- Plant Ecology and Nature Conservation, Institute of Biochemistry and Biology, University of Potsdam, Potsdam, Germany
Climate conditions severely impact the activity and, consequently, the fitness of wildlife species across the globe. Wildlife can respond to new climatic conditions, but the pace of human-induced change limits opportunities for adaptation or migration. Thus, how these changes affect behavior, movement patterns, and activity levels remains unclear. In this study, we investigate how extreme weather conditions affect the activity of European hares (Lepus europaeus) during their peak reproduction period. When hares must additionally invest energy in mating, prevailing against competitors, or lactating, we investigated their sensitivities to rising temperatures, wind speed, and humidity. To quantify their activity, we used the overall dynamic body acceleration (ODBA) calculated from tri-axial acceleration measurements of 33 GPS-collared hares. Our analysis revealed that temperature, humidity, and wind speed are important in explaining changes in activity, with a strong response for high temperatures above 25°C and the highest change in activity during temperature extremes of over 35°C during their inactive period. Further, we found a non-linear relationship between temperature and activity and an interaction of activity changes between day and night. Activity increased at higher temperatures during the inactive period (day) and decreased during the active period (night). This decrease was strongest during hot tropical nights. At a stage of life when mammals such as hares must substantially invest in reproduction, the sensitivity of females to extreme temperatures was particularly pronounced. Similarly, both sexes increased their activity at high humidity levels during the day and low wind speeds, irrespective of the time of day, while the effect of humidity was stronger for males. Our findings highlight the importance of understanding the complex relationships between extreme weather conditions and mammal behavior, critical for conservation and management. With ongoing climate change, extreme weather events such as heat waves and heavy rainfall are predicted to occur more often and last longer. These events will directly impact the fitness of hares and other wildlife species and hence the population dynamics of already declining populations across Europe.
1 Introduction
Global changes in climate and landscape structure can have severe ecological consequences for wildlife across organizational levels (genes, individuals, populations, communities, Møller, 2013; Lau and terHorst, 2020; Ruland and Jeschke, 2020; Theodoridis et al., 2021). Wildlife is capable of responding to new climatic conditions with local genetic adaptation, migration to more favorable conditions, or phenotypic plasticity (Walther et al., 2002; Hetem et al., 2014). However, human-induced changes in climate and landscape connectivity are rapid and severe, thereby limiting local genetic adaptation and opportunities for migration (Meester et al., 2018). Genetic adaptations are likely to fail in the case of wildlife species that have long generation times and low reproductive output (and therefore low population sizes), making it difficult to keep up with the pace of climate changes (Boutin and Lane, 2014; Hoffmann et al., 2017). With increasing habitat loss and ongoing landscape fragmentation, climate-induced migration and dispersal movements are often limited (Collingham and Huntley, 2000; Walther et al., 2002; Travis, 2003). In fragmented landscapes, phenotypic plasticity including changes in phenology, physiology, and behavior (Boutin and Lane, 2014; Hetem et al., 2014), is likely the most immediate response of wildlife to counter the negative effects of rapidly changing climatic conditions on their activity and energy budgets (Noonan et al., 2018). One of the most fragmented landscapes worldwide are agricultural landscapes in Europe where changes in climate affect the temperate seasonal weather conditions: summers tend to cover a more extended period accompanied by higher temperatures and less rainfall, and winters get shorter with more precipitation and increasing temperatures (Klein Tank et al., 2002; Ergon et al., 2018). In Nordic regions, the annual average temperature increased by more than 2°C since the middle of the 19th century, and a prolongation of the growing season by more than a month is predicted by the end of the 21st century (Ruosteenoja et al., 2011; Ruosteenoja et al., 2016). Increases in rainfall events and precipitation intensity have been observed for locations across northern and middle Europe (Widmann and Schär, 1997; Hanssen-Bauer and Førland, 1998; New et al., 2001). Further, wind speeds are predicted to increase slightly (Pryor et al., 2006) and extreme weather events like heat waves or heavy rain events are predicted to increase in frequency (Coumou and Rahmstorf, 2012; IPCC, 2014).
For wildlife, changing weather conditions due to climate change can strongly affect their activity, movement behavior, and energy expenditure, crucial for an individual’s fitness (Brown et al., 2004). Unfavorable conditions like cold and humid weather increase the energy investment necessary for the thermoregulation of homeothermic animals (Seltmann et al., 2009; Klüg-Baerwald et al., 2016; Lenis Sanin et al., 2016), and may enhance the development of pathogens, thereby possibly increasing the spread of diseases (Altizer et al., 2006; Rödel and Dekker, 2012) and the risk of predation (Mech et al., 1987; Rödel and Dekker, 2012). A large proportion of energy is required for movements to reach and explore resources for foraging, finding mates, or withstanding adverse situations that might lower survival probability (Alexander, 2006). Depending on the internal state of an individual (e.g., whether it is hungry or not) and the environmental conditions, movements vary in speed, acceleration, or maneuverability (Nathan et al., 2008; Spiegel et al., 2017). Hence, energy investments differ even between recurring behaviors (e.g., foraging, mating) (Börger et al., 2020; Shaw, 2020; Wilson et al., 2020) in relation to the context-specific environmental condition with the prevailing weather as an important factor. For example, red deer (Cervus elaphus) in Europe increase their home range sizes with rising temperatures during winter and vice versa during summer (Rivrud et al., 2010). In addition, behavior and activity adjustments have been shown for different species globally. Japanese macaques (Macaca fuscata) decrease their movement activity during rainfall (Hanya et al., 2018), and North American passerines adjust their daily movement distances depending on wind speed and temperature (Grubb, 1978). Also, unfavorable weather conditions like cold or wet conditions decreased activity and shifted the temporal peaks of activity in tropical bats (Appel et al., 2019). With changing weather conditions, the activity and, consequently, the energy expenditure of animals can change to adapt to favorable or adverse conditions (Vickery and Bider, 1981; Hanya et al., 2018; Noonan et al., 2018; Appel et al., 2019).
A widespread species in Europe and parts of Asia is the European hare (Lepus europaeus, Pallas, 1778) (Hackländer and Schai-Braun, 2019). Its range covers the temperate zones of Europe from the Mediterranean zones in the south to the cold zones in the north (Figure 1). The European hare, a common umbrella species in agricultural landscapes, can indicate threats to a variety of species in ecological communities (Schai-Braun et al., 2020). However, hare populations have declined for the last few decades, mainly attributed to the intensification of agriculture (Tapper and Barnes, 1986; Vaughan et al., 2003). In addition, seasonally unfavorable weather conditions are yet another reason for the decline, as energy demand inevitably increases and, if not met, can even cause mortalities (Tapper and Barnes, 1986). Hares, especially the leverets, experience higher mortality rates under wet and cold conditions (Hackländer et al., 2002; Smith et al., 2005; Van Wieren et al., 2006), while warm temperatures lead to longer breeding seasons and higher pregnancy rates (Hewson and Taylor, 1975). On the other hand, high temperatures can also increase the risk of spreading diseases (Smith et al., 2005), and, extremely high temperatures may exacerbate food shortages during drought periods (Baudach et al., 2021). Hares’ native habitat is steppe landscapes (Baudach et al., 2021), which are usually warm and dry. We, therefore, assume that rising temperatures are not necessarily disadvantageous for hares and that they adjust their activity accordingly. They do not have to spend as much energy on thermoregulation and can consequently invest more in increased activity or fighting off pathogens (Lenis Sanin et al., 2016). Conditions like high humidity, known for its adverse effects on leveret survival (Hackländer et al., 2002), and the cooling effect at high wind speeds, could be detrimental by further decreasing an individual’s body temperature. We assume that they will negatively affect hares’ activity, while the activity increases with lower humidity and lower wind speed. On the other hand, the cooling effect of wind at high temperatures can be beneficial and support thermoregulation.
