- 1MSU-DOE Plant Research Laboratory, Department of Biochemistry and Molecular Biology, East Lansing, MI, United States
- 2Great Lakes Bioenergy Research Center, East Lansing, MI, United States
- 3Plant Resilience Institute, Michigan State University, East Lansing, MI, United States
Isoprene is one of the most abundant volatile organic compounds produced by some, though not all, plant species. It confers stress tolerance in both emitting and non-emitting species and has large impacts on gene regulation as well as on atmospheric chemistry. Understanding the control of isoprene emission from plants is important to understanding plant responses to future atmospheric conditions. In this study we determined that suppression of isoprene emission from plants by high CO2 concentrations is reduced but not eliminated by high temperature. We tested whether the CO2 suppression is caused by the reduction in ATP or NADPH availability caused by triose phosphate utilization (TPU) limitation of photosynthesis at high CO2. We measured CO2 assimilation as well as several photosynthetic electron transport parameters under multiple atmospheric conditions in four plant species grown at ambient CO2. While CO2 sensitivity of isoprene emission was somewhat correlated with TPU in some species, in other species it was not. Poplar exhibited significant CO2 suppression of isoprene emission but no evidence for TPU so we investigated further, measuring the electrochromic shift that gives information on ATP synthesis and photosystem I oxidation state. In all cases photosynthetic parameters were unchanged while isoprene emission dropped in response to increasing CO2. Non-photorespiratory conditions (2% O2) led to an increase in isoprene emission at low CO2 but did not alleviate suppression by high CO2. In all measured species the combination of higher temperature along with higher CO2 concentrations led to a net increase of isoprene emission in response to a moderate scenario for temperature and CO2 concentration in 2100 in the upper Midwest.
Introduction
Isoprene is emitted in large amounts (typically 2% of photosynthesis) by some, but not all, plants (Sharkey et al., 2008). Exogenous isoprene changes gene expression and protects leaves from oxidative and thermal stress in non-emitting plants (Velikova et al., 2014; Harvey and Sharkey, 2016; Zuo et al., 2019), while knocking down emission in native emitters reduces thermal tolerance (Sharkey et al., 2001; Behnke et al., 2007). The large amount of isoprene emitted to the atmosphere (600 Tg/year) has significant atmospheric effects, contributing to ozone and aerosol production in the troposphere and increasing the lifetime of methane (Guenther et al., 2006; Zhang et al., 2007; Pike and Young, 2009; Young et al., 2009). For these reasons an accurate understanding of the physiological control of isoprene and its propensity for change as global temperatures and atmospheric CO2 concentrations rise is essential for understanding plant health and stress tolerance as well as changing atmospheric chemistry.
Isoprene is produced by isoprene synthase, a TPS-b terpene synthase, from dimethylallyl diphosphate (DMADP) in the chloroplast (Silver and Fall, 1995; Sharkey et al., 2013). Chloroplastic DMADP is produced by the methylerythritol 4-phosphate (MEP) pathway, also called the non-mevalonate pathway (Figure 1). The MEP pathway consumes one pyruvate and one glyceraldehyde 3-phosphate molecule (derived from photosynthesis), one NADPH and four ferredoxin molecules, one CTP, and one ATP, to produce a single DMADP or isopentenyl diphosphate (IDP) molecule (Figure 1). The final enzyme in the pathway, HMBDP reductase (HDR), produces more IDP than DMADP; IDP isomerase (IDI) converts this to a final ratio of two DMADP for every IDP (Zhou et al., 2013). DMADP production has a similar temperature sensitivity as photosynthesis, although isoprene emission has a much higher temperature maximum (Li et al., 2011).
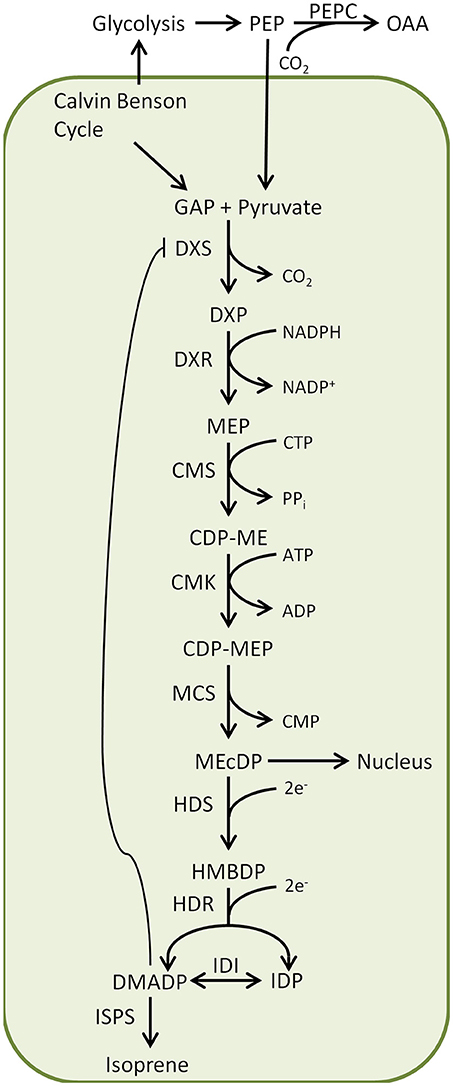
Figure 1. The MEP pathway in plants. Isoprenoids are produced by the MEP pathway under light-dependent reactions in the chloroplast. Enzymes: PEPC, phosphoenolpyruvate carboxylase; DXS, 1-deoxy-D-xylulose-5-phosphate synthase; DXR, 1-deoxy-D-xylulose-5-phosphate reductoisomerase; CMS/MCT, 4-diphosphocytidyl-2-C-methylerythritol synthase/2-C-methyl-D-erythritol-4-phosphate cytidylyltransferase; CMK, 4-(cytidine 5′-diphospho)-2-C-methyl-d-erythritol kinase; MCS, 2-C-methyl-D-erythritol-2,4-cyclodiphosphate synthase; HDS, 4-hydroxy 3-methylbut-2-enyl-diphosphate synthase; HDR, 4-hydroxy-3-methylbut-2-enyl-diphosphate reductase; IDI, isopentenyl diphosphate isomerase. In isoprene emitting plants the conversion of DMADP to isoprene is catalyzed by isoprene synthase (ISPS). Metabolites: PEP, phosphoenolpyruvate; OAA, oxaloacetic acid; GAP, glyceraldehyde 3-phosphate; DXP, 1-deoxy-D-xylulose-5-phosphate; MEP, methylerythritol 4-phosphate; CDP-ME, 4-(cytidine-5′-diphospho)-2-C-methyl-D-erythritol; CDP-MEP, 4-(cytidine-5′-diphospho)-2-C-methyl-D-erythritol phosphate; MEcDP, 2-C-methyl-D-erythritol-2,4-cyclodiphosphate; HMBDP, 4-hydroxy-3-methylbut-2-enyl-diphosphate; IDP, isopentenyl diphosphate; DMADP, dimethylallyl diphosphate. Two reducing equivalents each are required to convert MEcDP into HMBDP and HMBDP into DMADP; these can be provided by NADPH or by two ferredoxin molecules.
The rate of isoprene emission is affected by environmental factors such as light, temperature, CO2 concentration, and O2 concentration. Isoprene emission increases with high temperature (Monson et al., 1992; Sharkey et al., 1996, 1999; Singsaas and Sharkey, 1998; Wiberley et al., 2008; Cole, 2016; Lindwall et al., 2016; Staudt et al., 2016) and decreases with increasing CO2 (Sharkey et al., 1991; Rosenstiel et al., 2003; Centritto et al., 2004; Scholefield et al., 2004; Pegoraro et al., 2005, 2007; Possell et al., 2005; Monson et al., 2007, 2016; Possell and Hewitt, 2011; Way et al., 2013), although some studies have reported that growth at high CO2 does not decrease isoprene emission (Sharkey et al., 1991; Tognetti, 1998; Buckley, 2001; Rapparini et al., 2004; Calfapietra et al., 2007; Wilkinson et al., 2009; Sun et al., 2012); a summary of the CO2 effects on isoprene can be found in Pacifico et al. (2009). CO2 inhibition of isoprene seems to be oxygen-insensitive, although low O2 increases isoprene emission at low CO2 (Loreto and Sharkey, 1990). As future CO2 levels have been predicted to double by the end of this century, a number of studies have been carried out to model the effect of rising CO2 on future isoprene emission rates. Hantson et al. (2017) used a dynamic vegetation model taking into consideration changes in CO2 levels and land use by natural and anthropogenic causes, to show that isoprene levels have steadily decreased over the Twentieth century. This decrease was predicted to continue over the next century (Hantson et al., 2017). Way et al. (2013) investigated physiology and metabolomics of poplar under three different CO2 levels that reflected preindustrial, present, and future atmospheric conditions. This study also indicated that isoprene emission could decrease under future high CO2 levels, and that such a decrease in isoprene emission would lead to a reduction in isoprene-mediated benefits to plants such as tolerance to abiotic stress. While both of these studies modeled the effect of temperature as well as CO2, they did not model the combined effect of CO2 and temperature. Increasing temperature has been shown to mitigate or entirely abolish the CO2 effect (Rasulov et al., 2010; Sun et al., 2013; Potosnak et al., 2014a). The reduced effect of CO2 on isoprene at higher temperature must be included in order to accurately predict future isoprene emission rates. In effect, there is a gap between models and reality (Monson et al., 2007; Arneth et al., 2008a), a gap which may be closed with a mechanistic understanding of physiological controls of isoprene emission (Rasulov et al., 2010; Morfopoulos et al., 2014).
