Evaluating methods for determining mercury concentrations in ancient marine fish and mammal bones as an approach to assessing millennial-scale fluctuations in marine ecosystems
- 1Water and Environmental Research Center, Institute of Northern Engineering, University of Alaska Fairbanks, Fairbanks, AK, United States
- 2Department of Veterinary Medicine, University of Alaska Fairbanks, Fairbanks, AK, United States
- 3Department of Anthropology, University at Buffalo, Buffalo, NY, United States
- 4Veterinary Integrative Biosciences, School of Veterinary Medicine & Biomedical Sciences, Texas A&M University, College Station, TX, United States
Millennial-scale datasets of heavy metals in biota are difficult to obtain but are important for determining patterns and underlying drivers of toxicant concentrations. This is particularly important to better discriminate contemporary natural and anthropogenic sources. Globally mercury is a contaminant of concern. Post-industrial increases in mercury in arctic biota have been documented and monitoring of Steller sea lions (Eumetopias jubatus) in the Aleutian Islands, Alaska, has revealed a high proportion of pups with fur mercury concentrations above thresholds of concern in some regions. As bone is a tissue that is well preserved in archeological middens, it may prove useful for developing long-term mercury data sets under appropriate conditions. The goal of this study was to evaluate methodologies for measuring mercury concentration in Steller sea lion bone using a direct mercury analyzer, considering sample preparation methods and variability among bone tissue types (e.g., compact versus spongy bone). Finally, we directly compare sensitivity and precision of two different direct mercury analyzer models. Based on the methods presented here, direct mercury analysis using the Nippon MA-3000 can quantify small (ppb) quantities of Hg accurately and precisely in 20 to 60mg of bone with minimal specimen processing. The described method is efficient, relatively inexpensive, and requires minimal bone, conserving rare and valuable specimens. Hydrogen peroxide cleaning and collagen extraction were not required, and may be detrimental for optimal Hg quantification in bone. Further, while homogenization of distinct compact and spongy bone did not impact concentration determination, variance of technical replicates was lower improving quantitation precision. Most importantly, significant differences between compact and spongy bone exist within some individual specimen; however, the difference is not consistent and may indicate differential Hg exposure windows influenced by turnover rate of bone types. We conclude bone provides a natural archive for mercury ecosystem dynamics over millennial time scales in regions where appropriate samples are available. Compact bone has lower and less variable [THg] simplifying analysis and interpretation of data; however, the more dynamic concentrations observed in spongy bone should not be dismissed as invaluable due to their variability in [THg]. Comparisons of [THg] between bone type within individual may provide insight into more acute changes in mercury exposure within an individual’s lifetime.
Introduction
Mercury (Hg) is one of the top 10 contaminants of concern for human health with estimates that between 1.5 and 17 per thousand children in coastal fishing communities exhibit toxic health effects from Hg exposure (WHO, 2010; Liu et al., 2014). Arctic coastal communities are especially vulnerable to the effects of Hg contamination due to their reliance on aquatic and marine ecosystems for sociocultural, economic, and subsistence resources (Basu et al., 2022; Dietz et al., 2022),. Predatory wildlife also serve as ecosystem sentinels for Hg exposure and potential impacts on human health due to their position in the food web and utilization as subsistence resources by Native communities (Basu and Head, 2010; Castellini et al., 2012). Relatively high total mercury concentrations ([THg]) have been found in some Steller sea lions (Eumetopias jubatus) in the Aleutian Islands, Alaska (Rea et al., 2020) prompting questions about long-term, millennial trends of [THg] in Aleutian Island food webs.
Millennial-scale datasets of heavy metals in biota are difficult to obtain but are important for determining patterns and underlying drivers of heavy metal concentrations. This is particularly important to better discriminate contemporary natural and anthropogenic sources (Barst et al., 2022; Chastel et al., 2022; Morris et al., 2022). While significant increases in Hg concentrations ([Hg]) have been observed over the past 150 years in parts of the Arctic (Dietz et al., 2022), limited information is available for longer term, pre-Industrial revolution (1750 CE) periods.
Bone may be a valuable tissue from which to develop long-term Hg data sets, as it is preserved in many archaeological sites. Mercury bioaccumulates in bone during an organism’s lifetime, and the long turnover time of this tissue removes the confounding factor of seasonality (Manolagas, 2000). Mercury content of bone has been examined in modern and historic humans (Rasmussen et al., 2013; Zioła-Frankowska et al., 2017; Álvarez-Fernández et al., 2020; López-Costas et al., 2020), seals (Aubail et al., 2010), and fish (Murray et al., 2015). Several studies have determined that Hg remains in human bone with undetectable leaching into adjacent soils (Yamada et al., 1995; Rasmussen et al., 2015; Álvarez-Fernández et al., 2022). Further, there is likely no uptake of Hg post-mortem in Hg-laden burial sites (Ãvila et al., 2014; Emslie et al., 2019) as bone Hg is present within localized osteon (areas of active remodeling) as inorganic mercuric sulfide (Swanston et al., 2015).
There are two general types of bone tissue: compact (cortical) and spongy (trabecular). The uniqueness in structure and physiology of each contributes to differences in organic and inorganic composition. Studies in humans indicate that specific bone elements (e.g., femur) vary both in the both carbon and nitrogen stable isotope and heavy metal concentration (Zioła-Frankowska et al., 2017). In the human skeleton, mercury concentration of compact bone is consistent while limited data suggests mercury content of spongy bone is more variable (Rasmussen et al., 2013; Zioła-Frankowska et al., 2017). These composition differences are likely related to the turnover rate of bone types and proportion of compact and spongy bone present in each bone sample (Manolagas, 2000; Rasmussen et al., 2013). The extracellular matrix (ECM) of osseous tissue within bone is comprised primarily (60%) of inorganic hydroxyapatite containing calcium and phosphate minerals, and organic proteins (30%) including collagen, non-collagen proteins, proteoglycans, and glycosaminoglycans (Le et al., 2017). The disulfide bridges formed in cystine rich proteins are well known to bind mercury (Song et al., 2021). The most abundant protein in bone, Type I collagen, is a cystine rich protein that likely sequesters mercury in the ECM; however, precise molecular interaction of mercury in bone is unknown.
This pilot investigation measured [THg] in bone fragments of ancient otariids (Steller sea lions and northern fur seals; Callorhinus ursinus) collected during archaeological excavation of ancestral Unanga (Alaska Native) middens in the regions of the eastern and western Aleutian Islands. Middens are layers of refuse that accrete over thousands of years and contain discarded plant and animal material utilized by Native people. Archaeological bones provide information about [THg] over several centuries to help us evaluate contemporary trends in [THg] observed in Aleutian Island sea lions (Rea et al., 2020). The goal of this study was to evaluate methods of bone mercury analysis using the direct mercury analyzer available in our laboratory, considering sample preparation, variability among bone tissue types (e.g., compact versus spongy bone). Finally, we will directly compare sensitivity (method detection limit) and precision (CV) of two different direct mercury analyzer units.
Our hypotheses are that (1) [THg] differs significantly between bone tissue types, (2) collagen extraction is not necessary for reliable quantification of bone Hg, and (3) peroxide treatment decreases [THg]. Reliable determination of [THg] in bone using a direct mercury analyzer provide ecologists significant opportunity to investigate natural variation in mercury dynamics across diverse ecosystems and across millennial timeframes using this relatively stable material that is well represented in ancient middens. Understanding these long-term natural mercury dynamics is important to help future studies identify what environmental factors contribute significantly to [THg] in ecosystem sentinels, both past and present.
Methods
Bone sample collection
Archaeological bone
Archaeological otariid (fur seal and sea lion) skeletal elements (or fragments thereof) were excavated from village middens of ancestral Unanga. Unanga (Aleut) are Alaska Natives who have lived in the Aleutian Islands for 9000 years. Otariid bones included Steller sea lions, northern fur seals (Callorhinus ursinus) and fragments that could not be identified to species. Specimen were acquired (n = 40) from middens located on Sanak and Kiska Islands, Alaska (Figure 1). These sites span the time period from 3700 to 180 years before present (YBP) as determined by radiocarbon dating (Misarti et al., 2011; Funk, 2018).
