Using Direct Phloem Transport Manipulation to Advance Understanding of Carbon Dynamics in Forest Trees
- 1Department of Organismic and Evolutionary Biology, Harvard University, Cambridge, MA, United States
- 2School of Informatics, Computing, and Cyber Systems, Northern Arizona University, Flagstaff, AZ, United States
- 3Center for Ecosystem Science and Society, Northern Arizona University, Flagstaff, AZ, United States
- 4Department of Geography, University of Cambridge, Cambridge, United Kingdom
- 5Swiss Federal Research Institute for Forest, Snow and Landscape Research WSL, Birmensdorf, Switzerland
Carbon dynamics within trees are intrinsically important for physiological functioning, in particular growth and survival, as well as ecological interactions on multiple timescales. Thus, these internal dynamics play a key role in the global carbon cycle by determining the residence time of carbon in forests via allocation to different tissues and pools, such as leaves, wood, storage, and exudates. Despite the importance of tree internal carbon dynamics, our understanding of how carbon is used in trees, once assimilated, has major gaps. The primary tissue that transports carbon from sources to sinks within a tree is the phloem. Therefore, direct phloem transport manipulation techniques have the potential to improve understanding of numerous aspects of internal carbon dynamics. These include relationships between carbon assimilation, nonstructural carbon availability, respiration for growth and tissue maintenance, allocation to, and remobilization from, storage reserves, and long-term sequestration in lignified structural tissues. This review aims to: (1) introduce the topic of direct phloem transport manipulations, (2) describe the three most common methods of direct phloem transport manipulation and review their mechanisms, namely (i) girdling, (ii) compression and (iii) chilling; (3) summarize the known impacts of these manipulations on carbon dynamics and use in forest trees; (4) discuss potential collateral effects and compare the methods; and finally (5) highlight outstanding key questions that relate to tree carbon dynamics and use, and propose ways to address them using direct phloem transport manipulation.
Introduction
Trees contain the majority of vegetation carbon worldwide (Pan et al., 2013), and forests, dominated and defined by trees, contribute the largest share to the global terrestrial gross primary productivity (Beer et al., 2010). While we have a mechanistic understanding of how carbon is fixed from the atmosphere, our understanding of the fate of assimilated carbon within a forest tree is much poorer (Friend et al., 2014). On one hand, carbon is transported via the phloem to the lateral meristem, where it can be sequestered in lignified structural tissues for centuries, on the other hand it may almost immediately return to the atmosphere through respiration. Understanding the mechanisms underlying internal carbon dynamics and use in trees (i.e., why, when, and how much carbon is allocated to different tissues and functions), is fundamentally linked to a wide range of physiological processes, such as respiration for growth and tissue maintenance, and remobilization of storage reserves, long-term sequestration in lignified structural tissues, and even feedbacks on carbon assimilation. Beyond the trees themselves, phloem transport and tree carbon allocation are also intrinsically linked to the whole ecosystem through processes such as carbon export to mycorrhizae and the rhizosphere. An integral knowledge of carbon dynamics and use within mature forest trees is fundamental to address questions about the carbon cycle at the forest scale, because it contributes to debates about forest productivity (Körner, 2006), mortality (McDowell et al., 2008; Steinkamp and Hickler, 2015), and hence resilience in the face of environmental change. Indeed, at a global scale, the residence time of carbon in vegetation on land, which is largely a function of internal carbon dynamics and use, is the single largest source of uncertainty in projections of global vegetation carbon under future climate and atmospheric CO2 (Friend et al., 2014). Most global vegetation models project a significant increase in plant growth and land carbon due to CO2-fertilization of photosynthesis (Cramer et al., 2001; Sitch et al., 2008). The prevailing modeling paradigm, also known as source-limitation (Fatichi et al., 2014), simply assumes that carbon gains due to the enhanced assimilation are proportionally passed down to growth in trees, thereby being sequestered for long-periods. However, this paradigm neither comprehensively reflects, nor is entirely compatible with, current knowledge of the controls of plant growth (Körner, 2015). In fact, an increasing number of studies ascribe primary importance to direct effects of environmental conditions on the activity of sink processes, such as wood formation (Rossi et al., 2008). Understanding carbon dynamics and use within forest trees is thus of paramount importance, not least to inform models and improve projections of the global carbon cycle.
Our eco-physiological knowledge relies strongly on studies performed on herbaceous plants, in which feedbacks and dynamics of sinks and sources of carbon have been extensively researched (reviewed by White et al., 2016). While carbon dynamics and use within a tree are likely dominated by a similar set of processes as in herbaceous plants, there are at least two significant differences: trees have comparatively large stored reserves of nonstructural carbon (e.g., Furze et al., 2018) and much longer signaling and transport pathways. Thus, caution must be exercised when extrapolating from studies of herbaceous plants to infer carbon dynamics and use in trees. Indeed, evidence from past phloem transport manipulations suggests that, like herbaceous plants, trees actively manage source and sink tissues (i.e., Poirier-Pocovi et al., 2018), thus they are not adequately represented by the prevailing modeling paradigm, that models sink activity as a proportion of source activity (Friend et al., 1997; Cramer et al., 2001; Smith et al., 2001; Ito and Oikawa, 2002; Sitch et al., 2003; Krinner et al., 2005; Inatomi et al., 2010; Tian et al., 2011).
In trees and herbaceous plants alike, carbon—mainly in the form of sucrose—moves along hydrostatic gradients in the phloem, from regions of high (normally near carbon source tissues) to low (normally near carbon sink tissues) concentrations as a result of osmotic effects [originally postulated by Münch (1927, 1930) and reviewed by De Schepper et al., 2013b]. Consequently, carbon asserts dual importance; first as a driver of phloem transport and second as a transported resource for growth, energy supply, and signaling. Although other tissues, such as the xylem, can transport substantial amounts of carbon for a part of the year in species such as Acer saccharum (Larochelle et al., 1998) and Betula pendula (Sauter and Ambrosius, 1986), carbon in trees is overwhelmingly transported via the phloem (Jensen et al., 2016). Since the phloem acts as the main conduit of transport between sources and sinks of carbon within trees, direct manipulations of phloem transport, such as girdling, phloem compression and phloem chilling (Figure 1), are proposed as a cost-effective and logistically simple method to restrict flow from sources to sinks, and study the different impacts of variations in carbon availability on upstream and downstream regions. Some alternative experimental techniques, such isotope labeling (Högberg et al., 2007) and CO2 enrichment (Norby et al., 2004), to investigate carbon dynamics and use in mature, long-lived forest trees are complex and involve expensive and time-consuming approaches. The simple and more complex methods can be complemented by other techniques that manipulate source and sink strength, such as canopy shading (O'Brien et al., 2014), defoliation (Wiley et al., 2013, 2017) or genetic manipulations of translocation (Payyavula et al., 2011). However, for the scope of this review we focus on the three aforementioned methods of direct phloem transport manipulation (i.e., girdling, compression, and chilling), because they are relatively easily implemented and still have a large unmet potential to contribute to our understanding of carbon dynamics and use in forest trees.
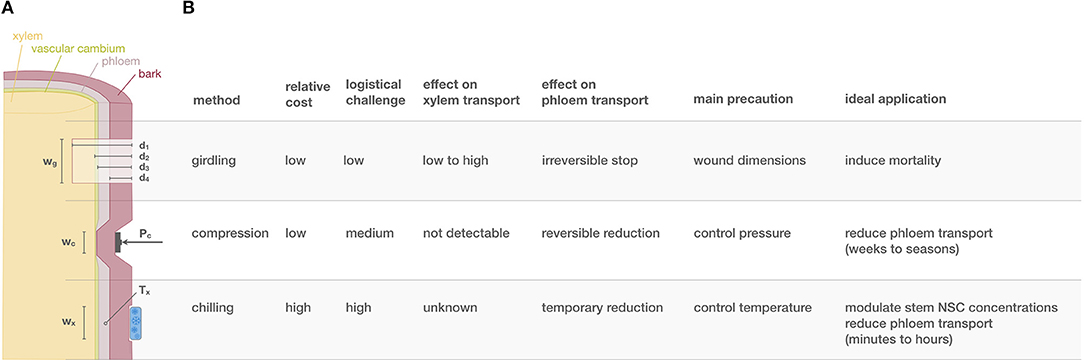
Figure 1. Provides a summary of key facts about different phloem transport manipulations. In particular, (A) illustrates a cross-sectional woody organ being girdled, compressed and chilled. The table in (B) summarizes key facts for the three most applied methods ranked from the most invasive (girdling) to the least invasive (phloem chilling). wg, wc, and wx mark the width of the zone for which tissue is affected during girdling, compression, and chilling, respectively. d marks the depth of girdling with four distinct categories (subscripts 1−4), that determine which tissues are affected. d1 includes destroying of bark, phloem, cambium and part of the functioning xylem, d2 spares the xylem, d3 only destroys the bark and phloem and d4 only the bark. Pc is the normal pressure applied during compression. Tx is the temperature experienced by the phloem during chilling.
Direct phloem transport manipulation, in particular girdling, have a rich history with first mentions by Theophrastus more than 2,000 years ago (Goren et al., 2004), and published studies on the subject going back more than 250 years (Fitzgerald, 1761). Since Fitzgerald's work on fruiting in 1761, girdling has also become a commonly practiced method in horticulture with many studies exploring its effects on reproductive effort (as reviewed by Goren et al., 2004). Despite its long and rich tradition, the fact that species and cultivars have been artificially selected for reproductive properties, and organisms are grown under highly manipulated, artificial conditions (i.e., irrigation, fertilization, low competition) limits the transferability of gained physiological knowledge to forest trees growing in a natural environment. While acknowledging the large body of horticultural literature on phloem transport manipulations on reproduction, this review focuses on studies performed to understand forest tree physiology. In turn, the issues discussed here, such as unreported methods and tree mortality, may not apply as strongly to horticultural studies. Across all disciplines, inconsistent methods and reporting have hindered the unambiguous interpretation of numerous experimental outcomes. For example, basic terms such as girdling, ring-barking, ringing, banding, and notching have been used interchangeably and inconsistently in the literature. Furthermore, previous definitions such as provided by Goren et al. (2004) have focussed on methods rather than mechamism. For example, Goren et al.'s (2004) definition of “strangulation” prescribes the instrument (here a steel wire), but does not define the pressure that needs to be applied. In general, important details have not always been reported and norms vary between sub-field (horticulture vs. silviculture). Girdling studies often omit to report whether the cambium was removed or not, or whether the phloem was fully, partially or not at all removed. This is important as the extent of cambial and phloem removal strongly affects the interpretation of experimental outcomes, as they control the potential for recovery of phloem flow in at least some species (Pang et al., 2008; Zhang et al., 2011; Chen et al., 2014), as well as the effective reduction of phloem transport (De Schepper et al., 2013a; Asao and Ryan, 2015; Jiménez et al., 2017). In fact, partial application (i.e., around only part of the circumference), even over three quarters of the circumference, has not resulted in a detectable reduction of phloem transport (Hossain et al., 2004; De Schepper et al., 2013a; Asao and Ryan, 2015; Jiménez et al., 2017). Trees that survive girdling have often been only partially girdled, or the cambium was only removed over a sufficiently narrow strip, so that regrowth and recovery were possible (Annighöfer et al., 2012). Nevertheless, most trees ultimately die due to stresses induced by complete girdling of the main bole, although in extreme cases death can be postponed by decades (Noel, 1970). Hence, omitting crucial details, such as which tissues were destroyed during girdling, compromises the reproducibility and interpretability of studies manipulating phloem transport.
