- 1Department of Geography and the Environment, Villanova University, Villanova, PA, United States
- 2School of Aquatic and Fishery Sciences, University of Washington, Seattle, WA, United States
- 3Department of Genome Sciences, University of Washington, Seattle, WA, United States
The persistence of coral reefs requires the survival of adult coral colonies and their continued sexual reproduction despite thermal stress. To assess the trophic pathway (i.e., autotrophy and/or heterotrophy) used to develop gametes following bleaching, we thermally stressed Montipora capitata for one month at a time when corals in Hawai’i typically experience elevated seawater temperatures. After six and nine months of recovery, we pulse-chased non-bleached and previously bleached colonies using a dual-label design to compare the allocation of carbon and nitrogen at significant stages of gamete development. Dissolved inorganic carbon- (DI13C) and nitrogen- (DI15N) labelled seawater or 13C- and 15N-labelled rotifers were used to assess the autotrophic and heterotrophic pathways, respectively. At multiple time points for up to two years later, we collected adult coral fragments and isolated host tissue, Symbiodiniaceae cells, and developing eggs and captured gamete bundles to analyze their carbon (δ13C) and nitrogen (δ15N) stable isotopes. We found that the presence of Symbiodiniaceae was important for gametogenesis in both non-bleached and previously bleached colonies in two main ways. First, autotrophically-acquired carbon and nitrogen were both allocated to gametes during development, suggesting that recovery of photosynthesis after bleaching is critical for gametogenesis. Second, only heterotrophically-acquired nitrogen, not carbon, was incorporated into gametes and was readily recycled between host tissues and Symbiodiniaceae cells. This suggests that one of the purposes of heterotrophy following coral bleaching for M. capitata may be to supplement the nitrogen pool, providing available nutrients for endosymbiotic algal growth. Allocation of carbon and nitrogen to eggs coincided with the period when vertical transmission of symbionts to gametes occurs, further supporting the important relationship between gametogenesis and availability of Symbiodiniaceae for M. capitata.
1 Introduction
Coral reefs are currently threatened by increasing sea surface temperatures, resulting in an increased frequency and intensity of coral bleaching events (Hughes et al., 2017; van Woesik et al., 2022). These are known to negatively impact coral physiology (Coles et al., 2018), and consequently, are hypothesized to limit species persistence and the potential for species adaptation (Torda et al., 2017). The persistence of coral reefs requires both the survival of adult corals and the successful establishment of new colonies through sexual reproduction. We have recently reported that coral adults can allocate carbon to their gametes even when the adults have previously bleached (Rodrigues and Padilla-Gamiño, 2022). However, the cycle of gametogenesis takes several months (Padilla-Gamiño et al., 2014; Randall et al., 2020) and gamete development can overlap with periods of elevated sea surface temperatures (Bahr et al., 2017; van Woesik et al., 2022). Understanding how coral bleaching affects a full cycle of gamete development is vital in predicting both the likelihood of successful sexual reproduction under changing climatic conditions, the effectiveness of reef restoration efforts, and the long-term future of coral reefs.
Reef-building corals and their endosymbiotic dinoflagellate algae (or Symbiodiniaceae) have a long evolutionary history that has resulted in the wide diversity of modern coral assemblages (LaJeunesse et al., 2018). This co-evolution has resulted in mutually beneficial physiological and metabolic characteristics, including the sharing of acquired nutritional resources in the form of carbon and nitrogen (Stanley and van de Schootbrugge, 2009) supplied by trophic strategies ranging from autotrophic, mixotrophic, or heterotrophic (Conti-Jerpe et al., 2020). There is a growing body of literature that supports the recycling of nutrients by partners within the coral-algal holobiont and emphasizes the co-evolution of both organisms (Conti-Jerpe et al., 2020; Martinez et al., 2022). The delicate symbiosis can be disrupted with thermal stress that results in coral bleaching and is known to alter the trophic strategy relied upon for carbon acquisition in some species (Grottoli et al., 2006). How coral bleaching affects nutrient fluxes between partners for nitrogen acquisition and allocation of carbon and nitrogen for gametogenesis has not been previously studied.
Carbon is required for calcification and growth (Osinga et al., 2011), and maintenance of energy reserves, in particular lipids (Rodrigues and Grottoli, 2007) that are incorporated into gametes as a resource for offspring (Padilla-Gamiño et al., 2013). Sharing of carbon within healthy coral holobionts is well known; up to 100% of their daily energetic requirements can be met from autotrophy alone (Muscatine et al., 1981; Grottoli et al., 2006; Palardy et al., 2008). When light is available, autotrophic acquisition of carbon occurs quickly by Symbiodiniaceae (Battey and Patton, 1984; Kopp et al., 2015). Some carbon is retained for their growth and maintenance, while the remaining is translocated to the coral host, where it is often stored as lipids (Patton et al., 1983; Kopp et al., 2015; Krueger et al., 2018). Host lipids containing photosynthetic products can become depleted by more than 90% through time (Trench, 1971; Crossland et al., 1980; Kopp et al., 2015), indicating metabolism and/or translocation of lipid products to other coral compartments. Gamete bundles acquired carbon from adults immediately prior to spawning and are one of the compartments known to receive allocated carbon following autotrophy (Rodrigues and Padilla-Gamiño, 2022).
Heterotrophy is known to supplement carbon availability when autotrophy is limited as some coral species that host Symbiodiniaceae can acquire up to 46% of their daily metabolic carbon requirements from heterotrophy alone (Anthony and Fabricius, 2000; Palardy et al., 2008; Ferrier-Pagés et al., 2011). In addition, heterotrophy may be a mechanism for resilience by some species to cope with coral bleaching events (Grottoli et al., 2006; Hughes and Grottoli, 2013), accounting for up to 100% of the daily metabolic requirements of bleached corals (Houlbrèque and Ferrier-Pagès, 2009). While carbon from heterotrophy was directly acquired by bleached and non-bleached coral hosts, it was quickly translocated to and shared with their Symbiodiniaceae cells (Hughes et al., 2010). Thus, suggesting strong maintenance of the host-algal relationship in some species even when bleached. Enhanced heterotrophy has also been hypothesized to facilitate continued gametogenesis and sexual reproduction despite bleaching (Grottoli et al., 2006; Cox, 2007). However, initial findings suggest that at eight months after bleaching, heterotrophic carbon was not allocated to egg production (Rodrigues and Padilla-Gamiño, 2022).
Nitrogen is important for protein development and the growth and density of algal symbionts (Rädecker et al., 2015). Nitrogen is also often limiting in tropical coral reef ecosystems. Therefore, once acquired, it is retained and recycled within the holobiont, and excess nitrogen is excreted (Martinez et al., 2022). In its inorganic form it can be autotrophically acquired by Symbiodiniaceae, but over slightly longer time periods than carbon (Kopp et al., 2013; Kopp et al., 2015; Krueger et al., 2018). Like carbon, nitrogen is also translocated to the coral host, where it is predominantly stored in proteins (Trench, 1971; Kopp et al., 2013; Kopp et al., 2015; Krueger et al., 2018). Heterotrophically-acquired nitrogen was found in host tissue, Symbiodiniaceae, and lipids several hours after feeding, suggesting efficient sharing of nitrogen among the holobiont (Krueger et al., 2018). Similar to heterotrophically-acquired carbon, nitrogen from heterotrophy has been found to be important to the nutritional profile of Symbiondiniaceae, perhaps having a role in stress tolerance of the holobiont (Martinez et al., 2022). However, acquisition and allocation of nitrogen by bleached corals or for coral egg development has not yet been directly studied.
Here we determined the physiological pathway of autotrophically and heterotrophically acquired carbon and nitrogen through adult tissue compartments (Symbiodiniaceae and coral host), and ultimate translocation to developing eggs and gamete bundles in non-bleached and previously bleached Montipora capitata corals. Our experiment was designed to mimic the sequence, timing, and scale of naturally occurring bleaching events in Hawai’i (Bahr et al., 2017) and the stages of the gametogenic cycle of M. capitata (Padilla-Gamiño et al., 2014). We hypothesized that there would be differences in the acquisition of carbon and nitrogen to gametes from non-bleached and previously bleached adult colonies; the stage of gamete development would be an important predictor of acquisition of carbon and nitrogen to gametes; and both autotrophy and heterotrophy would reflect co-evolutionary strategies of recycling of carbon and nitrogen between endosymbiotic algae and host tissues.
2 Methods
2.1 Coral collection and experimental bleaching
Montipora capitata is a dominant Hawaiian reef-building coral that is a broadcast spawner with vertical transmission or direct inheritance of Symbiodiniaceae from parents to offspring. In August 2017, forty colonies of M. capitata were collected from the inner lagoon surrounding the Hawai’i Institute of Marine Biology (HIMB) in Kāne’ohe Bay, Hawai’i (21°26.18’N, 157°47.46’W). We collected corals on snorkel at depths up to 1 m. All colonies had a branching morphology, were not visibly bleached and appeared healthy, and were approximately 15-30 cm in diameter. Colonies were divided in half using a chisel and mallet to produce two genetically identical halves (80 total). These were transferred to eight outdoor flow-through seawater tanks (1.4 m x 1.8 m x 0.5 m) to induce bleaching. Complete details of the experimental bleaching are reported in (Axworthy et al., 2022). Briefly, one half of each colony was randomly assigned to one of four tanks that were maintained at the ambient seawater temperature (28°C) for 29 days. The other half of each colony was randomly assigned to one of four tanks with elevated seawater temperatures for the same 29-day period. Using aquarium heaters, we increased the temperature by 0.6°C per day over four days to reach an average maintained temperature of 30.4°C (Figure 1A). Each tank contained ten colony halves that were rotated in groups among all tanks of the same treatment each week to control for tank effects. After 29 days, corals from the increased temperature tanks were completely white or bleached (n=39, 2.5% mortality) and corals from the ambient tanks remained brown (n=39, 2.5% mortality). Dead colonies were observed without living tissue on their skeletons and were removed from further analyses. Bleaching status for both groups was confirmed at the end of the bleaching event with chlorophyll a concentrations and symbiont counts (Axworthy et al., 2022). All colonies were returned to racks near their original collection site to recover under ambient conditions until March or June 2018 (six or nine months after bleaching, respectively), when pulse-chase experiments were conducted (Figure 1).