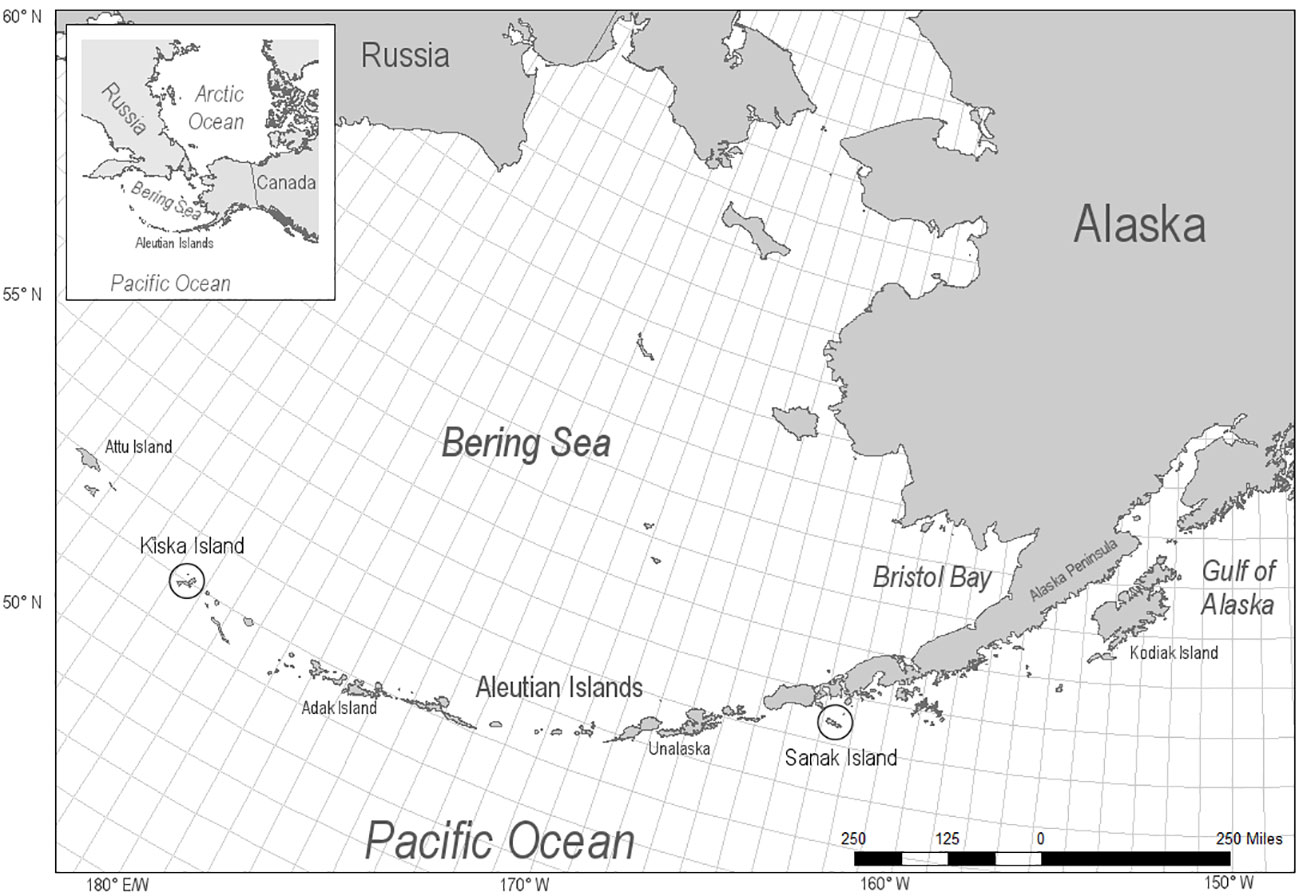
Figure 1 Locations of archeological otariid specimen, Steller sea lion and northern fur seals, from Sanak Island (n = 36) in the Eastern Aleutian Islands and Kiska (n = 4) in the Western Aleutian Islands, Alaska.
This study included skeletal elements from the axial and appendicular skeleton (Table S1) with varying quantities of compact and spongy bone. Archaeological samples were from a range of age categories. Based on osteological criteria, including degree of fusion of epiphyseal plate, most of the specimens were mature adults (Grayson, 1984). Archeological bones and bone fragments were used to compare [THg] between compact and spongy bone within individuals and to assess analytical differences between homogenized and non-homogenized bone (Figure 2).
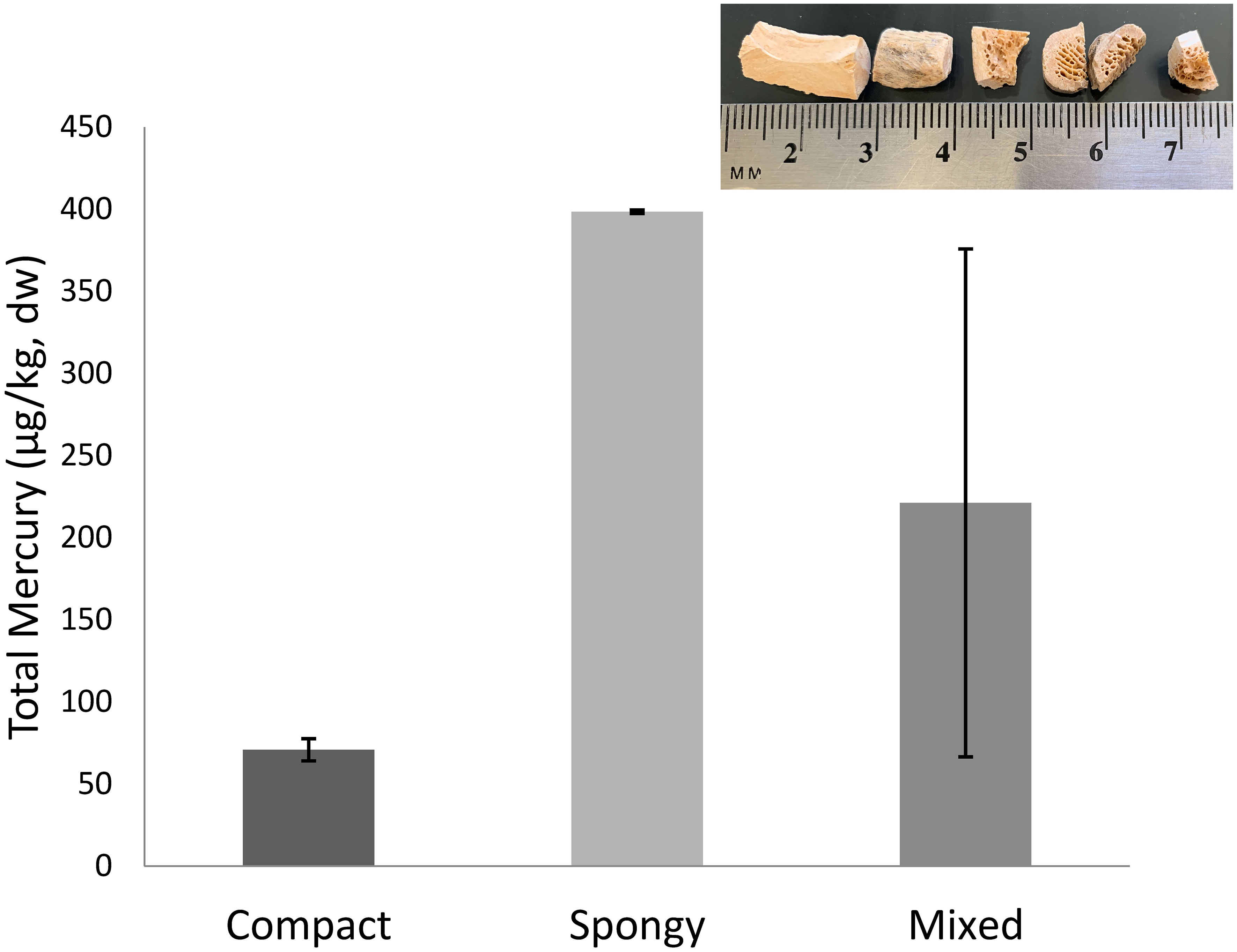
Figure 2 Total mercury concentration (µg/kg, dry weight) of a mixed bone fragment compared with analysis of separated spongy and compact bone segments from the same specimen. Bars represent standard deviation of technical replicates. (Top Inset Image) Bone fragments illustrating structural difference in solid, superficial cortical or compact bone and porous, deep trabecular or spongy bone. Bone types are mechanically separated and analyzed independently for mercury content.