We hope that this paper stimulates greater reproducibility and interpretability of future direct phloem transport manipulations studies. To this end, we focus on three established techniques, namely (i) girdling, (ii) compression, and (iii) chilling. We define these terms and describe their mechanisms and methodological details. We discuss the impacts of phloem transport manipulation, raise some caveats and challenges associated with these techniques and briefly compare the three methods. Finally, we highlight a set of outstanding questions regarding carbon dynamics and use in trees and propose ways to address these questions using phloem transport manipulation.
Description of Techniques to Manipulate Phloem Transport in Trees
Girdling, phloem compression and phloem chilling (Figure 1) are the most common techniques to directly manipulate phloem transport and have been applied for very different purposes (see list of references and objectives over the last decade in Supplementary Table 1). While girdling has been used in numerous publications for a wide range of applications such as accelerating and investigating successional dynamics (Hardiman et al., 2013) or studies on priorities of nonstructural carbon allocation (Oberhuber et al., 2017), phloem compression and chilling have been pioneered only in recent years with regard to forest tree physiology (important aspects of key studies are listed in Table 1). To our knowledge, phloem compression has been applied only in one published study, on young and mature Pinus sylvestris (Henriksson et al., 2015), while phloem chilling has been reported in one laboratory study on young Quercus robur (De Schepper et al., 2011), as well as on mature plantation-grown Pinus taeda (Johnsen et al., 2007), and on individual branches of naturally-growing mature Acer saccharum (Schaberg et al., 2017).
Definitions
Girdling
Girdling (variations are also known as barking, ring-barking, ringing, banding, cincturing, hacking, frilling, scoring, stripping, and notching) is the circumferential destruction of a band of surface tissue with a specific width (wg in Figure 1) and depth (d in Figure 1) around the entire woody branch, stem or root.
Girdling stops phloem flow by simply destroying or removing the conduits. For girdling, the depth of the wound and, more importantly the nature of the destroyed and damaged tissues (bark, cambium, phloem, and/or xylem), determines the potential for recovery and the effectiveness of the phloem transport disruption. In forestry, girdling is often performed using chainsaws or axes to kill trees (Bauhus et al., 2009) or induce desired wood properties (Gref and Ståhl, 1994; Taylor and Cooper, 2002). With such crude methods, the functioning xylem is generally damaged (see Figure 2B). In contrast, horticultural girdling is generally performed with specifically designed tools, such as double-bladed knives, to improve crop quantity and quality of fruits without substantial permanent damage and does not destroy the cambium or xylem (Goren et al., 2004).
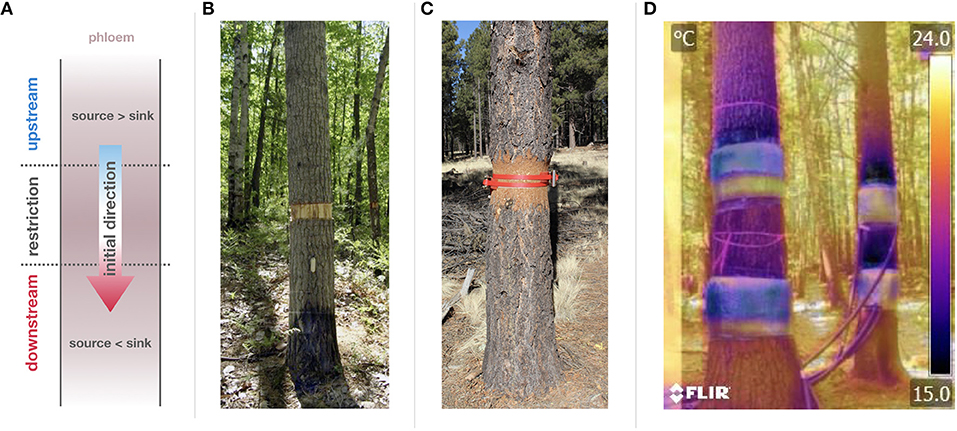
Figure 2. (A) The phloem transport manipulation (which applied in the restriction zone) block or reduce the regular phloem flow causing an increase of the source to sink ratio in the upstream regions and a decrease in downstream regions. Upstream and downstream are defined in accordance with the initial direction of flow, as indicated. (B) Displays a girdled (executed by chainsaw) Populus grandidentata in the Forest Accelerated Succession Experiment (FASET) at the University of Michigan Biological Station. (C) Shows a mature Pinus ponderosa in the arboretrum of Northern Arizona University being compressed using two compression collars of ratcheted polyester webbing with a 180° rotational offset. (D) Shows an overlay of a thermal image and a photo to display the thermal gradient created when phloem chilling is applied (here to mature Pinus strobus at Harvard Forest).
Phloem Compression
Phloem compression (also known as strangulation or wiring) as a reversible method of phloem transport manipulation is the temporary application of a circumferential pressure that exceeds the phloem internal pressure, but does not cause permanent damage to the underlying cambium and xylem.
In contrast to girdling, which severs the phloem, compression restricts phloem flow by either collapsing phloem cells, or damaging them without permanent harm to the underlying cambium. The phloem cells appear able to resume normal function a few weeks after the release of compression in Pinus sylvestris and Pinus strobus, due to the re-opening, repair, or replacement of collapsed and/or damaged phloem cells (Henriksson et al., 2015). Phloem compression has been performed with heavy-duty steel bands (Henriksson et al., 2015) and ratcheted polyester cargo webbing (see Figure 2C). Phloem internal pressure, which needs to be overcome for effective transport reduction, is believed to range between one and two MPa (Wright and Fisher, 1980), but measurements of phloem internal pressure are difficult and have only been performed on a few species (e.g., Sovonick-Dunford et al., 1981; Nikinmaa et al., 2014).
Phloem Chilling
Phloem chilling (also known as cold, cold-block, or physiological girdling) as an effective method of phloem transport manipulation is defined as a reduction of the phloem temperature to just above the freezing point of water around the entire stem, branch or root without actually freezing any living tissue.
Phloem chilling causes a temporary blockage of phloem flow within the chilled section due to the exponential increase in sap viscosity with decreasing temperatures toward 0°C. This blockage of phloem flow is only maintained for minutes to hours (Gould et al., 2004; Thorpe et al., 2010). In fact, the increased resistance to flow in the chilled section is eventually overcome by a stronger osmotic gradient resulting from accumulation and depletion of sugar in upstream and downstream regions, respectively (Gould et al., 2004; Thorpe et al., 2010). Despite the mass flow eventually resuming, a large difference in sugar concentration across the zone of chilling persists until chilling is switched off (Ntsika and Delrot, 1986; Peuke et al., 2006; De Schepper et al., 2011).
Impacts of Phloem Transport Manipulations on Carbon Dynamics and Use
Direct phloem transport manipulation has been shown to effectively modify the availability of mobile carbon both upstream (i.e., in the region with a source activity previously exceeding sink activity; see Figure 2A) and downstream (i.e., the region with sink activity previously exceeding source activity; see Figure 2A) of the girdling, compression or chilling zone. This manipulation can trigger several feedbacks that can alter the carbon dynamics and use within a tree (Figure 3).
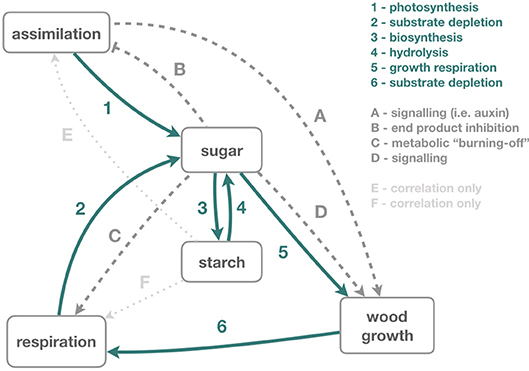
Figure 3. Illustrates the impacts of phloem transport manipulation on carbon dynamics and use. These changes are described in the main text (section Impacts of phloem transport manipulations on carbon dynamics and use). While some feedback processes are well understood (dark green solid arrows), others lack a mechanistic understanding (dark gray dashed), and some are only suspected (light gray dotted). (1) During assimilation, carbon from atmospheric CO2 increases leaf and phloem sugar concentrations. Sugar is transported, mainly via the phloem, to all living tissues. (2) In living tissues, sugar is respired to generate ATP, which reduces the local sugar concentrations, (3) and starch is synthesized from sugars locally for longer term storage. (4) These local stores can be remobilized by hydrolysis, especially in times of high demand. (5) Sugars in the phloem and adjacent xylem provide the raw materials to build cell walls during wood growth, which also (6) needs energy from respiration for the transformation of sugar to cell walls. (A) Wood growth is further affected by signaling compounds such as auxin. (B) At least, for sugar beet, a mechanism connecting high sucrose concentration in the phloem (and consequently in the leaves) and photosynthesis inhibition has been shown (Vaughn et al., 2002). (C) Respiration also seems to respond to locally high sugar concentrations by “burning-off” some sugar. (D) Sugar concentrations have been observed to correlate with wood growth but there is no evident causal relationship. Equally, changes in starch concentrations often correlate with changes in (E) assimilation and (F) respiration, but causal linkages are unclear.
A common outcome of phloem transport manipulation experiments is a reduction in the rate of photosynthesis (Myers et al., 1999; Iglesias et al., 2002; De Schepper and Steppe, 2011; De Schepper et al., 2011), which is indicative of the fact that carbon source activity can respond to the removal of sinks. Three different hypotheses have been proposed to explain photosynthetic inhibition (Figure 3, interaction B).
The first hypothesis proposes that sugar accumulation in leaves results in end-product inhibition (Iglesias et al., 2002), a common response to the removal of sinks (Paul and Foyer, 2001). To get high leaf sugar concentrations, a relative reduction in phloem loading compared to carbon assimilation is a prerequisite. Of those tree species that have been characterized, most have been determined to be symplastic loaders (Davidson et al., 2011; Liesche, 2017), which load the phloem passively by diffusion (Jensen et al., 2013). For passive loaders, a high phloem sugar concentration, as observed above the treatment zone for direct phloem transport manipulations, would be sufficient to reduce phloem loading. The reduced phloem loading would subsequently cause an accumulation of sugars in leaves, although there is some evidence for a short-term regulation of phloem export in passive loaders, that is independent of rates of photosynthesis and sugar remobilization (Liesche, 2017). In fact, a mechanistic pathway of photosynthesis inhibition, based on low sucrose export from leaves under high phloem sucrose concentrations, has been characterized in sugar beet (Vaughn et al., 2002), an herbaceous symplastic loader. For actively loading species, a reduction in leaf sugar export may not be achieved at all, but will at least be delayed due to their capacity to continue leaf sugar export even against high phloem sugar concentration (e.g., Oner-Sieben and Lohaus, 2014). The effects on photosynthesis of sugar accumulation in leaves due to reductions in phloem loading have been extensively studied in herbaceous plants (Ainsworth and Bush, 2011), but have rarely been studied in trees. In girdled Citrus reticulate trees, leaf sugar accumulation occurred, resulting in photosynthetic inhibition correlated with a reduced quantum yield in photosystem II (Rivas et al., 2007), but details of the biochemical pathways have not yet been elucidated.
