate hosts (Gao and Deviche, 2019) which may allow Plasmodium to increase its replication rate and investment in gametocyte production. But the increase in parasite burden in the blood compartment will reactivate the adaptive immune response (Doolan et al., 2009) which can then negatively influence gametocyte infectivity (Drakeley et al., 1999; Mackinnon and Read, 2003) and therefore parasite transmission from vertebrate host to mosquito. Finally, the parasite strains used in our experiments may have evolved during lab passages, which were performed intraperitoneally (i.e., without mosquitoes), leading to the selection of variants which invest less in the production of transmissible stage (i.e., gametocytes) or which are less able to adjust their investment in the transmission in response to within-host conditions. Producing gametocytes is likely to be costly in a lab environment where transmission is carried out by injection of infected red blood cells. However, a serial passage analysis showed no effect of repeated passages by intraperitoneal injection on the investment of P. relictum in the production of transmissible stages (Pigeault et al., 2015).
To conclude, we identified stress as one of the triggers for avian malaria recurrences. We showed that both artificial and natural increase in host stress-related hormone levels boost P. relictum investment in its replication during the chronic stage of the vertebrate infection. It remains to be determined whether this phenomenon is, as suggested in the literature, mediated by the immunosuppressive effect of stress. However, the stress to which the birds were exposed during our experiments is probably much greater than the stressful events they encounter in natural conditions. It is likely that in the natural environment, malaria recurrences are due to cumulative effects of various factors that may weaken host immunity such as i) exposure to acute stress, ii) a decrease in the allocation of resources in immunity due to a strong investment in reproduction or iii) an infection by a new pathogen. Finally, we show that the handling of birds induces stress that can have strong consequences on the dynamics of the infection within the host and thus act as a confounding effect on the interpretation of the results of experimental studies conducted under laboratory conditions but also of the results of epidemiological surveillance in wildlife.
Data availability statement
The datasets presented in this study can be found in online repositories. The names of the repository/repositories and accession number(s) can be found below: https://figshare.com/s/099861f53eb25f9a4eb5.
Ethics statement
This study was approved by the Ethical Committee of the Vaud Canton veterinary authority. The Ethics Commission of the Veterinary Authority of the Canton of Vaud is the commission that issues authorizations for experiments performed in the Canton of Vaud and thus at the University of Lausanne. This commission is part of the federal food safety and veterinary office of Switzerland. Our authorization number is 1730.4. At the end of the experiment all individuals were treated to clear infection with a dose of commercially available Malarone, a mix of atovaquone/proguanil at a concentration of 1.08 mg in 40 μL.
Author contributions
RP, PC and OG designed the study. RP, AR, MB, JI and OG collected data. RP performed the statistical analyses. RP wrote the first draft of the manuscript with input from all authors. All authors contributed to the article and approved the submitted version.
Funding
This project was funded by the Swiss National Science Foundation (SNSF), grants 31003A_179378 to PC and CRSK-3_190197 to RP.
Acknowledgments
We would like to thank the two reviewers for helpful and constructive comments.
Conflict of interest
The authors declare that the research was conducted in the absence of any commercial or financial relationships that could be construed as a potential conflict of interest.
Publisher’s note
All claims expressed in this article are solely those of the authors and do not necessarily represent those of their affiliated organizations, or those of the publisher, the editors and the reviewers. Any product that may be evaluated in this article, or claim that may be made by its manufacturer, is not guaranteed or endorsed by the publisher.