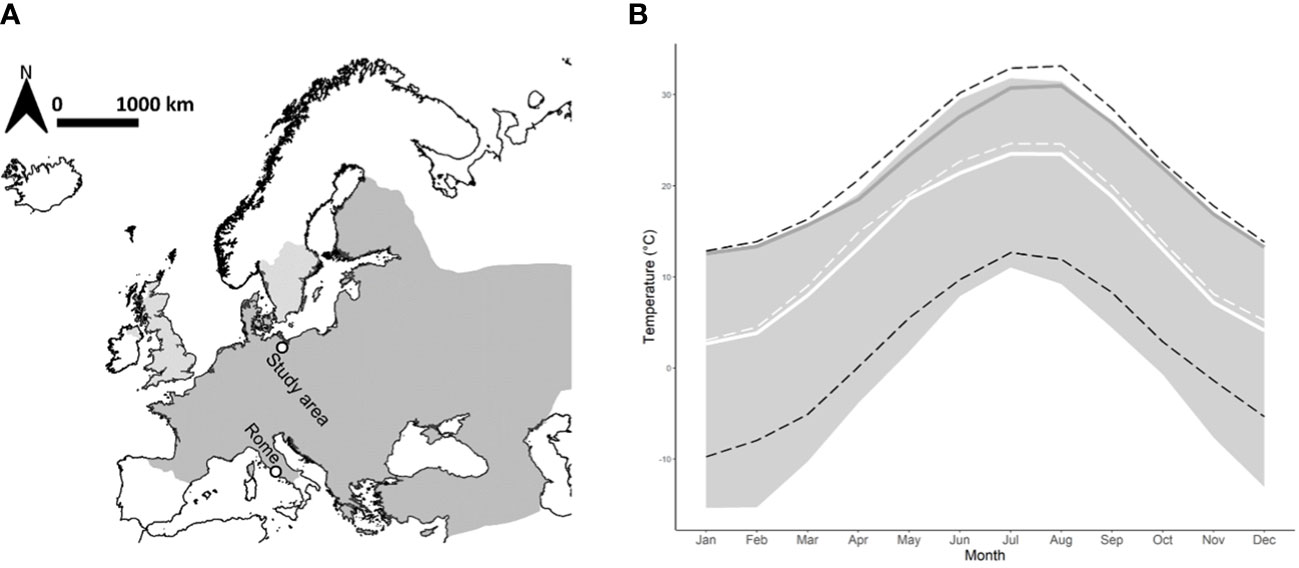
Figure 1 Distribution range of European hares (A) with the respective temperature range (B). (A) Native distribution area (dark grey) and areas where hares were introduced (light grey) (GBIF.org, 2023). Dots indicate the AgroScapelabQuillow study area north of Berlin (Germany) and Rome (Italy) as an example of the southern edge of the distribution range. (B) Temperature range within the distribution area (grey) calculated from monthly temperature values of 10 European cities, the AgroScapelabQuillow study area (solid white line), and Rome (dark grey line) between 1970 and 2010. The dashed lines indicate the warming of the last decade and show the mean temperatures of the study area (white dashed line) and the hottest and coldest places in the distribution area between 2010 and 2020.
In this study, we analyze how extreme weather conditions affect the activity of European hares in a central part of their distribution range. We were particularly interested in their sensitivities to rising temperatures, wind speed, and humidity during the reproduction period, when hares have to additionally invest energy in mating, prevailing against competitors (males), or lactating (females) (Lincoln, 1974). 1,237,176 acceleration measurements of 33 GPS-collared individuals were used to calculate overall dynamic body acceleration (ODBA), a proxy for hare activity (Wilson et al., 2006; Halsey et al., 2009). Changes in hare activity in response to ambient weather were analyzed separately for their active period at night and the resting period during the day. We hypothesize they react more strongly to extreme weather conditions during the active period at night, with males being more active than females due to larger home ranges and longer daily distances traveled (Rühe and Hohmann, 2004; Mayer et al., 2019). Further, since females must care for and feed their offspring irrespective of prevailing weather conditions, they are less capable of adapting their behavior accordingly. Thus, we expect sex-specific responses to extreme weather events.
2 Material and methods
2.1 Study area
The study area is located in an agricultural landscape 100 km northeast of Berlin (53°21’22 N, 13°48’03 E; WGS84) within the “AgroScapeLab Quillow” catchment, the research platform of the BioMove research training group and the Leibniz Centre for Agricultural Landscape Research (ZALF). The landscape is characterized by arable land with relatively large fields of an average size of 27.5 ± 1.1 ha, dominated by intensive cultivation of winter cereals, rapeseed, and maize (Ullmann et al., 2020).
Agricultural land covers approximately 74% of the area. The remaining area consists of patchy grasslands, several small water bodies, small forest areas, a few small villages, and single houses. The climate is described as continental-Atlantic transition with long dry phases in spring and cold winters. The mean annual precipitation is 486 mm, and the mean annual air temperature is 8.4°C (Ullmann et al., 2020; Stiegler et al., 2022).
2.2 Animal tracking data
We used accelerometer data of 33 hares (11 females, 22 males, all adults) recorded in 2014, 2015, 2019, and 2020 containing 1,237,176 measurements (Table S1). Individuals were tagged with e-obs GPS collars containing internal tri-axial accelerometers (model 1AA, 69g, e-obs GmbH, Germany) (Ullmann et al., 2018; Stiegler et al., 2022). Trapping, collaring, and data collection are described in detail in Rühe and Hohmann (2004), and Ullmann et al. (2018). Tri-axial acceleration bursts were recorded every four minutes in 2014 and 2015 (n = 20, Table S1), and every 2 minutes in 2019 and 2020 (n = 13, Table S1). Bursts were recorded at a frequency of 33 Hz for 3.3 sec (resulting in 110 acceleration samples per axis and burst). For each burst, we then calculated the overall dynamic body acceleration (ODBA) as a proxy for hare activity (Stiegler et al., 2022), using the R package moveACC (Scharf, 2018) with , where Ax, Ay, and Az are the derived dynamic accelerations corresponding to the three perpendicular axes of the sensor (Qasem et al., 2012). Activity data (ODBA values measured in g-force) were subsequently averaged over 10-minute intervals (i.e., 2 to 5 measurements) and merged with corresponding weather data. For our analysis, we only considered hares with more than 30 consecutive tracking days within the peak reproduction period (May–August, Table S1) (Hewson and Taylor, 1975) where approximately 75% of the annual leverets are born and nursed (Hansen, 1992).
2.3 Weather data
Weather data originated from a local (within 14 km of the collared hares) weather station (56 m a.s.l., 53°31’53 N, 13°93’38 E, WGS84) managed by the DWD (Deutscher Wetterdienst, opendata.dwd.de). Weather data contained 10-minute measurements of temperature (°C), precipitation (mm), relative humidity (%), and wind speed (m/s) over a period of 27 years (1993–2020).
2.4 Statistical analyses
All analyses were conducted in R version 4.0.3 (R Core Team, 2020) and R-Studio version 1.4.1103 (R Studio Team, 2021). We applied generalized additive models (GAM), with the R package mgcv, version 1.8-40 (Wood, 2011) to estimate the effects of weather conditions (temperature, wind speed, and humidity; Pearson correlation coefficient< 0.7) on the activity of hares (ODBA). As an extension to generalized linear models, GAMs use smooth functions to account for non-linear relationships between response and explanatory variables. Thus, a flexible description of the dependency between the response variable and predictors is possible (Wood, 2011). In our case, it is natural to expect a different movement behavior under extreme temperatures than under äverage” weather conditions. Therefore, a simple linear model would not explain as much of the variation of the ODBA as the GAM approach.