Modern bone
Due to the large quantity of bone material needed to assess the impact of collagen extraction and peroxide masceration on [THg] quantification, and to validate [THg] measures between two models of direct mercury analyzers, modern bone specimen was utilized. Modern otariid bone were collected opportunistically from Steller sea lion pup carcasses found during population counts completed by NOAA in 2011 on Agattu Island in the Western Aleutian Islands (n = 2) and in 2012-2013 on Ulak and Seguam Islands in the Central Aleutian Islands (n = 4; total n = 6, ADF&G MMPA/ESA Permit No. 14325). Carcasses were processed according to methods outlined in Correa et al. (2014). The skeletal muscle was mechanically removed from the bones, which were subsequently placed in distilled water for 3 weeks to facilitate mechanical removal of the remaining fascial tissue. The clean, whole femur and the 4th rib bone were freeze dried and homogenized using a Retsch Cryomill (Retsch Inc, Newton, PA). Dried homogenized bone samples, with femur and rib combined as a representative sample of bone (Brookens et al., 2008) were stored at room temperature in a polyethylene Whirlpak® until analysis. Since these samples were collected and processed as a part of another study compact and spongy bone were not separated (Correa et al., 2014) and thus all modern mammal specimens in this study represent combined compact and spongy bone homogenates. The modern, homogenized Steller sea lion samples were used in this study to evaluate methods of collagen extraction and to compare [THg] measurements between different direct mercury analyzer models.
Lastly, modern Pacific cod vertebrae (n = 3) from commercial fisheries catches between 2013 and 2015 (Cyr et al., 2019) were used to examine the effects of peroxide maceration (a common bone preparation method for curation) on measurement of [THg].
Bone preparation
Compact and spongy bone types
To address high variability observed in some early whole bone technical replicates archaeological bone fragments (n = 19) were manually separated into compact and spongy bone to assess difference in [THg] between bone tissue types (Figure 2). Utilizing a small chisel and hammer compact and spongy bone were separated. Transition line between the bone types was avoided. Compact bone fragment was visually inspected to assure no spongy bone was present.
Homogenization of archaeological samples
Many bone samples collected from archeological sites are very small, especially after separating compact from spongy bone. In our early analyses, specimens were not homogenized due to very small nature of the bone fragments available. Our concern was that homogenization result in further sample loss and could limit whether sufficient sample was available; however, there was high variability between technical replicates sampled from the same fragment. We compared the [THg] of non-homogenized bone fragments to [THg] measured in samples of the bone after homogenization to determine the effect of homogenization on precision of [THg] analysis. Compact and spongy bone types from archaeological bone samples were mechanically separated and ground independently in 2 ml polypropylene tubes (Sarstedt, Newton, NC) with two 5 mm steel ball bearings at room temp, 30 Hz for 12 min using a Retsch mixer mill MM 400 (Retsch Inc, Newton, PA). Total Hg was quantified from matched fragment and homogenized bone from 7 individuals with four technical replicates each.
Direct mercury analysis
Total mercury concentration was quantified in dried samples of bone (20 to 60mg) analyzed in triplicate (μg/kg dry weight) on a Milestone DMA-80 (dual cell; Shelton, CT). Samples that had technical replicates with greater than 10% variability were reanalyzed. The practical method detection limit (PMDL; mean of blanks + SD × 5; 0.0876 ± 0.0290 ng) was less than the lowest calibration point and too low to produce reproducible replicate measurements. The second lowest calibration point (1 ng) was conservatively used as the DMA-80 method detection limit (MDL) as this limit resulted in replicates with variability < 10%. With specimen mass of 25 to 60 mg the MDL was 17 to 40 µg/g. Blanks, liquid standards (10 and 100 µg/kg), and certified standard reference materials (SRM; Spinach NIST 1570a, 29.7 ± 2 µg/kg; NRC DORM-4, 412 ± 36 µg/kg) were included in each analytical run. Bone meal (NIST SRM 1486; reference value 2.3 ± 1.4 µg/kg was initially included as a matrix matched reference material; however, [THg] was below the detection limit of the DMA-80 so use of this SRM was discontinued with this instrument.
Percentage recoveries were 97.4 ± 4.30% (90 to 105%, 100 μg/kg calibration standard), 96.5 ± 5.8% (88.2 to 110%, DORM-4), and 98.6 ± 10.2% (82 to 114%, spinach).
Milestone DMA-80 and Nippon MA-3000 comparison
After the initial pilot study was completed on the DMA-80, we gained access to a Nippon MA-3000 (Shibuya City, Tokyo) Direct Mercury Analyzer which has a lower reported detection limit. According to the manufacturer the Nippon method detection limit was less than 0.001 ng with a practical quantitation limit between 0.01 to 0.02 ng. Although we intended to run all remaining bone samples on the new instrumentation, it was important to conduct a comparison between these instruments to confirm that analyses on archaeological bone fragments included in the pilot study were comparable and could be included in our overall study.
Archived samples of modern bone homogenates (n = 6) from a previous study (20 to 45 mg; Correa et al., 2014) were analyzed to compare [THg] quantification between the DMA-80 and Nippon MA-3000. Ten technical replicates were run for each homogenized specimen on each mercury analyzer. Technical replicate mean, standard deviation, and percentage relative standard deviation (%RSD) was calculated for each specimen. For comparison, values [mean ± SD (%RSD)] for low and high concentration specimen were reported in the results section for each analyzer.
Both the less conservative technical method detection limit (TMDL; mean of blanks + SD × 3) and more conservative PMDL were calculated for the DMA-80 and Nippon instruments. The PMDL was similar to the lowest calibration point (0.05 ng) and was used as the method detection limit that resulted in replicates with variability consistently < 10% for all subsequent analyses. Blanks, liquid standards and certified SRM as listed above were included in each analytical run.
Nippon MA-3000 percentage recoveries were 109.3 ± 0.1% (10 μg/kg calibration standard), 107.6 ± 0.8% (100 μg/kg calibration standard), 105.5 ± 3.8% (DORM-4), 85.9 ± 1.6% (spinach), and 77.8 ± 4.1% (bone meal). Importantly, although the recovery of bone meal (matrix match SRM) was low, based on the recovery SD, the measure was consistent. Further, the mean concentration of 1.75 μg/kg is within the reported acceptable range for this SRM is 3.7 to 0.9 μg/kg. DMA-80 percentage recoveries were 92.9 ± 7.6% (10 μg/kg calibration standard), 103.4 ± 1.0% (100 μg/kg calibration standard), 101.3 ± 2.6% (DORM-4), 74.0 ± 0.6% (spinach), and 76.1 ± 27.1% (bone meal). Although, the mean concentration of bone meal quantified on the DMA-80 was within acceptable range (1.75 μg/kg) the large SD may indicate imprecision at low concentrations.