The second hypothesis to explain photosynthetic inhibition proposes that girdling reduces the export of abscisic acid from leaves, and the abscisic acid accumulation, not the sugar accumulation, triggers photosynthetic inhibition via reductions in stomatal conductance (Asao and Ryan, 2015). Accumulation of abscisic acid in leaves after girdling has been observed in four tropical tree species (Hieronyma alchorneoides, Pentaclethra macroloba, Virola koschnyi, and Vochysia guatemalensis; Asao and Ryan, 2015) and in Pinus radiata (Mitchell et al., 2017). Girdling was also found to reduce stomatal conductance, which is a well know consequence of leaf-level accumulation of abscisic acid, in Pinus ponderosa (Domec and Pruyn, 2008).
The third hypothesis to explain photosynthetic inhibition proposes a mechanism related to increases in leaf starch concentrations (Figure 3, interaction F), as observed after girdling in Citrus unshiu (Iglesias et al., 2002) and Quercus petrea (Maunoury-Danger et al., 2010). There is evidence for various pathways of high leaf starch concentrations directly damaging the photosynthetic apparatus, which results in reduced photosynthesis for herbaceous plants (Paul and Foyer, 2001). Significantly higher leaf starch concentrations after stem girdling have been observed in Citrus reticulata (Rivas et al., 2007), Juglans regia (Moscatello et al., 2017) and Pinus taeda (Myers et al., 1999), but not in Populus deltoides (Regier et al., 2010). For Juglans regia (Moscatello et al., 2017), Populus deltoides (Regier et al., 2010), and Pinus taeda (Maier et al., 2010) increases of stem starch concentrations above and decreases below the girdling zone were also apparent. Presumably, the increase of phloem sugar and starch concentrations above the girdling zone would eventually lead to increased leaf starch concentrations for passively loading pines. Interestingly, for Pinus taeda the changes in stem starch concentration were only significant when the girdling was performed in spring, as opposed to autumn (Maier et al., 2010), indicating that photosynthetic activity during the peak growing season, or underlying seasonal fluctuations in nonstructural carbohydrate reserves (Richardson et al., 2013; Furze et al., 2019), might drive this change. Despite the evidence for changes in starch remobilization, evidence for the relationship between starch accumulation and photosynthesis inhibition in trees is merely correlative at the moment, but leaf chlorosis due to starch accumulation is a common symptom of girdling in the horticultural experiments (Goren et al., 2004). This leaves open the possibility that photosynthetic inhibition arises from concomitant changes in starch biosynthesis (Figure 3, interaction 3) and/or hydrolysis (Figure 3, interaction 4), which in turn are caused by changes in sugar availability in the phloem and leaves (hypothesis 1). The feedbacks, if driven by phloem sugar concentrations, are likely to vary between passively vs. actively loading species.
As implied above, phloem transport manipulation can induce the remobilization of nonstructural carbon reserves in adjacent tissues. Remobilization and use of nonstructural carbon reserves downstream of the girdling zone has been shown for Pinus taeda (Maier et al., 2010), Populus deltoides (Regier et al., 2010), Prunus persica (Jordan and Habib, 1996), Quercus petrea (Maunoury-Danger et al., 2010), and Scleronema micranthum (Muhr et al., 2018). Using radiocarbon dating of the respired CO2, Muhr et al. (2018) showed that such remobilized reserves after girdling were a decade old in the tropical species Scleronema micranthum. This capacity to remobilize even decade-old reserves (Vargas et al., 2009; Carbone et al., 2013) likely explains the large autonomy displayed by isolated woody organs after girdling (Hoch, 2005) and phloem chilling (Schaberg et al., 2017).
Remobilization and storage of nonstructural carbon after phloem transport manipulation in trees also partially drive alterations in respiratory rates (Figure 3, interactions C & F), which are consistently higher upstream and lower downstream in girdled (Domec and Pruyn, 2008; Maier et al., 2010; Maunoury-Danger et al., 2010), compressed (Henriksson et al., 2015), and chilled stems (De Schepper et al., 2011). Leaf respiration has also been shown to increase after girdling (Maunoury-Danger et al., 2010; Poirier-Pocovi et al., 2018) and phloem chilling (De Schepper et al., 2011). These changes in respiration rates result from a combination of changes in metabolic demand to fuel starch biosynthesis or hydrolysis (Figure 3, interaction F), and changes in growth respiration (Figure 3, interaction 6). However, the magnitude of respiration enhancement appears to exceed purely metabolic demands (Domec and Pruyn, 2008; Maier et al., 2010), and hence has been hypothesized to constitute a mechanism for “burning off” surplus mobile carbon (Figure 3, interaction C). Domec and Pruyn (2008) have argued that such a “burning-off” of carbon could help to reduce phloem sugar concentrations when a backlog develops after girdling. It is possible that this metabolic burning is a safety mechanism to avoid damage to the photosynthetic apparatus, due to high leaf sugar concentration. The excess respiration could use alternative metabolic pathways, such as alternative oxidases (Amthor, 2000), that do not result in a net production of ATP (Lambers and Ribas-Carbo, 2005). In conclusion, in phloem transport manipulations, respiration is down-regulated downstream and up-regulated upstream of the treatment zone, where it appears to exceed enhanced metabolic demand.
Direct phloem transport manipulation has also been shown to influence rates of wood formation, with girdling in trees always leading to enhanced wood formation upstream and gradual cessation of growth downstream (Wilson, 1968; Maier et al., 2010), which is also a common side-effect in horticultural girdling and compression treatments (Goren et al., 2004). For example, clear increases in rates of cell division, cell enlargement and cell wall thickening were found above the girdling zone, and decreased rates below the girdling zone in young Pinus sylvestris (Winkler and Oberhuber, 2017). Phloem chilling has also been shown to lead to increases in wood growth upstream and decreases in wood growth downstream of the chilling zone (De Schepper et al., 2011). These results have been interpreted to imply carbon limitation of wood growth (Figure 3, interaction D; Winkler and Oberhuber, 2017), but they could also result from changes in hormonal signaling (Figure 3, interaction A) or osmotically-induced changes in turgor due to variations in phloem sugar concentrations.
Finally, girdling has been used to block the export of current assimilates to roots, and hence reduce substrates for root growth, respiration, and exudation. This then permits partitioning of soil respiration into autotrophic and heterotrophic components (Högberg et al., 2001; Subke et al., 2004, 2011; Scott-Denton et al., 2006; Levy-Varon et al., 2012). The resulting partitioning estimates are likely biased, because of permanent damage to trees and enhanced fine root death. This enhanced decomposition due to root death has been hypothesized to partially (Högberg et al., 2009) or fully (Kuzyakov and Gavrichkova, 2010) compensate for reduced root respiration and exudation, leading to an underestimation of the autotrophic component. In contrast, Johnsen et al. (2007) observed only a small, but statistically significant, reduction in soil respiration following phloem chilling in Pinus taeda. At the ecosystem scale, girdling has also been used to study the coupling between carbon assimilation and soil respiration on timescales ranging from a few hours to days (Mencuccini and Hölttä, 2010), although such experiments need to be interpreted with care (Stoy et al., 2007; Risk et al., 2012).
Contrary to the implied exclusive control of carbon dynamics and use in trees by source activity (i.e., carbon supply), evidence outlined above paints a more nuanced picture with complex feedbacks and dependencies. Wood growth, respiration and remobilization of nonstructural carbon reserves are all affected by phloem transport manipulations and even photosynthesis is downregulated.
Collateral Effects and Practical Challenges
Despite all three manipulation techniques triggering similar effects on mobile carbon supply, some peculiarities intrinsic to each method have been identified. When choosing a method of direct phloem transport manipulation, these collateral impacts need to be considered, avoided, monitored and/or accounted for as much as possible.
Collateral Effects
The degree of invasiveness is a primary aspect differentiating the three methods. Girdling that damages the functioning xylem is by far the most invasive and least reversible, while phloem chilling is the least invasive and most quickly reversible (Figure 1). Wounding is likely to result from girdling as well as overly-aggressive and persistent compression or chilling treatments, which may ultimately lead to mortality. This damage triggers other (perhaps undesirable) physiological responses, including the production and translocation of signaling compounds, or the translocation of nutrients and water. In contrast to girdling, phloem compression over days to months is believed to be reversible. For example, after stem compression for a period of 27 days during the second half of the growing season, all treated trees (Pinus sylvestris) had fully recovered phloem functionality in the following spring (Henriksson et al., 2015). With phloem chilling, a potential risk is that temperatures below freezing might cause permanent damage to unhardened tissues—for example, freezing damage to the phloem and cambium could effectively girdle the tree.
Girdling has been shown to disrupt hormonal flows, including basipetal auxin transport (Dann et al., 1985) and acropetal abscisic acid, cytokinin, gibberellin, amino acid and amide transport (Skogerbo, 1992; Cutting and Lyne, 1993; Havelange et al., 2000; Mitchell et al., 2017). However, the influence of phloem compression and chilling on the flow of signaling compounds in trees is not known. Phloem compression might allow for some leakage across the treatment zone, especially given the non-uniform pressure distributions resulting from current methods. In fact, respiration rates downstream of the compression zone do not drop as low as in girdled trees (Henriksson et al., 2015), which arguably results from assimilate leakage. However, mobile carbon concentrations in the phloem downstream of the girdling and compression zones are similar (Henriksson et al., 2015). By comparison, phloem chilling only temporarily blocks phloem transport of mobile carbon, without clearly reducing concentrations of inorganic solutes such potassium and amino acids in downstream phloem sap, at least for Ricinus communis (Peuke et al., 2006).
Phloem transport manipulation is clearly accompanied by collateral effects on adjacent tissues, in particular water transport in the xylem. Phloem and xylem transport are known to interact (Steppe et al., 2015), so consequences of phloem transport manipulations for xylem transport are to be expected. In particular, it has been implied that leaving the xylem exposed to the atmosphere may cause air seeding of cavitation (Ueda et al., 2014) or tissue dehydration (Bloemen et al., 2014), but studies monitoring water transport after girdling detected no clear reduction of water transport within 1 year, if the xylem remains intact and is sealed (Maier et al., 2010; Hubbard et al., 2013). Nonetheless, protecting the wound by sealing it with paraffin film (e.g., Cernusak and Marshall, 2001), silicone grease (e.g., Christman et al., 2012) or aluminum foil (e.g., De Schepper and Steppe, 2011; Oberhuber et al., 2017) should be considered and reported. Given the lack of a detectable and acute reduction of water transport in girdled trees, compression treatments are unlikely to substantially affect water transport in the xylem. For chilling, the viscosity of water in the xylem depends on temperature and will be directly affected by chilling treatments. Whether this effect is substantial warrants more research. Overall, current evidence suggests that phloem transport manipulation allows the investigation of carbon dynamics without causing confounding effects driven by altered water transport.