The physiological response of isoprene emission to the combination of changing temperature and CO2 has been well studied (Rasulov et al., 2009b, 2010; Potosnak et al., 2014a; Monson et al., 2016), as it is vital to modeling isoprene emission under future climate scenarios. Despite this, the mechanisms controlling emission rate are still under debate. Multiple hypotheses have been formulated to explain the decrease in isoprene emission under high CO2. One hypothesis is that high CO2 concentration stimulates phosphoenolpyruvate carboxylase (PEPC), which competes for the pyruvate required for the MEP pathway (Figure 1) (Rosenstiel et al., 2003, 2004; Wilkinson et al., 2009). However, competitive inhibitors of PEPC such as malate and diethyl oxalacetate do not impact the inhibition of isoprene emission by CO2 (Rasulov et al., 2018). An alternative hypothesis is that the physiological control of DMADP, and therefore isoprene emission, is based on energy status of the chloroplast (Niinemets et al., 1999; Rasulov et al., 2009b, 2016; Morfopoulos et al., 2014). Under conditions of high CO2 where triose phosphate utilization (TPU) limits photosynthesis, both ATP and NADPH production are reduced, which under the energy hypothesis would reduce DMADP production.
TPU limitation occurs when downstream sinks of photosynthetic carbon (such as sucrose, starch, and amino acid synthesis) are unable to keep up with the flux of triose phosphates produced by the Calvin-Benson cycle, which leads to feedback control of photosynthesis (Sharkey, 1985; McClain and Sharkey, 2019). Under TPU limitation, buildup of phosphorylated intermediates lowers the free phosphate in the chloroplast, slowing ATP synthase, which in turn lowers ATP levels and decreases the pH of the lumen, leading to a broad alteration in chloroplastic conditions. Ultimately under TPU limitation, proton motive force increases, while carbon assimilation flattens and electron transport declines with increasing CO2 (Yang et al., 2016). TPU limitation occurs at high CO2 when rubisco and ribulose 1,5-bisphosphate (RuBP) regeneration are not limiting, and is more likely at low temperature where TPU capacity is reduced. Like the CO2 sensitivity of isoprene emission, TPU limitation is temperature sensitive; it is rarely seen above 30°C (Harley and Sharkey, 1991). When photosynthesis is limited by TPU, it has no, or reverse, sensitivity to oxygen; that is, unlike under other limitations, photosynthesis is not increased, and sometimes is inhibited, by low oxygen (Sharkey et al., 1986; McClain and Sharkey, 2019). In this study we tested the hypothesis that changes in the energy status of the cell resulting from TPU limitation at high CO2 concentrations drive the sensitivity of isoprene emission to CO2.
To identify the underlying energetics and biochemical mechanisms responsible for the response of isoprene emission to high CO2, we measured the combined effect of temperature and CO2 concentration on isoprene emission, assimilation, and chlorophyll fluorescence in four species-Nicotianum tabacum var. Samsun (tobacco, a non-emitter) genetically engineered to emit isoprene (Vickers et al., 2009), and three native isoprene emitters: Phragmites australis (phragmites grass, a high-emitting monocot), Platanus × acerfolia (sycamore, a dicot tree) and Populas nigra × maximowiczii NM6 (poplar, a dicot tree used for commercial biomass production). We hypothesized that changes in the energy status of the chloroplast would explain the changes in isoprene emission at high CO2 and temperature. While previous studies have suggested this effect, they did not measure the energy status in vivo, and TPU limitation was not observed in all data sets. In fact, we observed that isoprene emission was not correlated with TPU limitation, and isoprene emission was highly sensitive to changing CO2 even under conditions where TPU limitation was not occurring and photochemical electron transport was constant. Isoprene emission was more strongly affected by temperature than by CO2. Therefore, in contrast to previous predictions, our data also support a significant increase in isoprene emission under future climate and CO2 conditions.
Methods
Plant Material
Nicotianum tabacum var. Samsun (tobacco) seeds transformed with Populus alba (poplar) isoprene synthase under control of a CaMV35 promoter were obtained from Claudia Vickers, University of Queensland. The plasmid construction and generation of the isoprene emitting and azygous lines are described in Vickers et al. (2009). We used isoprene emitting line 32, which was reported to have the highest isoprene emission, and matching azygous line [see Figure 1 in Vickers et al. (2009)]. Seeds were planted in Suremix growing medium (Michigan Grower Products, MI, USA) in 3 L pots and grown in a growth chamber (Big-Foot, BioChambers), under a 16 h photoperiod at a light intensity of 400 μmol m−2 s−1, day/night temperature of 25°C/22°C, and humidity of 60% for bulk seed production. For experiments reported here, seeds were planted in Suremix growing medium (Michigan Grower Products, MI, USA) in 3 L pots and grown in a greenhouse with supplemental lighting to extend the daylength to 16 h with a mean daytime light intensity of 300 μmol m−2 s−1 PAR, day/night temperature of 27°C/22°C, and at least 60% relative humidity. Plants were watered every other day with half strength Hoagland's solution (Hoagland and Arnon, 1938). Four to six-week-old plants were taken to the lab for experiments.
Phragmites australis (phragmites) was wild harvested from June to July from a colony on the campus of Michigan State University 42°43'13.5“N 84°28'22.6”W. Stalks were cut in the morning each day before the start of experiments. The cut ends were immediately placed in water, the stems were cut again under water to prevent air bubble formation in the xylem, and transferred to the lab, where they were kept in water throughout the day. Cuttings were discarded at the end of day.
Platanus × acerfolia (sycamore) was wild harvested from four trees outside the Plant Biology Building, Michigan State University 42°43'22.9“N 84°28'28.8”W. Branches were cut in the morning each day before the start of experiments. The branches were immediately placed in water, the petioles were cut again under water, and transferred to the lab. Petioles were kept in water throughout the day. Cuttings were discarded at the end of day.
Populas nigra × maximowiczii NM6 (poplar) trees were grown from cuttings provided by the Great Lakes Bioenergy Research Center (GLBRC). Cuttings were grown in 11 L pots filled with Suremix growing medium and watered daily with de-ionized water. Pots were kept under greenhouse conditions of 16-hour photoperiod with a mean daytime light intensity of 300 μmol m−2 s−1 PAR, and mean day/night temperature of 28°C/22°C. Figures 3, 5, 6 represent data obtained from 2, 3, and 5 month old cuttings, respectively. Plants were taken to the lab to perform experiments on attached leaves.
Gas Exchange and Chlorophyll Fluorescence Studies
Gas exchange and chlorophyll fluorescence measurements were taken simultaneously on individual, fully mature leaves using a LI-6800 Portable Photosynthesis System (LI-COR Biosciences, Lincoln, NE) connected to a Multiphase Flash™ Fluorometer and a 6 cm2 chamber with controlled light and gas flow (6800-01A). Leaves were equilibrated at each temperature (25, 30, or 35°C), 420 ppm reference air CO2 concentration, 1,000 μmol m−2 s−1 actinic light (10% blue, 90% red LEDs), and a constant vapor pressure difference (VPD) of between 1.6 and 2 kPa, depending on temperature. For ambient oxygen experiments, room air was used and conditioned by the LI-6800. For the low oxygen experiment, pure oxygen and nitrogen gas from tanks (Airgas, Radnor, PA) were mixed at the desired ratio and provided to the LI-6800 for control of water and CO2 concentration. Chlorophyll fluorescence measurements were taken using the multiphase flash setting as described by Avenson and Saathoff (2018). A-Ci curves were performed from low to high CO2 concentration, leaving the leaf at each CO2 concentration for 2–3 min before continuing to the next.
Real-time isoprene emission measurements were taken with a Fast Isoprene Sensor (FIS). The FIS uses the chemiluminescent reaction of isoprene and ozone to rapidly, sensitively, and specifically detect isoprene (Guenther and Hills, 1998). We used the instrument as described in Guenther and Hills (1998). Approximately 60% of the airflow coming over the leaf was redirected from the LI-6800 output to the FIS for isoprene analysis. The air flow rate in the LI-6800 was set to 500 μmol s−1 and the FIS sample flow rate to 400 sccm (280 μmol s−1). A 3.25 ppm isoprene standard (in nitrogen) purchased from Airgas was used for calibration of the FIS.
Leaf Spectroscopy Measurements
Electrochromic shift (ΔA520, a measure of proton-motive force, PMF) and ΔA820 (a measure of photosystem I (PSI) oxidation measurements were taken with a modified IDEA spec (Hall et al., 2013). The IDEA spec described by Hall et al. (2013) was modified by addition of blue LEDs to supplement the red actinic light of the original spec. When the IDEA spec is set to 1,000 μmol m−2 s−1 PAR, blue light represents less than 10% of the total light, similar to the LI-6800 fluorescence head. In addition, the light guide was adapted to fit the LI-6800 clear top chamber (6800-12A), a 9 cm2 square chamber. Electrochromic shift measurements were taken as described in Kanazawa et al. (2017), except that the modified IDEA spec does not have far red capability and so the measurement was taken without the benefit of far red to fully oxidize PSI. As such, the measurements of oxidation state presented here are estimates based on measurements in which PSI may not have been fully oxidized. Electrochromic shift measurements were taken and fitted as described in Takizawa et al. (2007) to determine PMF.
Metabolite Measurements
The post-illumination method for quantification of (MEcDP) and DMADP in vivo was performed as described in Li and Sharkey (2013). For post-illumination isoprene emission measurements, a poplar leaf was equilibrated in a LI-6800 as described above. Chamber clearing was determined by running isoprene standard into the LI-6800 fluorescence head via a needle, then removing the needle. After correcting for chamber clearing, the total isoprene emitted for the first 3 min after turning the light off (the initial decay in isoprene emission) was calculated which corresponds with the amount of DMADP present. Between 12 and 25 min a post-illumination “burst” of isoprene is observed and the total isoprene emitted in this time frame corresponds with the amount of MEcDP present in the leaf (Li and Sharkey, 2013). A sample burst with chamber clearing is shown in Figure 2.