Testing the impact of bone preparation techniques commonly used in archeology
Collagen extraction
Three preparation methods were tested to determine if archives of previously extracted collagen could be utilized for total mercury quantification. Collagen was extracted from six homogenized modern SSL bone samples (combined rib and femur from Correa et al., 2014) using three extraction methods (Methods 1–3) using a separate subsample for each method. Method 1 only included lipid extraction and demineralization of bone; Method 2 added collagen gelatinization to Method 1 preparations and Method 3 added a filtration step to Method 2 (Misarti et al., 2009; Clark et al., 2017). Lipids were removed from each subsample of bone homogenate (~200 mg) using the 2:1 chloroform:methanol (v/v) procedure described by Bligh and Dyer (1959) and Folch et al. (1957). For all methods, lipid-free samples were demineralized using three sequential 30-minute incubations in 3mL 1N HCl followed by centrifugation (2 minutes at 3,000 rpm) and removal of acidic supernatant. Following acid incubations samples were rinsed 3 times with ultrapure water. For each rinse samples were vortexed for 30 seconds, centrifuged for 2 minutes at 3,000 rpm and supernatant decanted. For Methods 2 and 3, demineralized samples were gelatinized in 20mL glass vials with 10mL ultrapure water and 3 drops of 3N HCl (0.5% HCl v/v), covered and placed in a vortex evaporator at 65°C until samples were completely gelatinized. For Method 3, gelatinized samples were filtered with a 0.45 µm filter. All preparations were frozen and subsequently freeze dried for 48 hours. The percent yield of collagen for each extraction method was calculated as (dry mass of collagen/dry mass of bone) × 100. Both collagen [THg] and the proportion of bone THg recovered in extracted collagen were compared among extraction methods. Collagen [THg] was calculated based on dry mass of extracted collagen (ng THg/g collagen). The proportion of bone THg recovered in collagen was calculated based on the [THg] and total dry mass of both untreated bone and extracted collagen (Table 1).
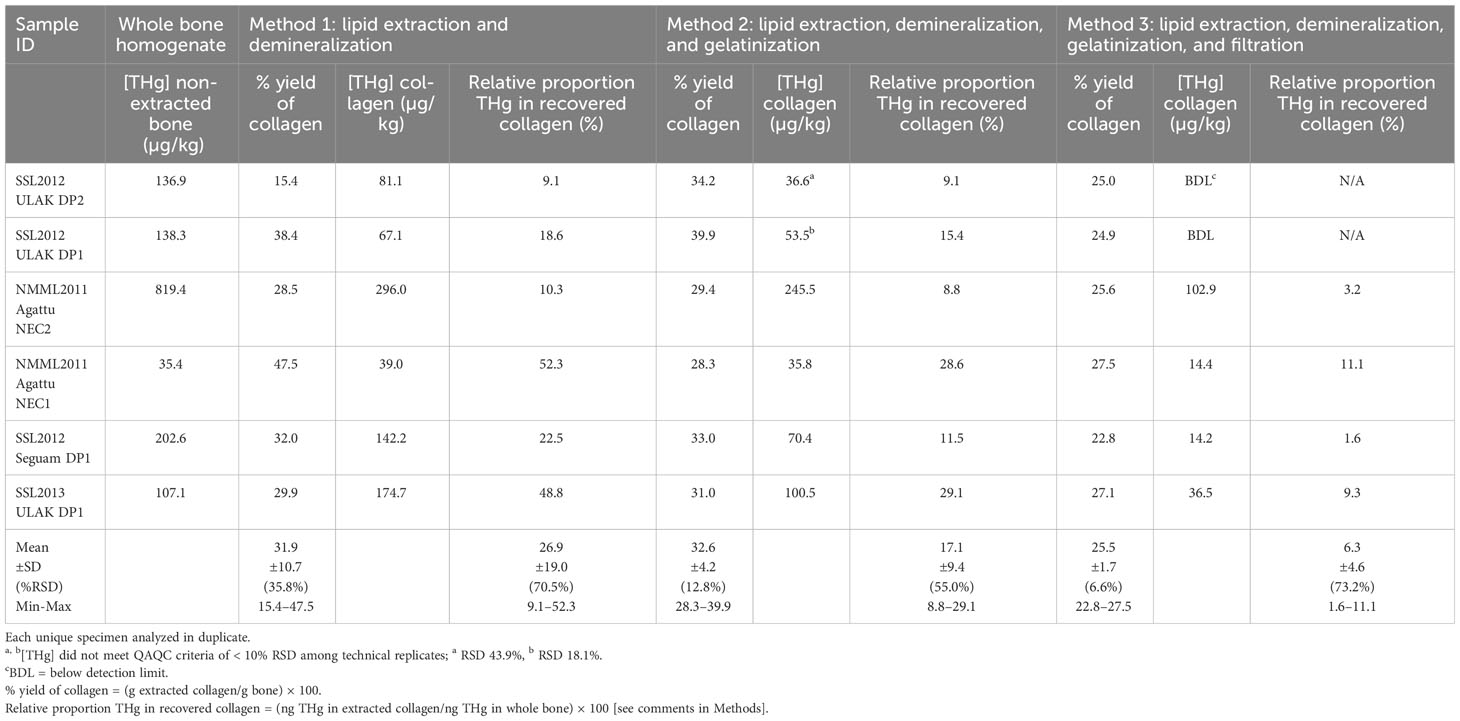
Table 1 Mean total mercury concentration ([THg]; µg/kg dry weight) and relative proportion of THg in whole bone and extracted collagen, using different extraction methods.
Total mercury concentration was quantified in collagen samples (15–35 mg) analyzed in triplicate (μg/kg dry weight) on a Milestone DMA-80 (tri-cell; Shelton, CT). Samples with technical replicates with greater than 10% variability were reanalyzed. The practical method detection limit (mean of blanks + 5 × SD) was less than the lowest calibration point and too low to produce reproducible replicate measurements the second lowest calibration point was conservatively used as the method detection limit as explained above. Blanks, liquid standards (10 and 100µg/kg), and certified standard reference materials (SRM; Spinach NIST 1570a, 29.7 ± 2 µg/kg; NRC DORM-4, 412 ± 36 µg/kg) were included in each analytical run.
Peroxide maceration
Peroxide treatment is a common method to speed maceration and remove soft tissue from bone. Modern cod specimen were utilized to test effects of peroxide maceration on [THg] quantification in bone. Vertebrae were excised from (n = 3) modern cod subsampled from commercial catches in the Aleutian Islands. Ultrapure water (100°C) was poured over vertebrae with attached flesh and allowed to come to room temperature. Maceration vessels were agitated every 12 hours for 48 hours. Loose flesh was manually removed. Once flesh was completely removed from bones two adjacent vertebrae were immersed in either ultrapure water or 3% hydrogen peroxide for 48 hours. Vertebrae were then freeze dried for 24 hours, pulverized, and [THg] quantified with the Milestone DMA-80 (tri-cell; Shelton, CT).
Statistical analyses
All statistical analyses were completed using SAS 9.4 (Cary, NC). Differences were considered statistically significant when p ≤ 0.05. Results are reported as mean ± standard deviation (SD) unless otherwise noted.
Compact and spongy bone types
Differences in [THg]dw between paired compact and spongy bone from the same archeological specimen were analyzed using a Paired T-Test. Homogeneity of variance (HOV) was determined with Levene’s Test.
Homogenization
Proc mixed repeated measures function in SAS was utilized to determine if homogenization of bone specimen was required for precise quantification of bone [THg]. Specimen ID was included as random effect and bone preparation process (homogenization vs. bone fragment), bone tissue type (compact vs. spongy), and the interaction term (process x type) were included in the model with process as the repeated unit. Heterogeneous compound symmetry covariance structure was the most parsimonious model fit as determined by convergence criteria and minimum AIC value. Difference of Least Square means was used to examine differences among process method and bone type on [THg] quantification precision. The model was run once with [THg] as the dependent response variable and again with variance of the 4 technical replicates as the dependent response variable.
Collagen extraction
Given that one-third of the [THg] were BDL for Method 3 (Table 1), data from this method was not included in the analysis. All other [THg] measures were above the detection limit.
