The impacts of host traits on parasite infection of montane birds in southwestern China
- 1State Key Lab for Biocontrol, School of Ecology, Sun Yat-sen University, Shenzhen, China
- 2Groningen Institute for Evolutionary Life Sciences, University of Groningen, Groningen, Netherlands
- 3Ministry of Education (MOE) Key Laboratory for Biodiversity Science and Ecological Engineering, College of Life Sciences, Beijing Normal University, Beijing, China
- 4School of Applied Sciences, Edinburgh Napier University, Edinburgh, United Kingdom
- 5Centre for Conservation and Restoration Science, Edinburgh Napier University, Edinburgh, United Kingdom
- 6Institute of Ecology and Evolution, University of Bern, Bern, Switzerland
- 7Department of Zoology, University of Cambridge, Cambridge, United Kingdom
- 8Princeton School of Public and International Affairs, Princeton University, Princeton, NJ, United States
Parasitic infections have the potential to impact the hosts’ body condition, elevate physiological responses, and ultimately lead to increased mortality. Host-parasite interactions are tied to the ecological and life-history traits of the hosts. While montane birds are susceptible to avian blood parasites, few studies have simultaneously assessed how inter- and intra-specific traits of hosts influence their probability of parasite infection. In this study, we screened for avian blood parasites across 214 individuals from 51 species at two sites, including a lowland farmland at 700 m and a highland forest at 2,500 m, in the Gaoligong Mountains in southwestern China. Overall blood parasite prevalence was 53.74%, with divergent species-specific prevalence ranging from 6.25% to 66.67%. We also measured traits indicative of body condition and physiological responses of each sampled individual. Using Bayesian phylogenetic logistic models, we assessed whether parasite infection probability is associated with ecological and life history traits of host species. Larger bird species were more likely to be infected than smaller bird species, and omnivore species showed lower susceptibility than those with other diets such as insectivores and herbivores. In contrast, foraging strata, nest type, and participation in mixed-species flocks of host species did not affect infection probability. We then used a reduced sample of eight species with more than five individuals, to assess the associations between intra-specific infection probability and host body condition, represented by fat and muscle reserves, and acute stress responses measured through breath rate. While infected individuals were likely to have more fat reserves than non-infected individuals, we did not find any association between infection probability and muscle reserve and body mass, nor with breath rate. Our results revealed that at the species level, specific traits (body mass and diet) of host species predict infection probability and implied a potential link at the individual level between enhanced body condition and increased resilience to parasite infection.
1 Introduction
Parasite infection can have severe consequences for wild species, resulting in alterations in their body condition, heightened physiological responses (O’Dwyer et al., 2020), and elevated mortalities among host species (Galen et al., 2022). Consisting of vector-borne parasites belonging to the genera Plasmodium, Haemoproteus, and Leucocytozoon, avian blood parasites (haemosporidian) provide an ideal system to investigate the consequences of parasite infection (Valkiunas, 2004). The life cycles of avian haemosporidian parasites are rather complex, requiring an arthropod vector (Diptera) for sexual reproduction and sporogony, and an avian host for asexual reproduction (Ilgūnas et al., 2019). Therefore, the transmission and prevalence of avian blood parasites can be jointly influenced by abiotic factors and biotic interactions, resulting in various patterns along major environmental gradients (Fecchio et al., 2021; Lau et al., 2023). As global refuges for diverse avifauna, montane ecosystems are particularly susceptible to avian blood parasites, primarily because of rising temperatures in higher elevations and increased rates of deforestation in lowlands due to agricultural conversion (LaPointe et al., 2012; Zamora-Vilchis et al., 2012; Reis et al., 2021; Theodosopoulos et al., 2023). While studies have increasingly looked at how inter-specific traits affect the infection patterns among different species along elevational gradients (Ishtiaq and Barve, 2018; Rodríguez-Hernández et al., 2021; Rodriguez et al., 2021), other studies have also found that intra-specific traits, such as body condition, can explain variability in infection probability within species (e.g., Rooyen et al., 2013). To gain a more comprehensive understanding of the link between species’ traits and parasite infection patterns, it is important to assess how both intra and inter-specific traits of the hosts affect their likelihood of parasite infection in highly heterogeneous montane environments.
Inter-specific variation in parasite prevalence is influenced by both evolutionary relatedness among species and ecological and life-history traits. For instance, parasite susceptibility can be highly phylogenetically conserved among avian hosts in the tropical Andes (Fecchio et al., 2017). Also, host species-specific traits play a significant role in shaping parasite prevalence patterns, considering the fact that some species exhibit higher susceptibility to parasites than others, even within the same environments (Pulgarín-R et al., 2018; Ellis et al., 2020; de Angeli Dutra et al., 2021). Such differences in parasite prevalence have been speculated as consequences of variations in exposure likelihood to haemosporidian vectors, or variations in resistance to infection (Medeiros et al., 2015; Fecchio et al., 2017). Indeed, a range of ecological and life-history traits related to vector exposure have been shown to be associated with parasite prevalence, including body size (Scheuerlein and Ricklefs, 2004), diet preferences (Wilson et al., 2020), foraging strata (Gupta et al., 2020), nest type (Rodriguez et al., 2021), and social tendencies (González et al., 2014), among others. More specifically, larger species, omnivores, lower-strata foragers, and flocking species showed greater blood parasite prevalence than other species (Rodríguez-Hernández et al., 2021; Penha et al., 2023).
Parasite infection probability can also be associated with intra-specific traits, especially those indicating body condition and physiological status of individuals. Typically, an individual is considered to be in good body condition when it possesses a higher body mass, indicating more muscle and/or fat storage, which suggests sufficient food intake despite energy expenditure (Pagani-Núñez et al., 2017; Barnett et al., 2018). Parasite infection can lead to a deterioration in an individual’s overall body condition, including loss of body mass and reduced haematocrit value (Yorinks and Atkinson, 2000; Palinauskas et al., 2008; Asghar et al., 2011). Infection by blood parasites can also increase energy expenditure and reduce oxygen-carrying capacity in the blood of the host individuals (Schall et al., 1982; Yorinks and Atkinson, 2000; Ishtiaq and Barve, 2018). As a response, infected individuals may activate their sympathetic nervous system and hypothalamic-pituitary-adrenal (HPA) axis, leading to elevated levels of hormones (Talbott and Ketterson, 2023) and increased respiratory responses. Breath rate, a repeatable, non-invasive, and less time-consuming measure of stress responses, has been used to study the stress responses of wild birds across diverse environments (Carere and Vanoers, 2004; Liang et al., 2018). While many studies have found increased stress levels in infected individuals by measuring oxidative status (Jiménez-Peñuela et al., 2023) and glucocorticoid hormone levels (O’Dwyer et al., 2020), only a few have examined the relationship between parasite infection and breath rate. These studies, focusing on Common Nightingales (Luscinia megarhynchos) (Marinov et al., 2017) and nestlings of the Common Buzzard (Buteo buteo) (Rinaud, 2023), indicated no notable increase in breath rates among infected individuals. With growing evidence in assessing the impacts of individual-level traits mostly on infection probability within a single species, very few studies have explored how individual-level traits might affect parasite infection patterns in multiple-species communities (but see Barrow et al., 2019; Gupta et al., 2020).
In this study, we investigated the associations between avian hosts’ traits and haemosporidian infection probability at the Gaoligong Mountains, a biodiversity hotspot in southwestern China (Myers et al., 2000). Here, lowlands have been transformed to agricultural areas and human settlement, whereas highland forests have been protected within the nature reserve (Liang et al., 2021). By screening avian blood parasites at two typical habitats (a highland forest site and a lowland farmland site) in this mountain, we assessed how these parasite prevalence patterns were associated with inter- and intra-specific trait variation in the host birds. We predicted that, at the species level, larger birds (Scheuerlein and Ricklefs, 2004) with generalized diets (Wilson et al., 2020), lower foraging strata (Gupta et al., 2020), open nested breeders (Rodriguez et al., 2021), and joining mixed-species flocks (González et al., 2014) would be more likely to be infected by parasites. We also predicted that, at the individual level, infected birds would show poorer body condition and higher physiological responses to acute stress than non-infected individuals (Hatchwell et al., 2001; Schoenle et al., 2017).