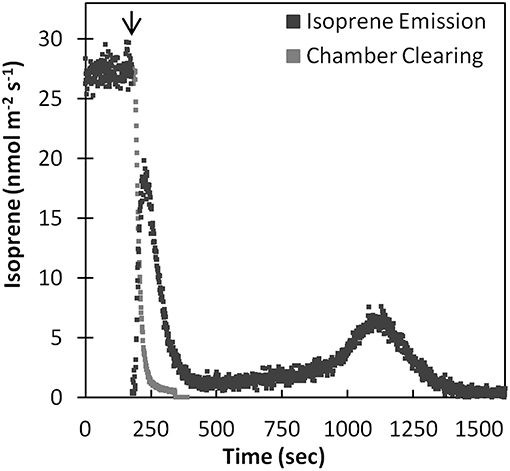
Figure 2. Sample post-illumination burst. A poplar leaf was equilibrated at 400 ppm CO2 for 40 min prior to turning the light off (shown at 178 s, marked with arrow). Chamber clearing (light gray squares) was determined by running an isoprene standard into the chamber, and then removing it; the time it takes the chamber to clear of isoprene is proportional to initial emission rate. Isoprene emission is shown after subtracting chamber clearing (dark gray squares). The area under the curve in isoprene emission between 178 and 428 s is proportional to the DMADP pool, which gets converted entirely by isoprene synthase post-illumination. The area under the curve between 428 and 1500 s is proportional to the MEcDP pool, which is slowly converted to DMADP in the dark.
Statistical Analyses
Statistical analyses were performed using Excel 2007 (Microsoft, Redmond, WA). Averages and standard error for n = 3 plants (n = 5 plants for phragmites) are reported. For data presented in Figures 4 and 7, one-factor ANOVA followed by a t-test was used to identify differences between means for each metabolite and isoprene emission under varying CO2 levels. For data presented in Figure 5, a representative sample is shown; this was selected from n = 4 plants. The raw spectroscopic data presented in Figure 5B was processed using an R script (R Foundation for Statistical Computing), which deconvoluted the changes in absorbance at 505 and 535 nm from the changes at 520 nm and fit the dark interval reaction kinetics to calculate PMF according to the principles reported in Kanazawa et al. (2017). R scripts are available upon request.
To compare the sensitivity of isoprene emission and assimilation rates to temperature, measurements taken at 400 ppm CO2 and from 25 to 35°C temperature settings were used to calculate the temperature coefficient (Q10) values for isoprene emission and assimilation. Q10 was calculated using the following equation: Q10 = (R2/R1)(10/T2−T1) where, T1 = 25°C, T2 = 35°C, and R1 and R2 are isoprene emission or assimilation rates measured at 25°C or 35°C, respectively. Isoprene emission rates collected at different CO2 and temperature levels were used to predict the combined effect of CO2 and temperature on isoprene emission under current climate conditions and in year 2100. Typical summer CO2 and temperature highs in Michigan under current conditions were considered as 400 ppm CO2 and 28°C, respectively. Based on the moderate climate scenario (RCP 6.0) proposed by the IPCC (Pachauri et al., 2014), a CO2 level of 800 ppm and a 5°C increase in temperature was assumed for the year 2100. Estimates were performed in Excel.
Results
The Combined Effects of Temperature and CO2 on Isoprene Emission and Assimilation
Regardless of the species, increasing temperature greatly increased isoprene emission at all CO2 concentrations (Figure 3, top), but had a much smaller effect on assimilation (Figure 3, middle). A sharp decline in isoprene emission was observed under high CO2 at 25°C and 30°C in all species. In phragmites and in tobacco, higher temperature (35°C) nearly abolished the CO2 inhibition of isoprene emission, although a small, but not statistically significant (at α = 0.05), decrease is still apparent. In poplar and sycamore, the CO2 inhibition of isoprene emission was still strong even at 35°C. TPU limitation should cause assimilation to plateau or even decrease at high CO2. We observed a flattening, although not a decrease, of the A-Cicurve in tobacco at 25°C, but not at higher temperatures or in the other plant species. This flattening suggests that tobacco plants had a mild TPU limitation under these conditions. The loss of TPU limitation at higher temperatures in some species is consistent with the previously characterized temperature sensitivity of TPU limitation (Harley and Sharkey, 1991). However, the inhibition of isoprene emission rate by increasing CO2 concentration was seen at 30°C in all species even when TPU was not occurring. This is in contrast to previous studies that showed that the CO2 sensitivity was temperature dependent and would not occur at high temperature (Potosnak et al., 2014a). In particular, we saw that poplar had no TPU limitation at any temperature and also showed strong suppression of isoprene by CO2 at every temperature, indicating that TPU limitation is not required for suppression of isoprene by CO2 in this species.
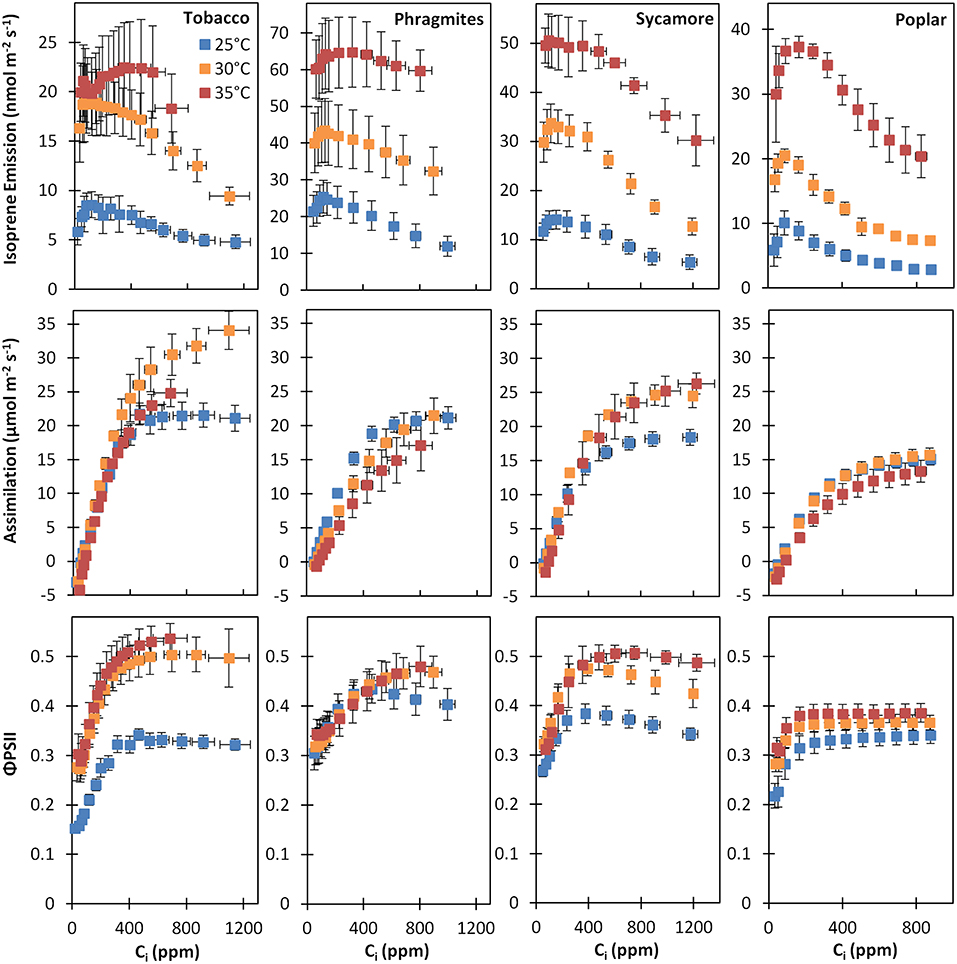
Figure 3. Comparison of isoprene emission, carbon assimilation, and electron transport under varying CO2 and temperature levels. The combined effect of CO2 and temperature on isoprene emission (top), carbon assimilation (middle), and ΦPSII (bottom) is presented for tobacco genetically engineered to emit isoprene, phragmites, sycamore, and poplar. Blue, orange, and red squares represent data at 25, 30, and 35°C respectively. For isoprene emission (top), different scales are indicated on the Y-axis for each species for clarity. Values represent the mean ± SE of n = 3 plants; n = 5 for phragmites. Poplar leaves were from two-month-old cuttings. Plants were allowed to equilibrate at 420 ppm CO2 (incoming air CO2 concentration) prior to beginning measurements for each A-Ci curve. Each point represents carbon assimilation or isoprene emission at the end of a 3-min time period at each CO2 concentration. Adjustment of CO2 concentration proceeded from low (25 ppm) to high (1,500 ppm) reference concentration. ΦPSII was derived from chlorophyll fluorescence, which was measured using the LI-6800 multiphase saturating flash at the end of each 3 min period.
The Q10 values for isoprene emission and assimilation, summarized in Table 1, clearly show that isoprene emission is far more sensitive to temperature than is assimilation (Table 1). We also calculated isoprene emission rates for all four species under conditions of global warming (Figure 4). In our region (northern Midwest, North America), where all species except tobacco are common urban plants, current summer CO2 and temperature conditions are estimated at 400 ppm and 28°C, respectively. Under the IPCC moderate climate scenario (RCP 6.0) (Pachauri et al., 2014), which predicts an increase in global CO2 concentrations from 400 to 800 ppm, temperature in our region is expected to increase by an average of 5°C, to new summer conditions of 33°C. We extrapolated data to these exact conditions from the data in Figure 3. Extrapolated Ci values are in Table 2. While the large change in CO2 does suppress isoprene emission somewhat, the change in temperature more than overcomes the suppression, leading to an increase of isoprene emission in all species (Figure 3, top, and Figure 4).