Proc mixed repeated measures function in SAS was used to identify differences in percentage yield of collagen, [THg] in collagen (ng/g) and relative proportion of THg in recovered collagen (dependent variables) due to collagen extraction method (independent variables). Percentage yield of collagen was arcsin transformed prior to statistical analysis, and run with an autoregressive covariance structure. Optimal covariance structure model fit was determined by convergence criteria and minimum AIC value. Specimen ID was included as random effect and method of collagen extraction was the repeated unit. Difference of Least Square means was used to determine differences among extraction methods. A one-tailed paired T-test was used to identify differences in [THg] between bone homogenate and collagen from Method 1. In addition, this test was also used to determine if the relative proportion of THg differed between Method 1 and 2. Regression (proc mixed) analyses were completed to examine the relationship between percentage yield of collagen and relative proportion of THg in recovered collagen. Extraction method and the interaction of method and percentage collagen extracted were included in the model.
Peroxide maceration
A paired T-test was used to determine if [THg] differed between water and peroxide maceration techniques.
Results
While direct comparison of [THg] between archaeological and modern time periods was beyond the scope of this study, measures ranged within a similar scale. Total mercury concentrations in archaeological bone specimens ranged from 8 to 267 µg/kg (76.4 ± 68.4 µg/kg) and 27 to 506 µg/kg (145 ± 130.9) in compact and spongy bone, respectively. In modern, homogenized bone samples acquired from a previous study (Correa et al., 2014) [THg] ranged from 35 to 819 µg/kg (240 ± 289.0 µg/kg).
Compact and spongy bone types
A representative specimen illustrating variability in technical replicates of mixed bone types compared with separate analysis of compact and spongy bone is provided (Figure 2). In paired specimen (t18 = −2.99, p = 0.008; unequal variance) spongy bone had greater [THg] compared with compact bone (Figure 3). The pattern of [THg] difference between the two bone types was similar among 17 of the 19 specimens with greater [THg] in spongy bone (145.3 ± 29.3 µg/kg) compared with compact bone (76.4 ± 11.9 µg/kg). However, 2 of 19 specimen had greater [THg] in compact bone. Unfortunately, since modern bone specimen were collected and processed for another study (Correa et al., 2014) it was not possible to separate compact and spongy bone for comparison with modern bone specimen although similar differences between bone types are likely for modern bone as well.
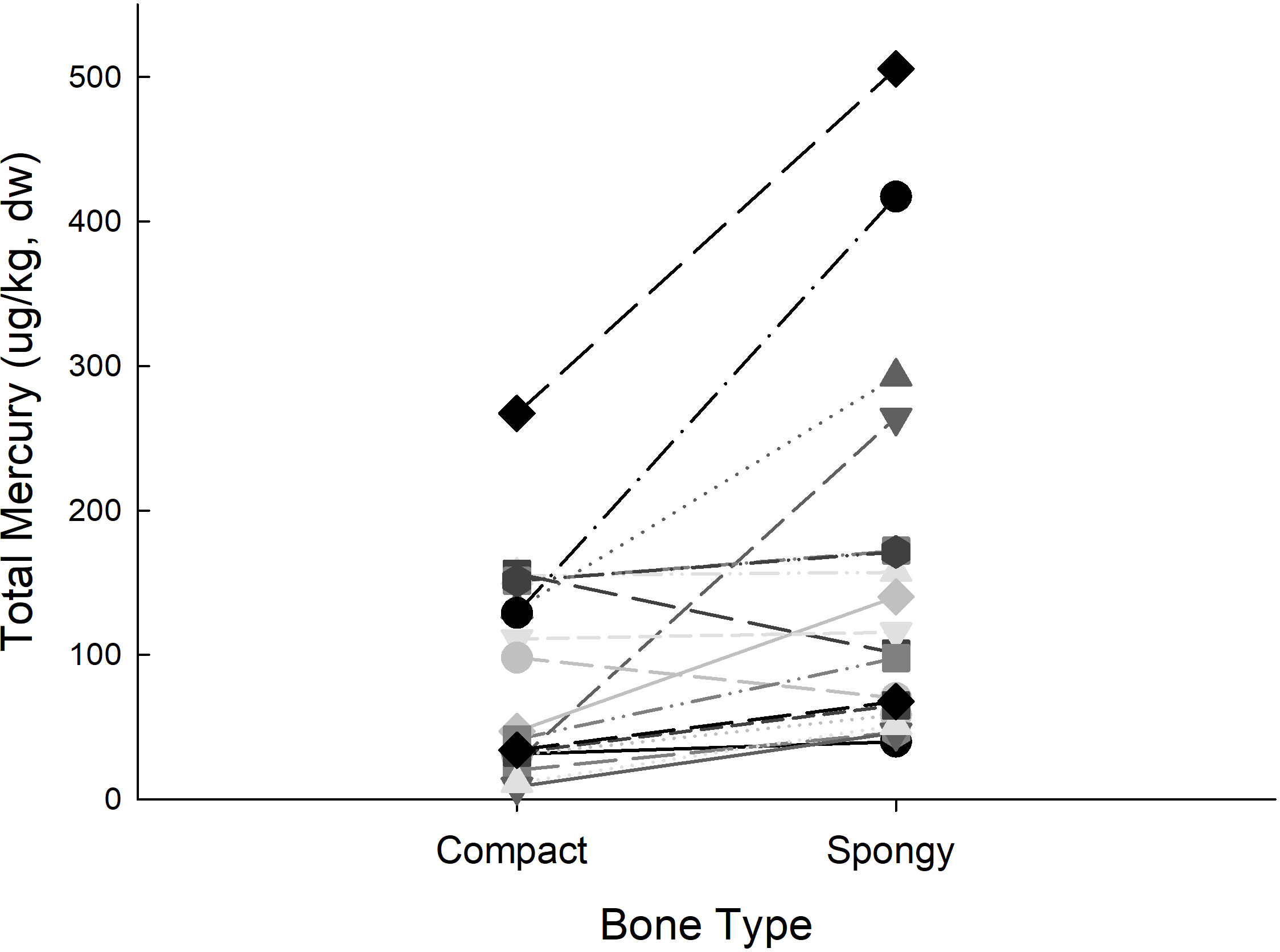
Figure 3 Total mercury concentration (µg/kg, dry weight) of archaeological bone fragments. Paired compact and spongy (trabecular) bone type from the same bone fragment are connected by lines. Despite some individual variability, mercury concentration in spongy bone was significantly greater than compact bone (t18 = −2.99, p = 0.008; unequal variance).
Homogenization
Variance of technical replicates was greater in bone fragments (13.89 ± 15.18 compact bone and 11.10 ± 11.97 spongy bone) compared with homogenized bone (p = 0.006; 0.14 ± 0.29 compact bone and 0.19 ± 0.17 spongy bone). Homogenized specimen had reduced variance compared with whole bone fragments (p = 0.006) while bone type (p = 0.715) and the interaction of bone type and process (p = 0.704) did not impact THg variance among technical replicates.
Milestone DMA-80 and Nippon MA-3000 comparison
Concentrations of THg for (n = 6) modern bone homogenates ranged from a low concentration of 30.1 ± 1.18 (2.38%RSD) and 759.7 ± 31.54 (4.90%RDS) µg/kg for the high concentration specimen utilizing the DMA-80. The same specimen analyzed with the MA-3000 were 38.9 ± 2.75 (2.13%RSD) for the low value to 799.4 ± 17.04 (7.07% RSD) µg/kg for the high concentration specimen. The Nippon MA-3000 consistently reported greater [THg] compared with the identical specimen run with the DMA-80 (t5 = 4.04, p = 0.010; 231.6 ± 6.45 and 210.9 ± 3.87, respectively). Measures of [THg] were highly correlated between the two mercury analyzers with slightly greater values achieved with the Nippon MA-3000 as indicated by a slope of 1.032 (p < 0.001, R2 = 0.9990). Further, the percentage difference between the mean values quantified with the DMA-80 and Nippon MA-3000 were 9.48% within our acceptable range (< 10%) for error among technical replicates.