The timing and duration of phloem transport manipulation can influence experimental outcomes. This is because the magnitude of phloem flow and the composition of phloem sap vary diurnally, seasonally, and interannually. Counterintuitively, preliminary evidence for girdling suggests that the seasonal timing of the girdling did not affect mortality (Percival and Smiley, 2015), or caused major differences in wood growth of girdled young Pinus sylvestris (Winkler and Oberhuber, 2017). In contrast, wood growth increased clearly above and decreased clearly below a chilling collar when phloem chilling was performed on ring-porous Quercus robur during the peak growing season, but not when chilling was performed during the early or late growing season (De Schepper et al., 2011). In Quercus petraea, also a ring-porous species, early season girdling followed by recovery did not result in a difference in wood growth compared to controls, but mid- and late-season girdling did (Maunoury-Danger et al., 2010). Spring girdling in Pinus taeda resulted in greater effects on stem respiration (e.g., increase above girdling and reduction below) than did autumn girdling (Maier et al., 2010). These seasonal differences corresponded to variations in stem soluble sugar and starch concentrations in girdled Pinus taeda (Maier et al., 2010), which was also the case for girdled Populus deltoides (Regier et al., 2010) and chilled Quercus robur (De Schepper et al., 2011), although it needs to be acknowledged that seasonal variations in nonstructural carbon concentrations are common for various species (Richardson et al., 2013; Furze et al., 2019). Overall, it is surprising, especially in locations with strong seasonality, that the timing of application in relation to reproductive and phenological cycles has not been unanimously observed to have strong effects on wood growth or tree mortality, especially given the importance of temperature for cambial re-activation (Oribe and Funada, 2017), hormonal signaling for cambial activity (Sorce et al., 2013), and the observed effects of treatment timing on respiration and nonstructural carbon remobilization. However, the limited number of studies with multiple timings and/or durations of phloem transport manipulation does not currently allow clear attribution of differences in outcomes to differences in phenology or morphology.
Practical Challenges
In addition to potential collateral effects with each of the three methods, there are various challenges associated with their implementation. The main challenge with girdling is the limitation of tissue destruction to the desired depth. Precise methods such as incision by razor blades take more time and require a good knowledge of the species' anatomy. Specifically designed instruments from horticulture could facilitate consistent and precise applications. Using a crude tool, such as an ax or chainsaw, is substantially quicker and easier (and suitable if tree mortality is the desired consequence), but will increase collateral effects through excessive tissue damage. If the cambium is not destroyed, regeneration of the phloem and bark can eventually occur in some species (Pang et al., 2008; Zhang et al., 2011; Chen et al., 2014), while wound closure is normally driven by callus cells proliferating from the edges until the callus tissue bridges the wound and re-differentiates into phloem and cambium. The duration until full regeneration appears to depend on the timing of the destruction, as observed in Pinus taeda (Maunoury-Danger et al., 2010). Given the wound closure dynamics, the dimensions of the wound are intuitively related to the probability of and duration until recovery, but we lack a quantitative understanding of this relationship. There are additional uncertainties associated with the timing of girdling (described above, section Collateral Effects), which pose challenges when designing a girdling study.
Phloem compression equally has some challenges. First, it is desirable to apply pressure uniformly around the stem, branch, or root, but this is difficult to achieve with current methods, because tree stems are rarely perfectly cylindrical. Further, if excessive pressure is applied and the cambium is permanently damaged, phloem compression may be equivalent to girdling. Second, fine tuning the pressure exerted by the collar is often difficult. In particular, when using ratcheted cargo webbing, the ratcheting mechanism used for tightening is, by design, not continuous. Third, the compression collar also exerts shear stresses, which can rupture the bark and underlying tissues. The likelihood of this damage can be minimized by pre-smoothing the bark and using, for example, polytetrafluouroethylene-based tape between the collar and the bark (e.g., Henriksson et al., 2015). In addition, stabilizing the collar, and tightening it slowly, is recommended to minimize unnecessary damage from shear stress. Any measures to minimize shear stress should be reported. Even when applied carefully, current techniques of phloem compression exert non-uniform pressure around the circumference. Metal bands, as pioneered by Henriksson et al. (2015), are particularly stiff, hence shear stress and surface irregularities result in substantial deviations from the desired pressure. The circumferential variation in pressure is generally less when using polyester cargo webbing, which can adapt to the shape of the stem, branch, or root. However, the metal ratchet used to tighten this webbing can itself be a source of shear stress and pressure variation over a smaller arc. One option is to use multiple collars, adjacent but rotationally offset, to homogenize circumferential pressure variation. Pressure around the circumference should be measured and reported whatever the exact setup. Fourth, weather and time may cause material fatigue of the compression collar given the high forces involved. This fatigue may result in a loss of pressure, possibly culminating in failure of the compression treatment. Fifth, relatively little is known about the amount of pressure required to collapse the phloem without causing permanent damage, leading to uncertainties about exactly how much pressure should be applied. Sixth, it is possible that the applied compression could have additional side-effects on adjacent tissues, such as the bark and xylem, or on phloem pressure gradients at the whole-tree level, with consequence that have not yet been studied.
Setting up a phloem chilling experiment also involves multiple challenges and uncertainties, as the method is new enough that answers to key questions are not yet documented in the literature. First, the necessary width of the chilling zone (wx in Figure 1) is still unknown (e.g., is there a minimum threshold to inhibit flow, is there a threshold above which collateral effects increase disproportionately, do such thresholds depend on tree size or sap flow?). Second, phloem chilling is most effective close to 0°C, but uncertainty remains how far and how long positive temperature deviations can be tolerated before the treatment ceases to be effective. Third, phloem chilling also reduces the temperature in adjacent tissues, such as xylem, cambium, and bark, with unknown implications. Any temperature reductions at the stem surface will propagate inwards and then predominantly upwards due to water transport in the xylem, resulting in three-dimensional temperature gradients along the stem. While the vertical gradients can be quantified using thermal imaging (e.g., Figure 2D), radial variations in temperature are more difficult to quantify and control for. Fourth, calculations of heat exchange when designing a study are complicated due to large uncertainties associated with the thermal properties of wood (Suleiman et al., 1999), variations in sap flow and the water content of bark, phloem and xylem (Steppe et al., 2015). Fifth, the thermal inertia of a chilling system is difficult to manage in field studies, particularly due to diurnal and day-to-day variations in ambient temperature. Positive temperature excursions during warm weather could result in flushes of carbon through the inadequately chilled phloem, while negative temperature deviations might freeze unhardened, living tissue and cause permanent damage (Vitasse et al., 2014). Consequently, the chilling capacity, and thus energy requirements, needed to adequately maintain effective phloem chilling treatments, even for medium-sized trees, in the field is substantial (e.g., roughly 60 kJ min−1 for a Pinus strobus with a 30 cm diameter at breast height assuming a maximal temperature difference between target temperature and ambient air temperature of 30 K). Any chilling system should include control loops that monitor phloem or bark temperature and adjust the chilling accordingly to maintain optimal chilling temperatures (e.g., just above freezing). Sixth, it is difficult to create a good interface—one that would maximize heat transfer—between chilling collars and phloem tissue. For example, whereas copper tubing conducts heat efficiently, it is difficult to shape to the rough exterior of a tree trunk, and the contact area of round tubing with the trunk is comparatively small.
Comparison
Girdling is a simple and low-cost method to manipulate phloem transport in mature and juvenile trees alike (e.g., Annighöfer et al., 2012). Phloem compression is, like girdling, a low-cost way to manipulate phloem transport over a period of weeks and can be applied to trees of various sizes (e.g., Henriksson et al., 2015). Unlike girdling, phloem compression is reversible, less invasive, and even when applied to the main bole, non-lethal. Thus, in many cases it is a good alternative to girdling because collateral effects are reduced. Phloem chilling is logistically the most challenging, and by far the most expensive, method to implement. However, the advantage of phloem chilling is that it provides an elegant switch (i.e., a quickly reversible method to manipulate phloem transport) without the application of mechanical force. Although phloem chilling is substantially more expensive than girdling or phloem compression, it is far less expensive than other methods typically used in carbon allocation studies (e.g., isotope labeling or CO2 enrichment). Because of logistical constraints, phloem chilling is better suited for intensive studies with a physiological focus and a relatively small sample size. Indeed, published studies of phloem chilling have been performed on just a few branches (Schaberg et al., 2017) or small trees (De Schepper et al., 2011). The one phloem chilling experiment on mid-sized mature trees (n = 12) took place in a Pinus taeda plantation (Johnsen et al., 2007). By comparison, girdling and phloem compression can more easily be applied to larger sample and organ sizes or more dispersed study designs (e.g., Högberg et al., 2001). The simplicity and low cost of girdling and phloem compression facilitate studies beyond the historic confines of laboratories and plantations of temperate forest trees (e.g., Asao and Ryan, 2015; Muhr et al., 2018). In conclusion, all three techniques still have potential to contribute substantially to the fledging field of phloem function and failure (Epron et al., 2019), as well as phloem recovery.
Reducing Uncertainties Associated With Collateral Effects
Although phloem transport manipulation treatments can provide novel insights into tree physiology, there is an urgent need to reduce uncertainties related to intrinsic collateral effects of these techniques. Reporting has not been consistent enough and these treatments (girdling excepted) have not been used widely enough yet to permit them to be considered “established” or “standard” methods. Additional research into both the optimal methodological details and the exact effects would be highly profitable and greatly inform the appropriate design and application of future experiments. For example, we recommend examining (i) whether, to what extent, and under what circumstances do these methods allow leakage of carbon across the treatment zone, and on what time scales; (ii) what substances continue to be transported, and what substances are blocked; (iii) what determines the potential for recovery of phloem transport (i.e., reversibility) after the treatment is stopped; and (iv) whether there are key treatment thresholds (e.g., wound dimensions of girdling, the applied pressure of compression, or the magnitude and duration of chilling) that need to be exceeded or avoided?
Outstanding Questions and Unmet Potential
Beyond questions concerning the methods themselves, key questions remain with regard to allocation and metabolic processes that determine the fate of carbon in trees. These remaining questions are fundamental to major uncertainties within our understanding of the global carbon cycle, such as the residence time of carbon in forests. In the following section, we discuss how phloem transport manipulation can be used to advance these important research avenues.
Photosynthesis Inhibition
As discussed, phloem transport manipulation has been shown to lead to a backup of mobile carbon in source tissues, which correlates with observations of photosynthetic downregulation (e.g., Iglesias et al., 2002; Rivas et al., 2007; De Schepper et al., 2011), confirming simulated feedback effects (Hölttä et al., 2017). This accumulation of mobile carbon provides a framework to test these simulated mechanisms of potential feedbacks between sink and source activity in trees. Phloem transport manipulation could be used to establish a mechanistic understanding of phloem loading under high mobile carbon concentrations in the phloem for passively and actively loading species and the subsequent inhibition of photosynthesis by addressing (i) whether and how increased mobile carbon concentration in the phloem translates to increased mobile carbon concentrations in leaves, (ii) what is the response curve of photosynthesis to mobile carbon concentrations in leaves, and (iii) whether these patterns differ for passively and actively loading species?
The backup of mobile carbon in the phloem resulting from phloem transport manipulations in passively loading species could be used to evaluate whether knowledge of leaf carbon export and photosynthesis downregulation from herbaceous plants is transferrable to trees. For example, phloem compression and phloem chilling could be used to isolate individual branches of a tree for varying durations creating a gradient of mobile carbon. This would allow the formulation of a response function of photosynthesis to mobile carbon concentrations in branches and leaves. Repeating the treatments starting at multiple dates throughout the season would further allow to account for variations due to phenological and reproductive cycles. A better understanding of the mechanism of feedbacks inhibiting photosynthesis under high mobile carbon concentrations is urgently needed for trees.