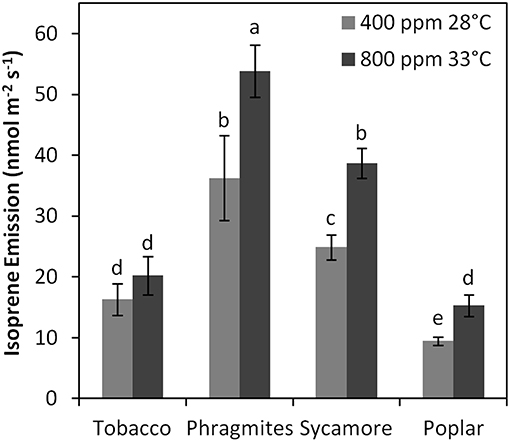
Figure 4. The combined effect of CO2 and temperature on isoprene emission in current day climate and in year 2100 based on moderate climate change scenario. Current year is shown in light gray and year 2100 scenario in dark gray. Four hundred parts per million CO2 and 28°C temperature represents a typical summer high in northern climates under current conditions. The future isoprene emissions are based on a moderate climate change scenario for the year 2100, which predicts CO2 concentrations at 800 ppm and a temperature rise of 5°C in northern climates (Pachauri et al., 2014). Values represent data extrapolated from Figure 3 shown as the mean ± SE of n = 3 plants; n = 5 for phragmites. Statistical differences at α = 0.05 are marked with lower-case letters.
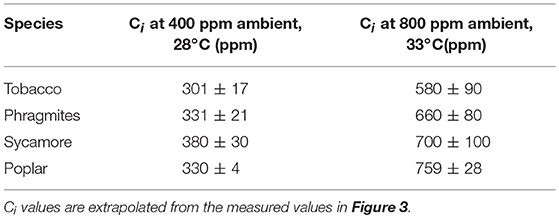
Table 2. Ci values for Figure 4.
Effect of CO2 on Energy Status of the Leaf
It is possible for TPU limitation to affect the energy status of the chloroplast before changes in assimilation are observed. Previous studies concluded that energy status of the chloroplast is responsible for changes in isoprene emission due to CO2 and temperature, but did not directly measure energy status. We measured energy status of the chloroplast using well established spectroscopic techniques. If high CO2 concentrations altered energy status of the leaf through TPU limitation or any other mechanism, ΦPSII, the ratio of excitons reaching photosystem II that are used for photochemistry, should decrease at high CO2 concentrations. ΦPSII is derived from chlorophyll fluorescence analysis. In tobacco, phragmites, and poplar, ΦPSII remained constant at CO2 concentrations that suppressed isoprene emission at 30°C and 35°C (Figure 3, bottom). However, in sycamore ΦPSII indicated there was a TPU limitation even at 35°C. The data from phragmites and sycamore is particularly interesting as at 25°C ΦPSII decreases slightly at high CO2, while assimilation does not show TPU limitation. Therefore, we did observe changes in the energy status of the chloroplast that did not cause an obvious TPU behavior in assimilation.
However, most important were the cases where ΦPSII was not decreasing and therefore could not have been limiting for isoprene emission, even though strong suppression of isoprene emission was observed. This is clearest in the poplar data, where this was observed at all temperatures, although also in other species at the higher temperatures (Figure 3). For this reason we chose poplar for further experiments to look at the electrochromic shift and PSI oxidation. Electrochromic shift is the shift in absorbance of carotenoids resulting from an electric field across the molecule (Witt, 1979; Takizawa et al., 2007). As such, it serves as an in vivo spectroscopic measure of proton-motive force (PMF). Following the methods and models of Takizawa et al. (2007) as implemented in our modified IDEA spec, we determined the change in PMF of poplar leaves under increasing CO2. While we continued to see the large decrease in isoprene emission under elevated CO2 (Figure 5A), PMF remained constant (Figure 5B). This spec can also measure the change in absorbance at 820 nm under normal or fully oxidized conditions, which is a measure of the oxidation status of PSI (Kanazawa et al., 2017). PSI oxidation was also constant under high CO2 that suppressed isoprene emission (Figure 5C).
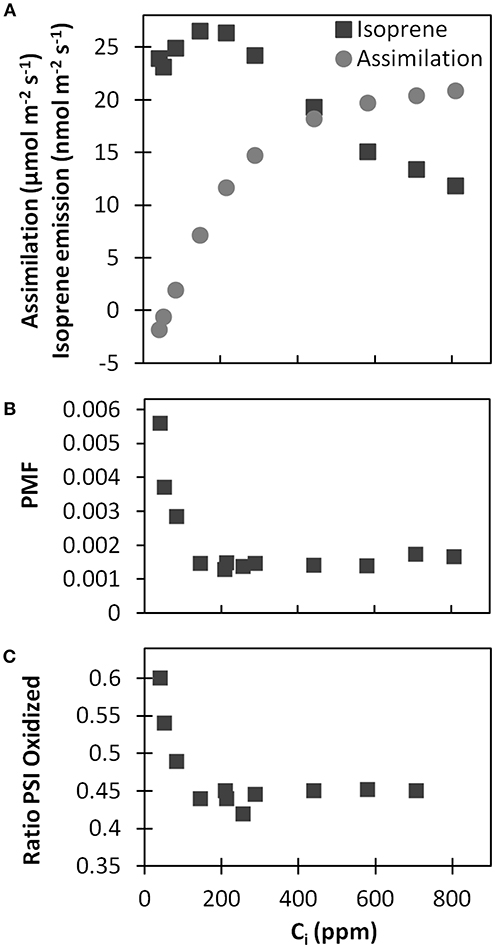
Figure 5. Effect of CO2 on ATP synthesis and photosystem I in poplar. Isoprene emission (squares) and carbon assimilation (circles) (A), the proton motive force (PMF) (B), and the ratio of oxidized to reduced PSI (C) under varying CO2 concentrations were measured in poplar leaves from three-month-old cuttings. Poplar leaves were placed in the 9 cm2 leaf chamber of the modified IDEA Spec which is a custom instrument that can measure ΦPSII, PSI oxidation (ΔA820), and electrochromic shift (ΔA520). Representative data is shown out of n = 4. Measurements were taken at 30°C and 1,000 μmol m−2 s−1 PAR.
The Effect of Oxygen on the Isoprene Response to CO2
To test whether energy consumed by photorespiration can explain the CO2 effect on isoprene when photorespiration decreases at high CO2, we measured the isoprene response to CO2 at low (2%) as well as normal (21%) oxygen concentrations in poplar (Figure 6A). Interestingly, poplar leaves used for the oxygen sensitivity measurements were exhibiting signs of TPU limitation, even though the conditions (30°C and 1,000 μmol m−2 light) used were the same as in Figures 3, 5. At 21% oxygen, both assimilation (Figure 6B) and ΦPSII (Figure 6C) began to decrease with increasing CO2 once past the rubisco limitation of photosynthesis, which is indicative of TPU limitation. Low (2%) oxygen increased isoprene emission at low CO2, but as CO2 concentration increased past 400 ppm, the inhibition of isoprene emission was sufficiently strong that the isoprene emission at 800 ppm and higher CO2 were identical under both conditions (Figure 6A).
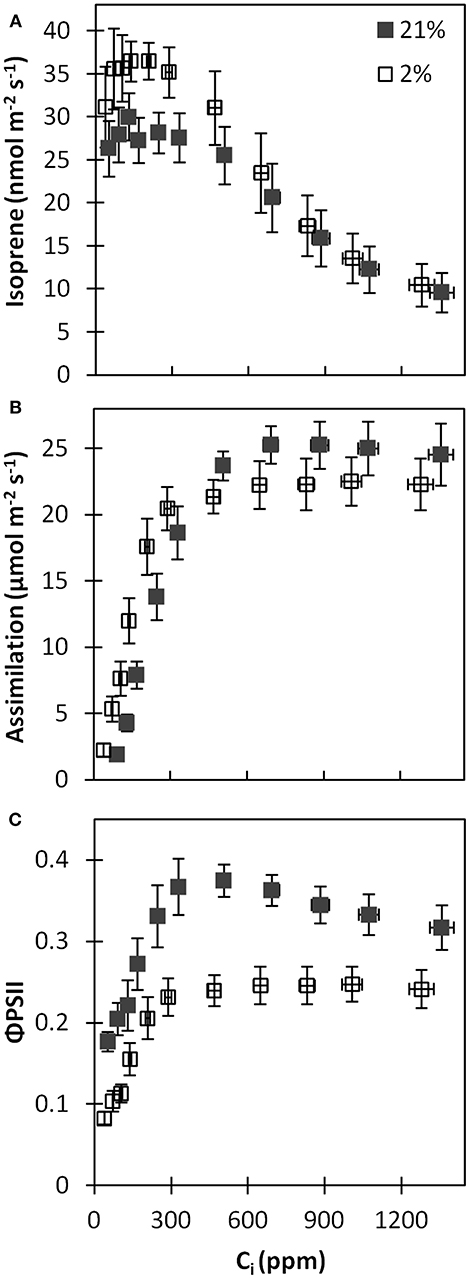
Figure 6. Effect of oxygen on the isoprene emission response to CO2 in poplar. Isoprene emission (A), carbon assimilation (B), and ΦPSII (C) under varying CO2, and 21% (filled squares) or 2% (empty squares) oxygen was measured simultaneously in poplar. Poplar leaves were from five-month-old cuttings. Values represent the mean ± SE and n = 3 plants.