ODBA values were log-transformed in order to assume normality. The explanatory variables in the model were the three aforementioned weather variables for the combinations of the levels of sex (female/male) and time of the day (day/night), as well as sex and month as fixed effects. As random effects, we included hour of the day, day of the year, and individual id. The day (7 AM–6 PM) reflects the resting period with the lowest activity, and the night (6 PM–7 AM) reflects the time with higher activity (Figure 2). In addition, we included the pairwise combined effects of our weather variables as bivariate smooth functions to account for interaction effects.
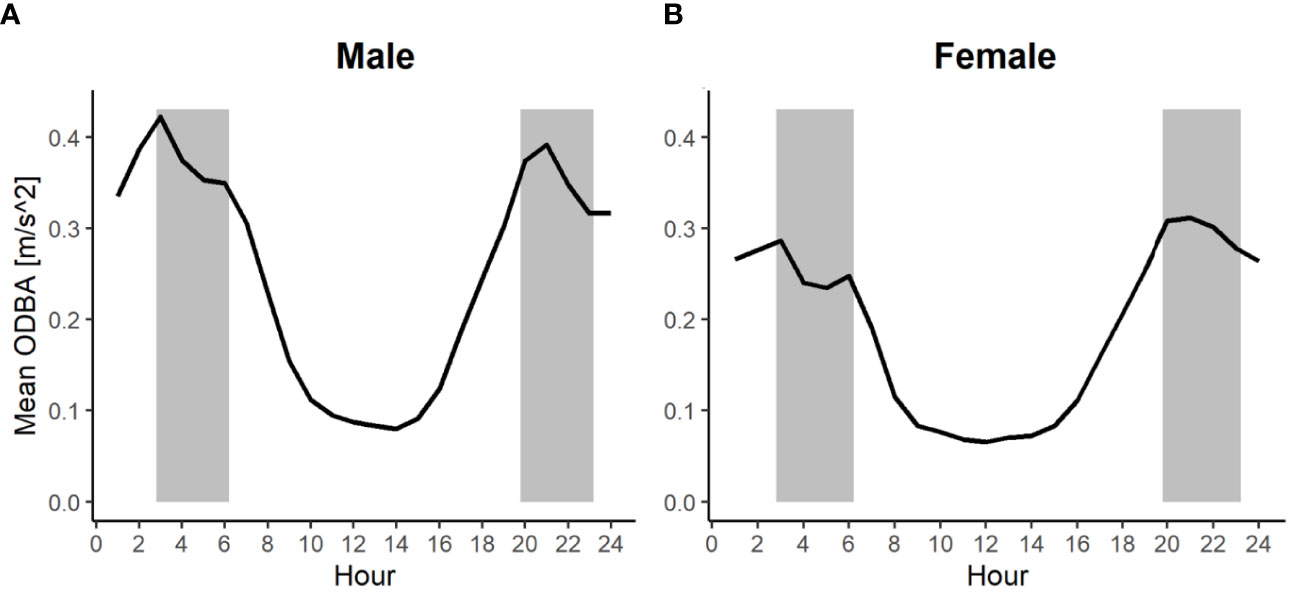
Figure 2 Change of hare activity over a day for males (A) and females (B). Shown is the mean hourly activity () for each hour of the day during the reproduction period (May–August) of males (n=22) and females (n=11). The grey areas indicate the approximate times of dawn and dusk (exact times depending on the time of the year).
We used our weather covariates in combination with the time of day and sex, respectively as smooth functions. This way, we could understand the effect of the weather variables more profoundly rather than understanding the overall effect of the weather variables on ODBA. As a smoothing basis, penalized splines were used for the weather variables to avoid overfitting. In addition, the hour of the day, the day of the year, and the individual ID were included as random effects since we expected them to influence ODBA. We used the lagged logarithmized ODBA as a smooth effect to respect the error terms independence assumption. In other words, we used the log(ODBA) at time t-1 to also explain the log(ODBA) at time t. Ignoring the independence assumption could lead to underestimating the uncertainty of the estimated effects and therefore provide false significant effects. Subsequently, we performed model selection (Appendix: Table S2) based on the deviance information criterion (AIC) using the dredge function implemented in the R package MuMIn (Bartoń, 2022), with the best-fit model including all variables. A possible pairwise correlation between the three continuous weather variables (10-min measurements) was tested and ruled out. Model validation was conducted with diagnostic residual plots and the ACF of the residuals. Thus, the estimated error terms were much less temporally autocorrelated as observed in the autocorrelation function [ACF, which displays how consecutive data points are correlated to each other in a time series (Turchin and Taylor, 1992)].
Our final model was specified as follows:
where id indicates the animal identifier and t the corresponding time point. In addition, s() indicates smooth functions. At last, u indicates the usage of random effects. As likelihood for the logarithmized ODBA, we assume a Gaussian distribution. The lagged logarithmized ODBA accounts for the temporal autocorrelation, which was clearly present for this kind of data.
3 Results
3.1 General activity
Based on our recollected data, hares were on average more active in the night than during the day . Thus, hares were on average 136% more active during the night (Figure 2). Highest activity peaked around dawn and dusk and was still comparatively high at night between dusk and dawn. In contrast, during the day between dawn and dusk, hares spent most of the time resting or doing other non-energy-demanding activities (e.g., grooming or moving slowly). The lowest activity was recorded around noon, between 11 AM and 2 PM (Figure 2) In addition, our data show that males were on average more active than females . This represents an average activity difference of 32% (Figure 2). This pattern was also supported by our model, which indicated that male individuals are likely to have a larger activity (coefficient = 0.15, p-value< 0.001) compared to females (Table 1).
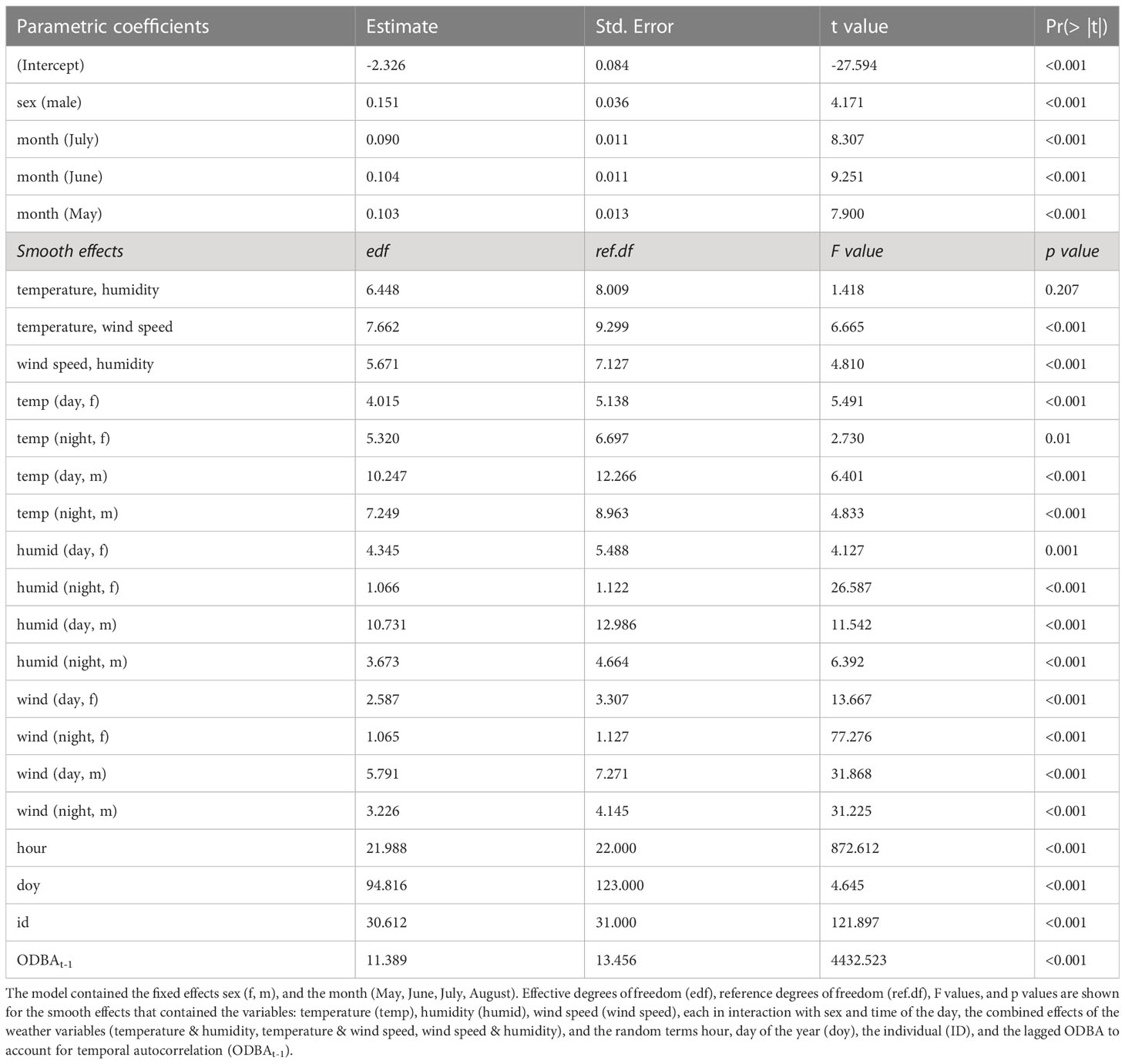
Table 1 Estimates (Est.), standard deviation (SD), t values, and p values are shown for the model’s fixed effects.