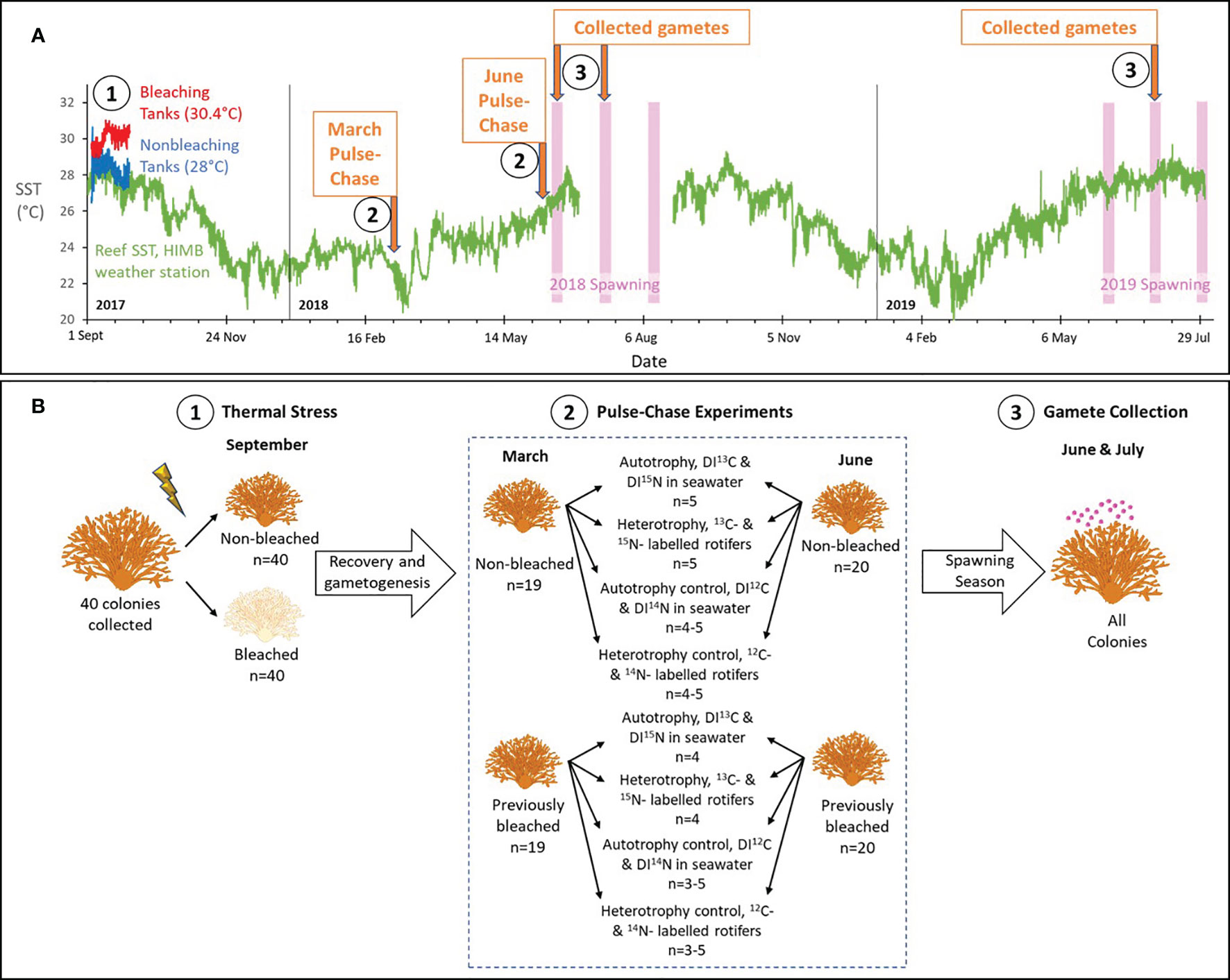
Figure 1 Experimental design. (A) The chronology of key events during the study from Sept 2017 through July 2019, including tank and sea surface temperature (SST) data, and the timing of predicted spawning for Montipora capitata and two pulse-chase experiments. Complete details are provided in the text. Briefly in Sept 2017, we experimentally bleached corals in tanks by raising the temperature to 30.4°C (red line), while non-bleached colonies were maintained in tanks at the ambient temperature of 28°C (blue line) (Axworthy et al., 2022). Available SST for a nearby reef from the weather station at the Hawai’i Institute of Marine Biology (HIMB) is shown in green. Predicted spawning for M. capitata is shown in the pink bars. Numbers in (A) correspond to key events depicted in more detail in (B), showing the design for thermal stress, pulse-chase experiments, and gamete collection. Briefly, (1) healthy colonies were collected, divided in half to produce genetically identical pairs, and one of the pairs was exposed to elevated seawater temperatures for 29 days to induce bleaching. The pairs recovered on the reef for 6-9 months. (2) In March and June, we pulse-chased colonies with 13C-labelled and 15N-labelled dissolved inorganic carbon and nitrogen (DI13C and DI15N) to assess autotrophy, 13C-labelled and 15N-labelled rotifers to assess heterotrophy, and controls for each using 12C and 14N labels. Sample sizes are shown. (3) We continued to follow the colonies for two spawning seasons and collected released gamete bundles from all colonies.
2.2 Pulse-chase experiments
Experiments occurred in two phases: a pulse phase, when artificially increased quantities of heavy or natural abundance (i.e., light) isotopes are introduced in a “pulse” or high quantity to allow for uptake by the coral, and a chase phase, when they are flushed to stop additional environmental uptake and to allow for the isotope to be “chased” or quantified through various coral tissue components. To compare the uptake of carbon and nitrogen at significant stages during egg development, we conducted pulse-chase experiments in March, when M. capitata eggs experience their most significant increase in growth, and in June, when eggs are fully developed and have obtained their endosymbiotic algae (Padilla-Gamiño and Gates, 2012; Padilla-Gamiño et al., 2014). Colonies were collected from racks and placed in flow-through seawater tanks in preparation for the pulse-chase experiments. Selected colonies had both bleached and non-bleached surviving halves and acclimated in tanks for at least 72 hours prior to experimentation. Both halves of one colony died prior to the start of the experiments, resulting in 19 colonies (or 38 halves) pulse-chased in March and 20 colonies (or 40 halves) pulse-chased in June (Figure 1B).
For the duration of each pulse-chase experiment colony halves were isolated in chambers (22 L each) as an independent experimental unit to avoid pseudoreplication. Isolation chambers were placed within flow-through seawater tanks maintained at the ambient temperature (22.45°C ± 0.6 in March, 25.89°C ± 0.5 in June) to control the temperature of the chambers. Each chamber was supplied with compressed air to provide water circulation and avoid anoxic conditions. Coral halves were acclimated in chambers for at least one hour prior to the start of each experiment. Within each pulse-chase month, colonies were randomly assigned to be labelled via the autotrophy or heterotrophy pathways, with nine colonies in March and ten colonies in June for each trophic pathway.
2.2.1 Autotrophy pulse phase
The autotrophy pulse phase began on 3 March 2018 and 4 June 2018. Experiments were conducted under natural light conditions approximately one hour after sunrise to maximize autotrophy. To minimize heterotrophy, each chamber was filled with 0.7 μm-filtered seawater. A total of five non-bleached and four previously bleached colonies in each month were dual treated with heavy carbon (13C) and heavy nitrogen (15N) stable isotopes during the 8-hour pulse phase (Figure 1B). We followed the concentrations used by Hughes et al. (2010). We used 0.117 M solutions each of 98 atom % 13C enriched NaHCO3 and 98 atom % 15N enriched NaNO3 to obtain final concentrations of dissolved inorganic carbon and nitrogen in each chamber of 0.000026 M (referred to as treatment samples). For the same 8-h pulse phase, control colonies (at least four non-bleached and three previously bleached colonies each month) were dual treated with light carbon (12C) and light nitrogen (14N) stable isotopes. We used 0.117 M solutions each of 12C NaHCO3 and 14N NaNO3 to obtain final concentrations of dissolved inorganic carbon and nitrogen in each chamber of 0.000026 M (referred to as control samples). Heavy and light isotope treatments were randomized in chambers within tanks to control for tank effects and to ensure that each chamber was a unique experimental unit. Paired previously bleached/non-bleached colony halves were assigned the same isotopic treatment to minimize genotype effects. During the 8-hour pulse phase, small 3-cm coral fragments were collected from each colony at 0, 1.5, 3, and 8 hours and frozen at -80°C for laboratory analyses.