The TMDL and PMDL for the DMA-80 was 0.0785 ± 0.0198 ng and 0.0876 ± 0.0290 ng, respectively. Sensitivity was improved with the Nippon MA-3000 yielding TMDL 0.0072 ± 0.0036 ng and PMDL 0.0110 ± 0.0054 ng. Given a sample mass of 20mg, based on PMDL the minimum detectable concentration was 6.24 ± 0.99 µg/kg and 0.76 ± 0.18 µg/kg for the DMA-80 and Nippon MA-3000, respectively.
Testing the impact of bone preparation techniques commonly used in archeology
Collagen extraction
The percentage yield of collagen was not significantly different among the 3 extraction methods (p = 0.184) likely due to high variability among the specimen within each method (Table 1). While Methods 1 and 2 produced similar mean collagen yields the results from Method 1 were highly variable, ranging from 15-48% (%RSD 36%). While Method 3 appeared to produce the most consistent yields, except for one individual it also produced the lowest yields (25.5 ± 1.7%).
While [THg] was higher in homogenized bone compared to Method 1 in most individuals (4 of 6), it was not always the case and there was no significant difference in [THg] between homogenized bone and Method 1 extracted collagen (t5 = 1.325, p = 0.242). Similarly, Method 2 had less [THg] than homogenized bone; however, due to small sample size and large variance this difference was not statistically significant (p = 0.066). Method 3 but was not included in the [THg] analysis since 2 individuals had [THg] BDL. The relative proportion of bone THg recovered in collagen was significantly lower using Method 2 compared with Method 1 (p = 0. 0.030). While collagen yield was positively associated (p = 0.036, r2 = 0.2465) with of proportion of THg recovered, the low r2 indicates that very little of the variance in [THg] was explained by collagen yield.
Peroxide maceration
Concentrations of THg were reduced in matched peroxide treated modern cod vertebrate (5.0 ± 0.4ng/g) compared with vertebra macerated with only water (10.2 ± 0.4 µg/kg; t = 7.91, p = 0.016). Percentage difference between the two groups indicated a 51.1 ± 4.9% decline in [THg] with peroxide treatment.
Discussion
Bone provides a stable, and accessible medium to examine millennial-scale variability in environmental Hg (Murray et al., 2015; Álvarez-Fernández et al., 2020). In this study we have shown that peroxide cleaning and collagen extraction are not required, and may be detrimental for optimal Hg quantification in bone. Further, while homogenization of distinct compact and spongy bone did not impact concentration determination, variance of technical replicates was lower improving quantitation precision. Most importantly, significant differences between compact and spongy bone exist within some individual specimen; however, the difference is not consistent and may indicate differential Hg exposure windows influenced by turnover rate of bone types.
Compact and spongy bone types
Data from the current study demonstrated differences in [THg] between compact and spongy bone. Previous human bone studies determined that spongy bone had greater quantity and variability in [THg] compared with compact bone in most individuals (Rasmussen et al., 2013; Zioła-Frankowska et al., 2017), although there was considerable individual variability. In this study most individuals had greater [THg] in spongy compared with compact bone; however, several individuals had either no difference between bone types or compact with greater concentrations. Separate analyses of these bone types prior to [THg] analysis is recommended to avoid high variability that could result among technical replicates based on the proportion of spongy and compact tissue included in each replicate.
Manolagas (2000) reports that in humans, complete turnover of compact bone occurs approximately every 30 years, while spongy bone rapidly remodels and completes turnover in 3 to 4 years. Rasmussen et al. (2013) proposed that these differences in the turnover time of compact versus spongy bone potentially provide two different temporal windows to explore past ecological or physiological impacts through analysis of a single bone sample. These different temporal windows should be considered in future analyses of [THg] and stable isotopes in bone. This would be particularly applicable when investigating changes in individual foraging pattern (e.g., change in trophic position), ontogenetic shifts in diet, or alterations in habitat selection of animals that would contribute to the amount of Hg an individual is exposed to in their diet (Chételat et al., 2020).
Homogenization
Due to the low [THg] in bone and high variability in the distribution of Hg throughout the bone, even within a single bone type, [THg] analyses of intact bone fragments were highly variable. The high variability among technical replicates led to replicates outside of the identified QAQC standard (RSD ≤ 20%). Due to the limited mass of archaeological fragments this resulted in a number of study individuals dropped from statistical analysis. Homogenization reduced the variance of technical replicates for THg determination and improved the precision of analyses. Thus, homogenization resulted in retention of a larger number of individuals in the study. We strongly recommend separate homogenization of compact and spongy bone types to improve precision and RSD of technical replicates. Other tissues such as liver are also known to have heterogenous distribution of THg due to the formation and sequestration of Selenium–Hg complexes (tiemannite) as a detoxification method. Although the concentrations of THg are low in bone, given the high percentage of total body volume and mass, bone could be an important location for Hg sequestration (Correa et al., 2014). The subcellar and extracellular location as well as molecular composition of mercury in bone remains an understudied topic.
Milestone DMA-80 and Nippon MA-3000 comparison
While both direct mercury analyzers were able of quantifying low [THg] found in bone there were some differences worth noting. Bone specimen were often close to the MDL with increased %RSD when run on the DMA-80. Further, utilization of matrix matched bone meal was not possible on the DMA-80 due to low recovery and high variability among replicates. The reduced sensitivity of the DMA-80 favors use of the more sensitive Nippon MA-3000 when quantifying specimen of low concentration and limited mass, such as highly valuable archaeological specimen.
Most previous research investigating bone [THg] utilized cold vapor atomic absorption (Rasmussen et al., 2013) or mass spectrometry methods (Kepa et al., 2012). While these methods are highly sensitive, they are expensive and require substantial time, effort, and caustic chemicals needed for chemical digestion. Direct Mercury Analysis offers an alternative analytical technique with high sensitivity and minimal specimen processing. Laser ablation techniques have also been utilized for quantification of heavy metals in various tissues (Pornwilard et al., 2013). Due to the high variability of [THg] throughout the bone matrix found in this study we would caution the use of laser ablation techniques, preferring the homogenization of the bone fragment before analysis.
Testing the impact of bone preparation techniques commonly used in archaeology
Collagen extraction
We investigated whether [THg] in extracted collagen was predictive of [THg] of intact bone fragments to determine if extensive archival collections of collagen extracted from archeological bone for stable isotope analysis could assist our study. Investigation of three different collagen extraction methods determined collagen did contain a large portion of THg quantified in bone; however, as evidenced by the greater [THg] in whole bone homogenates, either collagen extraction methods resulted in liberation of some mercury from collagen or mercury is associated with other bone components.
The degree of processing (acid demineralization and gelatinization, and filtering) impacted collagen yield (percentage). Improved collagen yield did not improve Hg detection and the proportion of collagen in the bone tissue was not correlated with [THg]. Given that Hg associates with cystine rich proteins, such as collagen, it is possible that the acidic collagen extraction methods liberated some forms of Hg from the cysteine residues (Jalilehvand et al., 2006). Interestingly, archaeology studies have found an inverse relationship with degree of mineralization (hydroxyapatite quantity) and mercury content of bone (Álvarez-Fernández et al., 2022). Young bone or newly remodeled bone generally has more collagen fibers and less mineralization. Given this, a reasonable hypothesis would be that young bone would have greater collagen and [THg] compared with bone from older animals. All the bone tested in this study was from young pups. The large variation in collagen percentage was surprising. This may be due to the semi-quantitative nature of the collagen extraction methods or the very small sample size. Regardless, the relationship between collagen content of bone, degree of bone mineralization, and [THg] should continue to be explored.
Peroxide maceration
Although peroxide maceration is commonly used to deflesh bone, we caution against using peroxide in studies measuring mercury in bone. More than 50% of the mercury quantified in cod vertebrae that was defleshed with only nanopure water was liberated during peroxide maceration. Similar to the results of this study, previous in-vitro studies found an increase in mercury ions liberated from dental amalgam following treatment with hydrogen peroxide which is often used as a dental whitening agent (Al-Salehi et al., 2007).