3.2 Impacts of weather on activity during peak reproduction period
While considering the interactions of sex and time of day; temperature, humidity, and wind speed influenced the activity of hares. Our model shows evidence that the ambient weather conditions likely affect their activity during the peak reproduction period (GAM R2 = 0.429, Table 1).
Based on our model, hares are likely to have the strongest reactions to extreme temperatures (Figure 3) compared to humidity and wind speed. We found a non-linear relationship between the temperature and activity of both males and females at day and nighttime. During the daytime, when hares mostly rest, both males and females increase activity at temperatures above 20°C, while females generally increase their activity with rising temperatures. For both sexes, the highest increment occurred during temperature extremes of over 35°C. In contrast, at night, when hares are usually active for foraging and other purposes, both sexes decrease activity at temperatures above 20°C (Figure 3). However, the absolute effect is larger for females than males during both night- and daytime temperatures. When warm temperatures (> 25°C) are accompanied by rather low wind speeds (< 3 m/s), activity is likely to decrease, while comparatively higher wind speeds combined with warmer temperatures result in increased activity of hare. When humidity is rather low (< 40%), our model indicates that hares are expected to increase their activity at particularly low (≤ 10°C) and high (> 35°C) temperatures (Figure S1).
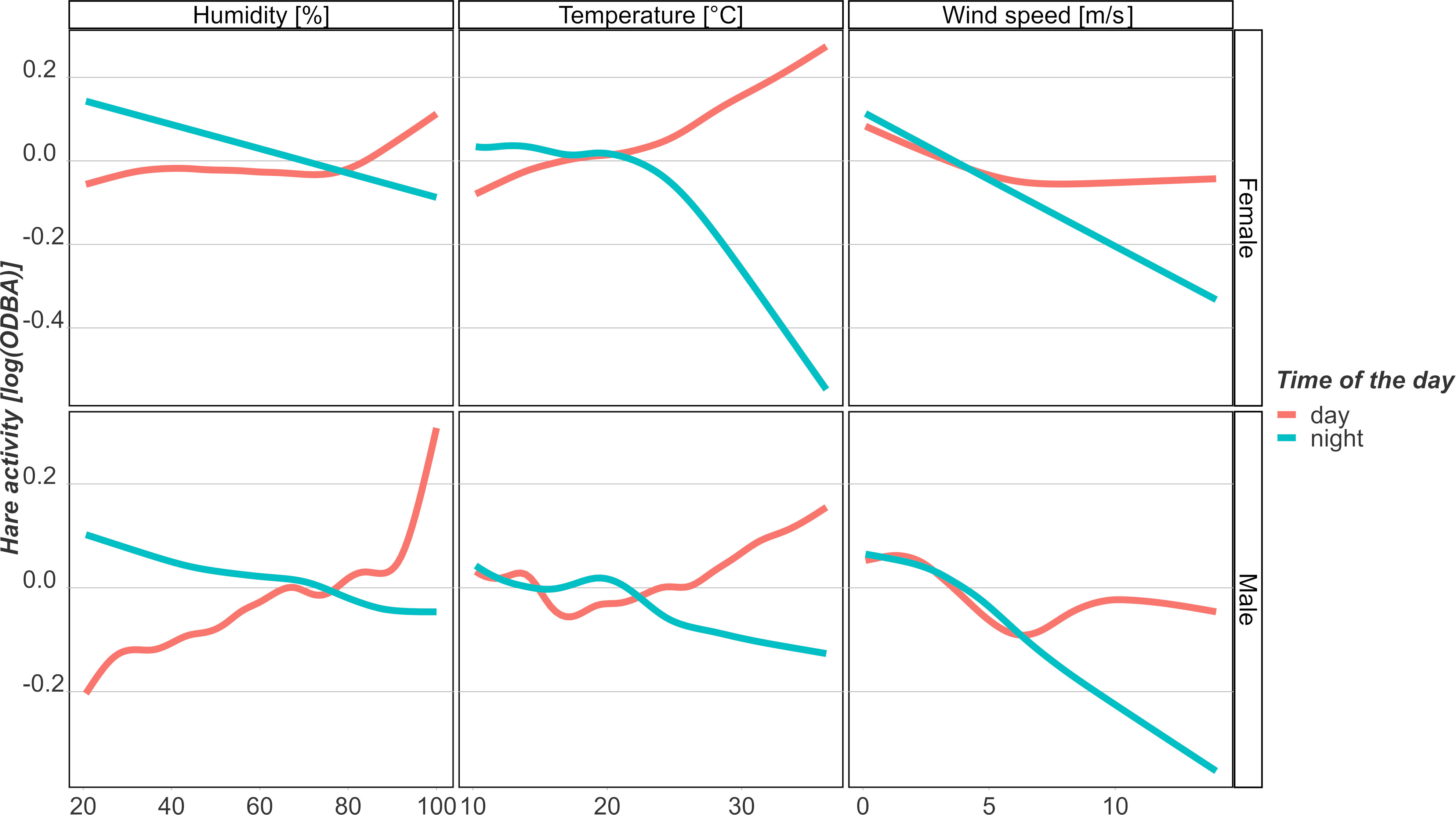
Figure 3 Impacts of temperature, humidity, and wind speed on changes in hare activity during peak reproduction period (May–August) for females (above) and males (below). Red lines represent activity changes for the resting period during the day, and blue lines for the active period during the night. Smooth plots show the average effects on the logarithmized ODBA of hares.
Our model indicates that the main effect of humidity on movement activity is stronger during the day (Figure 3). During this resting period, males are expected to increase their activity drastically compared to females when humidity is above 80%,especially in combination with higher wind speeds (> 8 m/s) (Figure S1). While the males decrease their activity towards lower humidity levels, females seem to remain more stable compared to males when humidity is below 40%. During the night in their active time, increasing humidity has a small negative effect on activity. This effect is similar for both sexes.