Supplementary material
The Supplementary Material for this article can be found online at: https://www.frontiersin.org/articles/10.3389/fevo.2023.1191664/full#supplementary-material
References
Angrisano F., Robinson L. J. (2022). Plasmodium vivax – how hidden reservoirs hinder global malaria elimination. Parasitol. Int. 87, 102526. doi: 10.1016/j.parint.2021.102526
Applegate J. E. (1970). Population changes in latent avian malaria infections associated with season and corticosterone treatment. J. Parasitol. 56, 439–443. doi: 10.2307/3277599
Applegate J. E. (1971). Spring relapse of Plasmodium relictum infections in an experimental field population of English sparrows (Passer domesticus). J. Wild. Dis 7, 37–42. doi: 10.7589/0090-3558-7.1.37
Applegate J. E., Beaudoin R. L. (1970). Mechanism of spring relapse in qvian malaria: effect of gonadotropin and corticosterone. J. Wild. Dis 6, 443–447. doi: 10.7589/0090-3558-6.4.443
Ashley E. A., White N. J. (2014). The duration of Plasmodium falciparum infections. Malar. J. 13, 500. doi: 10.1186/1475-2875-13-500
Bates D., Mächler M., Bolker B., Walker S. (2015). Fitting linear mixed-effects models using lme4. J. Statis. Soft. 67 (1), 1–48. doi: 10.18637/jss.v067.i01
Battle K. E., Karhunen M. S., Bhatt S., Gething P. W., Howes R. E., Golding N., et al. (2014). Geographical variation in Plasmodium vivax relapse. Malar. J. 13, 144. doi: 10.1186/1475-2875-13-144
Becker D. J., Singh D., Pan Q., Montoure J. D., Talbott K. M., Wanamaker S. M., et al. (2020). Artificial light at night amplifies seasonal relapse of haemosporidian parasites in a widespread songbird. Proc. R. Soc B Biol. Sci. 287, 20201831. doi: 10.1098/rspb.2020.1831
Belden L. K., Kiesecker J. M. (2005). Glucocorticosteroid hormone treatment of larval treefrogs increases infection by alaria sp. trematode cercariae. J. Parasitol. 91, 686–688. doi: 10.1645/GE-397R
Betuela I., Rosanas-Urgell A., Kiniboro B., Stanisic D. I., Samol L., de Lazzari E., et al. (2012). Relapses contribute significantly to the risk of Plasmodium vivax infection and disease in Papua new guinean children 1–5 years of age. J. Infect. Dis. 206, 1771–1780. doi: 10.1093/infdis/jis580
Breuner C. W., Greenberg A. L., Wingfield J. C. (1998). Noninvasive corticosterone treatment rapidly increases activity in gambel’s white-crowned sparrows (Zonotrichia leucophrys gambelii). Gen. Comp. Endocrinol. 111, 386–394. doi: 10.1006/gcen.1998.7128
Cain D. W., Cidlowski J. A. (2017). Immune regulation by glucocorticoids. Nat. Rev. Immunol. 17, 233–247. doi: 10.1038/nri.2017.1
Christe P., Glaizot O., Strepparava N., Devevey G., Fumagalli L. (2011). Twofold cost of reproduction: an increase in parental effort leads to higher malarial parasitemia and to a decrease in resistance to oxidative stress. Proc. R. Soc. B. Biol. Sci, 279, 1142–1149. doi: 10.1098/rspb.2011.1546
Cockrem J. F. (2007). Stress, corticosterone responses and avian personalities. J. Ornithol. 148, 169–178. doi: 10.1007/s10336-007-0175-8
Cogswell F. B. (1992). The hypnozoite and relapse in primate malaria. Clin. Microbiol. Rev. 5, 26–35. doi: 10.1128/CMR.5.1.26
Colangeli R., Gupta A., Vinhas S. A., Chippada Venkata U. D., Kim S., Grady C., et al. (2020). Mycobacterium tuberculosis progresses through two phases of latent infection in humans. Nat. Commun. 11, 4870. doi: 10.1038/s41467-020-18699-9
Commons R. J., Simpson J. A., Watson J., White N. J., Price R. N. (2020). Estimating the proportion of Plasmodium vivax recurrences caused by relapse: a systematic review and meta-analysis. Am. J. Trop. Med. Hyg. 103, 1094–1099. doi: 10.4269/ajtmh.20-0186