The Effect of CO2 on MEcDP and DMADP
If either NADPH availability or ATP/CTP availability was responsible for the changes in isoprene emission under elevated CO2, then distinct patterns in the MEP pathway metabolites should be visible. If reducing power (electron availability) is limiting, then MEcDP should build up, as HDS and HDR are extremely dependent on reducing power availability. We measured MEcDP and DMADP in poplar leaves by measuring the decay in isoprene emission immediately after turning off the light (which is proportional to the amount of DMADP present) and during the burst of isoprene 12 to 25 min after turning off the light (which is proportional to the amount of MEcDP present) (Rasulov et al., 2009a; Li and Sharkey, 2013). We saw a decrease in MEcDP and DMADP with increasing CO2 (Figure 7), indicating that electron availability is not responsible for the reduction in isoprene emission at high CO2 in poplar.
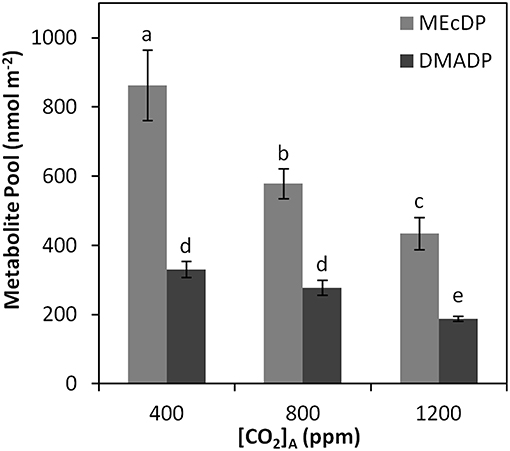
Figure 7. The effect of high CO2 on downstream MEP pathway metabolite levels in poplar. MEcDP (light gray) and DMADP (dark gray) levels in poplar leaves were measured by the post-illumination method. Leaves were equilibrated in the LI-6800 for 40 min at 30°C, 400, 800, or 1200 ppm chamber CO2 concentration, and 1,000 μmol m−2 s−1 actinic light. After turning off the light, isoprene emission continues for about 3 min. Between 3 and 12 min a burst of isoprene emission is observed. This continuing post-illumination emission directly correlates with DMADP and MEcDP pool sizes respectively (Li and Sharkey, 2013). Values represent the mean ± SE of n = 4 plants for the 400 ppm data and 3 for the 800 and 1200 ppm data. Statistical differences at α = 0.05 are marked with lower-case letters.
Discussion
We investigated the combined effects of temperature and CO2 on isoprene emission and found that isoprene emission was more strongly affected by temperature than by CO2. We hypothesized that TPU limitation would explain the changes in isoprene emission at high CO2 and temperature. In fact, we observed that isoprene emission was not correlated with TPU limitation, and that isoprene emission was highly sensitive to changing CO2 even under conditions where TPU limitation was not occurring. We confirmed these measurements in four species, including a transgenic tobacco plant that has been widely used to probe the effects of isoprene emission on plants. While TPU limitation can occur at the same time as CO2 suppression of isoprene emission, sufficient data show that TPU limitation is not necessary for this suppression to occur. Furthermore, the leaf energetic status remained constant, which indicated that isoprene is not solely controlled by the energy status of the leaf, but other drivers must be necessary in order to explain the changes in isoprene emission. The significance of above findings and alternative hypotheses that may explain the suppression of isoprene emission under high CO2 are discussed below.
Suppression of Isoprene Emission by High CO2 Can Occur in the Absence of TPU Limitation
Previous studies have indicated that the conditions under which decreased isoprene emission at high CO2 occur correlate with the conditions under which TPU limitation occurs (Monson et al., 2016; Rasulov et al., 2016). The CO2 sensitivity of isoprene emission is temperature sensitive, and is abolished under high temperature, an effect we see in two species but not in data from all species. TPU limitation is also temperature sensitive and rarely seen above 30°C (Harley and Sharkey, 1991). TPU limitation is also oxygen insensitive and still occurs under low oxygen, unlike photorespiratory effects (Sharkey et al., 1986). Loreto and Sharkey (1990) observed that the effect of CO2 on isoprene was also oxygen insensitive. We observed that at low CO2, low O2 increased isoprene emission, but isoprene emission rapidly decreased with increasing CO2; that is, the CO2 effect on isoprene is oxygen insensitive. Given the effect of TPU limitation on ATP synthesis and electron transport, previous studies concluded that the changes in energy status in the chloroplast are responsible for the changes in isoprene emission in response to CO2 and temperature (Rasulov et al., 2009a, 2016; Monson et al., 2016). However, none of these studies directly measured energy status in the chloroplast under high CO2 when a decrease in isoprene emission was observed.
Using combined CO2 and temperature treatments on four species representative of broad isoprene emitting capabilities, we clearly showed that suppression of isoprene emission under high CO2 can occur when TPU limitation of assimilation and electron transport (ΦPSII) is absent. This was previously observed, but we hypothesized that changes in the energy status of the chloroplast can happen even when there are no indications of TPU limitation based on assimilation rate. Using several in vivo spectroscopic techniques we characterized in detail the energy status of poplar under high CO2 and found that while PMF and photosystem I oxidation were constant, isoprene emission was still reduced under high CO2. These both measure the ability of the plant to make ATP and if they are constant, the ATP available for isoprene emission is probably constant. In multiple species our data show that changes in ΦPSII are not necessary in order to see decreases in isoprene emission at high CO2. We further confirmed this by measuring the MEcDP and DMADP pools, which showed that MEcDP was decreasing with increasing CO2. If electron transport rate (as opposed to ATP) was limiting, we would expect MEcDP to build up as ferredoxin is required to convert it to DMADP. While in some conditions we did see ΦPSII decrease with increasing CO2, it was not required for isoprene emission to decrease. Taken together, this data shows that the suppression of isoprene emission rate by CO2 is not dependent on the energy status of the chloroplast.
Alternate Hypotheses for the CO2 Effect on Isoprene Emission
Given that our data rules out TPU limitation and reduced energy availability as causes, we can speculate several possibilities to explain the observed reduction in isoprene emission under high CO2. Our data is consistent with the carbon limitation (PEP carboxylase) hypothesis for the suppression of isoprene emission. However, this hypothesis was previously ruled out by Rasulov et al. (2018), and our data is not sufficient to re-open this hypothesis for investigation. CO2 sensing, either through direct sensors in the stomata, or indirect sensing through a method such as calcium release from the apoplast, may lead to changes in the MEP pathway that would explain this response. Feeding EGTA, a specific chelator of Ca2+ has previously been shown to alter bursts or changes in isoprene emission due to wounding (Loreto and Sharkey, 1993). We attempted to test this hypothesis by feeding EGTA to detached poplar leaves; however, the EGTA led to such broad systemic changes, including a massive decrease in ΦPSII, that no conclusion could be drawn about isoprene emission specifically. Calfapietra et al. (2007) showed that expression of MEP pathway genes and isoprene synthase protein levels can decrease in plants grown under high CO2 and Scholefield et al. (2004) saw a similar effect in extractable enzyme activity, although these differences were not significant. More research is required to determine if changes in isoprene synthase levels or activity can explain changes in isoprene emission at high CO2. Even if this is a potential explanation for plants grown at high CO2, we saw significant effects within 10 min (the length of a rapid A-Ci curve), which is too short a time scale for substantial changes in gene expression or protein levels to occur. Wiberley et al. (2009) calculated that the lifetime for isoprene synthase was 3.4 days at 30°C. Therefore, a metabolic change at high CO2 is still necessary in order to explain these short-term changes.
Effect of CO2 on Isoprene Emission Models
Prior studies showed that the global increase in temperature due to climate change would not be sufficient to overcome the suppression of isoprene emission by increasing atmospheric CO2 concentrations (Possell and Hewitt, 2011; Hantson et al., 2017). These models were based on data that showed both the CO2 and temperature effects on isoprene, but not their combination, and showed an overall decrease in global isoprene emissions. We found no support for electron-transport based models of isoprene emission that predict decreased isoprene emission rates (Niinemets et al., 1999; Grote et al., 2014; Morfopoulos et al., 2014). Changing land use in the future will likely decrease the biomass of isoprene emitting species (Arneth et al., 2008b; Hantson et al., 2017); but increased temperature may select for isoprene emission on an ecosystem level (Purves et al., 2004; Mutanda et al., 2016; Taylor et al., 2018). Long term growth at high CO2 leads to increased leaf area in Populus species (Rey and Jarvis, 1998; Murthy et al., 2005; Pegoraro et al., 2007); under drought stress or in Eucalyptus species leaf area may not increase (Potosnak et al., 2014b; Duursma et al., 2016). Pegoraro et al. (2005) concluded that the increased leaf area under high CO2 conditions will lead to a smaller decrease in ecosystem isoprene emissions than would be indicated by leaf-level measurements corrected by leaf area. Another concern is that the overall emission rates used in these studies are lower under all conditions than is typically reported for these species, indicating that perhaps the conditions do not reflect real-world forest conditions (Possell et al., 2005; Possell and Hewitt, 2011; Hantson et al., 2017). Some studies showed that high temperature (35°C) abolishes the CO2 effect (Potosnak et al., 2014a; Monson et al., 2016). We did not see that high temperature abolished the CO2 effect in sycamore or poplar, although it did in phragmites and tobacco. We show that even small changes in temperature increase isoprene emission by far more than a doubling of CO2 concentration would decrease it. We calculated isoprene emissions in all four species under current-day summer conditions (400 ppm and 28°C) and predicted year 2100 conditions under an IPCC climate model (800 ppm and 33°C) based on our short-term data. While the global change in temperature is only 3°C in this scenario, data supports larger changes in northern climates compared to the global average, up to 5°C in the northern United States. In contrast to the commonly accepted models, we predict that global isoprene emissions will increase greatly under future climate scenarios. This may help protect plants against increasing thermal stress as well as ozone and increase the lifetime of methane in the lower atmosphere.