Carbon Remobilization Dynamics
Storage of assimilates, in the form of nonstructural carbohydrates, provides important reserves that enable trees to survive periods of dormancy or unfavorable growing conditions (Rosas et al., 2013; Dietze et al., 2014). Remobilization of stored reserves supports metabolic processes and growth when the supply of recent assimilates is insufficient. Phloem transport manipulation can be used to isolate stem sections from current assimilates as well as from stored reserves in distant organs (e.g., roots and branches). By forcing metabolic reliance on locally stored reserves, phloem transport manipulation could be used to study remobilization dynamics. For example, Muhr et al. (2018) found that control trees relied on recent assimilates to fuel stem respiration, whereas girdled trees relied on progressively older stored reserves. Experimental phloem transport manipulation could help answer a range of questions such as (i) under what circumstances does remobilization occur; (ii) what is the age and availability of stored reserves for remobilization; and (iii) does remobilization use reserves in reverse chronological order - the “last in, first out” paradigm (Lacointe et al., 1993; Carbone et al., 2013)—of when they were stored; (iv) how much mixing between younger and older reserves occurs in different tissues?
Starch biosynthesis has been shown to be enhanced with high local mobile carbon concentrations above the zone of girdling, whereas starch hydrolysis is amplified with low local mobile carbon concentrations below the zone of girdling (Maier et al., 2010; Maunoury-Danger et al., 2010; Regier et al., 2010; Moscatello et al., 2017). But, it is not known whether these changes are driven by local carbon availability, or by co-transported signaling compounds. Previous phloem transport manipulation studies have typically used just a single phloem transport bottleneck on each treated tree (with the exception of De Schepper et al., 2007 and Winkler and Oberhuber, 2017), but by creating two bottlenecks - a restriction above and a restriction below an effectively isolated stem section—it would be possible to cut off transport both down from the canopy and up from stored reserves in roots. This would lead to a hypothesized gradient in carbon availability, from highest above the top restriction, to intermediate below the bottom restriction, to lowest between the two restrictions. Wood growth and maintenance respiration of the isolated section would be dependent on the remobilization of local reserves, and radiocarbon dating could be used to determine the age of stored reserves used to support respiration (by trapping emitted CO2; e.g., Muhr et al., 2016, 2018) and new tissue production (by cellulose extraction; e.g., Carbone et al., 2013). This experiment would also enable inferences about internal mixing of stored reserves and the affinity of remobilization for more recently stored substrates (Carbone et al., 2013; Richardson et al., 2015). To the extent that the transport of carbon and signaling compounds may be differentially affected by girdling, phloem compression, and phloem chilling, such an experiment might yield different results depending on the phloem transport manipulation that is applied. While girdling has been recommended to address whether “regular” allocation is maintained under carbon limitation (Hartmann and Trumbore, 2016), and what the effects on carbon remobilization are (Muhr et al., 2016), we argue that phloem compression and phloem chilling are better suited, because collateral effects are minimized. Regardless of the approach, phloem transport manipulations provide a way to elucidate changes in carbon allocation under both high and low carbon availability, and thus potentially offering insights that cannot be obtained from costly CO2 enrichment experiments.
CO2 Emissions From Respiration
Respiration—the metabolic oxidation of glucose to CO2, H2O, and energy—can rapidly return recently assimilated and stored nonstructural carbon to the atmosphere. Phloem transport manipulation provides a valuable tool to investigate the rate of respiration in relation to locally available carbon, metabolic activity associated with growth of new tissues, and remobilization of stored reserves. Carbone et al. (2013) used radiocarbon dating to show that the substrate for growing season stem respiration was recently assimilated carbon compounds, whereas during the dormant season there was reliance on older stored reserves. Further insights could be provided by combining studies like this with phloem transport manipulation (Muhr et al., 2018). Specific questions could include: (i) does respiration increase proportionally with metabolic demand; (ii) what proportion of emitted CO2 is respired locally vs. transported in xylem sap from distant tissues (Teskey et al., 2008; Bloemen et al., 2014; Tarvainen et al., 2014); and (iii) are younger (more recently assimilated) substrates used preferentially over older substrates?
Effects of Carbon Availability on Wood Growth
Wood formation is the key process through which atmospheric carbon is sequestered in tree biomass (Rathgeber et al., 2016). By creating local gradients of carbon availability, phloem transport manipulation can be used to help understand the extent to which wood formation is limited by carbon, as long as concurrent osmotically-induced changes in turgor and other signaling compounds are accounted for. Specific questions that could be addressed by phloem compression and phloem chilling are: (i) how much is wood growth limited and/or controlled by carbon availability, and does this relationship vary with tree age or size; (ii) is the response of wood growth to carbon availability linear or non-linear; and (iii) how do rates of cell division, cell expansion and cell wall thickening change in response to carbon availability?
Measurements of wood formation and structure in young Pinus sylvestris after girdling are consistent with carbon limitation of wood growth (Winkler and Oberhuber, 2017). However, modeling (Hayat et al., 2017) and theoretical arguments (Körner, 2003, 2006) suggest that with increasing size, tree growth switches from being limited by carbon source activity to being limited by sink activity. In future studies of the controls of tree growth, phloem compression and phloem chilling would have a distinct advantage over girdling, because they are reversible and less invasive. In particular, they appear to restrict carbon transport but may allow the passage of other compounds, that might stimulate or even drive wood growth. For example, phloem chilling of Ricinus communis was shown to maintain a gradient of sugar concentrations across the zone of chilling without clearly impeding the phloem transport of signaling compounds and inorganic solutes (Peuke et al., 2006). When phloem chilling is used, stem temperatures in close proximity to the sample locations for xylogenesis need to be monitored, as they directly affect cambial activity (Oribe and Funada, 2017). Alternatively, compression could be used to avoid collateral effects of temperature on cambial activity in the first place. All three methods can clearly contribute to the investigation of carbon limitations of wood formation in mature forest trees, which remains an important and open question.
Root Respiration, Root Exudates and Transport of Carbon to the Rhizosphere
The irreversible and invasive nature of girdling, when applied to the main bole, which blocks the downstream flow of current assimilates into the soil, has been harnessed to reduce carbon fluxes to soils across large areas and numbers of individuals. Previous studies have evaluated the impacts of girdling on mycorrhizal abundance and community structure (Dannenmann et al., 2009), and have used girdling to partition soil respiration into autotrophic and heterotrophic components (Högberg et al., 2001; Bhupinderpal-Singh et al., 2003; Subke et al., 2004). However, phloem compression may improve partitioning estimates by reversibly restricting nonstructural carbon transport to roots, without inducing fine root mortality (Henriksson et al., 2015), although leakage and the large reserves of nonstructural carbon reserves in roots could still lead to underestimation of the autotrophic component (Aubrey and Teskey, 2018). In fact, phloem chilling has been used to reduce carbon transport to soils and had a similar effect as girdling on a Pinus taeda plantation, but was reversible (Johnsen et al., 2007). Thus, compared to girdling of the main bole, non-lethal methods to manipulate phloem transport belowground have the potential to minimize effects of fine root mortality, enhanced decomposition, and subsequent effects on microbial communities (Kaiser et al., 2010; Pena et al., 2010; Rasche et al., 2011), which arguably lead to biases in studies using girdling (Högberg et al., 2009; Kuzyakov and Gavrichkova, 2010). Some questions that non-lethal and reversible techniques could answer are: (i) how long can root nonstructural carbon reserves buffer reductions in the phloem flow of assimilated carbon; (ii) what is the temporal coupling between carbon assimilation and soil respiration; and (iii) how long does it take for soil respiration to recover after a temporary blockage of carbon fluxes?
Conclusions
We have reviewed the three most common direct methods to manipulate phloem transport, and have assessed and evaluated their effectiveness and potential to answer questions related to carbon dynamics and use in forest trees. In particular, non-lethal methods of phloem compression and phloem chilling have the potential to further contribute to the understanding of carbon dynamics and use in trees. We propose some key experiments which could help develop a more mechanistic understanding of the fate of assimilated carbon, and the timescales on which it returns to the atmosphere. In addition, we argue that accurate interpretation, reproducibility, and assessment of experimental results are reliant on the recording, reporting and publishing of relevant methodological details, and ancillary measurements. Full and accurate reporting is essential if we are to correctly interpret these experiments and unravel the mechanisms underlying growth and metabolic responses to phloem transport manipulation. Combining several methodological approaches, direct phloem transport manipulations can provide simple, yet rigorous tests challenging our current knowledge of phloem function, failure and their environmental dependencies. Such a quantitative understanding of how activities of carbon sources (recent assimilates from photosynthesis, as well as stored nonstructural carbon reserves) and sinks (growth and maintenance respiration, and new tissue growth) in trees are controlled by environmental conditions and internal feedbacks, is required for accurate projections of forest growth and hence the land carbon cycle under future conditions, especially of elevated atmospheric CO2.
Author Contributions
TR and AR planned and defined the scope of the paper. TR drafted the paper and produced the figures. TR, DB and JL provided images for the figures. TR and AE-S assembled the tables. All co-authors discussed ideas, provided feedback, edited the manuscript draft, and approved the manuscript for submission.
Conflict of Interest Statement
The authors declare that the research was conducted in the absence of any commercial or financial relationships that could be construed as a potential conflict of interest.
Acknowledgments
AF, AR, and TR acknowledge support from the Natural Environment Research Council—National Science Foundation International Collaboration program, under grants NE/P011462/1 and DEB- 1741585. AR is also supported by NSF DEB-1237491. DB acknowledges support through the Swiss National Science Foundation (PSBSP3-168701). We thank Nils Henriksson and two reviewers for valuable feedback on the manuscript, which helped improve the accuracy and clarity of the manuscript.