Cornelius J. M., Zylberberg M., Breuner C. W., Gleiss A. C., Hahn T. P. (2014). Assessing the role of reproduction and stress in the spring emergence of hematozoan parasites in birds. J. Exp. Biol. 217, 841–849. doi: 10.1242/jeb.080697
Cornet S., Bichet C., Larcombe S., Faivre B., Sorci G. (2014a). Impact of host nutritional status on infection dynamics and parasite virulence in a bird-malaria system. J. Anim. Ecol. 83, 256–265. doi: 10.1111/1365-2656.12113
Cornet S., Nicot A., Rivero A., Gandon S. (2014b). Evolution of plastic transmission strategies in avian malaria. PloS Pathog. 10, e1004308. doi: 10.1371/journal.ppat.1004308
Cosgrove C. L., Wood M. J., Day K. P., Sheldon B. C. (2008). Seasonal variation in Plasmodium prevalence in a population of blue tits Cyanistes caeruleus. J. Anim. Ecol. 77, 540–548. doi: 10.1111/j.1365-2656.2008.01370.x
Dhondt A. A., Dobson A. P. (2017). Stress hormones bring birds, pathogens and mosquitoes together. Trends Parasitol. 33, 339–341. doi: 10.1016/j.pt.2017.01.001
Doolan D. L., Dobaño C., Baird J. K. (2009). Acquired immunity to malaria. Clin. Microbiol. Rev. 22, 13–36. doi: 10.1128/CMR.00025-08
Drakeley C. J., Secka I., Correa S., Greenwood B. M., Targett G. A. (1999). Host hematological factors influencing the transmission of Plasmodium falciparum gametocytes to Anopheles gambiae s.s. mosquitoes. Trop. Med. Int. Health 4, 131–138. doi: 10.1046/j.1365-3156.1999.00361.x
Eggermann J., Theuerkauf J., Pirga B., Milanowski A., Gula R. (2013). Stress-hormone levels of wolves in relation to breeding season, pack size, human activity, and prey density. Ann. Zool. Fenn. 50, 170–175. doi: 10.5735/086.050.0304
Fradejas I., Rubio J. M., Martín-Díaz A., Herrero-Martínez J. M., Ruiz-Giardin J. M., Rojo-Marcos G., et al. (2019). Prevalence of submicroscopic malaria infection in immigrants living in Spain. Malar. J. 18, 242. doi: 10.1186/s12936-019-2870-3
Gao S., Deviche P. J. (2019). The causative effects of corticosterone on innate immunity during the stress response in the house sparrow, Passer domesticus. gen. Comp. Endocrinol. 275, 30–37. doi: 10.1016/j.ygcen.2019.02.002
Gervasi S. S., Burgan S. C., Hofmeister E., Unnasch T. R., Martin L. B. (2017). Stress hormones predict a host superspreader phenotype in the West Nile virus system. Proc. R. Soc B Biol. Sci. 284, 20171090. doi: 10.1098/rspb.2017.1090
Hasker E., Kansal S., Malaviya P., Gidwani K., Picado A., Singh R. P., et al. (2013). Latent infection with Leishmania donovani in highly endemic villages in bihar, India. PloS Negl. Trop. Dis. 7, e2053. doi: 10.1371/journal.pntd.0002053
Hellgren O., Waldenström J., Bensch S. (2004). A new PCR assay for simultaneous studies of leucocytozoon, plasmodium, and Haemoproteus from avian blood. J. Parasitol. 90, 797–802. doi: 10.1645/GE-184R1
Huldén L., Huldén L. (2011). Activation of the hypnozoite: a part of plasmodium vivax life cycle and survival. Malar. J. 10, 90. doi: 10.1186/1475-2875-10-90
Ishtiaq F. (2021). Ecology and evolution of avian malaria: implications of land use changes and climate change on disease dynamics. J. Indian Inst. Sci. 101, 213–225. doi: 10.1007/s41745-021-00235-3
Kavaliers M., Colwell D. D. (1996). Synergism between stress responses induced by biting fles and predator odors. Ethology 102, 89–98. doi: 10.1111/j.1439-0310.1996.tb01106.x
Kuo T., McQueen A., Chen T.-C., Wang J.-C. (2015). Regulation of glucose homeostasis by glucocorticoids. Adv. Exp. Med. Biol. 872, 99–126. doi: 10.1007/978-1-4939-2895-8_5
Lalubin F., Delédevant A., Glaizot O., Christe P. (2013). Temporal changes in mosquito abundance (Culex pipiens), avian malaria prevalence and lineage composition. Parasites Vectors 6, 307. doi: 10.1186/1756-3305-6-307