2 Materials and methods
2.1 Sampling procedure and body condition measurements
We conducted our study on the eastern slope of the southern part of the Gaoligong Mountains in Yunnan Province, southwestern China, from December 2016 to April 2017, covering both breeding (April) and non-breeding (December and January) seasons of birds in this region (Liang et al., 2020; Liang et al., 2021). Similar to other mountains in the region, high-elevation forest areas are protected by the nature reserve, while low-elevation areas have mostly been converted into agricultural land or occupied by human settlements (Liang et al., 2021). We selected two study sites at different elevations and habitats. One was located at a higher elevation in the evergreen broadleaf forest near Yaojiaping field station (2500 m, 25°58′16.00″N, 98°42′37.70″E), and the other was in a lowland agricultural area near Saige village within the northern Salween River valley (750 m, 25°7′38.57″N, 98°51′22.00″E).
At each site, we used mist nets to capture birds from dawn to dusk. We set mist nets with a total length of 70 m, at a height of 3 m above the ground. We conducted hourly inspections of the nets, and carefully extracted all captured birds. This protocol was based on a feasibility analysis of our capacity to properly extract and process all captured individuals. If the number of captured birds was too high, we simply closed the nets to minimize any disturbances caused by this procedure. After extracting an individual bird, we placed the bird into a bag for a minimum of five minutes for it to recover. We first measured the breath rate while handling, to ensure that it was not affected by the following procedures such as measuring and blood sampling. This can systematically decrease individual stress responses, allowing comparison of breath rates between individuals (Carere and Vanoers, 2004). We determined the breath rate by counting the number of respirations in 30 seconds while handling the individual (Liang et al., 2018). We also assessed the pectoral muscle and fat reserves. The muscle and fat scores are commonly used as proxies for assessing body condition (Pagani-Núñez et al., 2017). Specifically, we evaluated muscle reserves using a three-level scale based on the prominence of the sternal keel (Gosler, 1991), and assessed fat reserves using a five-level scale, ranging from 0 (no fat) to 4 (fat filled up to distal portion of interclavicles) (Kaiser, 1993). Before banding and releasing each individual bird, we collected a blood sample of approximately 10 μL by using a sterile 30-gauge needle in a syringe (the needle had a diameter of 0.3 mm; B. Braun Melsungen AG, Germany) to puncture either the brachial vein or the medial metatarsal vein. We subsequently stored these blood samples in absolute (97%) ethyl alcohol at a temperature of -20°C. Finally, we uniquely banded each individual with plastic bands, and included only measurements from their first capture in our analyses. All samplings were completed before dusk. We conducted our sampling for a total of 18 days, including 7 days at Saige and 11 days at Yaojiaping. We obtained blood samples from 99 individuals from 29 different species at Saige and from 115 individuals from 24 species at Yaojiaping, resulting in a total of 214 individuals encompassing 51 bird species (Table S1). Only two species, the Slaty-blue flycatcher (Ficedula tricolor) and the Small niltava (Niltava macgrigoriae), were sampled at both sites (Table S1).
2.2 DNA extraction and parasite screening
Both avian host and parasite DNA were extracted simultaneously using TIANamp Genomic DNA kit (TIANGEN) following its provided protocol. We assessed extraction quality using a Nanodrop 2000 Spectrophotometer (Thermo Scientific). As the low concentration of DNA samples may cause false negatives, we increased the concentration of the DNA samples with levels below 30 ng/mL using Eppendorf Concentrator plus. We then performed nested polymerase chain reaction (PCR) assays targeting the cytochrome b gene of the parasites (Hellgren et al., 2004). This allowed us to detect avian blood parasites including species in Haemoproteus, Plasmodium, and Leucocytozoon (Hellgren et al., 2004). We performed the first PCR, in volumes of 25 μL, comprising 16.8 μL ddH2O, 2.5 μL 10× buffer, 1.5 μL dNTPs (2.5μM), 1 μL primer (HaemNFI–HaemNR3), 0.2 μL Taq DNA polymerase, and 2 μL DNA templates. The temperature profile for each cycle was set at 94°C for 30 s, 50°C for 30 s, and 72°C for 45 s, with 30 repeats. For the second PCR, we used 2 μL of the first run product as the template, replaced the primer with 1 μL HaemF–HaemR2 (for Haemoproteus spp. and Plasmodium spp.), and used 1 μL HaemFL–HaemR3L (for Leucocytozoon spp.) separately. We increased the number of PCR cycles to 35. In each cycle, we retained the same temperature profile and all other settings used in the first PCR. In both PCR assays, we included a negative control sample using ddH2O instead of a DNA template to detect false positives. We confirmed individual infection status by testing each blood sample at least twice to avoid false negatives. To achieve better sequencing quality in some samples, we replaced the primers with HAEMNF-HAEMNR2 in the first run and HAEMF-HAEMR2 in the second run while maintaining the same temperature scheme. These primers amplified an overlapped fragment with the primers described above (Hellgren et al., 2004; Waldenström et al., 2004). All DNA sequencing was subsequently conducted by Tianyihuiyuan Company (Guangzhou).
2.3 Categorizing the infection status of sampled individuals
We categorized the infection status of the sampled individual birds into “infected” or “non-infected” for the three parasite genera combined, and also separately for each parasite genus: Plasmodium, Haemoproteus, and Leucocytozoon. For the three parasite genera combined, we were able to confirm the infection status for all sampled individual birds based on the sequencing results. We classified individuals with positive sequencing results as infected, while those with negative sequencing results as non-infected. We then performed a visual inspection and trimming of the sequences for each infected individual using SeqMan 7 (Swindell and Plasterer, 1997). We identified multi-infections among 21 individuals whose sequencing results containing double phases. As we were unable to confirm the parasite haplotype for these multi-infected individuals, we excluded them when categorizing the infection status for the individual parasite genus (Table S1). We were able to confirm the infection status of individual parasite genus (Plasmodium, Haemoproteus, and Leucocytozoon) for 193 sampled individual birds. We performed alignment of parasite sequences using the default setting of MUSCLE in MEGA 7 (Kumar et al., 2016). We obtained 96 aligned sequences of 367 bp for downstream analyses. We then generated haplotypes of parasites of each infected individual with single infection using DnaSP v6, and confirmed their genus by comparing them with sequences deposited in the MalAvi database (accessed on February 27th 2022, Bensch et al., 2009).
2.4 Phylogenetic analyses
We assessed phylogenetic relationships among the detected parasite haplotype. We obtained 39 unique haplotypes and renamed sequences that had a 100% match in the MalAvi database according to the deposited names. We considered cytb haplotypes differing by one or more base pairs to represent a new lineage. We applied a strict clock model with Yule speciation prior in BEAST v1.10.4 (Drummond and Rambaut, 2007). We compared the AICc values of various nucleotide substitution models and chose the GTR+I+G model as the best-fitting one, based on its lowest AICc value, using jModelTest (Version 2.1.1) (Posada, 2008). We ran for 1,000,000 generations with sampling every 100 iterations. Subsequently, we used Tracer v1.7.1 (Rambaut et al., 2018) to check the convergence in the case that Effective Sample Size (ESS) exceeded 200 and constructed a maximum clade credibility tree using TreeAnnotator v1.10.4 (burn-in = 2,500, node height = median heights) (Drummond and Rambaut, 2007).