2.2.2 Heterotrophy pulse phase
To prepare for the heterotrophy pulse-chase experiment, we cultured monocultures of naturally occurring Hawaiian phytoplankton (Nannochloropsis oculata) and zooplankton (Brachionus plicatilis) species. Four separate phytoplankton cultures were grown in sterile seawater containing 0.000021 M each of 13C (98 at. % 13C enriched NaHCO3), 15N (98 at. % 15N enriched NaNO3), 12C (NaHCO3), or 14N (NaNO3). Phytoplankton cultures were grown with full spectrum Light Emitting Diode (LED) grow lights and continuously supplied with air using a 1030 gallons per hour (GPH) aquarium air pump. Isotopic additions were made to each culture every 48 hours for four days, resulting in two dual-labeled phytoplankton cultures (Rodrigues and Padilla-Gamiño, 2022). Two separate zooplankton cultures were grown in sterile seawater and continuously supplied with air using two 126 GPH aquarium air pumps. The populations were diluted with sterile seawater every 48 hours to avoid population collapse. After phytoplankton cultures had been labeled for at least 24 hours, one zooplankton culture was fed with both 13C and 15N-labeled phytoplankton and the other was fed with both 12C and 14N-labeled phytoplankton at least every 48 hours for four days, resulting in two dual-labeled zooplankton cultures.
The heterotrophy pulse phase began on 6 March 2018 and 7 June 2018 one hour after sunset under natural dark conditions to maximize heterotrophy. To minimize heterotrophy from unknown sources, each chamber was filled with 0.7 μm-filtered seawater. 13C- and 15N-labeled zooplankton were added to isolation chambers, reaching a final concertation of 880 zooplankton per liter of seawater. At least five non-bleached colonies and four previously bleached colonies in each month were fed 13C- and 15N-labeled zooplankton for the 8-hour pulse phase, referred to as treatment samples (Figure 1B). Similarly, control colonies (at least four non-bleached and three previously bleached colonies each month) were fed 12C- and 14N-labeled zooplankton, referred to as control samples. Heavy- and light-labeled zooplankton treatments were randomized in chambers within tanks to control for tank effects and to ensure that each chamber was a unique experimental unit. Paired previously bleached/non-bleached colony halves were assigned the same isotopic treatment to minimize genotype effects. During the 8-hour pulse phase, small 3-cm coral fragments were collected at 0, 1.5, 3, and 8 hours and frozen at -80°C for laboratory analyses.
2.2.3 Autotrophy and heterotrophy chase phases
After eight hours of the pulse phase in both the autotrophy and heterotrophy experiments, the “chase” phase began when seawater within each chamber was replaced with fresh, unfiltered and unlabeled seawater. During the initial 72-hour period of the chase, seawater in chambers was refreshed every 12 hour in both autotrophy and heterotrophy experiments. In addition to the fragments collected during both pulse phases, small 3-cm coral fragments were collected at 24 and 72 hours during the chase phase and frozen at -80°C for laboratory analyses. For assessment of in situ developing eggs, additional fragments approximately 5-8 cm in length were collected at 8 and 72 hours and stored in 0.2 μm-filtered seawater with 1.85% formaldehyde. After the initial 72 hours of the pulse-chase experiments, colonies were returned to the reef until spawning was expected in summer 2018 and 2019.
2.3 Coral spawning
We collected coral gamete bundles (egg + sperm) during three subsequent spawning periods after the pulse-chase experiments from 12 to 17 June 2018, 7 to 16 July 2018, and 28 June to 5 July 2019 (Figure 1). All colonies that were pulse-chased in both March and June were isolated in chambers (22 L) within flow-through seawater tanks approximately one hour before sunset. They were monitored for the release of gamete bundles that were collected from the surface of the isolation chambers using pipettes, immediately frozen in liquid nitrogen, and stored at -80°C for laboratory analyses.
2.4 Laboratory analyses
Frozen coral fragments and gamete bundles were transported to Villanova University and stored at -80°C. In the laboratory, coral fragments were divided into three pieces of approximately 1 cm3 each for analyses. Different laboratory instruments and glassware were used for heavy and light isotope samples and were thoroughly cleaned between samples to avoid cross-contamination. The whole tissue was removed from the skeleton with an airbrush and the resulting tissue slurry was separated into symbiont cells and host tissue with a tissue grinder and centrifugation. A microspatula was used to separate symbiont from host, and repeated tissue grinding and centrifugation was used as needed to maintain pure samples of each fraction. Separated cells and tissue were pipetted into different tin capsules (EA Consumables, LLC, Marlton, NJ), and dried at 60°C for at least 24 hours. We visually checked samples under a microscope to ensure no skeletal pieces were in each capsule and no symbionts were in host capsules. All capsules were folded into small, uniform pellets in preparation for isotopic analyses.
Coral fragments collected for analysis of in situ developing eggs were completely decalcified with Cal-Ex II Fixative/Decalcifier containing 10.6% formic acid and 7.4% formaldehyde. Decalcified samples were rinsed in 70% ethanol and developing eggs were dissected from the surrounding tissue under a dissecting microscope. Isolated eggs were similarly pipetted into separate tin capsules, dried and folded prior to isotopic analyses. Gamete bundles collected in the field were thawed and similarly transferred to tin capsules, dried and folded prior to isotopic analyses.
All folded capsules were combusted in an Elementar Pyrocube and the resulting CO2 and N2 gases were analyzed with an Elementar Isoprime100 isotope ratio mass spectrometer at the Academy of Natural Sciences (ANS) at Drexel University in Philadelphia, Pennsylvania, United States or at the Stable Isotope Ecology Laboratory, Center for Applied Isotope Studies, University of Georgia in Athens (UGA), Georgia, United States. δ13C values are reported relative to Vienna Peedee Belemnite Limestone Standard (vPDB) or the per mil (‰) deviation of the ratio of 13C:12C relative to vPDB. δ15N values are reported relative to air or the per mil (‰) deviation of the ratio of 15N:14N relative to air. Approximately 79% of samples were measured in duplicate. At ANS, the standards (± precision) B2150 ± 0.16‰ for δ13C and ± 0.32‰ for δ15N (EA Consumables, LLC, Marlton, New Jersey, United States), internal elk tissue ± 0.11‰ for δ13C and ±0.32‰ for δ15N, and DORM (fish muscle) ± 0.16‰ for δ13C and ± 0.31‰ for δ15N were analyzed. At UGA, bovine liver standard (1577c-B) ± 0.07‰ for δ13C and ± 0.12‰ for δ15N and spinach ± 0.09‰ for δ13C and ± 0.13‰ for δ15N were analyzed.
2.5 Statistical analyses
Statistically significant differences in δ13C and δ15N values were determined separately for each isotope and trophic pulse-chase experiment with mixed effects modeling. These compared the effects of prior bleaching status (bleached, non-bleached), pulse period treatment (13C- or 15N-labeled, 12C- or 14N-labeled), and tissue type (symbiont, host, in situ eggs), and the repeated effect of time during the pulse-chase (0, 1.5, 3, 8, 24, and 72 hours). Each model included a random effect of genotype and tissue type (symbiont, host, in situ eggs) that was nested within prior bleaching status.
To statistically compare the isotopic enrichment of gamete bundles, we used similar mixed effects modeling described for adults. Statistically significant differences in δ13C and δ15N values were determined separately for each isotope and trophic pulse-chase experiment with mixed effects modeling. These compared the effects of prior bleaching status (bleached, non-bleached), pulse period treatment (13C- or 15N-labeled, 12C- or 14N-labeled), and the repeated effect of spawning month during the pulse-chase (June 2018, July 2018, or July 2019). Each model included a random effect of genotype and spawning day was nested within prior bleaching status.
All random and repeated effects within each model were compared with covariance parameter estimates and the fit statistic, -2res log-likelihood, was compared with and without each effect to determine the significance to the model. Post-hoc slice tests were conducted for all models except the heterotrophic acquisition of δ13C since there was a lack of statistical significance among the main effects for that model. For all analyses, p ≤ 0.05 was considered statistically significant. We calculated percent enrichment values to compare average labeled values to their respective controls for each pulse-chase experiment and isotope for visual representation. All statistical analyses were generated using SAS statistical software Version 9.4 of the SAS System for Windows.
3 Results
3.1 Autotrophically- and heterotrophically-acquired carbon
There was significant enrichment of 13C to adult coral symbiont cells and host tissue by the autotrophic pathway in both March and June and a trend of 13C enrichment in developing eggs (Supplementary Table 1). Throughout the pulse-chase, allocation of autotrophic 13C was highest to Symbiodiniaceae, then to host tissue, then to developing eggs (Figure 2). Furthermore, 13C increased in gamete bundles compared to controls, with significant 13C enrichment measured in gamete bundles from the June pulse-chase (Supplementary Table 2). Interestingly, there was no overall difference in the amount of carbon acquired by non-bleached and bleached adult colonies or their developing eggs, but the specific timing of acquisition and subsequent allocation differed between non-bleached and bleached corals. In both March and June, adult hosts and Symbiodiniaceae from non-bleached colonies consistently acquired 13C earlier (at 3 hours during the pulse) compared to bleached colonies that acquired 13C at 8 hours (Figure 2; Supplementary Tables 3, 4). These differences between adults based on past bleaching status, also contributed to differences in allocation of 13C to gamete bundles in June, but not March. In June, gamete bundles from bleached colonies had significantly more 13C than those from non-bleached colonies (Supplementary Table 4). At 72 hours, there was more variability in individual colony responses than compared to the earlier hours of the pulse-chase, resulting in an overall depletion of 13C from adult corals in March (Figures 2A, B). Non-bleached corals in June maintained significant enrichment of 13C in Symbiodiniaceae and hosts, which likely contributed to statistically significant enrichment of 13C in developing eggs and released gamete bundles (Figures 2C, D).