Lawaly R., Konate L., Marrama L., Dia I., Diallo D., Sarr F. D., et al. (2012). Impact of mosquito bites on asexual parasite density and gametocyte prevalence in asymptomatic chronic Plasmodium falciparum infections and correlation with IgE and IgG titers. Infect. Immun. 80, 2240–2246. doi: 10.1128/IAI.06414-11
Mackinnon M. J., Read A. F. (2003). The effects of host immunity on virulence–transmissibility relationships in the rodent malaria parasite Plasmodium chabaudi. Parasitology 126, 103–112. doi: 10.1017/S003118200200272X
Markus M. B. (2018). Biological concepts in recurrent Plasmodium vivax malaria. Parasitology 145, 1765–1771. doi: 10.1017/S003118201800032X
Martin L. B. (2009). Stress and immunity in wild vertebrates: timing is everything. Gen. Comp. Endocrinol. 163, 70–76. doi: 10.1016/j.ygcen.2009.03.008
Martin L. B., Kernbach M. E., Unnasch T. R. (2019). Distinct effects of acute versus chronic corticosterone exposure on zebra finch responses to West Nile virus. Conserv. Physiol. 7, coz094. doi: 10.1093/conphys/coz094
Martínez-de la Puente J., Merino S., Tomás G., Moreno J., Morales J., Lobato E., et al. (2011). Nest ectoparasites increase physiological stress in breeding birds: an experiment. Naturwissenschaften 98, 99–106. doi: 10.1007/s00114-010-0746-z
Matson K. D., Tieleman B. I., Klasing K. C. (2006). Capture stress and the bactericidal competence of blood and plasma in five species of tropical birds. Physiol. Bioch. Zool. 79, 556–564. doi: 10.1086/501057
Names G. R., Schultz E. M., Krause J. S., Hahn T. P., Wingfield J. C., Heal M., et al. (2021). Stress in paradise: effects of elevated corticosterone on immunity and avian malaria resilience in a Hawaiian passerine. J. Exp. Biol. 224, jeb242951. doi: 10.1242/jeb.242951
Pearson R. D. (2002). Is prolactin responsible for avian, saurian, and mammalian relapse and periodicity of fever in malarial infections? Can. J. Zool. 80, 1313–1315. doi: 10.1139/z02-121
Pigeault R., Caudron Q., Nicot A., Rivero A., Gandon S. (2018). Timing malaria transmission with mosquito fluctuations. Evol. Lett. 2, 378–389. doi: 10.1002/evl3.61
Pigeault R., Vézilier J., Cornet S., Zélé F., Nicot A., Perret P., et al. (2015). Avian malaria: a new lease of life for an old experimental model to study the evolutionary ecology of Plasmodium. phil. Trans. R. Soc B 370, 20140300. doi: 10.1098/rstb.2014.0300
Popovici J., Pierce-Friedrich L., Kim S., Bin S., Run V., Lek D., et al. (2019). Recrudescence, reinfection, or relapse? a more rigorous framework to assess chloroquine efficacy for Plasmodium vivax malaria. J. Infect. Dis. 219, 315–322. doi: 10.1093/infdis/jiy484
Reedy A. M., Edwards A., Pendlebury C., Murdaugh L., Avery R., Seidenberg J., et al. (2014). An acute increase in the stress hormone corticosterone is associated with mating behavior in both male and female red-spotted newts, Notophthalmus viridescens. gen. Comp. Endocrinol. 208, 57–63. doi: 10.1016/j.ygcen.2014.08.008
Rivero A., Gandon S. (2018). Evolutionary ecology of avian malaria: past to present. Trends Parasitol. 34, 712-726. doi: 10.1016/j.pt.2018.06.002
Schall J. J. (1996). Malarial parasites of lizards: diversity and ecology. Adv. Parasitol. 37, 255–333. doi: 10.1016/s0065-308x(08)60222-5
Schoenle L. A., Moore I. T., Dudek A. M., Garcia E. B., Mays M., Haussmann M. F., et al. (2019). Exogenous glucocorticoids amplify the costs of infection by reducing resistance and tolerance, but effects are mitigated by co-infection. Proc. R. Soc B Biol. Sci. 286, 20182913. doi: 10.1098/rspb.2018.2913
Shanks G. D. (2015). Historical review: does stress provoke Plasmodium falciparum recrudescence? Trans. R. Soc Trop. 109, 360–365. doi: 10.1093/trstmh/trv032