2.5 Statistical analyses
We first applied the Bayesian Generalized Multilevel Model (BGMM) to assess how inter-specific traits of the avian hosts influenced their parasite infection probability. We excluded an individual Collared owlet (Glaucidium brodiei) from these analyses since this is the only carnivore species in our sampled dataset. In this model, the status of parasite infection of each of the 213 sampled individuals of 50 species was treated as a response variable (binary: coded as 1 for infected individuals, and 0 for non-infected individuals). We used the ‘Bernoulli’ family with a logistic distribution. We used default priors, and ran four chains, with 10,000 iterations per chain. In each chain, the first 5,000 iterations were used as burn-in and were discarded. We accounted for phylogenetic relatedness among 50 species by incorporating a covariance matrix among species as a random effect. To do this, we pruned 10,000 phylogenetic trees for these species from a global dataset (birdtree.org) (Jetz et al., 2012), and subsequently computed a Max Clade Credit tree for further analyses using the “maxCladeCred” function in the R package “phangorn” (Schliep, 2011). As predictors, we included a range of ecological and life history traits for each species, encompassing body mass, nest type (categorized as open vs. closed nests), diet (classified as omnivores, insectivores, or herbivores), foraging strata (ranked from 1 to 5 representing ground, understory, middle-high, canopy and aerial), and mixed-species flock tendency (mixed-species flocker vs. non-flocker). We calculated the mean body mass for each species at each site based on our own measurements. We obtained the nest type (Billerman et al., 2020), diet (Wilman et al., 2014), and foraging strata (Wilman et al., 2014) from global datasets. Additionally, we checked the mixed-species flock tendency for each species from a published dataset pertaining to birds in our region (Zhou et al., 2019). We also included the site as a random effect to account for infection differences of species between the two sites. We conducted similar analyses for three parasite genera separately.
Then, we used a BGMM to assess the association between intra-specific traits and the parasite infection probability of individual birds. We were interested in exploring how the body condition and physiological responses of individuals related to variations in infection probability within species. Thus, we retained species with more than five individuals from our analyses, resulting in a dataset of 121 individuals from eight species. We included, species, and phylogeny as random variables in the model to control for the interspecific trait variation. We also included site as random effect to account for variation explained by environmental effects. The status of parasite infection for each individual bird was again modeled as a binary response variable. We included four individual-level traits as predictors. These included three traits indicative of the body condition of individual birds, encompassing body mass, fat and muscle reserves; as well as one trait serving as a proxy of acute stress response, namely breath rate. To test the potential influence of sampling time on breath rate, we extracted the residuals from a separate linear mixed-effects model, in which breath rate was included as a response variable, and time of day (number of hours since dawn) as a predictor, together with species identity and site as the random effects, following Liang et al. (2018). We standardized the residual breath rate, and another continuous variable, body mass, to have a mean of 0, and a standard deviation of 1.
We used “brms” package (Bürkner, 2017) to run our BGMM, “nlme” package (Pinheiro et al., 2017) to run linear mixed effects model, and ggplot2 (Wickham et al., 2020) for visualization in R software v4.2.1 (R Core Team, 2019). In each model, we considered variable(s) with 95% credible intervals (CI) that did not overlap with zero as statistically significant.
3 Results
3.1 Infection prevalence and parasite haplotypes
Among 214 individual birds screened, 53.74% tested positive for blood parasites. The prevalence of haemosporidian at the lowland agriculture site was 69.70% (69 out of 99 sampled individuals), and 40.00% (46 out of 115 sampled individuals) at the highland forest site. When categorized by season, the prevalence during breeding and non-breeding season was 38.64% (17 out of 44 individuals) and 57.65% (98 out of 170 individuals), respectively.
Prevalence varied among species, from 6.25% in the Green-tailed Sunbird (Aethopyga nipalensis) (N = 16 individuals) to 66.67% in the Scaly-breasted Munia (Lonchura punctulata) (N = 24 individuals) (Table S1). The prevalence of Haemoproteus, Plasmodium, and Leucocytozoon were 16.36% (highland forest site: 2.61%, lowland agricultural site: 32.32%), 16.36% (highland forest site: 2.61%, lowland agricultural site: 31.31%), and 11.68% (highland forest site: 17.39%, lowland agricultural site: 6.06%), respectively. We found multi-infections in 21 (out of 214, 9.81%) individuals from nine species (Table S1). These samples were discarded in the following analysis.
We detected 39 parasite lineages, including 11 Haemoproteus lineages, 10 Plasmodium lineages, and 18 Leucocytozoon lineages (Figure 1). Among the 39 detected lineages, 43.59% (17 lineages) were found only in highland forest birds and 51.28% (20 lineages) found only in lowland farmland birds. Only 5.13% (two lineages) were found in both sites, and which were recorded in different species (Table S1). The prevalence of Haemoproteus, Plasmodium, and Leucocytozoon were 18.13% (highland forest site: 3.12%, lowland agricultural site: 32.65%), 18.13% (highland forest site: 4.17%, lowland agricultural site: 31.63%), and 13.47% (highland forest site: 20.83%, lowland agricultural site: 6.12%), respectively. We detected 21 new lineages that have not been previously reported in the MalAvi database, including five Haemoproteus lineages, three Plasmodium lineages, and 13 Leucocytozoon lineages (Table S1).
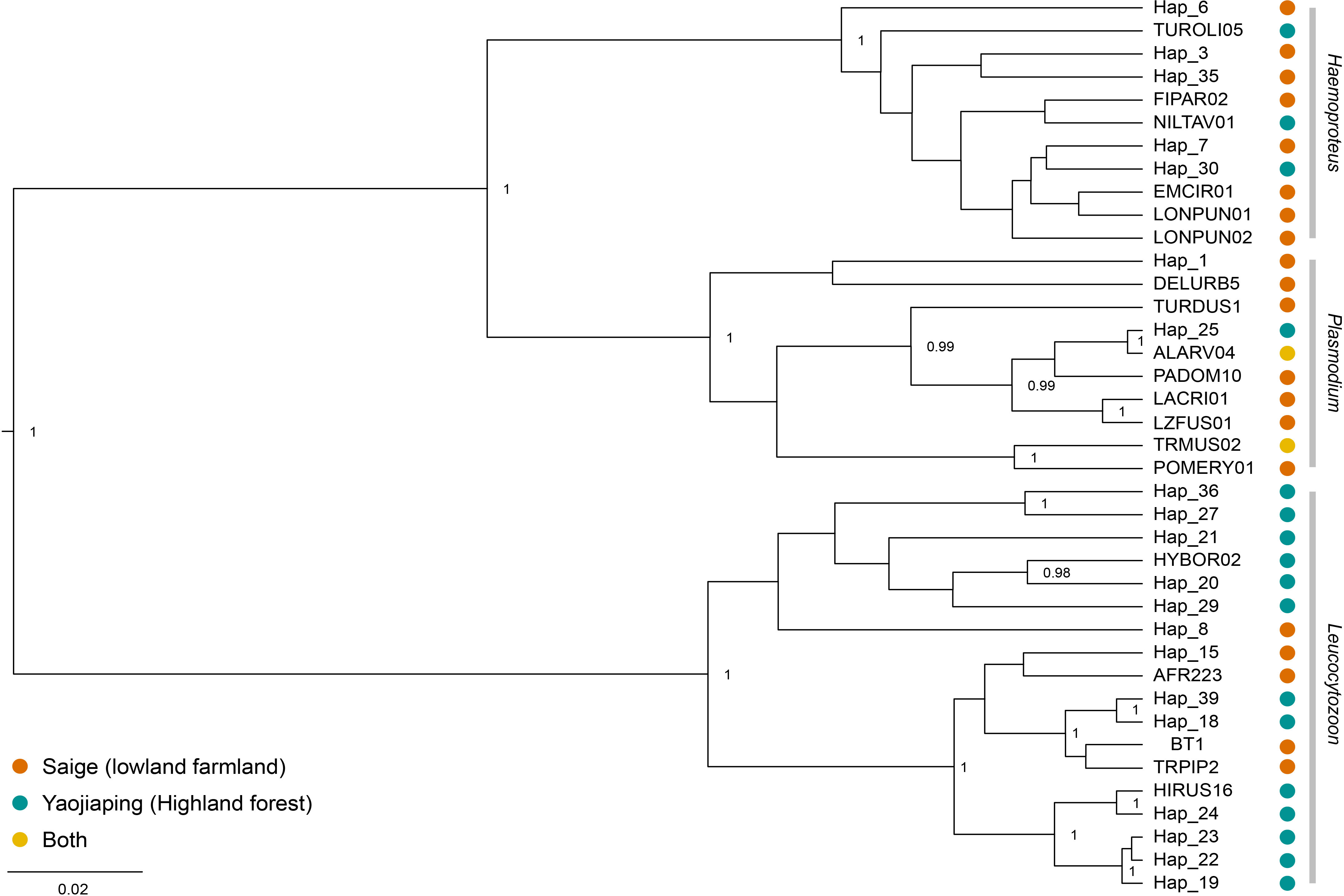
Figure 1 Phylogenetic relationships between parasite haplotypes detected in forest and farmland birds at the Gaoligong Mountains in southwestern China. The parasite haplotype found only in lowland farmland or highland forest was indicated by blue or red circle, respectively. The yellow circle indicates the parasite haplotype found in both sites.