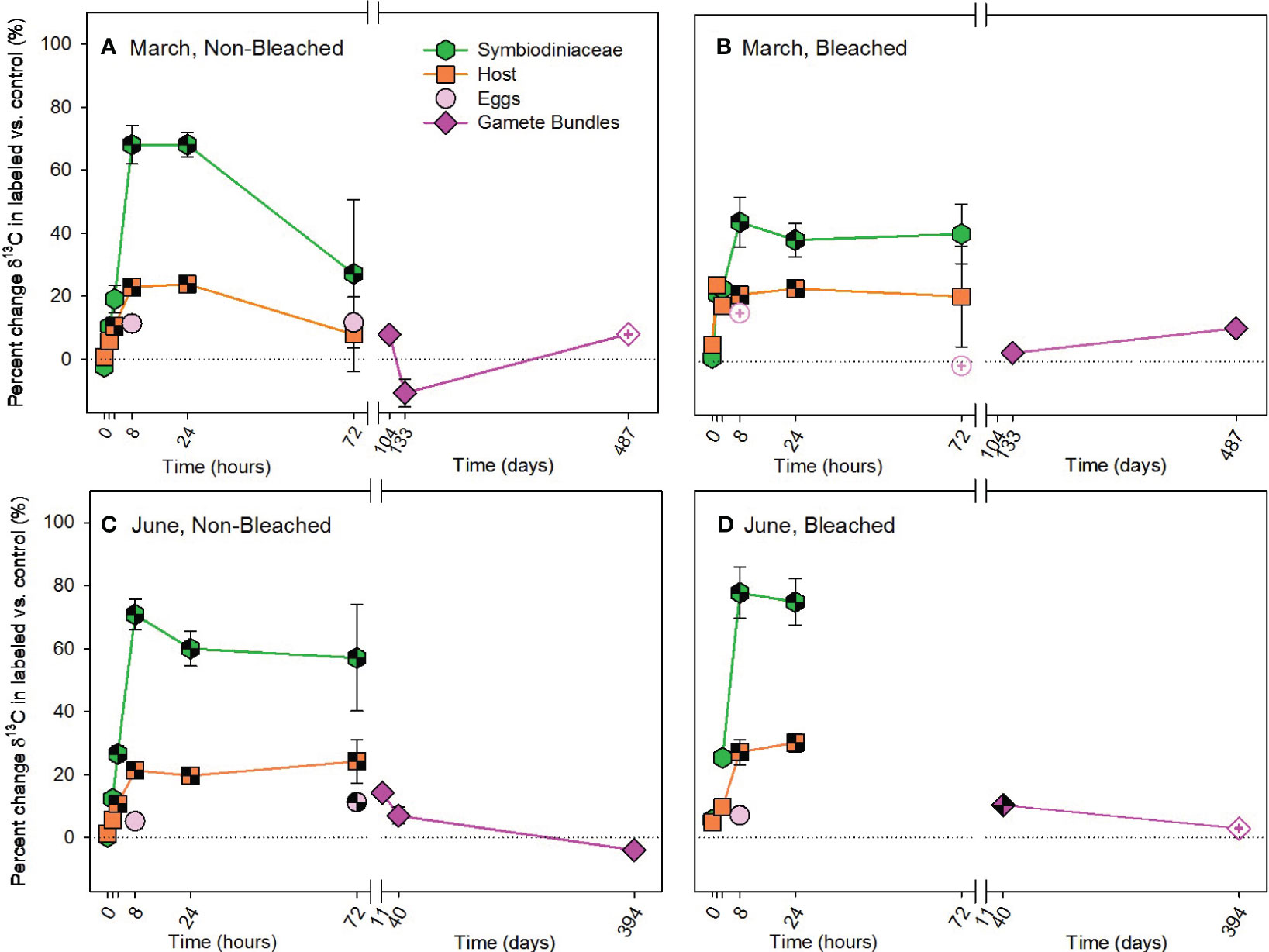
Figure 2 Autotrophic acquisition of carbon during the (A, B) March pulse-chase experiment and (C, D) June pulse-chase experiment. Values are shown as the percent change of δ13C (the average difference between the isotope value of 13C-labeled treatment samples and 12C-control samples) ± 1 propagated standard deviation for symbiont and host fractions of the adults, in situ developing eggs, and released gametes in non-bleached (A, C) and bleached (B, D) colonies. In each case, the pulse phase ended at 8 hours, followed by the chase phase for the remainder of the study. Symbols with a checked pattern indicate statistically significant differences between 13C-labeled treatment samples and 12C-control samples (i.e., significant differences from zero on each graph). Filled symbols without a pattern indicate no statistical difference between treatment and control samples. Symbols with a plus at their center indicate samples that were missing their paired control samples. In these cases for visualization and not statistical comparisons, we calculated percent change by comparing our 13C-labeled treatment samples to natural abundance δ13C reported in the literature for Montipora capitata (Rodrigues and Grottoli, 2006; Padilla-Gamiño et al., 2013). Note that the x-axis is shown in units of hours and days, before and after the axis break, respectively. Data are shown in Supplementary Table 11.
In contrast to the autotrophic pathway, there was no significant enrichment of 13C to adult corals by the heterotrophic pathway in either March or June (Figure 3; Supplementary Table 5). There was minimal difference in the incorporation of 13C between labeled and control colonies, ranging from a difference of -8.75% to 6.43%, representing minimal uptake of 13C. Likewise, no significant enrichment of 13C was measured in developing eggs or in released gamete bundles (Supplementary Table 6).
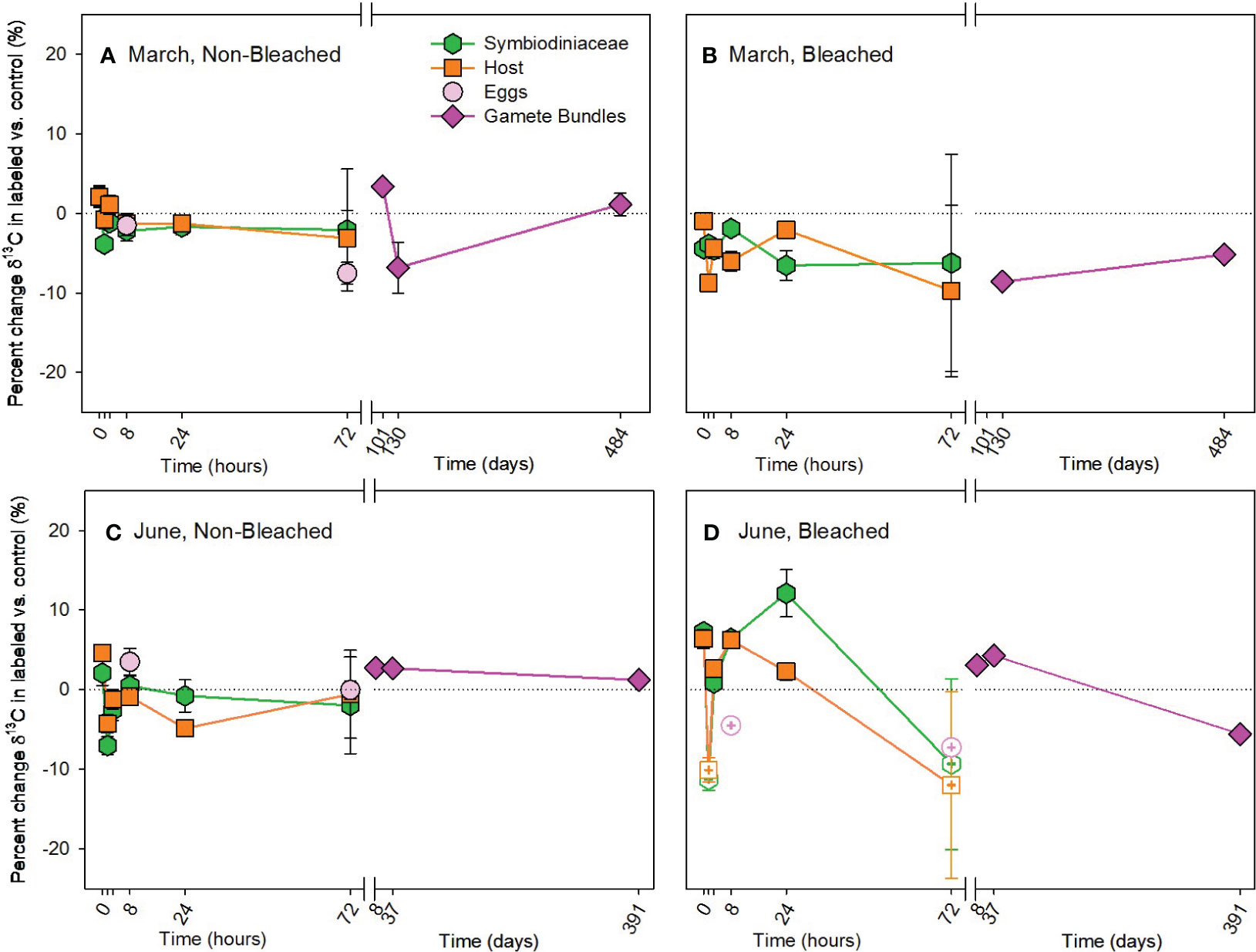
Figure 3 Heterotrophic acquisition of carbon during the (A, B) March pulse-chase experiment and (C, D) June pulse-chase experiment. Values are shown as the percent change of δ13C (the average difference between the isotope value of 13C-labeled treatment samples and 12C-control samples) ± 1 propagated standard deviation for symbiont and host fractions of the adults, in situ developing eggs, and released gametes in non-bleached (A, C) and bleached (B, D) colonies. In each case, the pulse phase ended at 8 hours, followed by the chase phase for the remainder of the study. Symbols with a checked pattern indicate statistically significant differences between 13C-labeled treatment samples and 12C-control samples (i.e., significant differences from zero on each graph). Filled symbols without a pattern indicate no statistical difference between treatment and control samples. Symbols with a plus at their center indicate samples that were missing their paired control samples. In these cases for visualization and not statistical comparisons, we calculated percent change by comparing our 13C-labeled treatment samples to natural abundance δ13C reported in the literature for Montipora capitata (Rodrigues and Grottoli, 2006; Padilla-Gamiño et al., 2013). Note that the x-axis is shown in units of hours and days, before and after the axis break, respectively. Data are shown in Supplementary Table 11.