Summary & conclusions
This study demonstrated that direct mercury analysis was a robust method to quantify small quantities of mercury accurately and precisely in small (20 mg) quantities of bone with minimal specimen processing improving analysis efficiency and reducing overall cost. Compared with the Milestone DMA-80, the Nippon MA-3000 provided 10-fold greater sensitivity determined by PMDL with very small sample size, 20mg. This is a significant advantage when quantifying low concentration specimen such as bone and rare specimen where sample conservation is essential. Homogenization of bone specimen improves precision of [THg] measures.
Different extraction methods did not result in consistent yields in collagen, [THg] in collagen, or proportion of collagen extracted from bone, making reported [THg] in collagen suspect as representing the actual [THg] in native collagen. The relationship of bone collagen and mercury is intriguing and warrants further investigation. Importantly, while many studies avoid quantification of [THg] in spongy bone, differences between compact and spongy bone within an individual may provide a unique bio-monitor of decadal change in environmental mercury given the differences in turnover rate. Archaeological bone is an extremely useful medium to examine long term variability in environmental mercury dynamics over millennial time scales.
Data availability statement
The raw data supporting the conclusions of this article will be made available by the authors, without undue reservation.
Ethics statement
Ethical approval was not required for the study involving animals in accordance with the local legislation and institutional requirements because no live animals were handled or sampled as a part of this study. Archaeological bone was obtained from village middens of ancestral Unanga with permission from Indigenous community members. Commercial fisheries donated modern cod specimen. Modern otariid bones were collected opportunistically from carcasses found during population counts conducted by our collaborators authorized under MMPA/ESA Permit No. 14325.
Author contributions
JA, NM, LR, TO’H and JC contributed to conception, design and funding of the study. JA and JC performed the statistical analysis. JA wrote the first draft of the manuscript. LR, JC, TO’H, NM, CF, MK, AG wrote sections of the manuscript. All authors contributed to manuscript revision, read, and approved the submitted version.
Acknowledgments
We gratefully thank Sanak Corporation, Pauloff Harbor Tribe, and the Aleut Corporation for providing excavation and archaeological faunal sampling permission. We thank Casey Clark for completion of the collagen extraction procedures and Stephanie Crawford for quantification of collagen [THg] as well as database management. Modern bone specimen collection was approved under MMPA Permit number 14325 held by Alaska Department of Fish & Game (ADF&G). Kiska archaeological excavation approved under ARPA Permit # R7(MAR)09-04; Research and Monitoring Special Use Application and Permit from the Alaska Maritime National Wildlife Refuge; Permit to Conduct Archaeological Research Permit #02-14 issued by the Aleut Corporation. Research funding was provided by a NOAA cooperative research agreement Award Number NA15NMF4390168 issued to Rea, O’Hara, and Misarti. Support for manuscript preparation was provided by NSF-ARCSS-ASSP collaborative award 1935816 Avery, Misarti, Rea, and 1935823 Funk.
Conflict of interest
The authors declare that the research was conducted in the absence of any commercial or financial relationships that could be construed as a potential conflict of interest.
Publisher’s note
All claims expressed in this article are solely those of the authors and do not necessarily represent those of their affiliated organizations, or those of the publisher, the editors and the reviewers. Any product that may be evaluated in this article, or claim that may be made by its manufacturer, is not guaranteed or endorsed by the publisher.
Supplementary material
The Supplementary Material for this article can be found online at: https://www.frontiersin.org/articles/10.3389/fevo.2023.1251282/full#supplementary-material
References
Al-Salehi S. K., Hatton P. V., McLeod C. W., Cox A. G. (2007). The effect of hydrogen peroxide concentration on metal ion release from dental amalgam. J. Dent. 35 (2), 172–176. doi: 10.1016/j.jdent.2006.07.003
Álvarez-Fernández N., Martínez Cortizas A., López-Costas O. (2020). Atmospheric mercury pollution deciphered through archaeological bones. J. Archaeol. Sci. 119, 105159–105159. doi: 10.1016/j.jas.2020.105159
Álvarez-Fernández N., Martínez Cortizas A., López-Costas O. (2022). Structural equation modelling of mercury intra-skeletal variability on archaeological human remains. Sci. Total Environ. 851 (Pt 1), 158015. doi: 10.1016/j.scitotenv.2022.158015
Aubail A., Dietz R., Rigét F., Simon-Bouhet B., Caurant F. (2010). An evaluation of teeth of ringed seals (Phoca hispida) from Greenland as a matrix to monitor spatial and temporal trends of mercury and stable isotopes. Sci. Total Environ. 408, 5137–5146. doi: 10.1016/j.scitotenv.2010.07.038
Ãvila A., Mansilla J., Bosch P., Pijoan C. (2014). Cinnabar in Mesoamerica: poisoning or mortuary ritual? J. Archaeol. Sci. 49, 48–56. doi: 10.1016/j.jas.2014.04.024
Barst B. D., Basu N., Chételat J. (2022). Toxicological risk of mercury for fish and invertebrate prey in the Arctic. Sci. Total Environ. 836, 155702. doi: 10.1016/j.scitotenv.2022.155702
Basu N., Abass K., Dietz R., Krümmel E., Rautio A., Weihe P. (2022). The impact of mercury contamination on human health in the Arctic: A state of the science review. Sci. Total Environ. 831, 154793. doi: 10.1016/j.scitotenv.2022.154793
Basu N., Head J. (2010). Mammalian wildlife as complementary models in environmental neurotoxicology. Neurotoxicol. Teratol. 32, 114–119. doi: 10.1016/j.ntt.2008.12.005
Bligh E. G., Dyer W. J. (1959). A rapid method of total lipid extraction and purification. Can. J. Biochem. Physiol. 37(8), 911–917. doi: 10.1139/o59-099
Brookens TJ, O’Hara TM, Taylor RJ, Bratton GR, Harvey JT (2008). Total mercury body burden in Pacific harbor seal, Phoca vitulina richardii, pups from central California. Mar. Pollut. Bull. 56(1), 27–41. doi: 10.1016/j.marpolbul.2007.08.010
Castellini J. M., Rea L. D., Lieske C. L., Beckmen K. B., Fadely B. S., Maniscalco J. M., et al. (2012). Mercury concentrations in hair from neonatal and juvenile Steller Sea Lions (Eumetopias jubatus): implications based on age and region in this northern Pacific marine sentinel piscivore. Ecohealth 9, 267–277. doi: 10.1007/s10393-012-0784-4
Chastel O., Fort J., Albert C., Angelier F., Basu N., Blévin P., et al. (2022). Mercury exposure and potential health risk for Arctic seabirds and shorebirds. Sci. Tot. Environ. 844, 156944. doi: 10.1016/j.scitotenv.2022.156944
Chételat J., Ackerman J. T., Eagles-Smith C. A., Hebert C. E. (2020). Methylmercury exposure in wildlife: A review of the ecological and physiological processes affecting contaminant concentrations and their interpretation. Sci. Total Environ. 711, 135117. doi: 10.1016/j.scitotenv.2019.135117
Clark C.T., Horstmann L., Misarti N. (2017). Quantifying variability in stable carbon and nitrogen isotope ratios within the skeletons of marine mammals of the suborder Caniformia. J. Archaeol. Sci. Rep. doi: 10.1016/j.jasrep.2017.09.007
Correa L., Rea L. D., Bentzen R., O’Hara T. M. (2014). Assessment of mercury and selenium tissular concentrations and total mercury body burden in 6 Steller sea lion pups from the Aleutian Islands. Mar. pollut. Bull. 82, 175–182. doi: 10.1016/j.marpolbul.2014.02.022
Cyr A., Lopez J. A., Rea L., Wooller M. J., Loomis T., McDermott S., et al. (2019). Mercury concentrations in marine species from the Aleutian Islands: Spatial and biological determinants. Sci. Total Environ. 664, 761–770. doi: 10.1016/j.scitotenv.2019.01.387
Dietz R., Wilson S., Loseto L., Dommergue A., Xie Z., Sonne C., et al. (2022). Special issue on the AMAP 2021 assessment of mercury in the Arctic. Sci. Total Environ. 843, 157020. doi: 10.1016/j.scitotenv.2022.157020
Emslie S. D., Alderman A., McKenzie A., Brasso R., Taylor A. R., Molina Moreno M., et al. (2019). Mercury in archaeological human bone: biogenic or diagenetic? J. Archaeological Sci. 108, 104969. doi: 10.1016/j.jas.2019.05.005
Folch J., Lees M., Sloane Stanley G. H. (1957). A simple method for the isolation and purification of total lipides from animal tissues. J. Biol. Chem., 226(1), 497–509.