With increasing wind speeds, males and females are likely to decrease their activity similarly during both day and night (Figure 3), except for particularly high wind speeds accompanied by humidity values above 80% (Figure S1). The relationship between the activity of females and the wind speed during the night was almost negative linear (edf: night = 1.06, Table 1). In addition, males start to decrease their activity to wind speeds at approximately 3 m/s. This effect was negative up to a threshold of 6 m/s. Above the threshold, the effect on activity levels out during the day. At night, it still decreases above 6 m/s but more decelerated.
4 Discussion
Temperature, humidity, and wind speed were all important in explaining changes in the activity of hares, a widespread wildlife species occurring in a range of climatic zones across Europe and Asia. At temperatures above 20°C, hares decreased their activity during the night but showed an increase during the day. While tropical nights are still rare in Central Europe, hot daytime temperatures well above 25°C combined with high solar radiation occur frequently, and extremes in both are increasing due to climate change (IPCC, 2014). Such elevated temperatures, as well as high humidity and high wind speeds, were most important for immediate and distinct adjustments in activity during both the active period at night and the resting period during the day. However, we also found that high wind speeds have a regulating effect when temperatures are particularly hot, presumably supporting thermoregulation. While earlier studies suggest prevailing weather to have little influence on habitat selection and daily home range size of hares (Mayer et al., 2019), we show clear impacts of extreme weather conditions on hare activity during peak reproduction time. Activity adjustments were similar in both sexes, and males were, on average, more active than females corresponding to generally larger home ranges of males recorded in earlier studies (Rühe and Hohmann, 2004; Ullmann et al., 2018; Mayer et al., 2019). Remarkably, we observed contrary reactions in activity between day and night.
Many mammals cope with the effects of rising temperatures associated with climate change by seeking out cooler microclimates and shifting their activity towards more suitable times of the day (Berry et al., 2023; Hetem et al., 2012; McFarland et al., 2014). The decreasing activity of hares during tropical nights could, therefore, also be a mechanism to avoid overheating, as observed in many wildlife species (Hill, 2006; Rivrud et al., 2010; Lenis Sanin et al., 2016). For example, the subterranean rodent coruro (xtitSpalacopus cyanus) was found to fully adjust its activity rhythm to the ambient temperature by switching from diurnal to nocturnal behavior with high temperatures (Rezende et al., 2003). However, in the animal kingdom, there is considerable variation in the ability of endotherms to tolerate high environmental temperatures (Boyles et al., 2011); hence, the rationale for the behavior of the hares remains speculative. The increasing activity of hares during extremely warm daytime temperatures seems counterintuitive, yet high wind speeds also had an antagonistic effect, presumably by supporting thermoregulation. We explain this increase by the resting habits of hares. They usually rest in depressions with good visibility to detect and allow escape movements from potential predators (e.g., Baudach et al., 2021). Therefore, these critical microhabitats are often exposed to direct sunlight without any cover from protective vegetation. Extremely elevated temperatures during the resting period shift the trade-off between predator detection and prevention of overheating. On days with extremely hot temperatures and intense insolation, hares need to search and move to shady depressions with more favorable microclimatic conditions for resting (Lenis Sanin et al., 2016), possibly at the cost of a reduced predator detection capacity. Mayer et al. (2019) showed that the habitat selection of hares changed with increasing temperatures towards areas containing a vegetation height above 50 cm. That suggests that hares react to thermal stress by looking for shelter. Although we did not quantify how temperature affects hares’ reproduction success, earlier studies show that the litter size of hares is generally larger under warmer temperatures (Hewson and Taylor, 1975). However, from the observed responses in the activity of the adult hares, we speculate that extremely hot temperatures reduce the survival of leverets. While hares are adapted to warm temperatures, there might be a temperature threshold where it gets too hot for them. A model by Acevedo et al. (2012) shows, that L. europaeus as well as two other hare species native in Europe (L. timidus, L. granatensis) are likely to shift their ranges northward to escape increasing temperatures predicted by the IPCC A2 emission scenarios.
Wet conditions, such as extended rainfall or high humidity periods, reduce leveret survival. The higher energy expenditure for thermoregulation during wet conditions and higher susceptibility to infections through pathogens and parasites (Smith et al., 2005; Rödel and Dekker, 2012) will consequently lead to increased leveret mortality (Hackländer et al., 2002). Adult hares in our study slightly decreased activity with increasing humidity at night, indicating that hares try to avoid activities such as exploration movements to search for high-quality food sources when they get wet. Indeed, the thermal loss of wet fur through evaporation requires additional energy investments for thermoregulation (Jennings et al., 2006). Further thermal losses through convection when moving may explain the observed reduction in the activity of hares under humid conditions and may be an energy-saving mechanism. In contrast, hares increased their activity during the daytime resting period when air humidity exceeded 80%, with the latter possibly also being related to rain events. While wet fur leads to a higher energy consumption due to heat loss (Seltmann et al., 2009), hares might also have started moving to get dry by producing body heat. High humidity during the resting period may be better countered with movement than during the active periods at night because muscular work to produce body heat is costly (Terrien et al., 2011).
Wind can have a cooling effect by increasing heat dissipation from the body due to higher airflow. (Chappell, 1980). Hence, hares slightly reduced their activity in response to high wind speeds. The reduction was observed in both sexes and was slightly more pronounced during the active period at night. During the day, the small but distinct depressions in the ground used for resting most probably already provided a protective wind shelter. A decrease in activity with increasing wind speeds has been observed for many different wildlife species (e.g., the red squirrel (Tamiasciurus hudsonicus), or the alpine chamois (Rupicapra rupicapra) (Williams et al., 2014; Brivio et al., 2016). Wind affects an animal’s visual, olfactory, and auditory senses (Ruzicka and Conover, 2012; Cherry and Barton, 2017), which often influences the detection probability of predators by their prey and vice versa. For prey species like hares, the best strategy with increasing wind speeds is to hide rather than move around and increase the probability of encountering predators. Terrestrial predators like red foxes (Vulpes vulpes) or raccoons (Procyon lotor) are most active with moderate wind speeds that provide the optimal conditions to detect prey’s odor and decrease their activity with low and high wind speeds (Ruzicka and Conover, 2011). Hence, with increasing wind speeds, hares select shelters containing a low vegetation cover, enabling them to detect predators as early as possible. However, when high wind speeds were accompanied by particularly high temperatures, hares increased their activity, presumably due to the cooling effect of the wind.
4.1 Conservation challenges under extreme climate conditions and climate change
In the future, extreme weather periods, such as heat waves or extreme precipitation events witnessed during the recent summers in Europe, are predicted to occur more often and last longer with ongoing climate change (IPCC, 2014). Our study area is located in one of the driest regions of Germany, with the fewest days of precipitation and already high temperatures in summer. For those parts, more days with heavy rain, an increasing temperature in summer, and more heat days are predicted towards the middle of the 21st century (IPCC, 2014). In Europe, the study area is in the middle of the temperature range of the current hare distribution (Figure 1). where the temperature has significantly increased in the last decade (2010-2020) compared to 1970—2010. Although temperature is considered less important than precipitation for short-term hare population fluctuations (Rödel and Dekker, 2012), we found strong evidence that hares immediately change their activity when confronted with high-temperature extremes (decreases in activity during hot tropical nights; increase in activity during the resting period at day). Particularly, the substantial decrease in activity during the night is lost time for essential activities such as foraging or mating. Extended heat waves in summer with dry conditions will additionally affect hares by decreasing food availability (Baudach et al., 2021) or limiting reproductive output due to aggravated heat dissipation (Kearney et al., 2013). With less forage to find, hares may have to increase their daily activity to cover larger distances for finding necessary resources. Badgers (Meles meles) have been shown to adapt their activity to the optimum food intake by avoiding non-essential energy expenditure. Considering the scenario of increasing weather extremes, they are predicted to compensate for milder and drier conditions with behavioral plasticity, i.e., an increase in night activity (Noonan et al., 2018). While hares may also have the potential to encounter weather extremes with behavioral plasticity, there might be limitations. Especially females can be affected by food limitations and additional movements during the resting period on hot summer days when hares are forced to search for depressions with more suitable microclimatic conditions. Females must produce highly nutritious milk to satisfy the energy demands of the leverets.