Our data is based on very short-term changes, from 10 min to an hour under high CO2 conditions. Long term changes may lead to different effects. Long term effects like suppression of gene expression at high CO2 may be offset by high temperature enhancement of gene expression and isoprene synthase protein accumulation (Wiberley et al., 2008). The delay between leaf emergence and isoprene emission could be significantly shortened at higher temperature (Monson et al., 1994). Plants grown under high CO2 seem to have less strong suppression of isoprene emission than plants that are held at high CO2 at short timescales (Scholefield et al., 2004; Possell et al., 2005; Calfapietra et al., 2007; Possell and Hewitt, 2011; Way et al., 2013), however suppression of isoprene emission is still evident. Long term temperature changes can also have different effects than short term ones (Hanson and Sharkey, 2001; Centritto et al., 2011; Fares et al., 2011; Rasulov et al., 2015; Arab et al., 2016). Rasulov et al. (2015) found that the isoprene emission of plants grown at high temperature were more responsive than that of plants grown at low temperature on a gram dry weight basis, but that the responsiveness was the same on a leaf area basis. Fares et al. (2011) found the opposite: the isoprene emission of plants grown at high temperature had a lower Q10 than those grown at low temperature. However, growing plants at high temperature still suppresses the effect of CO2, leading to overall higher isoprene emissions (Potosnak et al., 2014a). Short term changes in metabolism under high CO2 are likely reflective of long term adaptation to high CO2, as is evident in the TPU literature (McClain and Sharkey, 2019). It is reasonable to expect that the same holds true for isoprene emission, and that our predictions based on short term measurements of changes in CO2 are reflective of what will hold true on longer time scales. There is likely to be more isoprene emission in the future in some, and perhaps many, terrestrial ecosystems.
Author Contributions
TDS conceived the experimental plan. CS and LG performed the assimilation, ΦPSII, and isoprene emission response to CO2 and temperature experiments. ATL performed the IDEA spec (ECS and PSI oxidation), low oxygen, and post-illumination experiments. JAC designed the interface between the IDEA spec and the LI-COR 6800 and provided expertise on interpretation of the IDEA spec data. AMM wrote R scripts to analyze the IDEA spec data. ATL and CS performed the data analysis. ATL wrote the first draft of the manuscript and SMW assisted in production of the manuscript. All authors contributed to manuscript writing and revision, read and approved the submitted version.
Funding
This material is based upon work supported in part by the Great Lakes Bioenergy Research Center, U. S. Department of Energy, Office of Science, Office of Biological and Environmental Research under Award Number DE-SC0018409, and in part by U. S. Department of Energy Grant DE-FG02-91ER2002. CS was supported by the Plant Genomics @ Michigan State University REU Program NSF DBI-1358474. ATL was supported by the Strategic Partnership Grant @ Michigan State University. LG was supported by the Great Lakes Bioenergy Research Center. SMW was supported by the DOE Plant Research Laboratory grant. TDS received partial salary support from Michigan AgBioResearch.
Conflict of Interest Statement
The authors declare that the research was conducted in the absence of any commercial or financial relationships that could be construed as a potential conflict of interest.
Acknowledgments
The authors would like to thank David Kramer for advice and consultation regarding design and measurements of the modified Idea Spec.
References
Arab, L., Kreuzwieser, J., Kruse, J., Zimmer, I., Ache, P., Alfarraj, S., et al. (2016). Acclimation to heat and drought - lessons to learn from the date palm (Phoenix dactylifera). Environ. Exp. Bot. 125, 20–30. doi: 10.1016/j.envexpbot.2016.01.003
Arneth, A., Monson, R. K., Schurgers, G., Niinemets, Ü., and Palmer, P. I. (2008a). Why are estimates of global terrestrial isoprene emissions so similar (and why is this not so for monoterpenes)? Atmos. Chem. Phys. 8, 4605–4620. doi: 10.5194/acp-8-4605-2008
Arneth, A., Schurgers, G., Hickler, T., and Miller, P. A. (2008b). Effects of species composition, land surface cover, CO2 concentration and climate on isoprene emissions from European forests. Plant Biol (Stuttg). 10, 150–162. doi: 10.1055/s-2007-965247
Avenson, T. J., and Saathoff, A. J. (2018). Sub-saturating multiphase flash irradiances to estimate maximum fluorescence yield. Methods Mol. Biol. 1770, 105–120. doi: 10.1007/978-1-4939-7786-4_7
Behnke, K., Ehlting, B., Teuber, M., Bauerfeind, M., Louis, S., Hänsch, R., et al. (2007). Transgenic, non-isoprene emitting poplars don't like it hot. Plant J. 51, 485–499. doi: 10.1111/j.1365-313X.2007.03157.x
Buckley, P. (2001). Isoprene emissions from a Florida scrub oak species grown in ambient and elevated carbon dioxide. Atmos. Environ. 35, 631–634. doi: 10.1016/S1352-2310(00)00332-0
Calfapietra, C., Wiberley, A. E., Falbel, T. G., Linskey, A. R., Mugnozza, G. S., Karnosky, D. F., et al. (2007). Isoprene synthase expression and protein levels are reduced under elevated O3 but not under elevated CO2 (FACE) in field-grown aspen trees. Plant Cell Environ. 30, 654–661. doi: 10.1111/j.1365-3040.2007.01646.x
Centritto, M., Brilli, F., Fodale, R., and Loreto, F. (2011). Different sensitivity of isoprene emission, respiration and photosynthesis to high growth temperature coupled with drought stress in black poplar (Populus nigra) saplings. Tree Physiol. 31, 275–286. doi: 10.1093/treephys/tpq112
Centritto, M., Nascetti, P., Petrilli, L., Raschi, A., and Loreto, F. (2004). Profiles of isoprene emission and photosynthetic parameters in hybrid poplars exposed to free-air CO2 enrichment. Plant Cell Environ. 27, 403–412. doi: 10.1111/j.1365-3040.2003.01156.x
Cole, M. (2016). Combinatory effect of changing CO2, temperature, and long-term growth temperature on isoprene emissions. Depaul Discoveries. 5:15. Available online at: https://via.library.depaul.edu/depaul-disc/vol5/iss1/15
Duursma, R. A., Gimeno, T. E., Boer, M. M., Crous, K. Y., Tjoelker, M. G., and Ellsworth, D. S. (2016). Canopy leaf area of a mature evergreen Eucalyptus woodland does not respond to elevated atmospheric [CO2] but tracks water availability. Glob. Chang. Biol. 22, 1666–1676. doi: 10.1111/gcb.13151
Fares, S., Mahmood, T., Liu, S. R., Loreto, F., and Centritto, M. (2011). Influence of growth temperature and measuring temperature on isoprene emission, diffusive limitations of photosynthesis and respiration in hybrid poplars. Atmos. Environ. 45, 155–161. doi: 10.1016/j.atmosenv.2010.09.036
Grote, R., Morfopoulos, C., Niinemets, Ü., Sun, Z., Keenan, T. F., Pacifico, F., et al. (2014). A fully integrated isoprenoid emissions model coupling emissions to photosynthetic characteristics. Plant Cell Environ. 37, 1965–1980. doi: 10.1111/pce.12326
Guenther, A., and Hills, A. J. (1998). Eddy covariance measurement of isoprene fluxes. J. Geophys. Res. Atmos. 103, 13145–13152. doi: 10.1029/97JD03283
Guenther, A., Karl, T., Harley, P., Wiedinmyer, C., Palmer, P. I., and Geron, C. (2006). Estimates of global terrestrial isoprene emissions using MEGAN (Model of Emissions of Gases and Aerosols from Nature). Atmos. Chem. Phys. 6, 3181–3210. doi: 10.5194/acp-6-3181-2006
Hall, C. C., Cruz, J. A., Wood, M., Zegarac, R., DeMars, D., Carpenter, J., et al. (2013). “Measurements with the IDEA spec: an integrated diode emitter array spectrophotometer/fluorometer,” in Photosynthesis Research for Food, Fuel and the Future Advanced Topics in Science and Technology in China, eds T. Kuang, C. Lu, and L. Zhang (Berlin; Heidelberg: Springer), 184–188. doi: 10.1007/978-3-642-32034-7_38
Hanson, D., and Sharkey, T. (2001). Rate of acclimation of the capacity for isoprene emission in response to light and temperature. Plant Cell Environ. 24, 937–946. doi: 10.1046/j.1365-3040.2001.00745.x
Hantson, S., Knorr, W., Schurgers, G., Pugh, T. A. M., and Arneth, A. (2017). Global isoprene and monoterpene emissions under changing climate, vegetation, CO2 and land use. Atmos. Environ. 155, 35–45. doi: 10.1016/j.atmosenv.2017.02.010
Harley, P. C., and Sharkey, T. D. (1991). An improved model of C3 photosynthesis at high CO2: reversed O2 sensitivity explained by lack of glycerate reentry into the chloroplast. Photosynthesis Res. 27, 169–178. doi: 10.1007/BF00035838
Harvey, C. M., and Sharkey, T. D. (2016). Exogenous isoprene modulates gene expression in unstressed Arabidopsis thaliana plants. Plant Cell Environ. 39, 1251–1263. doi: 10.1111/pce.12660
Hoagland, D. R., and Arnon, D. I. (1938). The water culture method for growing plants without soil. Calif. Agric. Exp. Stn. Circ. 347:32.