3.2 Effects of species’ ecological and life-history traits
For three parasite genera, two key traits, body mass and diet, are important traits in explaining inter-specific differences in infection probability (Figure 2). Larger species were more likely to be infected than smaller species (Table S2). The infection probability in omnivore species was significantly lower than that of herbivore and insectivore species (Table S2). In contrast, nest type, foraging strata, and the tendency to join mixed-species flocks did not show any significant associations with infection probability (Figure 2, Table S2). These patterns were primarily driven by the infection of Plasmodium (Table S2). No significant associations were recorded between any of these traits and the infection probabilities of Haemoproteus and Leucocytozoon (Table S2).
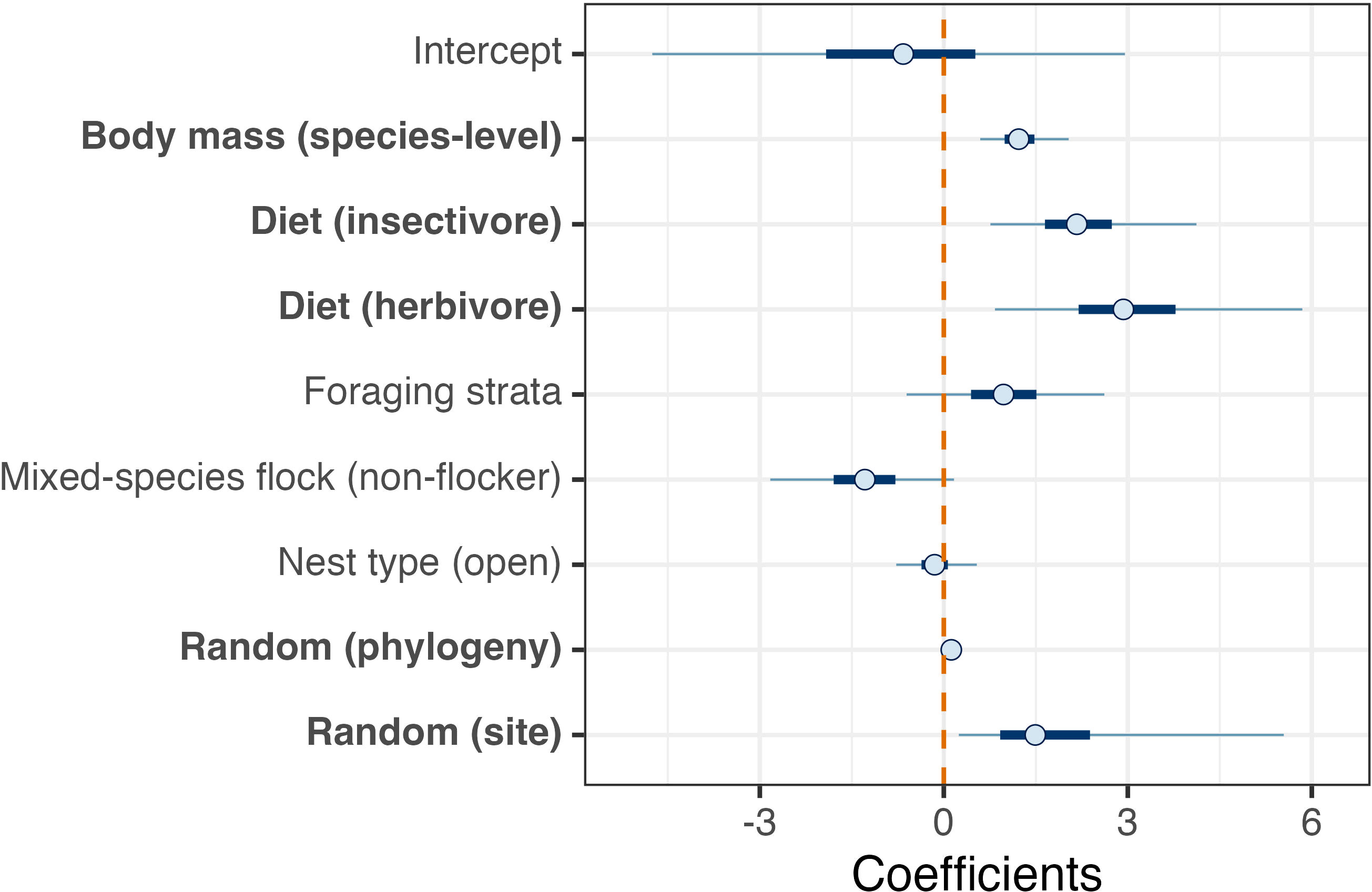
Figure 2 Impacts of inter-specific traits of avian hosts on overall parasite infection probability. Significant effects (95%CI of coefficients do not overlap with zero) are marked in bold. Detailed model results can be found in Table S2.
3.3 Effects of individuals’ body condition and stress responses
For the eight species with more than five individuals (marked in bold in Table S1; see Figure S1 for the prevalence of individual species), we detected an overall parasite prevalence of 58.68% (71 out of 121 individuals). Among the three individual traits related to body condition, fat reserves, but not body mass and muscle reserves, was the important factor in explaining variations of intra-specific differences in infection probability (Figure 3). The infected individuals were those with better body condition, as represented by greater fat reserves (Table S3). In contrast, there was no significant association between individual infection probability and stress response, as indicated by breath rate (Figure 3).