The predicted increase in the number of days with heavy rainfall and the linked increase of extended conditions with high air humidity will likely additionally negatively affect the energy budgets of hares. Hares will have to adjust their activity under these conditions to save energy or get wet and presumably must invest more energy in thermoregulation. In both scenarios, either lost foraging time or foraging with higher energetic demands will directly affect the fitness of lactating females and the leverets (Hackländer et al., 2002; Rödel and Dekker, 2012), and hence population dynamics of many already threatened hare populations across Europe (Hackländer and Schai-Braun, 2019).
Data availability statement
The raw data supporting the conclusions of this article will be made available by the authors, without undue reservation.
Ethics statement
The animal study was reviewed and approved by the animal ethics committee of the University of Potsdam and by the local nature conservation authority (reference numbers: AZ 2347−6−2019, LUGV V3-2347-22-2013, and 55.2-1-54-2532-229-13), and in accordance with the German Federal Nature Conservation Act (§ 45 Abs. 7 Nr. 3).
Author contributions
NB and JS conceived the idea. WU and JS carried out most of the fieldwork, JP analyzed the data and contributed strongly to manuscript writing while RAG led the statistic approach. NB and JS led the revisions of the manuscript while NB supervised throughout the process. All authors contributed to the article and approved the submitted version.
Funding
Open Access funding enabled and organized by Projekt DEAL. This work was supported by the DFG-funded research training group “BioMove” (DFG−GRK 2118/1).
Acknowledgments
We thank all employees of the field station of the Leibniz Centre for Agricultural Research (ZALF) in Dedelow and all the helpers in the field that participated in hare catching and collaring for their support and commitment to the study. Further, we would like to thank two reviewers for their thorough comments and substantial improvements to the manuscript.
Conflict of interest
The authors declare that the research was conducted in the absence of any commercial or financial relationships that could be construed as a potential conflict of interest.
Publisher’s note
All claims expressed in this article are solely those of the authors and do not necessarily represent those of their affiliated organizations, or those of the publisher, the editors and the reviewers. Any product that may be evaluated in this article, or claim that may be made by its manufacturer, is not guaranteed or endorsed by the publisher.
Supplementary material
The Supplementary Material for this article can be found online at: https://www.frontiersin.org/articles/10.3389/fevo.2023.1193861/full#supplementary-material
References
Acevedo P., Jiménez-Valverde A., Melo-Ferreira J., Real R., Alves P. C. (2012). Parapatric species and the implications for climate change studies: a case study on hares in Europe. Global Change Biol. 18 (5), 1509–1519. doi: 10.1111/j.1365-2486.2012.02655.x
Alexander R. M. (2006). Principles of animal locomotion (Princeton, Oxford: Princeton University Press).
Altizer S., Dobson A., Hosseini P., Hudson P., Pascual M., Rohani P. (2006). Seasonality and the dynamics of infectious diseases. Ecol. Lett. 9 (4), 467–484. doi: 10.1111/j.1461-0248.2005.00879.x
Appel G., López-Baucells A., Magnusson W. E., Bobrowiec P. E. D. (2019). Temperature, rainfall, and moonlight intensity effects on activity of tropical insectivorous bats. J. Mammalogy 100 (6), 1889–1900. doi: 10.1093/jmammal/gyz140
Barton K. (2022). MuMIn: Multi-Model Inference. R package version 1.47.1. Available at: https://CRAN.R-project.org/package=MuMIn.
Baudach F., Greiser G., Martin I., Ponick W. (2021). “Status und entwicklung ausgewählter wildtierarten in deutschland. (Current state and development of selected wildlife species in Germany),” in Jahresbericht 2019. wildtier-informationssystem der länder (WILD) deutschlands (Berlin: Deutscher Jagdverband).
Berry P., Dammhahn M., Blaum N, and . (2023). Keeping cool on hot days: Activity responses of African antelope to heat extremes, Frontiers in Ecology and Evolution. 11. doi: 10.3389/fevo.2023.1172303
Börger L., Bijleveld A. I., Fayet A. L., Machovsky-Capuska G. E., Patrick S. C., Street G. M., et al. (2020). Biologging special feature. J. Anim. Ecol. 89 (1), 6–15. doi: 10.1111/1365-2656.13163
Boutin S., Lane J. E. (2014). Climate change and mammals: evolutionary versus plastic responses. Evolutionary Appl. 7 (1), 29–41. doi: 10.1111/eva.12121
Boyles J. G., Seebacher F., Smit B., McKechnie A. E. (2011). Adaptive thermoregulation in endotherms may alter responses to climate change. Integr. Comp. Biol. 51 (5), 676–690. doi: 10.1093/icb/icr053
Brivio F., Bertolucci C., Tettamanti F., Filli F., Apollonio M., Grignolio S. (2016). The weather dictates the rhythms: alpine chamois activity is well adapted to ecological conditions. Behav. Ecol. Sociobiology 70 (8), 1291–1304. doi: 10.1007/s00265-016-2137-8
Brown J. H., Gillooly J. F., Allen A. P., Savage V. M., West G. B. (2004). Toward a metabolic theory of ecology. Ecology 85 (7), 1771–1789. doi: 10.1890/03-9000
Chappell M. A. (1980). Thermal energetics and thermoregulatory costs of small arctic mammals. J. Mammalogy 2 (61), 278–291. doi: 10.2307/1380049
Cherry M. J., Barton B. T. (2017). Effects of wind on predator-prey interactions. Food Webs 13, 92–97. doi: 10.1016/j.fooweb.2017.02.005
Collingham Y. C., Huntley B. (2000). Impacts of habitat fragmentation and patch size upon migration rates. Ecol. Appl. 10 (1), 131–144. doi: 10.1890/1051-0761(2000)010[0131:IOHFAP]2.0.CO;2
Coumou D., Rahmstorf S. (2012). A decade of weather extremes. Nat. Climate Change 2 (7), 491–496. doi: 10.1038/nclimate1452
Ergon Å, Seddaiu G., Korhonen P., Virkajärvi P., Bellocchi G., Jørgensen M., et al. (2018). How can forage production in Nordic and Mediterranean Europe adapt to the challenges and opportunities arising from climate change? Eur. J. Agron. 92, 97–106. doi: 10.1016/j.eja.2017.09.016
Global Biodiversity Information Facility (GBIF) Lepus europaeus (GBIF Occurrence Download) (Accessed February 22, 2023).