Slowinski S. P., Geissler A. J., Gerlach N., Heidinger B. J., Ketterson E. D. (2022). The probability of being infected with haemosporidian parasites increases with host age but is not affected by experimental testosterone elevation in a wild songbird. J. Avian Biol. doi: 10.1111/jav.02819
Tang L., Cai N., Zhou Y., Liu Y., Hu J., Li Y., et al. (2022). Acute stress induces an inflammation dominated by innate immunity represented by neutrophils in mice. Front. Immunol. 13. doi: 10.3389/fimmu.2022.1014296
Taylor A. R., Watson J. A., Chu C. S., Puaprasert K., Duanguppama J., Day N. P. J., et al. (2019). Resolving the cause of recurrent Plasmodium vivax malaria probabilistically. Nat. Commun. 10, 5595. doi: 10.1038/s41467-019-13412-x
Thaker M., Lima S. L., Hews D. K. (2009). Acute corticosterone elevation enhances antipredator behaviors in male tree lizard morphs. Horm. Behav. 56, 51–57. doi: 10.1016/j.yhbeh.2009.02.009
Thaker M., Vanak A. T., Lima S. L., Hews D. K. (2010). Stress and aversive learning in a wild vertebrate: the role of corticosterone in mediating escape from a novel stressor. Am. Nat. 175, 50–60. doi: 10.1086/648558
Valkiunas G. (2004). Avian malaria parasites and other haemosporidia (London, New York, Washington: CRC Press. Boca Raton).
Vézilier J., Nicot A., Gandon S., Rivero A. (2010). Insecticide resistance and malaria transmission: infection rate and oocyst burden in Culex pipiens mosquitoes infected with Plasmodium relictum. Mal. J. 9, 379. doi: 10.1186/1475-2875-9-379
Wang B., Yang X., Lu J., Ntim M., Xia M., Kundu S., et al. (2022). Two-hour acute restraint stress facilitates escape behavior and learning outcomes through the activation of the Cdk5/GR p S211 pathway in male mice. Exp. Neurol. 354, 114023. doi: 10.1016/j.expneurol.2022.114023
White M. T., Shirreff G., Karl S., Ghani A. C., Mueller I. (2016). Variation in relapse frequency and the transmission potential of Plasmodium vivax malaria. Proc. R. Soc B Biol. Sci. 283, 20160048. doi: 10.1098/rspb.2016.0048
Wingfield J. C., Romero L. M. (2011). Adrenocortical responses to stress and their modulation in free-living vertebrates, in: comprehensive physiology. Am. Cancer Soc., 4, 211–234. doi: 10.1002/cphy.cp070411
Keywords: avian malaria, corticosterone, oocyst burden, Plasmodium, recrudescence, relapses, within-host infection dynamic
Citation: Pigeault R, Ruiz De Paz A, Baur M, Isaïa J, Glaizot O and Christe P (2023) Impact of host stress on the replication rate of Plasmodium: take it easy to avoid malaria recurrences. Front. Ecol. Evol. 11:1191664. doi: 10.3389/fevo.2023.1191664
Received: 22 March 2023; Accepted: 13 June 2023;
Published: 30 June 2023.
Edited by:
Ilaria Agostini, National Scientific and Technical Research Council (CONICET), ArgentinaReviewed by:
Carolina Romeiro Fernandes Chagas, Nature Research Centre, LithuaniaYukita Sato, Nihon University, Japan
Copyright © 2023 Pigeault, Ruiz De Paz, Baur, Isaïa, Glaizot and Christe. This is an open-access article distributed under the terms of the Creative Commons Attribution License (CC BY). The use, distribution or reproduction in other forums is permitted, provided the original author(s) and the copyright owner(s) are credited and that the original publication in this journal is cited, in accordance with accepted academic practice. No use, distribution or reproduction is permitted which does not comply with these terms.
*Correspondence: Romain Pigeault, romain.pigeault@univ-poitiers.fr
†ORCID: Romain Pigeault, orcid.org/0000-0002-8011-4600
Julie Isaïa, orcid.org/0000-0001-7737-9133
Olivier Glaizot, orcid.org/0000-0001-9116-3355
Philippe Christe, orcid.org/0000-0002-8605-7002