Kanazawa, A., Ostendorf, E., Kohzuma, K., Hoh, D., Strand, D. D., Sato-Cruz, M., et al. (2017). Chloroplast ATP synthase modulation of the thylakoid proton motive force: implications for Photosystem I and Photosystem II photoprotection. Front. Plant Sci. 8:719. doi: 10.3389/fpls.2017.00719
Li, Z., Ratliff, E. A., and Sharkey, T. D. (2011). Effect of temperature on postillumination isoprene emission in oak and poplar. Plant Physiol. 155, 1037–1046. doi: 10.1104/pp.110.167551
Li, Z., and Sharkey, T. D. (2013). Metabolic profiling of the methylerythritol phosphate pathway reveals the source of post-illumination isoprene burst from leaves. Plant Cell Environ. 36, 429–437. doi: 10.1111/j.1365-3040.2012.02584.x
Lindwall, F., Svendsen, S. S., Nielsen, C. S., Michelsen, A., and Rinnan, R. (2016). Warming increases isoprene emissions from an arctic fen. Sci. Total Environ. 553, 297–304. doi: 10.1016/j.scitotenv.2016.02.111
Loreto, F., and Sharkey, T. D. (1990). A gas-exchange study of photosynthesis and isoprene emission in Quercus rubra L. Planta 182, 523–531. doi: 10.1007/BF02341027
Loreto, F., and Sharkey, T. D. (1993). Isoprene emission by plants is affected by transmissible wound signals. Plant Cell Environ. 16, 563–570. doi: 10.1111/j.1365-3040.1993.tb00904.x
McClain, A. M., and Sharkey, T. D. (2019). Triose phosphate utilization and beyond: from photosynthesis to end-product synthesis. J. Exp. Bot. 70, 1755–1766. doi: 10.1093/jxb/erz058
Monson, R. K., Harley, P. C., Litvak, M. E., Wildermuth, M., Guenther, A. B., Zimmerman, P. R., et al. (1994). Environmental and developmental controls over the seasonal pattern of isoprene emission from aspen leaves. Oecologia 99, 260–270. doi: 10.1007/BF00627738
Monson, R. K., Jaeger, C. H., Adams, W. W., Driggers, E. M., Silver, G. M., and Fall, R. (1992). Relationships among isoprene emission rate, photosynthesis, and isoprene synthase activity as influenced by temperature. Plant Physiol. 98, 1175–1180. doi: 10.1104/pp.98.3.1175
Monson, R. K., Neice, A. A., Trahan, N. A., Shiach, I., McCorkel, J. T., and Moore, D. J. (2016). Interactions between temperature and intercellular CO2 concentration in controlling leaf isoprene emission rates. Plant Cell Environ. 39, 2404–2413. doi: 10.1111/pce.12787
Monson, R. K., Trahan, N., Rosenstiel, T. N., Veres, P., Moore, D., Wilkinson, M., et al. (2007). Isoprene emission from terrestrial ecosystems in response to global change: minding the gap between models and observations. Philos. Trans. A Math. Phys. Eng. Sci. 365, 1677–1695. doi: 10.1098/rsta.2007.2038
Morfopoulos, C., Sperlich, D., Penuelas, J., Filella, I., Llusia, J., Medlyn, B. E., et al. (2014). A model of plant isoprene emission based on available reducing power captures responses to atmospheric CO2. New Phytol. 203, 125–139. doi: 10.1111/nph.12770
Murthy, R., Barron-Gafford, G., Dougherty, P. M., Engel, V. C., Grieve, K., Handley, L., et al. (2005). Increased leaf area dominates carbon flux response to elevated CO2 in stands of Populus deltoides (Bartr.). Glob. Chang. Biol. 11, 716–731. doi: 10.1111/j.1365-2486.2005.00923.x
Mutanda, I., Inafuku, M., Saitoh, S., Iwasaki, H., Fukuta, M., Watanabe, K., et al. (2016). Temperature controls on the basal emission rate of isoprene in a tropical tree Ficus septica: exploring molecular regulatory mechanisms. Plant Cell Environ. 39, 2260–2275. doi: 10.1111/pce.12797
Niinemets, U., Tenhunen, J. D., Harley, P. C., and Steinbrecher, R. (1999). A model of isoprene emission based on energetic requirements for isoprene synthesis and leaf photosynthetic properties for Liquidambar and Quercus. Plant Cell Environ. 22, 1319–1335. doi: 10.1046/j.1365-3040.1999.00505.x
Pachauri, R. K., Allen, M. R., Barros, V. R., Broome, J., Cramer, W., Christ, R., et al. (2014). Climate Change 2014: Synthesis Report. Contribution of Working Groups I, II and III to the Fifth Assessment Report of the Intergovernmental Panel on Climate Change. Geneva: IPCC.
Pacifico, F., Harrison, S. P., Jones, C. D., and Sitch, S. (2009). Isoprene emissions and climate. Atmos. Environ. 43, 6121–6135. doi: 10.1016/j.atmosenv.2009.09.002
Pegoraro, E., Potosnak, M., Monson, R., Rey, A., Barron-Gafford, G., and Osmond, C. (2007). The effect of elevated CO2, soil and atmospheric water deficit and seasonal phenology on leaf and ecosystem isoprene emission. Funct. Plant Biol. 34, 774–784. doi: 10.1071/FP07021
Pegoraro, E., Rey, A., Barron-Gafford, G., Monson, R., Malhi, Y., and Murthy, R. (2005). The interacting effects of elevated atmospheric CO2 concentration, drought and leaf-to-air vapour pressure deficit on ecosystem isoprene fluxes. Oecologia 146, 120–129. doi: 10.1007/s00442-005-0166-5
Pike, R. C., and Young, P. J. (2009). How plants can influence tropospheric chemistry: the role of isoprene emissions from the biosphere. Weather 64, 332–336. doi: 10.1002/wea.416
Possell, M., and Hewitt, C. N. (2011). Isoprene emissions from plants are mediated by atmospheric CO2 concentrations. Glob. Chang. Biol. 17, 1595–1610. doi: 10.1111/j.1365-2486.2010.02306.x
Possell, M., Hewitt, C. N., and Beerling, D. J. (2005). The effects of glacial atmospheric CO2 concentrations and climate on isoprene emissions by vascular plants. Glob. Chang. Biol. 11, 60–69. doi: 10.1111/j.1365-2486.2004.00889.x
Potosnak, M. J., Lestourgeon, L., and Nunez, O. (2014a). Increasing leaf temperature reduces the suppression of isoprene emission by elevated CO2 concentration. Sci. Total Environ. 481, 352–359. doi: 10.1016/j.scitotenv.2014.02.065
Potosnak, M. J., LeStourgeon, L., Pallardy, S. G., Hosman, K. P., Gu, L. H., Karl, T., et al. (2014b). Observed and modeled ecosystem isoprene fluxes from an oakdominated temperate forest and the influence of drought stress. Atmos. Environ. 84, 314–322. doi: 10.1016/j.atmosenv.2013.11.055
Purves, D. W., Caspersen, J. P., Moorcroft, P. R., Hurtt, G. C., and Pacala, S. W. (2004). Human-induced changes in US biogenic volatile organic compound emissions: evidence from long-term forest inventory data. Glob. Chang. Biol. 10, 1737–1755. doi: 10.1111/j.1365-2486.2004.00844.x
Rapparini, F., Baraldi, R., Miglietta, F., and Loreto, F. (2004). Isoprenoid emission in trees of Quercus pubescens and Quercus ilex with lifetime exposure to naturally high CO2 environment. Plant Cell Environ. 27, 381–391. doi: 10.1111/j.1365-3040.2003.01151.x
Rasulov, B., Bichele, I., Hüve, K., Vislap, V., and Niinemets, Ü. (2015). Acclimation of isoprene emission and photosynthesis to growth temperature in hybrid aspen: resolving structural and physiological controls. Plant Cell Environ. 38, 751–766. doi: 10.1111/pce.12435
Rasulov, B., Copolovici, L., Laisk, A., and Niinemets, U. (2009a). Postillumination isoprene emission: in vivo measurements of dimethylallyldiphosphate pool size and isoprene synthase kinetics in aspen leaves. Plant Physiol. 149, 1609–1618. doi: 10.1104/pp.108.133512
Rasulov, B., Hüve, K., Bichele, I., Laisk, A., and Niinemets, U. (2010). Temperature response of isoprene emission in vivo reflects a combined effect of substrate limitations and isoprene synthase activity: a kinetic analysis. Plant Physiol. 154, 1558–1570. doi: 10.1104/pp.110.162081
Rasulov, B., Hüve, K., Välbe, M., Laisk, A., and Niinemets, U. (2009b). Evidence that light, carbon dioxide, and oxygen dependencies of leaf isoprene emission are driven by energy status in hybrid aspen. Plant Physiol. 151, 448–460. doi: 10.1104/pp.109.141978
Rasulov, B., Talts, E., Bichele, I., and Niinemets, Ü. (2018). Evidence that isoprene emission is not limited by cytosolic metabolites. Exogenous malate does not invert the reverse sensitivity of isoprene emission to high [CO2]. Plant Physiol. 176, 1573–1586. doi: 10.1104/pp.17.01463
Rasulov, B., Talts, E., and Niinemets, U. (2016). Spectacular oscillations in plant isoprene emission under transient conditions explain the enigmatic CO2 response. Plant Physiol. 172, 2275–2285. doi: 10.1104/pp.16.01002
Rey, A., and Jarvis, P. G. (1998). Long-term photosynthetic acclimation to increased atmospheric CO2 concentration in young birch (Betula pendula) trees. Tree Physiol. 18, 441–450. doi: 10.1093/treephys/18.7.441