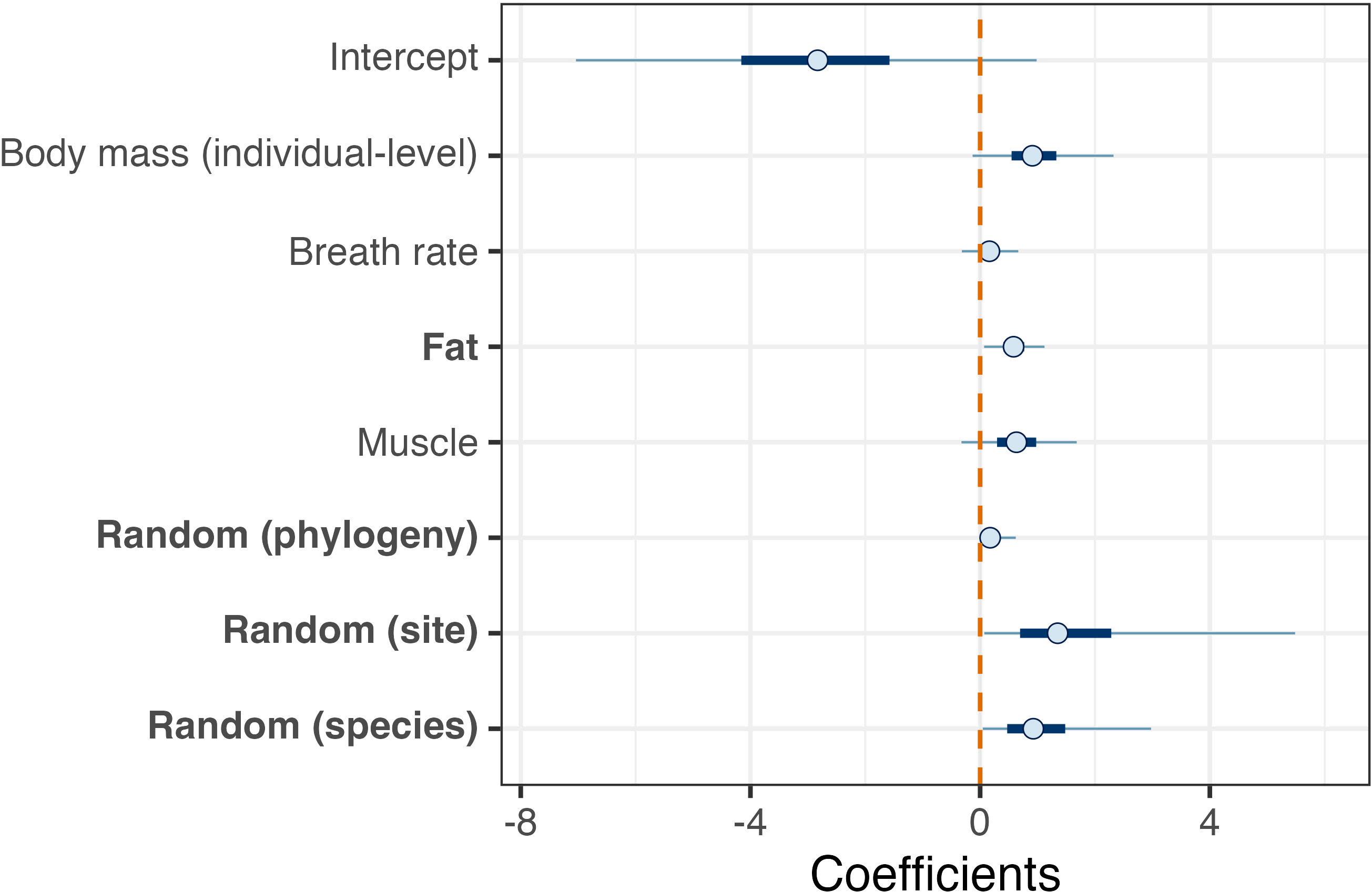
Figure 3 Impacts of intra-specific traits of avian hosts on overall parasite infection probability. Significant effects (95%CI of coefficients do not overlap with zero) are marked in bold. Detailed model results can be found in Table S3.
4 Discussion
In this study, we assessed how host traits influenced inter- and intra-specific variation in susceptibility to parasite infection in two typical habitats within a biodiversity hotspot in southwestern China. Smaller bird species and omnivores exhibited lower susceptibility to parasite infections when compared to larger species and those with specialized diets such as insectivores and herbivores. Interestingly, among individuals of common species, infected individuals tended to show better overall body condition, as indicated by their greater fat reserves.
While accounting for phylogenetic and site factors, our inter-specific analyses have indicated an association between diet, body mass, and the likelihood of species being infected. As anticipated and consistent with previous research (Scheuerlein and Ricklefs, 2004; Filion et al., 2020), our findings demonstrate that larger species tend to have a higher probability of infection. Body mass may influence infection probability at the species level in two ways. First, larger host species often emit more carbon dioxide, which serves as a cue for dipteran vectors seeking hosts and may attract them more to larger species (Estep et al., 2012; Gutiérrez-López et al., 2019). Secondly, larger host species are more visually conspicuous, which could make them more susceptible to be attacked by haemosporidian vectors. Contrary to our initial prediction, omnivore species exhibited a lower infection probability compared to insectivores and herbivores. This pattern could be partially attributed to the prevalence of omnivores with low infection rates in the highland forest site. Among the 43 omnivore individuals in our study, 37% (16 individuals) belonged to the Green-tailed Sunbird, which is an abundant species in our highland forest site. Notably, this species showed a very low infection probability, with only one out of 16 individuals being infected. Potential confounding environmental factors, such as low temperature and heavy wind, may limit the abundance of mosquito vectors at higher elevations, resulting in a low parasite prevalence of the omnivores dwelling in those environments.
Contrary to our prediction, we showed that infected individuals are those with better body condition, characterized by greater fat reserves, although there were no differences in body mass and muscle score between infected and non-infected individuals. While a negative relationship between body condition and parasite susceptibility was typically anticipated, this assumption has been challenged by research employing various indicators of body condition (Sánchez et al., 2018; Rodriguez et al., 2021). Our findings aligned with recent studies that have also indicated that individuals with better body condition are more likely to be infected (Jiménez-Peñuela et al., 2019; Gupta et al., 2020). This may suggest that individuals in better condition could exhibit greater tolerance for higher parasite loads, which can be more easily detected, while those in worse body condition might experience higher mortality rates or only be tolerant to lower parasite loads that are more challenging to be detected (Sánchez et al., 2018). Here, we also explored relationship between parasite infection and stress/respiration responses, as measured by breath rate across species in different communities. We did not find a significant association between parasite infection and breath rate, after accounting for the effect of sampling times (Liang et al., 2018). This result, together with Marinov et al. (2017) who observed comparable breath rate between infected and non-infected nightingales, may suggest a lack of respiratory responses to acute restress among chronically parasitized birds (Cornelius et al., 2014). Alternatively, breath rate may not be a reliable measurement for capturing physiological responses to parasite exposure, as other correlational and experimental studies have demonstrated the increased stress responses with parasite exposure using other stress indicators (Schoenle et al., 2017; Krause et al., 2021). Finally, we note that our samples only allow us to assess the relationships between the infection of the overall haemosporidian (i.e., pooled three parasite genera) and breath rate. Additional sampling is required to attain a sufficient sample size for examining this relationship for individual parasite lineages, which may result in varying physiological consequences to the hosts (Fecchio et al., 2020).
Our data did not allow for a statistically comparison of infection probability between the two habitats and among seasons. It is likely that lowland farmland birds (prevalence: 69.70%) had a greater susceptibility to parasite infection than highland forest birds (prevalence: 40.00%). Also, highland forest birds seem more susceptible to Leucocytozoon haplotypes and more likely to harbor multi-infections (19 individuals out of 21 detected). These patterns might have been influenced by both environmental factors and species characteristics. First, compared to higher elevations, the valley in this region has significantly warmer temperatures (Pan et al., 2019), which may facilitate a higher abundance of mosquitoes, and, consequently, a greater probability of encountering mosquito vectors (Garamszegi, 2011; Zamora-Vilchis et al., 2012; Padilla et al., 2017). Supporting this view, previous studies conducted in our study region suggested that both mosquito abundance and richness decrease as elevation rises from 900 to 3,280 m (Sun et al., 2009). Second, similar effects might be caused by open farmland environments and human habituation being close to water sources, resulting in more available mosquito hosts in our lowland farmland site (Li et al., 2017). Third, susceptibility to parasite infection likely differs between farmland and forest birds. We recorded very different species in these two environments and only two species were found in both areas (Table S1). Lastly, Leucocytozoon haplotypes seem to adapt better to cooler environments, with a similar pattern found in the New World bird communities (Reis et al., 2021; Fecchio et al., 2023). Moreover, previous studies suggested higher parasite prevalence during the breeding season than in the non-breeding season (Cosgrove et al., 2008). Our study didn’t aim to assess seasonal pattern of parasite infections due to the unbalanced sampling, although we observed high prevalence in non-breeding season. Future studies with additional samplings in more sites, wider elevational gradient, diverse habitats, and more seasons are needed for a broader understanding of how abiotic environmental factors affect parasite infection patterns.