Supplementary Material
The Supplementary Material for this article can be found online at: https://www.frontiersin.org/articles/10.3389/ffgc.2019.00011/full#supplementary-material
References
Ainsworth, E. A., and Bush, D. R. (2011). Carbohydrate export from the leaf: a highly regulated process and target to enhance photosynthesis and productivity. Plant Physiol. 155, 64–69. doi: 10.1104/pp.110.167684
Amthor, J. S. (2000). The McCree–de Wit–Penning de Vries–Thornley respiration paradigms: 30 years later. Ann. Bot. 86, 1–20. doi: 10.1006/anbo.2000.1175
Annighöfer, P., Schall, P., Kawaletz, H., Mölder, I., Terwei, A., Zerbe, S., et al. (2012). Vegetative growth response of black cherry (Prunus serotina) to different mechanical control methods in a biosphere reserve. Can. J. For. Res. 42, 2037–2051. doi: 10.1139/cjfr-2012-0257
Asao, S., and Ryan, M. G. (2015). Carbohydrate regulation of photosynthesis and respiration from branch girdling in four species of wet tropical rain forest trees. Tree Physiol. 35, 608–620. doi: 10.1093/treephys/tpv025
Aubrey, D. P., and Teskey, R. O. (2018). Stored root carbohydrates can maintain root respiration for extended periods. New Phytol. 218, 142–152. doi: 10.1111/nph.14972
Bauhus, J., Puettmann, K., and Messier, C. (2009). Silviculture for old-growth attributes. For. Ecol. Manag. 258, 525–537. doi: 10.1016/j.foreco.2009.01.053
Beer, C., Reichstein, M., Tomelleri, E., Ciais, P., Jung, M., Carvalhais, N., et al. (2010). Terrestrial gross carbon dioxide uptake: global distribution and covariation with climate. Science 329, 834–838. doi: 10.1126/science.1184984
Bhupinderpal-Singh, Nordgren, A., Ottosson Lofvenius, M., Hogberg, M. N., Mellander, P.-E., Högberg, P., et al. (2003). Tree root and soil heterotrophic respiration as revealed by girdling of boreal Scots pine forest: extending observations beyond the first year. Plant Cell Environ. 26, 1287–1296. doi: 10.1046/j.1365-3040.2003.01053.x
Bloemen, J., Agneessens, L., Van Meulebroek, L., Aubrey, D. P., McGuire, M. A., Teskey, R. O., et al. (2014). Stem girdling affects the quantity of CO2 transported in xylem as well as CO2 efflux from soil. New Phytol. 201, 897–907. doi: 10.1111/nph.12568
Carbone, M. S., Czimczik, C. I., Keenan, T. F., Murakami, P. F., Pederson, N., Schaberg, P. G., et al. (2013). Age, allocation and availability of nonstructural carbon in mature red maple trees. New Phytol. 200, 1145–1155. doi: 10.1111/nph.12448
Cernusak, L. A., and Marshall, J. D. (2001). Responses of foliar 13C, gas exchange and leaf morphology to reduced hydraulic conductivity in Pinus monticola branches. Tree Physiol. 21, 1215–1222. doi: 10.1093/treephys/21.16.1215
Chen, J.-J., Zhang, J., and He, X.-Q. (2014). Tissue regeneration after bark girdling: an ideal research tool to investigate plant vascular development and regeneration. Physiol. Plant. 151, 147–155. doi: 10.1111/ppl.12112
Christman, M. A., Sperry, J. S., and Smith, D. D. (2012). Rare pits, large vessels and extreme vulnerability to cavitation in a ring-porous tree species. New Phytol. 193, 713–720. doi: 10.1111/j.1469-8137.2011.03984.x
Cramer, W., Bondeau, A., Woodward, F. I., Prentice, I. C., Betts, R. A., Brovkin, V., et al. (2001). Global response of terrestrial ecosystem structure and function to CO2 and climate change: results from six dynamic global vegetation models. Glob. Change Biol. 7, 357–373. doi: 10.1046/j.1365-2486.2001.00383.x
Cutting, J. G. M., and Lyne, M. C. (1993). Girdling and the reduction in shoot xylem sap concentrations of cytokinins and gibberellins in peach. J. Hortic. Sci. 68, 619–626. doi: 10.1080/00221589.1993.11516393
Dann, I., Jerie, P., and Chalmers, D. (1985). Short-term changes in cambial growth and endogenous IAA concentrations in relation to phloem girdling of peach, Prunus persica (L.) Batsch. Funct. Plant Biol. 12:395. doi: 10.1071/PP9850395
Dannenmann, M., Simon, J., Gasche, R., Holst, J., Naumann, P. S., Kögel-Knabner, I., et al. (2009). Tree girdling provides insight on the role of labile carbon in nitrogen partitioning between soil microorganisms and adult European beech. Soil Biol. Biochem. 41, 1622–1631. doi: 10.1016/j.soilbio.2009.04.024
Davidson, A., Keller, F., and Turgeon, R. (2011). Phloem loading, plant growth form, and climate. Protoplasma 248, 153–163. doi: 10.1007/s00709-010-0240-7
De Schepper, V., Bühler, J., Thorpe, M., Roeb, G., Huber, G., van Dusschoten, D., et al. (2013a). 11C-PET imaging reveals transport dynamics and sectorial plasticity of oak phloem after girdling. Front. Plant Sci. 4:200. doi: 10.3389/fpls.2013.00200
De Schepper, V., De Swaef, T., Bauweraerts, I., and Steppe, K. (2013b). Phloem transport: a review of mechanisms and controls. J. Exp. Bot. 64, 4839–4850. doi: 10.1093/jxb/ert302
De Schepper, V., and Steppe, K. (2011). Tree girdling responses simulated by a water and carbon transport model. Ann. Bot. 108, 1147–1154. doi: 10.1093/aob/mcr068
De Schepper, V., Steppe, K., and Lemeur, R. (2007). Distinction between water and sugar transport by double girdling on an oak tree. Comm. Appl. Biol. Sci. 71:6.
De Schepper, V., Vanhaecke, L., and Steppe, K. (2011). Localized stem chilling alters carbon processes in the adjacent stem and in source leaves. Tree Physiol. 31, 1194–1203. doi: 10.1093/treephys/tpr099
Dietze, M. C., Sala, A., Carbone, M. S., Czimczik, C. I., Mantooth, J. A., Richardson, A. D., et al. (2014). Nonstructural carbon in woody plants. Annu. Rev. Plant Biol. 65, 667–687. doi: 10.1146/annurev-arplant-050213-040054
Domec, J.-C., and Pruyn, M. L. (2008). Bole girdling affects metabolic properties and root, trunk and branch hydraulics of young ponderosa pine trees. Tree Physiol. 28, 1493–1504. doi: 10.1093/treephys/28.10.1493
Epron, D., Dannoura, M., and Hölttä, T. (2019). Introduction to the invited issue on phloem function and dysfunction. Tree Physiol. 39, 167–172. doi: 10.1093/treephys/tpz007
Fatichi, S., Leuzinger, S., and Körner, C. (2014). Moving beyond photosynthesis: from carbon source to sink-driven vegetation modeling. New Phytol. 201, 1086–1095. doi: 10.1111/nph.12614
Fitzgerald, K. (1761). Experiments on checking the too luxuriant growth of fruit-tress, tending to dispose them to produce fruit. Philos. Trans. R. Soc. B Biol. Sci. 52, 71–75. doi: 10.1098/rstl.1761.0013
Friend, A. D., Lucht, W., Rademacher, T. T., Keribin, R., Betts, R., Cadule, P., et al. (2014). Carbon residence time dominates uncertainty in terrestrial vegetation responses to future climate and atmospheric CO 2. Proc. Natl. Acad. Sci. U.S.A. 111, 3280–3285. doi: 10.1073/pnas.1222477110
Friend, A. D., Stevens, A. K., Knox, R. G., and Cannell, M. G. R. (1997). A process-based, terrestrial biosphere model of ecosystem dynamics (Hybrid v3.0). Ecol. Model. 95, 249–287. doi: 10.1016/S0304-3800(96)00034-8
Furze, M. E., Huggett, B. A., Aubrecht, D. M., Stolz, C. D., Carbone, M. S., and Richardson, A. D. (2019). Whole-tree nonstructural carbohydrate storage and seasonal dynamics in five temperate species. New Phytol. 221, 1466–1477. doi: 10.1111/nph.15462
Furze, M. E., Trumbore, S., and Hartmann, H. (2018). Detours on the phloem sugar highway: stem carbon storage and remobilization. Curr. Opin. Plant Biol. 43, 89–95. doi: 10.1016/j.pbi.2018.02.005
Goren, R., Huberman, M., and Goldschmidt, E. E. (2004). Girdling: physiological and horticultural aspects. Hortic. Rev. 30, 1–36. doi: 10.1002/9780470650837.ch1
Gould, N., Minchin, P. E. H., and Thorpe, M. R. (2004). Direct measurements of sieve element hydrostatic pressure reveal strong regulation after pathway blockage. Funct. Plant Biol. 31:987. doi: 10.1071/FP04058
Gref, R., and Ståhl, E. (1994). Lightwood induction in Pinus sylvestris by means of mechanical wounding. Scand. J. For. Res. 9, 382–385. doi: 10.1080/02827589409382855
Hardiman, B., Bohrer, G., Gough, C., and Curtis, P. (2013). Canopy structural changes following widespread mortality of canopy dominant trees. Forests 4, 537–552. doi: 10.3390/f4030537
Hartmann, H., and Trumbore, S. (2016). Understanding the roles of nonstructural carbohydrates in forest trees - from what we can measure to what we want to know. New Phytol. 211, 386–403. doi: 10.1111/nph.13955
Havelange, A., Lejeune, P., and Bernier, G. (2000). Sucrose/cytokinin interaction in Sinapis alba at floral induction: a shoot-to-root-to-shoot physiological loop. Physiol. Plant. 109, 343–350. doi: 10.1034/j.1399-3054.2000.100316.x
Hayat, A., Hacket-Pain, A. J., Pretzsch, H., Rademacher, T. T., and Friend, A. D. (2017). Modeling tree growth taking into account carbon source and sink limitations. Front. Plant Sci. 8:182. doi: 10.3389/fpls.2017.00182
Henriksson, N., Tarvainen, L., Lim, H., Tor-Ngern, P., Palmroth, S., Oren, R., et al. (2015). Stem compression reversibly reduces phloem transport in Pinus sylvestris trees. Tree Physiol. 35, 1075–1085. doi: 10.1093/treephys/tpv078
Hoch, G. (2005). Fruit-bearing branchlets are carbon autonomous in mature broad-leaved temperate forest trees. Plant Cell Environ. 28, 651–659. doi: 10.1111/j.1365-3040.2004.01311.x
Högberg, P., Bhupinderpal-Singh, Löfvenius, M. O., and Nordgren, A. (2009). Partitioning of soil respiration into its autotrophic and heterotrophic components by means of tree-girdling in old boreal spruce forest. For. Ecol. Manag. 257, 1764–1767. doi: 10.1016/j.foreco.2009.01.036
Högberg, P., Högberg, M. N., Göttlicher, S. G., Betson, N. R., Keel, S. G., Metcalfe, D. B., et al. (2007). High temporal resolution tracing of photosynthate carbon from the tree canopy to forest soil microorganisms. New Phytol. 177, 220–228. doi: 10.1111/j.1469-8137.2007.02238.x
Högberg, P., Nordgren, A., Buchmann, N., Taylor, A. F., Ekblad, A., Högberg, M. N., et al. (2001). Large-scale forest girdling shows that current photosynthesis drives soil respiration. Nature 411, 789–792. doi: 10.1038/35081058
Hölttä, T., Lintunen, A., Chan, T., Mäkelä, A., and Nikinmaa, E. (2017). A steady-state stomatal model of balanced leaf gas exchange, hydraulics and maximal source–sink flux. Tree Physiol. 37, 851–868. doi: 10.1093/treephys/tpx011
Hossain, A. B. M. S., Mitzutani, F., and Onguso, J. M. (2004). Effect of partial and complete ringing on carbohydrates, mineral content and distribution pattern 13C-photoassimilates in young peach trees. Asian J. Plant Sci. 3, 498–507. doi: 10.3923/ajps.2004.498.507
Hubbard, R. M., Rhoades, C. C., Elder, K., and Negron, J. (2013). Changes in transpiration and foliage growth in lodgepole pine trees following mountain pine beetle attack and mechanical girdling. For. Ecol. Manag. 289, 312–317. doi: 10.1016/j.foreco.2012.09.028
Iglesias, D. J., Lliso, I., Tadeo, F. R., and Talon, M. (2002). Regulation of photosynthesis through source: sink imbalance in citrus is mediated by carbohydrate content in leaves. Physiol. Plant. 116, 563–572. doi: 10.1034/j.1399-3054.2002.1160416.x
Inatomi, M., Ito, A., Ishijima, K., and Murayama, S. (2010). Greenhouse gas budget of a cool-temperate deciduous broad-leaved forest in Japan estimated using a process-based model. Ecosystems 13, 472–483. doi: 10.1007/s10021-010-9332-7
Ito, A., and Oikawa, T. (2002). A simulation model of the carbon cycle in land ecosystems (Sim-CYCLE): a description based on dry-matter production theory and plot-scale validation. Ecol. Model. 151, 143–176. doi: 10.1016/S0304-3800(01)00473-2
Jensen, K. H., Berg-Sørensen, K., Bruus, H., Holbrook, N. M., Liesche, J., Schulz, A., et al. (2016). Sap flow and sugar transport in plants. Rev. Mod. Phys. 88:035007. doi: 10.1103/RevModPhys.88.035007
Jensen, K. H., Savage, J. A., and Holbrook, N. M. (2013). Optimal concentration for sugar transport in plants. J. R. Soc. Interface 10:20130055. doi: 10.1098/rsif.2013.0055
Jiménez, E., Vega, J. A., and Fernández, C. (2017). Response of Pinus pinaster Ait. trees to controlled localised application of heat to stem and crown. Trees 31, 1203–1213. doi: 10.1007/s00468-017-1538-2
Johnsen, K., Maier, C., Sanchez, F., Anderson, P., Butnor, J., Waring, R., et al. (2007). Physiological girdling of pine trees via phloem chilling: proof of concept. Plant Cell Environ. 30, 128–134. doi: 10.1111/j.1365-3040.2006.01610.x
Jordan, M.-O., and Habib, R. (1996). Mobilizable carbon reserves in young peach trees as evidenced by trunk girdling experiments. J. Exp. Bot. 47, 79–87. doi: 10.1093/jxb/47.1.79
Kaiser, C., Koranda, M., Kitzler, B., Fuchslueger, L., Schnecker, J., Schweiger, P., et al. (2010). Belowground carbon allocation by trees drives seasonal patterns of extracellular enzyme activities by altering microbial community composition in a beech forest soil. New Phytol. 187, 843–858. doi: 10.1111/j.1469-8137.2010.03321.x
Körner, C. (2003). Carbon limitation in trees. J. Ecol. 91, 4–17. doi: 10.1046/j.1365-2745.2003.00742.x
Körner, C. (2006). Plant CO2 responses: an issue of definition, time and resource supply. New Phytol. 172, 393–411. doi: 10.1111/j.1469-8137.2006.01886.x
Körner, C. (2015). Paradigm shift in plant growth control. Curr. Opin. Plant Biol. 25, 107–114. doi: 10.1016/j.pbi.2015.05.003
Krinner, G., Viovy, N., de Noblet-Ducoudré, N., Ogée, J., Polcher, J., Friedlingstein, P., et al. (2005). A dynamic global vegetation model for studies of the coupled atmosphere-biosphere system: DVGM for coupled climate studies. Glob. Biogeochem. Cycles 19:GB1015. doi: 10.1029/2003GB002199
Kuzyakov, Y., and Gavrichkova, O. (2010). Time lag between photosynthesis and carbon dioxide efflux from soil: a review of mechanisms and controls. Glob. Change Biol. 16, 3386–3406. doi: 10.1111/j.1365-2486.2010.02179.x
Lacointe, A., Kajji, A., Daudet, F.-A., Archer, P., Frossard, J.-S., Saint-Joanis, B., et al. (1993). Mobilization of carbon reserves in young walnut trees. Acta Bot. Gallica 140, 435–441. doi: 10.1080/12538078.1993.10515618
Lambers, H., and Ribas-Carbo, M. (2005). “Plant respiration,” in Regulation of Respiration in vivo, eds H. Lambers, S. A. Robinson, and M. Ribas-Carbo (Dordrecht: Springer Netherlands), 1–15.