Rosenstiel, T. N., Ebbets, A. L., Khatri, W. C., Fall, R., and Monson, R. K. (2004). Induction of poplar leaf nitrate reductase: a test of extrachloroplastic control of isoprene emission rate. Plant Biol. 6, 12–21. doi: 10.1055/s-2003-44722
Rosenstiel, T. N., Potosnak, M. J., Griffin, K. L., Fall, R., and Monson, R. K. (2003). Increased CO2 uncouples growth from isoprene emission in an agriforest ecosystem. Nature 421, 256–259. doi: 10.1038/nature01312
Scholefield, P. A., Doick, K. J., Herbert, B. M. J., Hewitt, C. N., Schnitzler, J. P., Pinelli, P., et al. (2004). Impact of rising CO2 on emissions of volatile organic compounds: isoprene emission from Phragmites australis growing at elevated CO2 in a natural carbon dioxide spring. Plant Cell Environ. 27, 393–401. doi: 10.1111/j.1365-3040.2003.01155.x
Sharkey, T. D. (1985). Photosynthesis in intact leaves of C3 plants: physics, physiology and rate limitations. Bot. Rev. 51, 53–105. doi: 10.1007/BF02861058
Sharkey, T. D., Chen, X., and Yeh, S. (2001). Isoprene increases thermotolerance of fosmidomycin-fed leaves. Plant Physiol. 125, 2001–2006. doi: 10.1104/pp.125.4.2001
Sharkey, T. D., Gray, D. W., Pell, H. K., Breneman, S. R., and Topper, L. (2013). Isoprene synthase genes form a monophyletic clade of acyclic terpene synthases in the TPS-B terpene synthase family. Evolution 67, 1026–1040. doi: 10.1111/evo.12013
Sharkey, T. D., Loreto, F., and Delwiche, C. F. (1991). High carbon dioxide and sun/shade effects on isoprene emission from oak and aspen tree leaves. Plant Cell Environ. 14, 333–338. doi: 10.1111/j.1365-3040.1991.tb01509.x
Sharkey, T. D., Singsaas, E. L., Lerdau, M. T., and Geron, C. D. (1999). Weather effects on isoprene emission capacity and applications in emissions algorithms. Ecol. Appl. 9, 1132–1137. doi: 10.1890/1051-0761(1999)009[1132:WEOIEC]2.0.CO;2
Sharkey, T. D., Singsaas, E. L., Vanderveer, P. J., and Geron, C. (1996). Field measurements of isoprene emission from trees in response to temperature and light. Tree Physiol. 16, 649–654. doi: 10.1093/treephys/16.7.649
Sharkey, T. D., Stitt, M., Heineke, D., Gerhardt, R., Raschke, K., and Heldt, H. W. (1986). Limitation of photosynthesis by carbon metabolism: II O2-insensitive CO2 uptake results from limitation of triose phosphate utilization. Plant Physiol. 81, 1123–1129. doi: 10.1104/pp.81.4.1123
Sharkey, T. D., Wiberley, A. E., and Donohue, A. R. (2008). Isoprene emission from plants: why and how. Ann. Bot. 101, 5–18. doi: 10.1093/aob/mcm240
Silver, G. M., and Fall, R. (1995). Characterization of aspen isoprene synthase, an enzyme responsible for leaf isoprene emission to the atmosphere. J. Biol. Chem. 270, 13010–13016. doi: 10.1074/jbc.270.22.13010
Singsaas, E. L., and Sharkey, T. D. (1998). The regulation of isoprene emission responses to rapid leaf temperature fluctuations. Plant Cell Environ. 21, 1181–1188. doi: 10.1046/j.1365-3040.1998.00380.x
Staudt, M., Morin, X., and Chuine, I. (2016). Contrasting direct and indirect effects of warming and drought on isoprenoid emissions from Mediterranean oaks. Reg. Environ. Change 17, 2121–2133. doi: 10.1007/s10113-016-1056-6
Sun, Z., Niinemets, U., Huve, K., Noe, S., Rasulov, B., Copolovici, L., et al. (2012). Enhanced isoprene emission capacity and altered light responsiveness in aspen grown under elevated atmospheric CO2 concentration. Glob. Chang. Biol. 18, 3423–3440. doi: 10.1111/j.1365-2486.2012.02789.x
Sun, Z. H., Hüve, K., Vislap, V., and Niinemets, Ü. (2013). Elevated [CO2] magnifies isoprene emissions under heat and improves thermal resistance in hybrid aspen. J. Exp. Bot. 64, 5509–5523. doi: 10.1093/jxb/ert318
Takizawa, K., Cruz, J. A., Kanazawa, A., and Kramer, D. M. (2007). The thylakoid proton motive force in vivo. Quantitative, non-invasive probes, energetics, and regulatory consequences of light-induced pmf. Biochim. Biophys. Acta. 1767, 1233–1244. doi: 10.1016/j.bbabio.2007.07.006
Taylor, T. C., McMahon, S. M., Smith, M. N., Boyle, B., Violle, C., van Haren, J., et al. (2018). Isoprene emission structures tropical tree biogeography and community assembly responses to climate. New Phytol. 220, 435–446. doi: 10.1111/nph.15304
Tognetti, R. (1998). Response of foliar metabolism in mature trees of Quercus pubescens and Quercus ilex to long-term elevated CO2. Environ. Exp. Bot. 39, 233–245. doi: 10.1016/S0098-8472(98)00013-6
Velikova, V., Ghirardo, A., Vanzo, E., Merl, J., Hauck, S. M., and Schnitzler, J. P. (2014). Genetic manipulation of isoprene emissions in poplar plants remodels the chloroplast proteome. J. Proteome Res. 13, 2005–2018. doi: 10.1021/pr401124z
Vickers, C. E., Possell, M., Cojocariu, C. I., Velikova, V. B., Laothawornkitkul, J., Ryan, A., et al. (2009). Isoprene synthesis protects transgenic tobacco plants from oxidative stress. Plant Cell Environ. 32, 520–531. doi: 10.1111/j.1365-3040.2009.01946.x
Way, D. A., Ghirardo, A., Kanawati, B., Esperschütz, J., Monson, R. K., Jackson, R. B., et al. (2013). Increasing atmospheric CO2 reduces metabolic and physiological differences between isoprene- and non-isoprene-emitting poplars. New Phytol. 200, 534–546. doi: 10.1111/nph.12391
Wiberley, A. E., Donohue, A. R., Meier, M. E., Westphal, M. M., and Sharkey, T. D. (2008). Regulation of isoprene emission in Populus trichocarpa leaves subjected to changing growth temperature. Plant Cell Environ. 31, 258–267. doi: 10.1111/j.1365-3040.2007.01758.x
Wiberley, A. E., Donohue, A. R., Westphal, M. M., and Sharkey, T. D. (2009). Regulation of isoprene emission from poplar leaves throughout a day. Plant Cell Environ. 32, 939–947. doi: 10.1111/j.1365-3040.2009.01980.x
Wilkinson, M. J., Monson, R. K., Trahan, N., Lee, S., Brown, E., Jackson, R. B., et al. (2009). Leaf isoprene emission rate as a function of atmospheric CO2 concentration. Glob. Chang. Biol. 15, 1189–1200. doi: 10.1111/j.1365-2486.2008.01803.x
Witt, H. T. (1979). Energy conversion in the functional membrane of photosynthesis. Analysis by light pulse and electric pulse methods. Biochim Biophys Acta Rev Bioenerg. 505, 355–427. doi: 10.1016/0304-4173(79)90008-9
Yang, J. T., Preiser, A. L., Li, Z., Weise, S. E., and Sharkey, T. D. (2016). Triose phosphate use limitation of photosynthesis: short-term and long-term effects. Planta 243, 687–698. doi: 10.1007/s00425-015-2436-8
Young, P. J., Arneth, A., Schurgers, G., Zeng, G., and Pyle, J. A. (2009). The CO2 inhibition of terrestrial isoprene emission significantly affects future ozone projections. Atmos. Chem. Phys. 9, 2793–2803. doi: 10.5194/acp-9-2793-2009
Zhang, Y., Huang, J.-P., Henze, D. K., and Seinfeld, J. H. (2007). Role of isoprene in secondary organic aerosol formation on a regional scale. J. Geophys. Res. 112. doi: 10.1029/2007JD008675
Zhou, C., Li, Z., Wiberley-Bradford, A. E., Weise, S. E., and Sharkey, T. D. (2013). Isopentenyl diphosphate and dimethylallyl diphosphate/isopentenyl diphosphate ratio measured with recombinant isopentenyl diphosphate isomerase and isoprene synthase. Anal. Biochem. 440, 130–136. doi: 10.1016/j.ab.2013.05.028
Keywords: isoprene, CO2, high temperature, climate change, triose phosphate utilization limitation, chloroplast
Citation: Lantz AT, Solomon C, Gog L, McClain AM, Weraduwage SM, Cruz JA and Sharkey TD (2019) Isoprene Suppression by CO2 Is Not Due to Triose Phosphate Utilization (TPU) Limitation. Front. For. Glob. Change 2:8. doi: 10.3389/ffgc.2019.00008
Received: 16 January 2019; Accepted: 25 March 2019;
Published: 12 April 2019.
Edited by:
Mark Potosnak, DePaul University, United StatesReviewed by:
Mauro Centritto, Istituto per la Valorizzazione del Legno e Delle Specie Arboree (IVALSA), ItalyVioleta Velikova, Institute of Plant Physiology and Genetics (BAS), Bulgaria
Copyright © 2019 Lantz, Solomon, Gog, McClain, Weraduwage, Cruz and Sharkey. This is an open-access article distributed under the terms of the Creative Commons Attribution License (CC BY). The use, distribution or reproduction in other forums is permitted, provided the original author(s) and the copyright owner(s) are credited and that the original publication in this journal is cited, in accordance with accepted academic practice. No use, distribution or reproduction is permitted which does not comply with these terms.
*Correspondence: Thomas D. Sharkey, dHNoYXJrZXlAbXN1LmVkdQ==