Grubb T. C. (1978). Weather-dependent foraging rates of wintering woodland birds. Auk 95, 370–376. doi: 10.2307/4085455
Hackländer K., Arnold W., Ruf T. (2002). Lepus europaeus. The IUCN Red List of ThreatenedSpecies 2019. J. Comp. Physiol. B 172 (2), 183–190. doi: 10.1007/s00360-001-0243-y
Hackländer K., Schai-Braun S. (2019). The IUCN red list of threatened species 2019 (Lepus europaeus). doi: 10.2305/IUCN.UK.2019-1.RLTS.T41280A45187424.en
Halsey L. G., Shepard E. L. C., Quintana F., Gomez Laich A., Green J. A., Wilson R. P. (2009). The relationship between oxygen consumption and body acceleration in a range of species. Comp. Biochem. Physiol. Part A Mol. Integr. Physiol. 152 (2), 197–202. doi: 10.1016/j.cbpa.2008.09.021
Hansen K. (1992). Reproduction in European hare in a Danish farmland. Acta Theriologica 37 (1-2), 27–40. doi: 10.4098/AT.arch.92-3
Hanssen-Bauer I., Førland E. J. (1998). Long-term trends in precipitation and temperature in the Norwegian Arctic: can they be explained by changes in atmospheric circulation patterns? Climate Res. 10, 143–153. doi: 10.3354/cr010143
Hanya G., Otani Y., Hongo S., Honda T., Okamura H., Higo Y. (2018). Activity of wild Japanese macaques in yakushima revealed by camera trapping: patterns with respect to season, daily period and rainfall. PloS One 13 (1), e0190631. doi: 10.1371/journal.pone.0190631
Hetem R. S., Fuller A., Maloney S. K., Mitchell D. (2014). Responses of large mammals to climate change. Temperature (Austin Tex.) 2 (1), 115–127. doi: 10.4161/temp.29651
Hetem R. S., Strauss W. M., Fick L. G., Maloney S. K., Meyer L. C. R., Shobrak M., et al. (2012). Activity re-assignment and microclimate selection of free-living Arabian oryx: responses that could minimise the effects of climate change on homeostasis? Zoology (Jena Germany) 115 (6), 411–416. doi: 10.1016/j.zool.2012.04.005
Hewson R., Taylor M. (1975). Embryo counts and length of the breeding season in European hares in Scotland from 1960–1972. Acta Theriogenology 20 (19), 247–254.
Hill R. A. (2006). Thermal constraints on activity scheduling and habitat choice in baboons. Am. J. Phys. Anthropology 129 (2), 242–249. doi: 10.1002/ajpa.20264
Hoffmann A. A., Sgrò C. M., Kristensen T. N. (2017). Revisiting adaptive potential, population size, and conservation. Trends Ecol. Evol. 32 (7), 506–517. doi: 10.1016/j.tree.2017.03.012
IPCC (2014). “Climate change 2014: synthesis report,” in Contribution of working groups I, II and III to the fifth assessment report of the intergovernmental panel on climate change. Eds. Pachauri R. K., Meyer L. A. (Geneva, Switzerland: IPCC).
Jennings N., Smith R. K., Hackländer K., Harris S., White P. C. L. (2006). Variation in demography, condition and dietary quality of hares lepus europaeus from high-density and low density populations. Wildlife Biol. 12 (2), 179–189. doi: 10.2981/0909-6396(2006)12[179:VIDCAD]2.0.CO;2
Kearney M. R., Simpson S. J., Raubenheimer D., Kooijman S. A. L. M. (2013). Balancing heat, water and nutrients under environmental change: a thermodynamic niche framework. Funct. Ecol. 27, 950–966. doi: 10.1111/1365-2435.12020
Klein Tank A. M. G., Wijngaard J. B., Können G. P., Böhm R., Demarée G., Gocheva A., et al. (2002). Daily dataset of 20th-century surface air temperature and precipitation series for the European climate assessment. Int. J. Climatology 22 (12), 1441–1453. doi: 10.1002/joc.773
Klüg-Baerwald B. J., Gower L. E., Lausen C. L., Brigham R. M. (2016). Environmental correlates and energetics of winter flight by bats in southern Alberta, Canada. Can. J. Zoology 94, 829–836. doi: 10.1139/cjz-2016-0055
Lau J. A., terHorst C. P. (2020). Evolutionary responses to global change in species-rich communities. Ann. New York Acad. Sci. 1476 (1), 43–58. doi: 10.1111/nyas.14221
Lenis Sanin Y., Cabrera A. M. Z., Morales A. M. T. (2016). Adaptive responses to thermal stress in mammals. Rev. Medicina Veterinaria 31, 121–135.
Lincoln G. A. (1974). Reproduction and “March madness” in the brown hare, lepus europaeus. J. Zoology 174, 1–14. doi: 10.1111/j.1469-7998.1974.tb03140.x
Mayer M., Ullmann W., Heinrich R., Fischer C., Blaum N., Sunde P. (2019). Seasonal effects of habitat structure and weather on the habitat selection and home range size of a mammal in agricultural landscapes. Landscape Ecol. 34 (10), 2279–2294. doi: 10.1007/s10980-019-00878-9
McFarland R., Barrett L., Boner R., Freeman N. J., Henzi S. P. (2014). Behavioral flexibility of vervet monkeys in response to climatic and social variability. Am. J. Phys. Anthropology 154 (3), 357–364. doi: 10.1002/ajpa.22518
Mech L. D., McRoberts R. E., Peterson R. O., Page R. E. (1987). Relationship of deer and moose populations to previous winters’ snow. J. Anim. Ecol. 56 (2), 615–627. doi: 10.2307/5072
Meester L., Stoks R., Brans K. I. (2018). Genetic adaptation as a biological buffer against climate change: potential and limitations. Integr. Zoology 13 (4), 372–391. doi: 10.1111/1749-4877.12298
Møller A. P. (2013). Biological consequences of global change for birds. Integr. Zoology 8 (2), 136–144. doi: 10.1111/1749-4877.12006
Nathan R., Getz W. M., Revilla E., Holyoak M., Kadmon R., Saltz D., et al. (2008). A movement ecology paradigm for unifying organismal movement research. Proc. Natl. Acad. Sci. United States America 105 (49), 19052–19059. doi: 10.1073/pnas.0800375105
New M., Todd M., Hulme M., Jones P. (2001). Precipitation measurements and trends in the twentieth century. Int. J. Climatology 21 (15), 1889–1922. doi: 10.1002/joc.680
Noonan M. J., Newman C., Markham A., Bilham K., Buesching C. D., Macdonald D. W. (2018). In situ behavioral plasticity as compensation for weather variability: implications for future climate change. Climatic Change 149 (3-4), 457–471. doi: 10.1007/s10584-018-2248-5
Pryor S. C., Schoof J. T., Barthelmie R. J. (2006). Winds of change?: projections of near-surface winds under climate change scenarios. Geophysical Res. Lett. 33 (11), 1–5. doi: 10.1029/2006GL026000
Qasem L., Cardew A., Wilson A., Griffiths I., Halsey L. G., Shepard E. L. C., et al. (2012). Tri-axial dynamic acceleration as a proxy for animal energy expenditure; should we be summing values or calculating the vector? PloS One 7 (2), e31187. doi: 10.1371/journal.pone.0031187
R Core Team (2020). R: a language and environment for statistical computing. (Vienna, Austria: R Foundation for Statistical Computing). Available at: https://www.r-project.org/.
Rezende E. L., Cortés A., Bacigalupe L. D., Nespolo R. F., Bozinovic F. (2003). Ambient temperature limits above-ground activity of the subterranean rodent spalacopus cyanus. J. Arid Environments 55 (1), 63–74. doi: 10.1016/S0140-1963(02)00259-8
Rivrud I. M., Loe L. E., Mysterud A. (2010). How does local weather predict red deer home range size at different temporal scales? J. Anim. Ecol. 79 (6), 1280–1295. doi: 10.1111/j.1365-2656.2010.01731.x
Rödel H. G., Dekker J. J. A. (2012). Influence of weather factors on population dynamics of two lagomorph species based on hunting bag records. Eur. J. Wildlife Res. 58 (6), 923–932. doi: 10.1007/s10344-012-0635-1
R Studio Team (2021). RStudio: integrated development environment for r (PBC, Boston, MA: RStudio). Available at: https://posit.co/products/open-source/rstudio/.