In summary, our study has identified certain species traits, such as body mass and diet of the host species, as important factors in explaining interspecific differences in infection patterns. Additionally, our intra-specific analyses have highlighted a potential link between improved body condition and increased resilience to high parasite loads. These findings provide further support for studies in other mountain regions demonstrating the influence of host traits, at both the species and individual levels, on interactions between parasites and hosts (Barrow et al., 2019; Gupta et al., 2020). To gain a more comprehensive understanding of how abiotic factors such as elevation, temperature, and rainfall, as well as human disturbances like habitat transformation, jointly influence the dynamics of host-parasite interactions in this biodiverse region, future research should expand its sampling to encompass a broader range of landscapes and elevations. Such efforts will shed further light on the complex ecological interaction between hosts and parasites in montane areas under climate and land use changes. Finally, our study showed high prevalence of parasite infections in lowland farmland birds within an understudied and highly biodiverse region, which is threatened by increasing land-use changes particularly linked to agriculture (Zhang et al., 2014; Liang et al., 2021). More research must be conducted on montane birds’ susceptibility to parasite infection, especially among communities threatened by dramatic land use changes.
Data availability statement
The original contributions presented in the study are publicly available. This data can be found here: GenBank, accession numbers: OR684526-OR684564.
Ethics statement
The animal study was approved by Institutional Ethical Committee of Animal Experimentation of Sun Yat-sen University. The study was conducted in accordance with the local legislation and institutional requirements.
Author contributions
XZ: Conceptualization, Data curation, Formal analysis, Investigation, Methodology, Software, Visualization, Writing – original draft, Writing – review & editing. XH: Methodology, Writing – review & editing. EP-N: Investigation, Validation, Writing – review & editing. QT: Investigation, Writing – review & editing. HH: Investigation, Writing – review & editing. WZ: Investigation, Writing – review & editing. YL: Conceptualization, Data curation, Funding acquisition, Project administration, Resources, Supervision, Writing – review & editing. DL: Conceptualization, Data curation, Formal analysis, Investigation, Methodology, Project administration, Software, Supervision, Visualization, Writing – original draft, Writing – review & editing.
Funding
The author(s) declare financial support was received for the research, authorship, and/or publication of this article. This work is financially supported by the China Scholarship Council (202207720062) for XZ, the National Natural Science Foundation of China (81961128002) , the Fundamental Research Funds for the Central Universities, provided by Sun Yat-sen University (161gpy34) for YL, the Ma Huateng Foundation, the High Meadows Foundation, and the Princeton Open Access Publication Fund Program for DL.
Acknowledgments
We thank Ge Gao, Wande Li, Guansheng Wang, Binqiang Li, and Xu Luo for their assistance to the fieldwork, and Ying Zhou for her help with the lab work.
Conflict of interest
The authors declare that the research was conducted in the absence of any commercial or financial relationships that could be construed as a potential conflict of interest.
The handling editor GS declared a past co-authorship with the author(s) YL.
Publisher’s note
All claims expressed in this article are solely those of the authors and do not necessarily represent those of their affiliated organizations, or those of the publisher, the editors and the reviewers. Any product that may be evaluated in this article, or claim that may be made by its manufacturer, is not guaranteed or endorsed by the publisher.
Supplementary material
The Supplementary Material for this article can be found online at: https://www.frontiersin.org/articles/10.3389/fevo.2024.1305305/full#supplementary-material
References
Asghar M., Hasselquist D., Bensch S. (2011). Are chronic avian haemosporidian infections costly in wild birds? J. Avian Biol. 42, 530–537. doi: 10.1111/j.1600-048X.2011.05281.x
Barnett L. K., Phillips B. L., Heath A. C. G., Coates A., Hoskin C. J. (2018). The impact of parasites during range expansion of an invasive gecko. Parasitology 145, 1400–1409. doi: 10.1017/S003118201800015X
Barrow L. N., McNew S. M., Mitchell N., Galen S. C., Lutz H. L., Skeen H., et al. (2019). Deeply conserved susceptibility in a multi-host, multi-parasite system. Ecol. Lett. 22, 987–998. doi: 10.1111/ele.13263
Bensch S., Hellgren O., PÉrez-Tris J. (2009). MalAvi: A public database of malaria parasites and related haemosporidians in avian hosts based on mitochondrial cytochrome b lineages. Mol. Ecol. Resour. 9, 1353–1358. doi: 10.1111/j.1755-0998.2009.02692.x
Billerman S. M., Keeney B. K., Rodewald P. G., Schulenberg T. S. (2020). Birds of the world (Ithaca, NY, USA: Cornell Laboratory of Ornithology). Available at: https://birdsoftheworld.org/bow/home.
Bürkner P.-C. (2017). brms: An R package for Bayesian Multilevel Models using Stan. J. Stat. Software 80, 1–28. doi: 10.18637/jss.v080.i01
Carere C., Vanoers K. (2004). Shy and bold great tits (Parus major): body temperature and breath rate in response to handling stress. Physiol. Behav. 82, 905–912. doi: 10.1016/S0031-9384(04)00312-9
Cornelius E. A., Davis A. K., Altizer S. A. (2014). How important are hemoparasites to migratory songbirds? Evaluating physiological measures and infection status in three neotropical migrants during stopover. Physiol. Biochem. Zool. 87, 719–728. doi: 10.1086/677541
Cosgrove C. L., Wood M. J., Day K. P., Sheldon B. C. (2008). Seasonal variation in Plasmodium prevalence in a population of blue tits Cyanistes caeruleus. J. Anim. Ecol. 77, 540 –548. doi: 10.1111/j.1365-2656.2008.01370.x
de Angeli Dutra D., Fecchio A., Martins Braga É., Poulin R. (2021). Migratory birds have higher prevalence and richness of avian haemosporidian parasites than residents. Int. J. Parasitol. 51, 877–882. doi: 10.1016/j.ijpara.2021.03.001
Drummond A. J., Rambaut A. (2007). BEAST: Bayesian evolutionary analysis by sampling trees. BMC Evol. Biol. 7, 1–8. doi: 10.1186/1471-2148-7-214
Ellis V. A., Huang X., Westerdahl H., Jönsson J., Hasselquist D., Neto J. M., et al. (2020). Explaining prevalence, diversity and host specificity in a community of avian haemosporidian parasites. Oikos 129, 1314–1329. doi: 10.1111/oik.07280
Estep L. K., McClure C. J. W., Burkett-Cadena N. D., Hassan H. K., Unnasch T. R., Hill G. E. (2012). Developing models for the forage ratios of Culiseta melanura and Culex erraticus using species characteristics for avian hosts. J. Med. Entomol. 49, 378–387. doi: 10.1603/ME11155
Fecchio A., Bell J. A., Williams E. J., Dispoto J. H., Weckstein J. D., de Angeli Dutra D. (2023). Co-infection with Leucocytozoon and other Haemosporidian parasites increases with latitude and altitude in New World bird communities. Microb. Ecol. 86, 2838–2846. doi: 10.1007/s00248-023-02283-x
Fecchio A., Chagas C. R. F., Bell J. A., Kirchgatter K. (2020). Evolutionary ecology, taxonomy, and systematics of avian malaria and related parasites. Acta Tropica 204, 105364. doi: 10.1016/j.actatropica.2020.105364
Fecchio A., Clark N. J., Bell J. A., Skeen H. R., Lutz H. L., de la Torre G. M., et al. (2021). Global drivers of avian haemosporidian infections vary across zoogeographical regions. Glob. Ecol. Biogeogr. 30, 2393–2406. doi: 10.1111/geb.13390