3.2 Autotrophically- and heterotrophically-acquired nitrogen
The overall pattern for the autotrophic acquisition of nitrogen by M. capitata was similar to that of the autotrophic acquisition of carbon, with no overall difference in the amount acquired due to prior bleaching status, but differences occurred in where and when nitrogen was allocated (Figure 4; Supplementary Table 1). Compared to 13C, 15N accumulated more quickly in adult hosts than Symbiodiniaceae, exhibited fewer differences in allocation between non-bleached and bleached colonies, and was allocated differently between March and June pulse-chases. In March, both non-bleached and bleached hosts acquired 15N at 1.5 hours, while Symbiodiniaceae of both types of colonies acquired 15N by 8 hours (Figures 4A, B; Supplementary Table 7). Acquisition took longer in June, with significant enrichment of 15N occurring in hosts and Symbiodiniaceae of non-bleached and bleached colonies at 8 hours (Figures 4C, D; Supplementary Table 8). Developing eggs were enriched in 15N by more than 50‰ in non-bleached and 40‰ in previously bleached colonies on average compared to 14N controls. This allocation of 15N to developing eggs was maintained in gamete bundles with statistically significant enrichment of 15N following March and June pulse-chases (Supplementary Table 2).
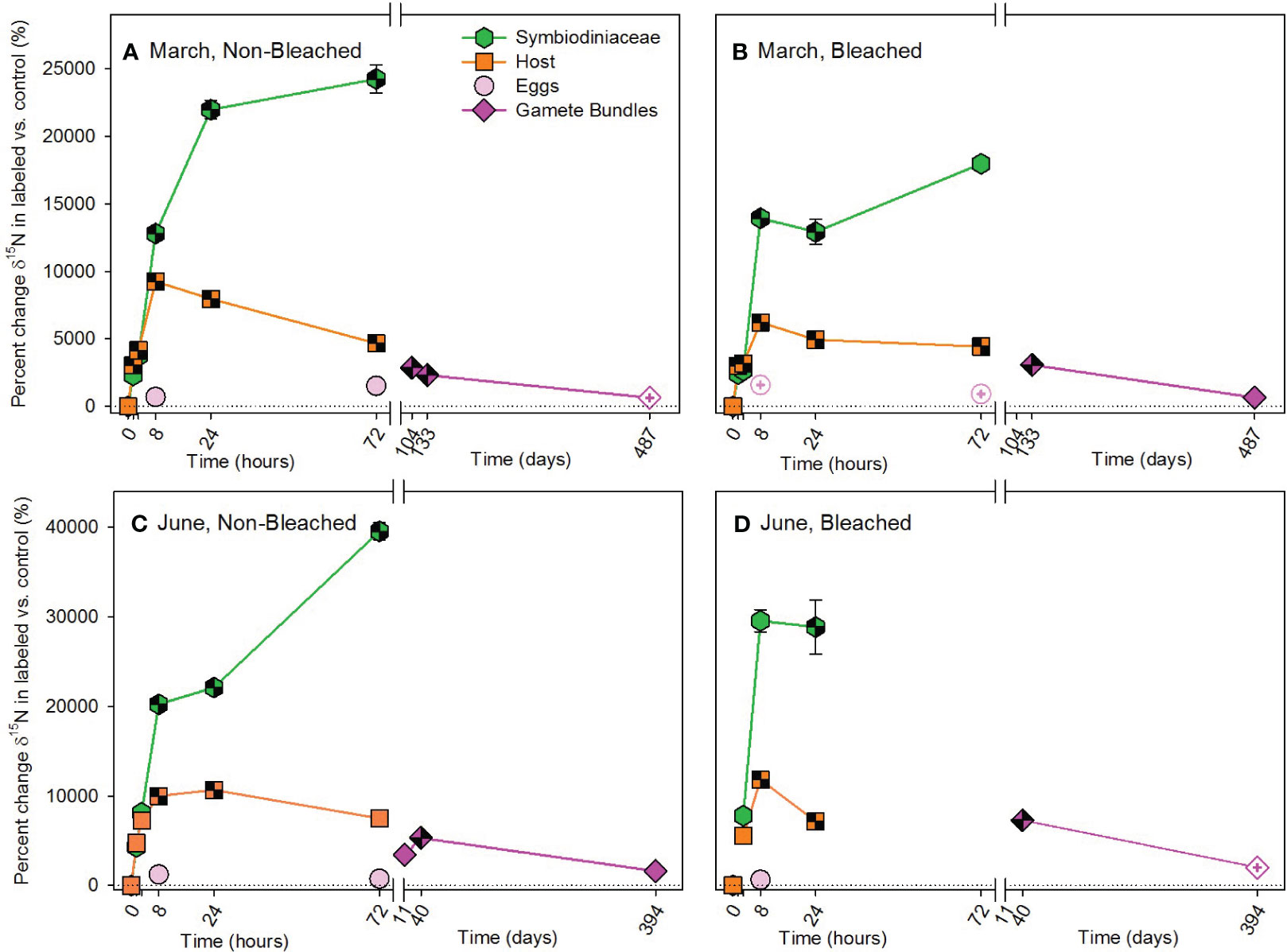
Figure 4 Autotrophic acquisition of nitrogen during the (A, B) March pulse-chase experiment and (C, D) June pulse-chase experiment. Values are shown as the percent change of δ15N (the average difference between the isotope value of 15N-labeled treatment samples and 14N-control samples) ± 1 propagated standard deviation for symbiont and host fractions of the adults, in situ developing eggs, and released gametes in non-bleached (A, C) and bleached (B, D) colonies. In each case, the pulse phase ended at 8 hours, followed by the chase phase for the remainder of the study. Symbols with a checked pattern indicate statistically significant differences between 15N-labeled treatment samples and 14N-control samples (i.e., significant differences from zero on each graph). Filled symbols without a pattern indicate no statistical difference between treatment and control samples. Symbols with a plus at their center indicate samples that were missing their paired control samples. In these cases for visualization and not statistical comparisons, we calculated percent change by comparing our 15N -labeled treatment samples to natural abundance δ15N reported in the literature for Montipora capitata (Rodrigues and Grottoli, 2006; Padilla-Gamiño et al., 2013). Note that the x-axis is shown in units of hours and days, before and after the axis break, respectively, and the y-axis for March and June are shown with different scales. Data are shown in Supplementary Table 11.
In contrast to carbon, nitrogen was acquired heterotrophically with significant differences in enrichment of 15N due to bleaching status, timing of acquisition and compartment used for allocation (Supplementary Table 5). Heterotrophic acquisition of 15N occurred early in both March and June pulse-chases, beginning at 1.5 hours (Figure 5; Supplementary Tables 9, 10). At that time, host tissues were allocated significantly more 15N than Symbiodiniaceae, with a large enrichment of 15N by the host at 1.5 hours. At 8 hours and for the remainder of both chases, the allocation reversed (i.e., symbiont > host). Enrichment of 15N was significantly higher in bleached than non-bleached colonies in March and June. While all Symbiodiniaceae and hosts maintained significant concentrations of 15N at 72 hours, allocation to in situ developing eggs was minimal during our observation times (Figure 5). Interestingly, 15N was not significantly translocated to gamete bundles in March (Figures 5A, B; Supplementary Table 9), but was in June (Figures 5C, D; Supplementary Table 10). Furthermore, in June significantly more 15N was allocated to gamete bundles from bleached colonies, than non-bleached colonies (Supplementary Table 10).