Funk C. (2018). Ethno-ornithology in the Rat Islands: Prehistoric Aleut relationships with birds in the western Aleutians, Alaska. J. Anthropol. Archaeol. 51, 144–158. doi: 10.1016/j.jaa.2018.06.001
Grayson D. K. (1984). Quantitative zooarchaeology: topics in the analysis of archaelogical faunas (Orlando, Florida: Elsevier).
Jalilehvand F., Leung B. O., Izadifard M., Damian E. (2006). Mercury(II) cysteine complexes in alkaline aqueous solution. Inorgan. Chem. 45 (1), 66–73. doi: 10.1021/ic0508932
Kepa M., Kozłowski T., Szostek K., Drozd A., Walas S., Mrowiec H., et al. (2012). Analysis of mercury levels in historical bone material from syphilitic subjects–pilot studies (short report). Anthropol. Anz 69, 367–377. doi: 10.1127/0003-5548/2012/0163
Le B. Q., Nurcombe V., Cool S. M., van Blitterswijk C. A., de Boer J., LaPointe V. L. S. (2017). The components of bone and what they can teach us about regeneration. Mater. (Basel). 11 (1), 14. doi: 10.3390/ma11010014
Liu J. L., Xu X. R., Yu S., Cheng H., Peng J. X., Hong Y. G., et al. (2014). Mercury contamination in fish and human hair from Hainan Island, South China Sea: Implication for human exposure. Environ. Res. 135, 42–47. doi: 10.1016/j.envres.2014.08.023
López-Costas O., Kylander M., Mattielli N., Álvarez-Fernández N., Pérez-Rodríguez M., Mighall T., et al. (2020). Human bones tell the story of atmospheric mercury and lead exposure at the edge of Roman World. Sci. Total Environ. 25 (710), 136319. doi: 10.1016/j.scitotenv.2019.136319
Manolagas S. C. (2000). Birth and death of bone cells: basic regulatory mechanisms and implications for the pathogenesis and treatment of osteoporosis. Endocr. Rev. 21, 115–137. doi: 10.1210/edrv.21.2.0395
Misarti N., Finney B., Maschner H., Wooller M. J. (2009). Changes in northeast Pacific marine ecosystems over the last 4,500 years: evidence from stable isotope analysis of bone collagen from archeological middens. The Holocene, 19(8), 1139–1151. doi: 10.1177/0959683609345075
Misarti N., Finney B., Maschner H. (2011). Reconstructing site organization in the eastern Aleutian Islands, AK using multi-element chemical analysis of soils. J. Archaeol. Sci. 38 (7), 1441. doi: 10.1016/j.jas.2011.02.007
Morris A. D., Wilson S. J., Fryer R. J., Thomas P. J., Hudelson K., Andreasen B., et al. (2022). Temporal trends of mercury in Arctic biota: 10 more years of progress in Arctic monitoring. Sci. Total Environ. 839, 155803. doi: 10.1016/j.scitotenv.2022.155803
Murray M. S., McRoy C. P., Duffy L. K., Hirons A. C., Schaaf J. M., Trocine R. P., et al. (2015). Biogeochemical analysis of ancient Pacific Cod bone suggests Hg bioaccumulation was linked to paleo sea level rise and climate change. Front. Environ. Sci. 3, 8. doi: 10.3389/fenvs.2015.00008
Pornwilard M., Weiskirchen R., Gassler N., Bosserhoff A. K., Becker J. S. (2013). Novel bioimaging techniques of metals by laser ablation inductively coupled plasma mass spectrometry for diagnosis of fibrotic and cirrhotic liver disorders. PloS One 8, e58702. doi: 10.1371/journal.pone.0058702
Rasmussen K. L., Skytte L., Jensen A. J., Boldsen J. L. (2015). Comparison of mercury and lead levels in the bones of rural and urban populations in Southern Denmark and Northern Germany during the Middle Ages. J. Archaeol. Sci.: Rep. 3, 358–370. doi: 10.1016/j.jasrep.2015.06.021
Rasmussen K. L., Skytte L., Pilekær C., Lauritsen A., Boldsen J. L., Leth P. M., et al. (2013). The distribution of mercury and other trace elements in the bones of two human individuals from medieval Denmark – the chemical life history hypothesis. Herit. Sci. 1, 10. doi: 10.1186/2050-7445-1-10
Rea L. D., Castellini J. M., Avery J. P., Fadely B. S., Burkanov V. N., Rehberg M. J., et al. (2020). Regional variations and drivers of mercury and selenium concentrations in Steller sea lions. Sci. Total Environ. 744, 140787. doi: 10.1016/j.scitotenv.2020.140787
Song S., Li Y., Liu Q. S., Wang H., Li P., Shi J., et al. (2021). Interaction of mercury ion (Hg2+) with blood and cytotoxicity attenuation by serum albumin binding. J. Hazard. Mater. 412, 125158. doi: 10.1016/j.jhazmat.2021.125158
Swanston T., Varney T., Coulthard I., George G. N., Pickering I. J., Murphy R., et al. (2015). Synchrotron X-ray fluorescence imaging evidence of biogenic mercury identified in a burial in colonial Antigua. J. Archaeol. Sci. 58, 26–30. doi: 10.1016/j.jas.2015.03.006
WHO (2010). “Ten chemicals of major public health concern,” in International Programme on Chemical Safety (Geneva, Switzerland: World Health Organization), 4.
Yamada M. O., Tohno S., Tohno Y., Minami T., Ichii M., Okazaki Y. (1995). Accumulation of mercury in excavated bones of two natives in Japan. Sci. Total Environ. 162, 253–256. doi: 10.1016/0048-9697(95)04435-4
Keywords: archaeology, Aleutian Islands Alaska, direct mercury analyser (DMA), steller sea lions (Eumetopias jubatus), Pacific cod (Gadus macrocephalus), cortical bone, trabecular (spongy) bone tissue
Citation: Avery JP, Castellini JM, Misarti N, Keenan M, Gastaldi A, Funk C, O’Hara TM and Rea LD (2023) Evaluating methods for determining mercury concentrations in ancient marine fish and mammal bones as an approach to assessing millennial-scale fluctuations in marine ecosystems. Front. Ecol. Evol. 11:1251282. doi: 10.3389/fevo.2023.1251282
Received: 08 July 2023; Accepted: 25 September 2023;
Published: 13 October 2023.
Edited by:
Laura Parducci, Sapienza University of Rome, ItalyReviewed by:
Constantin Nechita, National Institute for Research and Development in Forestry Marin Dracea (INCDS), RomaniaInês Martins, University of the Azores, Portugal
Copyright © 2023 Avery, Castellini, Misarti, Keenan, Gastaldi, Funk, O’Hara and Rea. This is an open-access article distributed under the terms of the Creative Commons Attribution License (CC BY). The use, distribution or reproduction in other forums is permitted, provided the original author(s) and the copyright owner(s) are credited and that the original publication in this journal is cited, in accordance with accepted academic practice. No use, distribution or reproduction is permitted which does not comply with these terms.
*Correspondence: Julie P. Avery, jpavery@alaska.edu