Fecchio A., Ellis V. A., Bell J. A., Andretti C. B., D’horta F. M., Silva A. M., et al. (2017). Avian malaria, ecological host traits and mosquito abundance in southeastern Amazonia. Parasitology 144, 1117–1132. doi: 10.1017/S003118201700035X
Filion A., Eriksson A., Jorge F., Niebuhr C. N., Poulin R. (2020). Large-scale disease patterns explained by climatic seasonality and host traits. Oecologia 194, 723–733. doi: 10.1007/s00442-020-04782-x
Galen S. C., Ray S., Henry M., Weckstein J. D. (2022). Parasite-associated mortality in birds: the roles of specialist parasites and host evolutionary distance. Biol. Lett. 18, 20210575. doi: 10.1098/rsbl.2021.0575
Garamszegi L. Z. (2011). Climate change increases the risk of malaria in birds. Glob. Change Biol. 17, 1751–1759. doi: 10.1111/j.1365-2486.2010.02346.x
González A. D., Matta N. E., Ellis V. A., Miller E. T., Ricklefs R. E., Gutiérrez H. R. (2014). Mixed species flock, nest height, and elevation partially explain avian haemoparasite prevalence in Colombia. PloS One 9, e100695. doi: 10.1371/journal.pone.0100695
Gosler A. G. (1991). On the use of greater covert moult and pectoral muscle as measures of condition in passerines with data for the Great Tit Parus major. Bird Study 38, 1–9. doi: 10.1080/00063659109477061
Gupta P., Vishnudas C. K., Robin V. V., Dharmarajan G. (2020). Host phylogeny matters: Examining sources of variation in infection risk by blood parasites across a tropical montane bird community in India. Parasitol. Vectors 13, 536. doi: 10.1186/s13071-020-04404-8
Gutiérrez-López R., Martínez-de La Puente J., Gangoso L., Soriguer R., Figuerola J. (2019). Effects of host sex, body mass and infection by avian Plasmodium on the biting rate of two mosquito species with different feeding preferences. Parasitol. Vectors 12, 87. doi: 10.1186/s13071-019-3342-x
Hatchwell B. J., Wood M. J., Anwar M. A., Chamberlain D. E., Perrins C. M. (2001). The haematozoan parasites of Common Blackbirds Turdus merula: associations with host condition. Ibis 143, 420–426. doi: 10.1111/ibi.2001.143.4.420
Hellgren O., Waldenström J., Bensch S. (2004). A new PCR assay for simultaneous studies of Leucocytozoon, Plasmodium, and Haemoproteus from avian blood. J. Parasitol. 90, 797–802. doi: 10.1645/GE-184R1
Ilgūnas M., Romeiro Fernandes Chagas C., Bukauskaitė D., Bernotienė R., Iezhova T., Valkiūnas G. (2019). The life-cycle of the avian haemosporidian parasite Haemoproteus majoris, with emphasis on the exoerythrocytic and sporogonic development. Parasitol. Vectors 12, 516. doi: 10.1186/s13071-019-3773-4
Ishtiaq F., Barve S. (2018). Do avian blood parasites influence hypoxia physiology in a high elevation environment? BMC Ecol. 18, 15. doi: 10.1186/s12898-018-0171-2
Jetz W., Thomas G. H., Joy J. B., Hartmann K., Mooers A. O. (2012). The global diversity of birds in space and time. Nature 491, 444–448. doi: 10.1038/nature11631
Jiménez-Peñuela J., Ferraguti M., Martínez-de la Puente J., Soriguer R., Figuerola J. (2019). Urbanization and blood parasite infections affect the body condition of wild birds. Sci. Total Environ. 651, 3015–3022. doi: 10.1016/j.scitotenv.2018.10.203
Jiménez-Peñuela J., Ferraguti M., Martínez-De La Puente J., Soriguer R. C., Figuerola J. (2023). Oxidative status in relation to blood parasite infections in house sparrows living along an urbanization gradient. Environ. Pollut. 316, 120712. doi: 10.1016/j.envpol.2022.120712
Kaiser A. (1993). A new multi-category classification of subcutaneous fat deposits of songbirds. J. Field Ornithol. 64, 246–255. Available at: https://www.jstor.org/stable/4513807.
Krause J. S., Schultz E. M., Angelier F., Parenteau C., Ribout C., Hahn T. P., et al. (2021). Relationships between avian malaria resilience and corticosterone, testosterone and prolactin in a Hawaiian songbird. Gen. Comp. Endocrinol. 308, 113784. doi: 10.1016/j.ygcen.2021.113784
Kumar S., Stecher G., Tamura K. (2016). MEGA7: Molecular evolutionary genetics analysis Version 7.0 for bigger datasets. Mol. Biol. Evol. 33, 1870–1874. doi: 10.1093/MOLBEV/MSW054
LaPointe D. A., Atkinson C. T., Samuel M. D. (2012). Ecology and conservation biology of avian malaria. Ann. N. Y. Acad. Sci. 1249, 211–226. doi: 10.1111/j.1749-6632.2011.06431.x
Lau G. C. F., Class Freeman A. M., Pulgarín-R P., Cadena C. D., Ricklefs R. E., Freeman B. G. (2023). Host phylogeny and elevation predict infection by avian haemosporidians in a diverse New Guinean bird community. J. Biogeogr. 50, 23–31. doi: 10.1111/jbi.14524
Li L., Guo X., Zhao Q., Tong Y., Fan H., Sun Q., et al. (2017). Investigation on mosquito-borne viruses at Lancang River and Nu River watersheds in southwestern China. Vector-Borne Zoonotic Dis. 17, 804–812. doi: 10.1089/vbz.2017.2164
Liang D., He C., Luo X., Liu Y., Goodale E., Pagani-Núñez E. (2018). Breath rate of passerines across an urbanization gradient supports the pace-of-life hypothesis and suggests diet-mediated responses to handling stress. Ecol. Evol. 8, 9526–9535. doi: 10.1002/ece3.4460
Liang D., Liu Y., Gao G., Luo X. (2020). Breeding biology of a high altitudinal Aethopyga sunbird in southwestern China. J. Nat. Hist. 54, 2381–2390. doi: 10.1080/00222933.2020.1845408
Liang D., Pan X., Luo X., Wenda C., Zhao Y., Hu Y., et al. (2021). Seasonal variation in community composition and distributional ranges of birds along a subtropical elevation gradient in China. Divers. Distrib. 27, 2527–2541. doi: 10.1111/ddi.13420
Marinov M. P., Zehtindjiev P., Dimitrov D., Ilieva M., Bobeva A., Marchetti C. (2017). Haemosporidian infections and host behavioural variation: a case study on wild-caught nightingales (Luscinia megarhynchos). Ethol. Ecol. Evol. 29, 126–137. doi: 10.1080/03949370.2015.1102776
Medeiros M. C. I., Ricklefs R. E., Brawn J. D., Hamer G. L. (2015). Plasmodium prevalence across avian host species is positively associated with exposure to mosquito vectors. Parasitology 142, 1612–1620. doi: 10.1017/S0031182015001183
Myers N., Mittermeier R. A., Mittermeier C. G., da Fonseca G. A. B., Kent J. (2000). Biodiversity hotspots for conservation priorities. Nature 403, 853–858. doi: 10.1038/35002501
O’Dwyer K., Dargent F., Forbes M. R., Koprivnikar J. (2020). Parasite infection leads to widespread glucocorticoid hormone increases in vertebrate hosts: A meta-analysis. J. Anim. Ecol. 89, 519–529. doi: 10.1111/1365-2656.13123
Padilla D. P., Illera J. C., Gonzalez-Quevedo C., Villalba M., Richardson D. S. (2017). Factors affecting the distribution of haemosporidian parasites within an oceanic island. Int. J. Parasitol. 47, 225–235. doi: 10.1016/j.ijpara.2016.11.008
Pagani-Núñez E., He C., Wu Y. W., Peabotuwage I., Goodale E. (2017). Foraging in the tropics: relationships among species’ abundances, niche asymmetries and body condition in an urban avian assemblage. Urban Ecosyst. 20, 1301–1310. doi: 10.1007/s11252-017-0682-1
Palinauskas V., Valkiūnas G., Bolshakov C. V., Bensch S. (2008). Plasmodium relictum (lineage P-SGS1): Effects on experimentally infected passerine birds. Exp. Parasitol. 120, 372–380. doi: 10.1016/j.exppara.2008.09.001
Pan X., Liang D., Zeng W., Hu Y., Liang J., Wang X., et al. (2019). Climate, human disturbance and geometric constraints drive the elevational richness pattern of birds in a biodiversity hotspot in southwest China. Glob. Ecol. Conserv. 18, e00630. doi: 10.1016/j.gecco.2019.e00630
Penha V. A., de S., Domingos F. M. C. B., Fecchio A., Bell J. A., Weckstein J. D., et al. (2023). Host life-history traits predict haemosporidian parasite prevalence in tanagers (Aves: Thraupidae). Parasitology 150, 32–41. doi: 10.1017/S0031182022001469
Pinheiro J., Bates D., DebRoy S., Sarkar D., Heisterkamp S., Van Willigen B., et al. (2017). Package ‘nlme.’. Linear Nonlinear Mix. Eff. Models Version 3, 274.