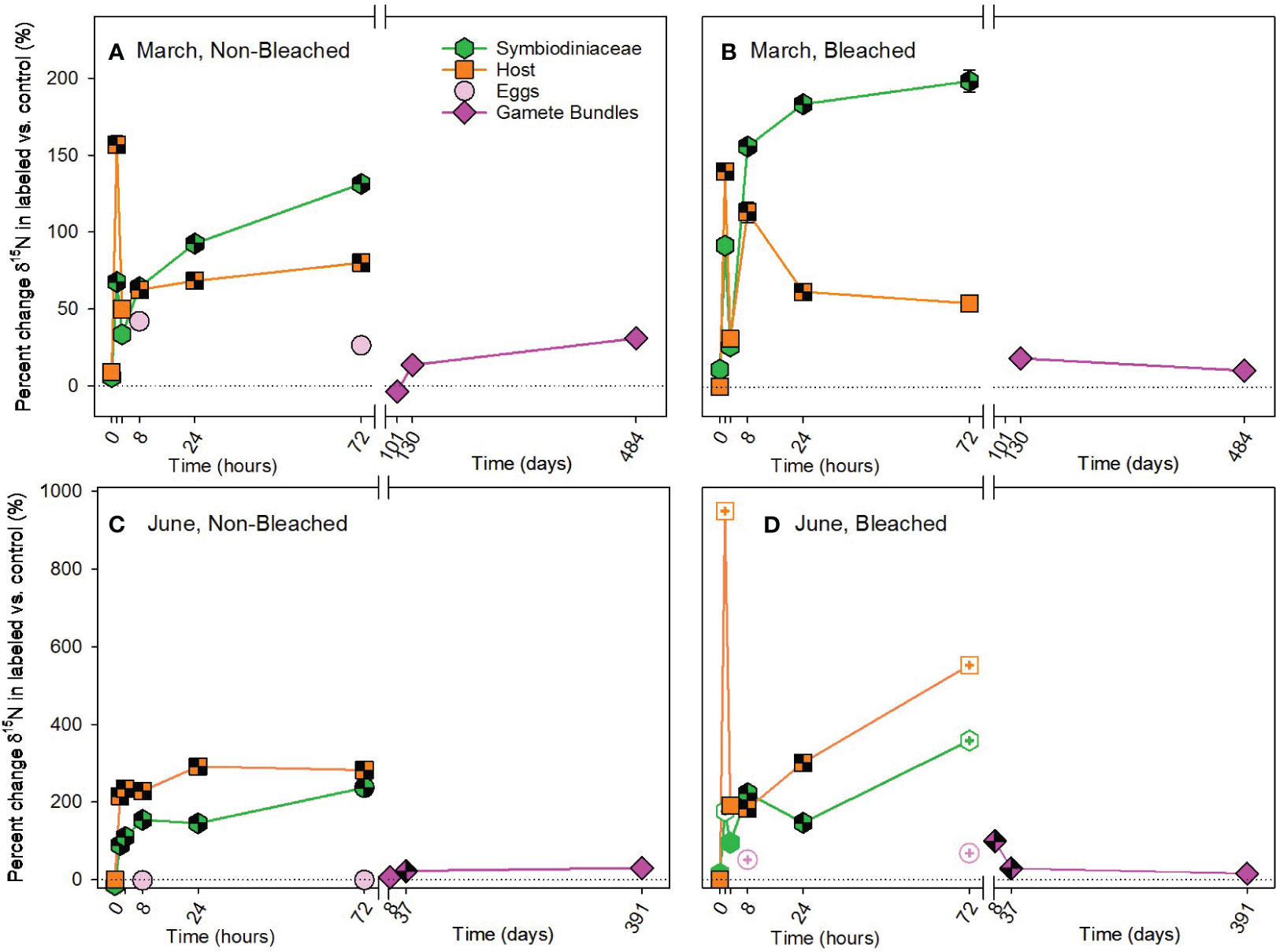
Figure 5 Heterotrophic acquisition of nitrogen during the (A, B) March pulse-chase experiment and (C, D) June pulse-chase experiment. Values are shown as the percent change of δ15N (the average difference between the isotope value of 15N-labeled treatment samples and 14N-control samples) ± 1 propagated standard deviation for symbiont and host fractions of the adults, in situ developing eggs, and released gametes in non-bleached (A, C) and bleached (B, D) colonies. In each case, the pulse phase ended at 8 hours, followed by the chase phase for the remainder of the study. Symbols with a checked pattern indicate statistically significant differences between 15N-labeled treatment samples and 14N-control samples (i.e., significant differences from zero on each graph). Filled symbols without a pattern indicate no statistical difference between treatment and control samples. Symbols with a plus at their center indicate samples that were missing their paired control samples. In these cases for visualization and not statistical comparisons, we calculated percent change by comparing our 15N -labeled treatment samples to natural abundance δ15N reported in the literature for Montipora capitata (Rodrigues and Grottoli, 2006; Padilla-Gamiño et al., 2013). Note that the x-axis is shown in units of hours and days, before and after the axis break, respectively, and the y-axis for March and June are shown with different scales. Data are shown in Supplementary Table 11.
4 Discussion
4.1 Montipora capitata relies on autotrophy and heterotrophy to develop gametes
For adult colonies, acquisition of 13C and 15N was similar to results of past studies, with autotrophic acquisition occurring quickly (within 1.5 hours for M. capitata colonies) and subsequent translocation from Symbiodiniaceae to the coral host within a similar timeframe (Battey and Patton, 1984; Tanaka et al., 2006; Hughes et al., 2010; Kopp et al., 2013; Kopp et al., 2015). Following autotrophy, 13C of the Symbiodiniaceae was significantly higher than the coral host, indicating a dilution effect of the larger coral host-carbon pool compared to the symbiont-carbon pool (Hughes et al., 2010). As a greater ratio of natural abundance (12C) to heavy isotopes was available, the host-carbon pool decreased slightly or stabilized during the chase (Hughes et al., 2010; Kopp et al., 2015). Enrichment of 13C in Symbiodiniaceae achieved a steady state between 8 and 72 hours, supporting the hypothesis that a fraction of the carbon is retained for symbiont growth and maintenance (Kopp et al., 2015; Krueger et al., 2018).
Autotrophically-acquired 15N was incorporated by host tissue 6.5 hours earlier than Symbiodiniaceae in March; however, labelled nitrogen was only available as nitrate (15NO3-), a dissolved inorganic form, available for uptake by autotrophs, typically not heterotrophs (Grover et al., 2003; Krueger et al., 2018; Ferrier-Pagès and Leal, 2019). One possibility is that 15N acquired by Symbiodiniaceae was immediately and rapidly translocated to the host to result in significant enrichment of host tissues before long-term allocation by Symbiodiniaceae. Rapid turnover between symbiont and host is not likely (Tanaka et al., 2006). Alternatively, relatively high concentrations of nitrate used as part of our pulse phase and not typically found in oligotrophic waters may have impacted host uptake of nitrate. By 8 hours, Symbiodiniaceae had accumulated a large 15N pool that remained relatively stable (Kopp et al., 2013; Kopp et al., 2015) and that continued to be cycled between Symbiodiniaceae and host tissues (Tanaka et al., 2006). In June, translocation of 15N from symbiont to host also occurred, but up to 6.5 hours slower than in March. These differences between acquisition in March and June may represent seasonal shifts in symbiont requirements for nitrogen. In June, when levels of solar radiation are higher than in March (Padilla-Gamiño et al., 2014), symbiont demand for nitrogen is also likely higher due to the greater opportunity to photosynthesize. Dissolved inorganic 15N was taken up and used by the symbiont in June, then, when excess was available 8 hours later both host and symbiont fractions stored 15N. Conversely in March, symbiont demand for nitrogen was likely lower, and available 15N was shared more quickly with hosts within just 1.5 hours. Our results for heterotrophically-acquired 15N in M. capitata further support the hypothesis that there are seasonal shifts in symbiont demand for nitrogen and strong coupling of host-symbiont nitrogen pools. We observed a large influx of heterotrophically-acquired 15N by the host at 1.5 months, particularly in March. This pool of 15N was then translocated to the Symbiodiniaceae as the dominant storage location for nitrogen (Kopp et al., 2013; Kopp et al., 2015). As demand for nitrogen by Symbiodiniaceae increased in June, the influx of 15N by non-bleached hosts was dampened and sharing with Symbiodiniaceae occurred more quickly than in March (Figure 5C).
Significant acquisition of 13C by gamete bundles following autotrophy and 15N following both autotrophy and heterotrophy indicates that M. capitata uses both trophic strategies to develop eggs and gamete bundles. Carbon acquired by heterotrophy was not relied upon for gamete development by M. capitata, confirming our prior study (Rodrigues and Padilla-Gamiño, 2022). For the first time we show that 15N was translocated by both trophic sources from adults to developing eggs and gamete bundles, indicating that nitrogen is an important resource that adults share with their offspring. Adult translocation of 13C to gametes was similar during different stages of the gametogenic cycle. For example, the transfer of 13C did not change when developing eggs experienced their most significant increase in growth rate in March or when they obtained their symbiotic algae in June (Padilla-Gamiño and Gates, 2012; Padilla-Gamiño et al., 2014). Physiological demand for carbon may remain consistent as eggs develop and grow. Alternatively, since M. capitata is a split spawner with fully developed and underdeveloped eggs occurring simultaneously within colonies throughout the gametogenic cycle (Padilla-Gamiño et al., 2014), our findings may represent the average amount of carbon translocated to all in situ eggs present.
15N enrichment to gametes preferentially occurred in June by both trophic strategies with significant allocation to gamete bundles that probably coincided with symbiont transmission to eggs (Padilla-Gamiño et al., 2014). Since Symbiodiniaceae are primarily responsible for the uptake of dissolved inorganic nitrogen for the holobiont (Rädecker et al., 2015), co-allocation of 15N and Symbiodiniaceae from parents to gametes is likely. Given the extended period between the pulse phase and collection of gametes (i.e., hundreds of days), it is probable that 15N incorporated by the holobiont during the pulse phase has been assimilated into biomolecules before being used for gamete production (Martinez et al., 2022). Similarly, the recycling of 15N-rich biomolecules may have occurred prior to gamete development.
4.2 Heterotrophy by previously bleached Montipora capitata supplies the nitrogen pool
In contrast to past studies of bleached M. capitata (Hughes et al., 2010; Hughes and Grottoli, 2013; Baumann et al., 2014), prior bleaching status did not affect the overall pattern of carbon allocation by adults or gametes in either month. This may be due to differences in the severity of the stressor, the time elapsed since bleaching stress, and/or the extent of physiological recovery since bleaching (Rodrigues and Grottoli, 2007; Schoepf et al., 2015; Leinbach et al., 2021). Our pulse-chase experiments occurred at six (i.e., March) and nine (i.e., June) months after bleaching stress when bleached and non-bleached colonies had similar chlorophyll concentrations and number of symbiont cells (Padilla-Gamiño et al., in prep). Therefore, by six months, these colonies may have recovered most of their pre-bleaching physiological processes that involve the carbon pool (Rodrigues and Grottoli, 2007). However, the timing of acquisition and translocation of carbon differed through the chase because of prior bleaching status, suggesting some underlying longer-term impacts of bleaching on physiological recovery.