Larochelle, F., Forget, É., Rainville, A., and Bousquet, J. (1998). Sources of temporal variation in sap sugar content in a mature sugar maple (Acer saccharum) plantation. For. Ecol. Manag. 106, 307–313. doi: 10.1016/S0378-1127(97)00306-X
Levy-Varon, J. H., Schuster, W. S., and Griffin, K. L. (2012). The autotrophic contribution to soil respiration in a northern temperate deciduous forest and its response to stand disturbance. Oecologia 169, 211–220. doi: 10.1007/s00442-011-2182-y
Liesche, J. (2017). Sucrose transporters and plasmodesmal regulation in passive phloem loading: mechanism and regulation of passive phloem loading. J. Integr. Plant Biol. 59, 311–321. doi: 10.1111/jipb.12548
Maier, C. A., Johnsen, K. H., Clinton, B. D., and Ludovici, K. H. (2010). Relationships between stem CO2 efflux, substrate supply, and growth in young loblolly pine trees. New Phytol. 185, 502–513. doi: 10.1111/j.1469-8137.2009.03063.x
Maunoury-Danger, F., Fresneau, C., Eglin, T., Berveiller, D., Francois, C., Lelarge-Trouverie, C., et al. (2010). Impact of carbohydrate supply on stem growth, wood and respired CO2 13C: assessment by experimental girdling. Tree Physiol. 30, 818–830. doi: 10.1093/treephys/tpq039
McDowell, N., Pockman, W. T., Allen, C. D., Breshears, D. D., Cobb, N., Kolb, T., et al. (2008). Mechanisms of plant survival and mortality during drought: why do some plants survive while others succumb to drought? New Phytol. 178, 719–739. doi: 10.1111/j.1469-8137.2008.02436.x
Mencuccini, M., and Hölttä, T. (2010). The significance of phloem transport for the speed with which canopy photosynthesis and belowground respiration are linked. New Phytol. 185, 189–203. doi: 10.1111/j.1469-8137.2009.03050.x
Mitchell, P. J., McAdam, S. A., Pinkard, E. A., and Brodribb, T. J. (2017). Significant contribution from foliage-derived ABA in regulating gas exchange in Pinus radiata. Tree Physiol. 37, 236–245. doi: 10.1093/treephys/tpw09
Moscatello, S., Proietti, S., Augusti, A., Scartazza, A., Walker, R. P., Famiani, F., et al. (2017). Late summer photosynthesis and storage carbohydrates in walnut (Juglans regia L.): feed-back and feed-forward effects. Plant Physiol. Biochem. 118, 618–626. doi: 10.1016/j.plaphy.2017.07.025
Muhr, J., Messier, C., Delagrange, S., Trumbore, S., Xu, X., and Hartmann, H. (2016). How fresh is maple syrup? Sugar maple trees mobilize carbon stored several years previously during early springtime sap-ascent. New Phytol. 209, 1410–1416. doi: 10.1111/nph.13782
Muhr, J., Trumbore, S., Higuchi, N., and Kunert, N. (2018). Living on borrowed time - Amazonian trees use decade-old storage carbon to survive for months after complete stem girdling. New Phytol. 220, 111–120. doi: 10.1111/nph.15302
Münch, E. (1927). Versuche über den Saftkreislauf. Berichte Dtsch. Bot. Ges. 45, 340–356. doi: 10.1111/j.1438-8677.1927.tb01184.x
Myers, D. A., Thomas, R. B., and DeLucia, E. H. (1999). Photosynthetic responses of loblolly pine (Pinus taeda) needles to experimental reduction in sink demand. Tree Physiol. 19, 235–242. doi: 10.1093/treephys/19.4-5.235
Nikinmaa, E., Sievänen, R., and Hölttä, T. (2014). Dynamics of leaf gas exchange, xylem and phloem transport, water potential and carbohydrate concentration in a realistic 3-D model tree crown. Ann. Bot. 114, 653–666. doi: 10.1093/aob/mcu068
Norby, R. J., Ledford, J., Reilly, C. D., Miller, N. E., and O'Neill, E. G. (2004). Fine-root production dominates response of a deciduous forest to atmospheric CO2 enrichment. Proc. Natl. Acad. Sci. U.S.A. 101, 9689–9693. doi: 10.1073/pnas.0403491101
Ntsika, G., and Delrot, S. (1986). Changes in apoplastic and intracellular leaf sugars induced by the blocking of export in Vicia faba. Physiol. Plant. 68, 145–153. doi: 10.1111/j.1399-3054.1986.tb06610.x
Oberhuber, W., Gruber, A., Lethaus, G., Winkler, A., and Wieser, G. (2017). Stem girdling indicates prioritized carbon allocation to the root system at the expense of radial stem growth in Norway spruce under drought conditions. Environ. Exp. Bot. 138, 109–118. doi: 10.1016/j.envexpbot.2017.03.004
O'Brien, M. J., Leuzinger, S., Philipson, C. D., Tay, J., and Hector, A. (2014). Drought survival of tropical tree seedlings enhanced by non-structural carbohydrate levels. Nat. Clim. Change 4, 710–714. doi: 10.1038/nclimate2281
Oner-Sieben, S., and Lohaus, G. (2014). Apoplastic and symplastic phloem loading in Quercus robur and Fraxinus excelsior. J. Exp. Bot. 65, 1905–1916. doi: 10.1093/jxb/eru066
Oribe, Y., and Funada, R. (2017). Locally heated dormant cambium can re-initiate cell production independently of new shoot growth in deciduous conifers (Larix kaempferi). Dendrochronologia 46, 14–23. doi: 10.1016/j.dendro.2017.09.001
Pan, Y., Birdsey, R. A., Phillips, O. L., and Jackson, R. B. (2013). The structure, distribution, and biomass of the world's forests. Annu. Rev. Ecol. Evol. Syst. 44, 593–622. doi: 10.1146/annurev-ecolsys-110512-135914
Pang, Y., Zhang, J., Cao, J., Yin, S.-Y., He, X.-Q., and Cui, K.-M. (2008). Phloem transdifferentiation from immature xylem cells during bark regeneration after girdling in Eucommia ulmoides Oliv. J. Exp. Bot. 59, 1341–1351. doi: 10.1093/jxb/ern041
Paul, M. J., and Foyer, C. H. (2001). Sink regulation of photosynthesis. J. Exp. Bot. 52, 1383–1400. doi: 10.1093/jexbot/52.360.1383
Payyavula, R. S., Tay, K. H., Tsai, C.-J., and Harding, S. A. (2011). The sucrose transporter family in Populus: the importance of a tonoplast PtaSUT4 to biomass and carbon partitioning. Plant J. 65, 757–770. doi: 10.1111/j.1365-313X.2010.04463.x
Pena, R., Offermann, C., Simon, J., Naumann, P. S., Gessler, A., Holst, J., et al. (2010). Girdling affects ectomycorrhizal fungal (EMF) diversity and reveals functional differences in EMF community composition in a beech forest. Appl. Environ. Microbiol. 76, 1831–1841. doi: 10.1128/AEM.01703-09
Percival, G. C., and Smiley, E. T. (2015). The influence of stem girdling on survival and long term health of English oak (Quercus robur L.) and silver birch (Betula pendula Roth.). Urban For. Urban Green. 14, 991–999. doi: 10.1016/j.ufug.2015.09.005
Peuke, A. D., Windt, C., and Van As, H. (2006). Effects of cold-girdling on flows in the transport phloem in Ricinus communis: is mass flow inhibited? Plant Cell Environ. 29, 15–25. doi: 10.1111/j.1365-3040.2005.01396.x
Poirier-Pocovi, M., Lothier, J., and Buck-Sorlin, G. (2018). Modelling temporal variation of parameters used in two photosynthesis models: influence of fruit load and girdling on leaf photosynthesis in fruit-bearing branches of apple. Ann. Bot. 121, 821–832. doi: 10.1093/aob/mcx139
Rasche, F., Knapp, D., Kaiser, C., Koranda, M., Kitzler, B., Zechmeister-Boltenstern, S., et al. (2011). Seasonality and resource availability control bacterial and archaeal communities in soils of a temperate beech forest. ISME J. 5, 389–402. doi: 10.1038/ismej.2010.138
Rathgeber, C. B., Cuny, H. E., and Fonti, P. (2016). Biological basis of tree-ring formation: a crash course. Front. Plant Sci. 7:734. doi: 10.3389/fpls.2016.00734
Regier, N., Streb, S., Zeeman, S. C., and Frey, B. (2010). Seasonal changes in starch and sugar content of poplar (Populus deltoides x nigra cv. Dorskamp) and the impact of stem girdling on carbohydrate allocation to roots. Tree Physiol. 30, 979–987. doi: 10.1093/treephys/tpq047
Richardson, A. D., Carbone, M. S., Huggett, B. A., Furze, M. E., Czimczik, C. I., Walker, J. C., et al. (2015). Distribution and mixing of old and new nonstructural carbon in two temperate trees. New Phytol. 206, 590–597. doi: 10.1111/nph.13273
Richardson, A. D., Carbone, M. S., Keenan, T. F., Czimczik, C. I., Hollinger, D. Y., Murakami, P., et al. (2013). Seasonal dynamics and age of stemwood nonstructural carbohydrates in temperate forest trees. New Phytol. 197, 850–861. doi: 10.1111/nph.12042
Risk, D., Nickerson, N., Phillips, C. L., Kellman, L., and Moroni, M. (2012). Drought alters respired δ13CO2 from autotrophic, but not heterotrophic soil respiration. Soil Biol. Biochem. 50, 26–32. doi: 10.1016/j.soilbio.2012.01.025
Rivas, F., Gravina, A., and Agustí, M. (2007). Girdling effects on fruit set and quantum yield efficiency of PSII in two Citrus cultivars. Tree Physiol. 27, 527–535. doi: 10.1093/treephys/27.4.527