Rühe F., Hohmann U. (2004). Seasonal locomotion and home-range characteristics of European hares (Lepus europaeus) in an arable region in central Germany. Eur. J. Wildlife Res. 50, 101–111. doi: 10.1007/s10344-004-0049-9
Ruland F., Jeschke J. M. (2020). How biological invasions affect animal behaviour: a global, cross-taxonomic analysis. J. Anim. Ecol. 89 (11), 2531–2541. doi: 10.1111/1365-2656.13306
Ruosteenoja K., Jylhä K., Kämäräinen M. (2016). Climate projections for Finland under the RCP forcing scenarios. Geophysica 1 (51), 17–50.
Ruosteenoja K., Räisänen J., Pirinen P. (2011). Projected changes in thermal seasons and the growing season in Finland. Int. J. Climatology 31 (10), 1473–1487. doi: 10.1002/joc.2171
Ruzicka R. E., Conover M. R. (2011). Influence of wind and humidity on foraging behavior of olfactory mesopredators. Can. Field-Naturalist 125 (2), 132. doi: 10.22621/cfn.v125i2.1196
Ruzicka R. E., Conover M. R. (2012). Does weather or site characteristics influence the ability of scavengers to locate food? Ethology 118 (2), 187–196. doi: 10.1111/j.1439-0310.2011.01997.x
Schai-Braun S. C., Ruf T., Klansek E., Arnold W., Hackländer K. (2020). Positive effects of set-asides on European hare (Lepus europaeus) populations: leverets benefit from an enhanced survival rate. Biol. Conserv. 244, 1–9. doi: 10.1016/j.biocon.2020.108518
Seltmann M. W., Ruf T., Rödel H. G. (2009). Effects of body mass and huddling on resting metabolic rates of post-weaned European rabbits under different simulated weather conditions. Funct. Ecol. 23 (6), 1070–1080. doi: 10.1111/j.1365-2435.2009.01581.x
Shaw A. K. (2020). Causes and consequences of individual variation in animal movement. Movement Ecol. 8, 12. doi: 10.1186/s40462-020-0197-x
Smith R. K., Jennings N. V., Harris S. (2005). A quantitative analysis of the abundance and demography of European hares lepus europaeus in relation to habitat type, intensity of agriculture and climate. Mammal Rev. 35 (1), 1–24. doi: 10.1111/j.1365-2907.2005.00057.x
Spiegel O., Leu S. T., Bull C. M., Sih A. (2017). What’s your move? movement as a link between personality and spatial dynamics in animal populations. Ecol. Lett. 20 (1), 3–18. doi: 10.1111/ele.12708
Stiegler J., Lins A., Dammhahn M., Eccard J., Kramer-Schadt S., Ortmann S., et al. (2022). Personality drives activity and space use in a mammalian herbivore. Movement Ecol. 10, 33. doi: 10.1186/s40462-022-00333-6
Tapper S. C., Barnes R. F. (1986). Influence of farming practice on the ecology of the brown hare (Lepus europaeus). J. Appl. Ecol. 23 (1), 39–52. doi: 10.2307/2403079
Terrien J., Perret M., Aujard F. (2011). Behavioral thermoregulation in mammals: a review. Front. Bioscience 16, 1428–1444. doi: 10.2741/3797
Theodoridis S., Rahbek C., Nogues-Bravo D. (2021). Exposure of mammal genetic diversity to mid-21st century global change. Ecography 44 (6), 817–831. doi: 10.1111/ecog.05588
Travis J. M. J. (2003). Climate change and habitat destruction: a deadly anthropogenic cocktail. Proc. R. Soc. B. Biol. Sci. 270 (1514), 467–473. doi: 10.1098/rspb.2002.2246
Turchin P., Taylor A. D. (1992). Complex dynamics in ecological time series. Ecology 1 (73), 289–305. doi: 10.2307/1938740
Ullmann W., Fischer C., Kramer-Schadt S., Pirhofer-Walzl K., Glemnitz M., Blaum N. (2020). How do agricultural practices affect the movement behaviour of European brown hares (Lepus europaeus)? Agriculture Ecosyst. Environ. 292, 106819. doi: 10.1016/j.agee.2020.106819
Ullmann W., Fischer C., Pirhofer-Walzl K., Kramer-Schadt S., Blaum N. (2018). Spatiotemporal variability in resources affects herbivore home range formation in structurally contrasting and unpredictable agricultural landscapes. Landscape Ecol. 33 (9), 1505–1517. doi: 10.1007/s10980-018-0676-2
Van Wieren S. E., Wiersma M., Prins H. H. (2006). Climatic factors affecting a brown hare (Lepus europaeus) population. Lutra 49 (2), 103–110.
Vaughan N., Lucas E.-A., Harris S., White P. C. L. (2003). Habitat associations of European hares lepus europaeus in England and Wales: implications for farmland management. J. Appl. Ecol. 40 (1), 163–175. doi: 10.1046/j.1365-2664.2003.00784.x
Vickery W. L., Bider J. R. (1981). The influence of weather on rodent activity. J. Mammalogy 62 (1), 140–145. doi: 10.2307/1380484
Walther G.-R., Post E., Convey P., Menzel A., Parmesan C., Beebee T. J., et al. (2002). Ecological responses to recent climate change. Nature 416), 389–395. doi: 10.1038/416389a
Widmann M., Schär C. (1997). A principal component and long-term trend analysis of daily precipitation in Switzerland. Int. J. Climatology 17 (12), 1333–1356. doi: 10.1002/(SICI)1097-0088(199710)17:12<1333::AID-JOC108>3.0.CO;2-Q
Williams C. T., Wilsterman K., Kelley A. D., Breton A. R., Stark H., Humphries M. M., et al. (2014). Light loggers reveal weather-driven changes in the daily activity patterns of arboreal and semifossorial rodents. titJournal Mammalogy 95 (6), 1230–1239. doi: 10.1644/14-MAMM-A-062
Wilson R. P., Börger L., Holton M. D., Scantlebury D. M., Gómez-Laich A., Quintana F., et al. (2020). Estimates for energy expenditure in free-living animals using acceleration proxies: a reappraisal. J. Anim. Ecol. 89 (1), 161–172. doi: 10.1111/1365-2656.13040
Wilson R. P., White C. R., Quintana F., Halsey L. G., Liebsch N., Martin G. R., et al. (2006). Moving towards acceleration for estimates of activity-specific metabolic rate in free-living animals: the case of the cormorant. J. Anim. Ecol. 75 (5), 1081–1090. doi: 10.1111/j.1365-2656.2006.01127.x
Keywords: activity, ODBA, animal tracking, European hare, extreme weather events, climate change
Citation: Stiegler J, Pahl J, Guillen RA, Ullmann W and Blaum N (2023) The heat is on: impacts of rising temperature on the activity of a common European mammal. Front. Ecol. Evol. 11:1193861. doi: 10.3389/fevo.2023.1193861
Received: 25 March 2023; Accepted: 05 June 2023;
Published: 16 June 2023.
Edited by:
Stephen T. Trumbo, University of Connecticut, United StatesReviewed by:
Stephan T. Leu, University of Adelaide, AustraliaPaola Lorena Sassi, CONICET Mendoza, Argentina
Copyright © 2023 Stiegler, Pahl, Guillen, Ullmann and Blaum. This is an open-access article distributed under the terms of the Creative Commons Attribution License (CC BY). The use, distribution or reproduction in other forums is permitted, provided the original author(s) and the copyright owner(s) are credited and that the original publication in this journal is cited, in accordance with accepted academic practice. No use, distribution or reproduction is permitted which does not comply with these terms.
*Correspondence: Jonas Stiegler, c3RpZWdsZXJAdW5pLXBvdHNkYW0uZGU=