In both March and June, adult hosts and Symbiodiniaceae from non-bleached colonies consistently acquired 13C about five hours earlier than bleached colonies (Figure 2; Supplementary Tables 3, 4). This difference suggests some underlying physiological consequence of bleaching that has a delayed impact on carbon acquisition up to nine months post-bleaching. Symbiodiniaceae may continue to experience photosystem II damage for several months after bleaching in M. capitata (Rodrigues et al., 2008), which could contribute to a delay in carbon acquisition. While previously bleached adults were slower to acquire carbon in both months, in June gamete bundles from bleached colonies acquired significantly more 13C than gamete bundles from non-bleached colonies. Since we followed spawning one week after the pulse-chase in June and one month later in July, higher concentrations of 13C may have been readily available following the June pulse-chase for incorporation into gamete bundles. This suggests that the weeks leading up to spawning are critical for allocation of resources to gametes (Padilla-Gamiño and Gates, 2012; Padilla-Gamiño et al., 2013; Padilla-Gamiño et al., 2014).
Carbon acquired by heterotrophy was not relied upon for gamete development by M. capitata even when previously bleached (Rodrigues and Padilla-Gamiño, 2022). Although M. capitata is known to significantly increase the contribution of heterotrophically acquired carbon to daily animal respiration (CHAR) immediately after bleaching, when non-bleached the species also had the lowest CHAR (Grottoli et al., 2006) with the lowest feeding rate (Palardy et al., 2008) of the three Hawaiian species tested. Carbon allocation to adult colonies and gametes of M. capitata supports earlier work on this species, suggesting that reliance on heterotrophy for carbon acquisition decreases in favor of autotrophy once concentrations of chlorophyll concentrations have recovered (Grottoli et al., 2006; Rodrigues and Grottoli, 2007; Palardy et al., 2008). The dual label design of our study and clear incorporation of heterotrophically-acquired 15N (Figure 5) indicates that both previously bleached and non-bleached colonies were feeding. Therefore, negligible 13C enrichment from heterotrophy by Symbiodiniaceae and host tissue is likely due to translocation and storage of 13C to the coral skeleton, limited heterotrophic feeding and subsequent rapid respiration of 13C, and/or excretion of 13C in mucus. In each of these scenarios, some enrichment of 13C in Symbiodiniaceae and/or host tissue would be expected (Hughes et al., 2010; Krueger et al., 2018). Previous heterotrophic pulse-chase studies found almost no 13C enrichment in the skeleton and qualitatively low enrichment in Symbiodiniaceae and host tissue (Hughes et al., 2010). Rapid respiration of heterotrophically-acquired 13C is the most likely scenario (Martinez et al., 2022). Future studies should assess carbon acquisition and translocation to gametes throughout the gametogenic cycle, including immediately after bleaching to assess the contribution of elevated rates of heterotrophic carbon to gamete development. In Kaneohe Bay, bleaching events due to thermal stress generally occur at the same time as the early stages of gametogenesis of M. capitata (Padilla-Gamiño et al., 2014; Bahr et al., 2017). Here we show that bleached M. capitata recovers after the thermal stress and continues gametogenesis by relying primarily on carbon from autotrophy, not heterotrophy. Thus, indicating that recovery of photosynthesis after bleaching is critical for gametogenesis in M. capitata.
While bleaching did not influence the autotrophic acquisition of 15N, heterotrophic acquisition of 15N was higher in bleached than non-bleached colonies. This suggests that one purpose of increased heterotrophy by M. capitata following bleaching may be to increase the amount of nitrogen available to the coral holobiont, especially in oligotrophic tropical environments (Tanaka et al., 2006; Kopp et al., 2013; Krueger et al., 2018). Nitrate in low concentrations is likely to enhance recovery, acquisition and/or both of Symbiodiniaceae and chlorophyll following coral bleaching (reviewed in Morris et al., 2019) and also seems to have positive implications for gametogenesis in M. capitata. Gamete bundles from previously bleached colonies had higher concentrations of 15N than non-bleached colonies in June only, most likely associated with transmission of 15N-enriched Symbiodiniaceae at that time. Therefore, species like M. capitata with vertical transmission of Symbiodiniaceae (Padilla-Gamiño et al., 2014) may prioritize heterotrophic acquisition of nitrogen following bleaching to have a ready supply of Symbiodiniaceae for their gametes. Observations of continued reproduction by M. capitata despite bleaching (Cox, 2007) may be more dependent on nitrogen acquisition, than carbon as previously hypothesized.
Data availability statement
The raw data supporting the conclusions of this article will be made available by the authors, without undue reservation.
Ethics statement
The manuscript presents research on animals that do not require ethical approval for their study.
Author contributions
MJ: Conceptualization; Formal analysis; Investigation; Visualization; Writing – original draft; Writing – review & editing. JP-G: Conceptualization; Funding acquisition; Writing – review & editing. BN: Funding acquisition; Writing – review & editing. LR: Conceptualization; Formal analysis; Funding acquisition; Supervision; Visualization; Writing – original draft; Writing – review & editing.
Funding
The author(s) declare financial support was received for the research, authorship, and/or publication of this article. This research was supported by National Science Foundation’s Division of Integrative Organismal Systems, Integrative Ecological Physiology Program (NSF IOS-IEP) 1655888 to LR and NSF IOS-IEP 1655682 to JP-G and BN.
Acknowledgments
We thank Dr. Ruth Gates and the Gates Coral Laboratory for our sponsorship at the Hawai’i Institute of Marine Biology. We thank T. Brown, G. Kreitman, P. Zelanko, J. Axworthy, S. Frangos, J. Davidson, E. Lenz, and C. Backstrom for field and laboratory support; D. Velinsky, P. Zelanko, and M. Gannon for isotopic analyses; K. Brittain for assistance with phytoplankton and zooplankton cultures; and P. Bernhardt for statistical assistance. This research was conducted under Hawai’i Department of Land and Natural Resources Special Activity Permit No. 2018-46. This is HIMB contribution number #1941 and School of Ocean and Earth Science and Technology (SOEST) contribution number #11748.
Conflict of interest
The authors declare that the research was conducted in the absence of any commercial or financial relationships that could be construed as a potential conflict of interest.
Publisher’s note
All claims expressed in this article are solely those of the authors and do not necessarily represent those of their affiliated organizations, or those of the publisher, the editors and the reviewers. Any product that may be evaluated in this article, or claim that may be made by its manufacturer, is not guaranteed or endorsed by the publisher.
Supplementary material
The Supplementary Material for this article can be found online at: https://www.frontiersin.org/articles/10.3389/fevo.2023.1251220/full#supplementary-material
References
Anthony K. R. N., Fabricius K. (2000). Shifting roles of heterotrophy and autotrophy in coral energetics under varying turbidity. J. Exp. Mar. Biol. Ecol. 252, 221–253. doi: 10.1016/S0022-0981(00)00237-9
Axworthy J. B., Timmins-Schiffman E., Brown T., Rodrigues L. J., Nunn B. L., Padilla-Gamiño J. L. (2022). Shotgun proteomics identifies active metabolic pathways in bleached coral tissue and intraskeletal compartments. Front. Mar. Sci. 9. doi: 10.3389/fmars.2022.797517
Bahr K. D., Rodgers K. S., Jokiel P. L. (2017). Impact of three bleaching events on the reef resiliency of Kāne’ohe Bay, Hawai’i. Front. Mar. Sci. 4. doi: 10.3389/fmars.2017.00398
Battey J. F., Patton J. S. (1984). A reevaluation of the role of glycerol in carbon translocation in zooxanthellae-coelenterate symbiosis. Mar. Biol. 79, 27–38. doi: 10.1007/BF00404982
Baumann J., Grottoli A. G., Hughes A. D., Matsui Y. (2014). Photoautotrophic and heterotrophic carbon in bleached and non-bleached coral lipid acquisition and storage. J. Exp. Mar. Biol. Ecol. 461, 469–478. doi: 10.1016/j.jembe.2014.09.017
Coles S. L., Bahr K. D., Rodgers K. S., May S. L., McGowan A. E., Tsang A., et al. (2018). Evidence of acclimatization or adaptation in Hawaiian corals to higher ocean temperatures. PeerJ 2018, 1–24. doi: 10.7717/peerj.5347
Conti-Jerpe I. E., Thompson P. D., Wong C. W. M., Oliveira N. L., Duprey N. N., Moynihan M. A., et al. (2020). Trophic strategy and bleaching resistance in reef-building corals. Sci. Adv. 6. doi: 10.1126/sciadv.aaz5443
Cox E. F. (2007). Continuation of sexual reproduction in Montipora capitata following bleaching. Coral Reefs 26, 721–724. doi: 10.1007/s00338-007-0251-9