Rosas, T., Galiano, L., Ogaya, R., Peñuelas, J., and Martínez-Vilalta, J. (2013). Dynamics of non-structural carbohydrates in three Mediterranean woody species following long-term experimental drought. Front. Plant Sci. 4:400. doi: 10.3389/fpls.2013.00400
Rossi, S., Deslauriers, A., Griçar, J., Seo, J.-W., Rathgeber, C. B., Anfodillo, T., et al. (2008). Critical temperatures for xylogenesis in conifers of cold climates. Glob. Ecol. Biogeogr. 17, 696–707. doi: 10.1111/j.1466-8238.2008.00417.x
Sauter, J. J., and Ambrosius, T. (1986). Changes in the partitioning of carbohydrates in the wood during bud break in Betula pendula Roth. J. Plant Physiol. 124, 31–43. doi: 10.1016/S0176-1617(86)80175-4
Schaberg, P. G., Murakami, P. F., Butnor, J. R., and Hawley, G. J. (2017). Experimental branch cooling increases foliar sugar and anthocyanin concentrations in sugar maple at the end of the growing season. Can. J. For. Res. 47, 696–701. doi: 10.1139/cjfr-2016-0534
Scott-Denton, L. E., Rosenstiel, T. N., and Monson, R. K. (2006). Differential controls by climate and substrate over the heterotrophic and rhizospheric components of soil respiration. Glob. Change Biol. 12, 205–216. doi: 10.1111/j.1365-2486.2005.01064.x
Sitch, S., Huntingford, C., Gedney, N., Levy, P. E., Lomas, M., Piao, S. L., et al. (2008). Evaluation of the terrestrial carbon cycle, future plant geography and climate-carbon cycle feedbacks using five Dynamic Global Vegetation Models (DGVMs). Glob. Change Biol. 14, 2015–2039. doi: 10.1111/j.1365-2486.2008.01626.x
Sitch, S., Smith, B., Prentice, I. C., Arneth, A., Bondeau, A., Cramer, W., et al. (2003). Evaluation of ecosystem dynamics, plant geography and terrestrial carbon cycling in the LPJ dynamic global vegetation model. Glob. Change Biol. 9, 161–185. doi: 10.1046/j.1365-2486.2003.00569.x
Skogerbo, G. (1992). Effects of root pruning and trunk girdling on xylem cytokinin content of apple (Malus x domestica Borkh.). Nor. J. Agric. Sci. 6, 499–507.
Smith, B., Prentice, I. C., and Sykes, M. T. (2001). Representation of vegetation dynamics in the modelling of terrestrial ecosystems: comparing two contrasting approaches within European climate space: vegetation dynamics in ecosystem models. Glob. Ecol. Biogeogr. 10, 621–637. doi: 10.1046/j.1466-822X.2001.00256.x
Sorce, C., Giovannelli, A., Sebastiani, L., and Anfodillo, T. (2013). Hormonal signals involved in the regulation of cambial activity, xylogenesis and vessel patterning in trees. Plant Cell Rep. 32, 885–898. doi: 10.1007/s00299-013-1431-4
Sovonick-Dunford, S., Lee, D. R., and Zimmermann, M. H. (1981). Direct and indirect measurements of phloem turgor pressure in White Ash. Plant Physiol. 68, 121–126. doi: 10.1104/pp.68.1.121
Steinkamp, J., and Hickler, T. (2015). Is drought-induced forest dieback globally increasing? J. Ecol. 103, 31–43. doi: 10.1111/1365-2745.12335
Steppe, K., Sterck, F., and Deslauriers, A. (2015). Diel growth dynamics in tree stems: linking anatomy and ecophysiology. Trends Plant Sci. 20, 335–343. doi: 10.1016/j.tplants.2015.03.015
Stoy, P. C., Palmroth, S., Oishi, A. C., Siqueira, M. B., Juang, J.-Y., Novick, K. A., et al. (2007). Are ecosystem carbon inputs and outputs coupled at short time scales? A case study from adjacent pine and hardwood forests using impulse–response analysis. Plant Cell Environ. 30, 700–710. doi: 10.1111/j.1365-3040.2007.01655.x
Subke, J.-A., Hahn, V., Battipaglia, G., Linder, S., Buchmann, N., and Cotrufo, M. F. (2004). Feedback interactions between needle litter decomposition and rhizosphere activity. Oecologia 139, 551–559. doi: 10.1007/s00442-004-1540-4
Subke, J.-A., Voke, N. R., Leronni, V., Garnett, M. H., and Ineson, P. (2011). Dynamics and pathways of autotrophic and heterotrophic soil CO2 efflux revealed by forest girdling: dynamics of autotrophic and heterotrophic soil CO2 efflux following girdling. J. Ecol. 99, 186–193. doi: 10.1111/j.1365-2745.2010.01740.x
Suleiman, B. M., Larfeldt, J., Leckner, B., and Gustavsson, M. (1999). Thermal conductivity and diffusivity of wood. Wood Sci. Technol. 33, 465–473. doi: 10.1007/s002260050130
Tarvainen, L., Räntfors, M., and Wallin, G. (2014). Vertical gradients and seasonal variation in stem CO2 efflux within a Norway spruce stand. Tree Physiol. 34, 488–502. doi: 10.1093/treephys/tpu036
Taylor, A., and Cooper, P. (2002). The effect of stem girdling on wood quality. Wood Fiber Sci. 34, 212–229.
Teskey, R. O., Saveyn, A., Steppe, K., and McGuire, M. A. (2008). Origin, fate and significance of CO2 in tree stems. New Phytol. 177, 17–32. doi: 10.1111/j.1469-8137.2007.02286.x
Thorpe, M. R., Furch, A. C., Minchin, P. E., Föller, J., Van Bel, A. J., and Hafke, J. B. (2010). Rapid cooling triggers forisome dispersion just before phloem transport stops. Plant Cell Environ. 33, 259–271. doi: 10.1111/j.1365-3040.2009.02079.x
Tian, H., Xu, X., Lu, C., Liu, M., Ren, W., Chen, G., et al. (2011). Net exchanges of CO2, CH4, and N2O between China's terrestrial ecosystems and the atmosphere and their contributions to global climate warming. J. Geophys. Res. 116:G02011. doi: 10.1029/2010JG001393
Ueda, M., Shibata, E., Fukuda, H., Sano, A., and Waguchi, Y. (2014). Girdling and tree death: lessons from Chamaecyparis pisifera. Can. J. For. Res. 44, 1133–1137. doi: 10.1139/cjfr-2014-0181
Vargas, R., Trumbore, S. E., and Allen, M. F. (2009). Evidence of old carbon used to grow new fine roots in a tropical forest. New Phytol. 182, 710–718. doi: 10.1111/j.1469-8137.2009.02789.x
Vaughn, M. W., Harrington, G. N., and Bush, D. R. (2002). Sucrose-mediated transcriptional regulation of sucrose symporter activity in the phloem. Proc. Natl. Acad. Sci.U.S.A. 99, 10876–10880. doi: 10.1073/pnas.172198599
Vitasse, Y., Lenz, A., and Körner, C. (2014). The interaction between freezing tolerance and phenology in temperate deciduous trees. Front. Plant Sci. 5:541. doi: 10.3389/fpls.2014.00541
White, A. C., Rogers, A., Rees, M., and Osborne, C. P. (2016). How can we make plants grow faster? A source–sink perspective on growth rate. J. Exp. Bot. 67, 31–45. doi: 10.1093/jxb/erv447
Wiley, E., Casper, B. B., and Helliker, B. R. (2017). Recovery following defoliation involves shifts in allocation that favour storage and reproduction over radial growth in black oak. J. Ecol. 105, 412–424. doi: 10.1111/1365-2745.12672
Wiley, E., Huepenbecker, S., Casper, B. B., and Helliker, B. R. (2013). The effects of defoliation on carbon allocation: can carbon limitation reduce growth in favour of storage? Tree Physiol. 33, 1216–1228. doi: 10.1093/treephys/tpt093
Wilson, B. F. (1968). Effect of girdling on cambial activity in white pine. Can. J. Bot. 46, 141–146. doi: 10.1139/b68-024
Winkler, A., and Oberhuber, W. (2017). Cambial response of Norway spruce to modified carbon availability by phloem girdling. Tree Physiol. 37, 1527–1535. doi: 10.1093/treephys/tpx077
Wright, J. P., and Fisher, D. B. (1980). Direct measurement of sieve tube turgor pressure using severed aphid stylets. Plant Physiol. 65, 1133–1135. doi: 10.1104/pp.65.6.1133
Keywords: phloem, girdling, compression, chilling, carbon, sink, source, allocation
Citation: Rademacher TT, Basler D, Eckes-Shephard AH, Fonti P, Friend AD, Le Moine J and Richardson AD (2019) Using Direct Phloem Transport Manipulation to Advance Understanding of Carbon Dynamics in Forest Trees. Front. For. Glob. Change 2:11. doi: 10.3389/ffgc.2019.00011
Received: 21 December 2018; Accepted: 01 April 2019;
Published: 02 May 2019.
Edited by:
Sanna Sevanto, Los Alamos National Laboratory (DOE), United StatesReviewed by:
Michael Clearwater, University of Waikato, New ZealandYann Salmon, University of Helsinki, Finland
Copyright © 2019 Rademacher, Basler, Eckes-Shephard, Fonti, Friend, Le Moine and Richardson. This is an open-access article distributed under the terms of the Creative Commons Attribution License (CC BY). The use, distribution or reproduction in other forums is permitted, provided the original author(s) and the copyright owner(s) are credited and that the original publication in this journal is cited, in accordance with accepted academic practice. No use, distribution or reproduction is permitted which does not comply with these terms.
*Correspondence: Tim T. Rademacher, trademacher@fas.harvard.edu