Posada D. (2008). jModelTest: Phylogenetic model averaging. Mol. Biol. Evol. 25, 1253–1256. doi: 10.1093/molbev/msn083
Pulgarín-R P. C., Gómez J. P., Robinson S., Ricklefs R. E., Cadena C. D. (2018). Host species, and not environment, predicts variation in blood parasite prevalence, distribution, and diversity along a humidity gradient in northern South America. Ecol. Evol. 8, 3800–3814. doi: 10.1002/ece3.3785
Rambaut A., Drummond A. J., Xie D., Baele G., Suchard M. A. (2018). Posterior summarization in Bayesian phylogenetics using Tracer 1.7. Syst. Biol. 67, 901–904. doi: 10.1093/sysbio/syy032
R Core Team (2019). R: A language and environment for statistical computing [Computer software manual] (Austria: Vienna).
Reis S., Melo M., Covas R., Doutrelant C., Pereira H., de Lima R., et al. (2021). Influence of land use and host species on parasite richness, prevalence and co-infection patterns. Int. J. Parasitol. 51, 83–94. doi: 10.1016/j.ijpara.2020.08.005
Rinaud T. (2023). Friends or foes? Blood parasite costs and defence abilities in young raptor hosts (Bielefeld: Universität Bielefeld). Available at: https://pub.uni-bielefeld.de/record/2984118.
Rodriguez M. D., Doherty P. F., Piaggio A. J., Huyvaert K. P. (2021). Sex and nest type influence avian blood parasite prevalence in a high-elevation bird community. Parasitol. Vectors 14, 145. doi: 10.1186/s13071-021-04612-w
Rodríguez-Hernández K., Álvarez-Mendizábal P., Chapa-Vargas L., Escobar F., González-García F., Santiago-Alarcon D. (2021). Haemosporidian prevalence, parasitaemia and aggregation in relation to avian assemblage life history traits at different elevations. Int. J. Parasitol. 51, 365–378. doi: 10.1016/j.ijpara.2020.10.006
Rooyen J., Lalubin F., Glaizot O., Christe P. (2013). Altitudinal variation in haemosporidian parasite distribution in great tit populations. Parasitol. Vectors 6, 1–10. doi: 10.1186/1756-3305-6-139
Sánchez C. A., Becker D. J., Teitelbaum C. S., Barriga P., Brown L. M., Majewska A. A., et al. (2018). On the relationship between body condition and parasite infection in wildlife: a review and meta-analysis. Ecol. Lett. 21, 1869–1884. doi: 10.1111/ele.13160
Schall J., Bennett A. F., Putnam R. W. (1982). Lizards infected with malaria: physiological and behavioral consequences. Science 217, 1057–1059. doi: 10.1126/science.7112113
Scheuerlein A., Ricklefs R. E. (2004). Prevalence of blood parasites in European passeriform birds. Proc. R. Soc Lond. B Biol. Sci. 271, 1363–1370. doi: 10.1098/rspb.2004.2726
Schliep K. P. (2011). phangorn: phylogenetic analysis in R. Bioinformatics 27, 592–593. doi: 10.1093/bioinformatics/btq706
Schoenle L. A., Kernbach M., Haussmann M. F., Bonier F., Moore I. T. (2017). An experimental test of the physiological consequences of avian malaria infection. J. Anim. Ecol. 86, 1483–1496. doi: 10.1111/1365-2656.12753
Sun X., Fu S., Gong Z., Ge J., Meng W., Feng Y., et al. (2009). Distribution of arboviruses and mosquitoes in Northwestern Yunnan Province, China. Vector-Borne Zoonotic Dis. 9, 623–630. doi: 10.1089/vbz.2008.0145
Swindell S. R., Plasterer T. N. (1997). “Seqman,” in Sequence data analysis guidebook (Totowa: Springer), 75–89.
Talbott K. M., Ketterson E. D. (2023). Physiological impacts of chronic and experimental Plasmodium infection on breeding-condition male songbirds. Sci. Rep. 13, 13091. doi: 10.1038/s41598-023-38438-6
Theodosopoulos A. N., Spellman G. M., Taylor S. A. (2023). Survey of haemosporidian parasite infections in an endangered high alpine bird. Parasitol. Vectors 16, 67. doi: 10.1186/s13071-023-05667-7
Waldenström J., Bensch S., Hasselquist D., Östman Ö. (2004). A new nested polymerase chain reaction method very efficient in detecting Plasmodium and Haemoproteus infections from Avian Blood. J. Parasitol. 90, 191–194. doi: 10.1645/GE-3221RN
Wickham H., Chang W., Henry L., Pedersen T. L., Takahashi K., Wilke C., et al. (2020). Package ‘ggplot2’: create elegant data visualisations using the grammar of graphics. R Package Version 3.0.0.
Wilman H., Belmaker J., Simpson J., de la Rosa C., Rivadeneira M. M., Jetz W. (2014). EltonTraits 1.0: Species-level foraging attributes of the world’s birds and mammals. Ecology 95, 2027–2027. doi: 10.1890/13-1917.1
Wilson A. G., Lapen D. R., Mitchell G. W., Provencher J. F., Wilson S. (2020). Interaction of diet and habitat predicts Toxoplasma gondii infection rates in wild birds at a global scale. Glob. Ecol. Biogeogr. 29, 1189–1198. doi: 10.1111/geb.13096
Yorinks N., Atkinson C. T. (2000). Effects of malaria on activity budgets of experimentally infected juvenile Apapane (Himatione SanGuinea). Auk 117, 731–738. doi: 10.1093/auk/117.3.731
Zamora-Vilchis I., Williams S. E., Johnson C. N. (2012). Environmental temperature affects prevalence of blood parasites of birds on an elevation gradient: Implications for disease in a warming climate. PloS One 7, e39208. doi: 10.1371/journal.pone.0039208
Zhang Y., Wu Y., Zhang Q., Su D., Zou F. (2014). Prevalence patterns of avian Plasmodium and Haemoproteus parasites and the influence of host relative abundance in Southern China. PloS One 9, e99501. doi: 10.1371/journal.pone.0099501
Keywords: avian malaria, body condition, haemosporidian parasites, species trait, stress response
Citation: Zhan X, Huang X, Pagani-Núñez E, Tang Q, Ho H, Zhou W, Liu Y and Liang D (2024) The impacts of host traits on parasite infection of montane birds in southwestern China. Front. Ecol. Evol. 12:1305305. doi: 10.3389/fevo.2024.1305305
Received: 01 October 2023; Accepted: 22 January 2024;
Published: 07 February 2024.
Edited by:
Gang Song, Chinese Academy of Sciences (CAS), ChinaReviewed by:
Luis Gerardo Herrera M., Universidad Nacional Autónoma de México, MexicoAntoine Filion, Oklahoma State University, United States
Copyright © 2024 Zhan, Huang, Pagani-Núñez, Tang, Ho, Zhou, Liu and Liang. This is an open-access article distributed under the terms of the Creative Commons Attribution License (CC BY). The use, distribution or reproduction in other forums is permitted, provided the original author(s) and the copyright owner(s) are credited and that the original publication in this journal is cited, in accordance with accepted academic practice. No use, distribution or reproduction is permitted which does not comply with these terms.
*Correspondence: Dan Liang, dliang@princeton.edu; Yang Liu, liuy353@mail.sysu.edu.cn