Crossland C. J., Barnes D. J., Borowitzka M. A. (1980). Diurnal lipid and mucus production in the staghorn coral Acropora acuminata. Mar. Biol. 60, 81–90. doi: 10.1007/BF00389151
Ferrier-Pagès C., Leal M. C. (2019). Stable isotopes as tracers of trophic interactions in marine mutualistic symbioses. Ecol. Evol. 9, 723–740. doi: 10.1002/ece3.4712
Ferrier-Pagés C., Peirano A., Abbate M., Cocito S., Negri A., Rottier C., et al. (2011). Summer autotrophy and winter heterotrophy in the temperate symbiotic coral Cladocora caespitosa. Limnol Oceanogr 56, 1429–1438. doi: 10.4319/lo.2011.56.4.1429
Grottoli A. G., Rodrigues L. J., Palardy J. E. (2006). Heterotrophic plasticity and resilience in bleached corals. Nature 440, 1186–1189. doi: 10.1038/nature04565
Grover R., Maguer J. F., Allemand D., Ferrier-Pagès C. (2003). Nitrate uptake in the scleractinian coral Stylophora pistillata. Limnol Oceanogr 48, 2266–2274. doi: 10.4319/lo.2003.48.6.2266
Houlbrèque F., Ferrier-Pagès C. (2009). Heterotrophy in tropical scleractinian corals. Biol. Rev. 84, 1–17. doi: 10.1111/j.1469-185X.2008.00058.x
Hughes A. D., Grottoli A. G. (2013). Heterotrophic compensation: A possible mechanism for resilience of coral reefs to global warming or a sign of prolonged stress? PLoS One 8, 1–10. doi: 10.1371/journal.pone.0081172
Hughes A. D., Grottoli A. G., Pease T. K., Matsui Y. (2010). Acquisition and assimilation of carbon in non-bleached and bleached corals. Mar. Ecol. Prog. Ser. 420, 91–101. doi: 10.3354/meps08866
Hughes T. P., Kerry J. T., Álvarez-Noriega M., Álvarez-Romero J. G., Anderson K. D., Baird A. H., et al. (2017). Global warming and recurrent mass bleaching of corals. Nature 543, 373–377. doi: 10.1038/nature21707
Kopp C., Domart-Coulon I., Escrig S., Humbel B. M., Hignette M., Meibom A. (2015). Subcellular investigation of photosynthesis-driven carbon assimilation in the symbiotic reef coral Pocillopora damicornis. mBio 6, 1–9. doi: 10.1128/mBio.02299-14
Kopp C., Pernice M., Domart-coulon I., Djediat C., Spangenberg J. E., Alexander D. T. L., et al. (2013). Highly dynamic cellular-level response of symbiotic coral to a. ISME J. 4, 1–9. doi: 10.1128/mBio.00052-13.Invited
Krueger T., Bodin J., Horwitz N., Loussert-Fonta C., Sakr A., Escrig S., et al. (2018). Temperature and feeding induce tissue level changes in autotrophic and heterotrophic nutrient allocation in the coral symbiosis – A NanoSIMS study. Sci. Rep. 8, 1–15. doi: 10.1038/s41598-018-31094-1
LaJeunesse T. C., Parkinson J. E., Gabrielson P. W., Jeong H. J., Reimer J. D., Voolstra C. R., et al. (2018). Systematic revision of symbiodiniaceae highlights the antiquity and diversity of coral endosymbionts. Curr. Biol. 28, 2570–2580.e6. doi: 10.1016/j.cub.2018.07.008
Leinbach S. E., Speare K. E., Rossin A. M., Holstein D. M., Strader M. E. (2021). Energetic and reproductive costs of coral recovery in divergent bleaching responses. Sci. Rep. 11, 1–10. doi: 10.1038/s41598-021-02807-w
Martinez S., Grover R., Baker D. M., Ferrier-Pagès C. (2022). Symbiodiniaceae are the first site of heterotrophic nitrogen assimilation in reef-building corals. mBio 13. doi: 10.1128/mbio.01601-22
Morris L. A., Voolstra C. R., Quigley K. M., Bourne D. G., Bay L. K. (2019). Nutrient availability and metabolism affect the stability of coral–symbiodiniaceae symbioses. Trends Microbiol. 27, 678–689. doi: 10.1016/j.tim.2019.03.004
Muscatine L., McCloskey L. R., Marian R. E. (1981). Estimating the daily contribution of carbon from zooxanthellae to coral animal respiration. Limnol Oceanogr 25, 601–611. doi: 10.4319/lo.1981.26.4.0601
Osinga R., Schutter M., Griffioen B., Wijffels R. H., Verreth J. A. J., Shafir S., et al. (2011). The biology and economics of coral growth. Mar. Biotechnol. 13, 658–671. doi: 10.1007/s10126-011-9382-7
Padilla-Gamiño J. L., Bidigare R. R., Barshis D. J., Alamaru A., Hédouin L., Hernández-Pech X., et al. (2013). Are all eggs created equal? A case study from the Hawaiian reef-building coral Montipora capitata. Coral Reefs 32, 137–152. doi: 10.1007/s00338-012-0957-1
Padilla-Gamiño J. L., Gates R. D. (2012). Spawning dynamics in the Hawaiian reef-building coral Montipora capitata. Mar. Ecol. Prog. Ser. 449, 145–160. doi: 10.3354/meps09530
Padilla-Gamiño J. L., Hédouin L., Waller R. G., Smith D., Truong W., Gates R. D. (2014). Sedimentation and the reproductive biology of the hawaiian reef-building coral Montipora capitata. Biol. Bull. 226, 8–18. doi: 10.1086/BBLv226n1p8
Palardy J. E., Rodrigues L. J., Grottoli A. G. (2008). The importance of zooplankton to the daily metabolic carbon requirements of healthy and bleached corals at two depths. J. Exp. Mar. Biol. Ecol. 367, 180–188. doi: 10.1016/j.jembe.2008.09.015
Patton J. S., Battey J. F., Rigler M. W., Porter J. W., Black C. C., Burris J. E. (1983). A comparison of the metabolism of bicarbonate 14C and acetate 1-14C and the variability of species lipid compositions in reef corals. Mar. Biol. 75, 121–130. doi: 10.1007/BF00405994
Rädecker N., Pogoreutz C., Voolstra C. R., Wiedenmann J., Wild C. (2015). Nitrogen cycling in corals: The key to understanding holobiont functioning? Trends Microbiol. 23, 490–497. doi: 10.1016/j.tim.2015.03.008
Randall C. J., Negri A. P., Quigley K. M., Foster T., Ricardo G. F., Webster N. S., et al. (2020). Sexual production of corals for reef restoration in the Anthropocene. Mar. Ecol. Prog. Ser. 635, 203–232. doi: 10.3354/MEPS13206
Rodrigues L. J., Grottoli A. G. (2006). Calcification rate and the stable carbon, oxygen, and nitrogen isotopes in the skeleton, host tissue, and zooxanthellae of bleached and recovering Hawaiian corals. Geochim Cosmochim Acta 70, 2781–2789. doi: 10.1016/j.gca.2006.02.014
Rodrigues L. J., Grottoli A. G. (2007). Energy reserves and metabolism as indicators of coral recovery from bleaching. Limnol Oceanogr 52, 1874–1882. doi: 10.4319/lo.2007.52.5.1874
Rodrigues L. J., Grottoli A. G., Lesser M. P. (2008). Long-term changes in the chlorophyll fluorescence of bleached and recovering corals from Hawaii. J. Exp. Biol. 211, 2505–2509. doi: 10.1242/jeb.012369
Rodrigues L. J., Padilla-Gamiño J. L. (2022). Trophic provisioning and parental trade-offs lead to successful reproductive performance in corals after a bleaching event. Sci. Rep. 12, 18702. doi: 10.1038/s41598-022-21998-4
Schoepf V., Grottoli A. G., Levas S. J., Aschaffenburg M. D., Baumann J. H., Matsui Y., et al. (2015). Annual coral bleaching and the long-term recovery capacity of coral. Proc. R. Soc. B: Biol. Sci. 282 (1819), 20151887. doi: 10.1098/rspb.2015.1887
Stanley G. D., van de Schootbrugge B. (2009). “The evolution of the coral – algal symbiosis,” in Coral Bleaching. Ecological Studies, vol. 205 . Eds. van Oppen M. J. H., Lough J. M. (Berlin Heidelberg: Springer Berlin Heidelberg), 7–19. doi: 10.1007/978-3-540-69775-6
Tanaka Y., Miyajima T., Koike I., Hayashibara T., Ogawa H. (2006). Translocation and conservation of organic nitrogen within the coral-zooxanthella symbiotic system of Acropora pulchra, as demonstrated by dual isotope-labeling techniques. J. Exp. Mar. Biol. Ecol. 336, 110–119. doi: 10.1016/j.jembe.2006.04.011
Torda G., Donelson J. M., Aranda M., Barshis D. J., Bay L., Berumen M. L., et al. (2017). Rapid adaptive responses to climate change in corals. Nat. Clim Chang 7, 627–636. doi: 10.1038/nclimate3374
Trench R. K. (1971). The physiology and biochemistry of zooxanthellae symbiotic with marine coelenterates I. The assimilation of photosynthetic products of zooxanthellae by two marine coelenterates. Proc. R. Soc. London. Ser. B. 177, 225–235. doi: 10.1098/rspb.1971.0026
Keywords: autotrophy/heterotrophy, coral bleaching, gametogenesis, Montipora capitata, stable isotopes, reproduction, resilience, Symbiodiniaceae
Citation: Jaffe MD, Padilla-Gamiño JL, Nunn BL and Rodrigues LJ (2023) Coral trophic pathways impact the allocation of carbon and nitrogen for egg development after bleaching. Front. Ecol. Evol. 11:1251220. doi: 10.3389/fevo.2023.1251220
Received: 01 July 2023; Accepted: 24 October 2023;
Published: 08 November 2023.
Edited by:
Vera Tai, Western University, CanadaReviewed by:
Jonathan D. Cybulski, Smithsonian Tropical Research Institute (Panama), PanamaGermán Zapata-Hernández, Anton Dohrn Zoological Station Naples, Italy
Mark McCauley, United States Department of the Interior, United States
Copyright © 2023 Jaffe, Padilla-Gamiño, Nunn and Rodrigues. This is an open-access article distributed under the terms of the Creative Commons Attribution License (CC BY). The use, distribution or reproduction in other forums is permitted, provided the original author(s) and the copyright owner(s) are credited and that the original publication in this journal is cited, in accordance with accepted academic practice. No use, distribution or reproduction is permitted which does not comply with these terms.
*Correspondence: Lisa J. Rodrigues, bGlzYS5yb2RyaWd1ZXNAdmlsbGFub3ZhLmVkdQ==