- 1Agroecology, Department of Crop Sciences, University of Göttingen, Göttingen, Germany
- 2Soil Science of Tropical and Subtropical Ecosystems, Faculty of Forest Sciences and Forest Ecology, University of Göttingen, Göttingen, Germany
- 3Agriculture Faculty, Tadulako University, Palu, Indonesia
- 4Department of Animal Ecology, J. F. Blumenbach Institute of Zoology and Anthropology, University of Göttingen, Göttingen, Germany
- 5A. N. Severtsov Institute of Ecology and Evolution, Russian Academy of Sciences, Moscow, Russia
- 6Biodiversity, Macroecology and Biogeography, University of Göttingen, Göttingen, Germany
- 7Department of Agricultural Economics and Rural Development, University of Göttingen, Göttingen, Germany
- 8Department of Ecology of Tropical Agricultural Systems, Institute of Tropical Agricultural Sciences, University of Hohenheim, Stuttgart, Germany
- 9Department of Plant Protection, IPB University, Bogor, Indonesia
- 10Collaborative Research Centre 990, University of Jambi, Jambi, Indonesia
- 11Tropical Silviculture and Forest Ecology, University of Göttingen, Göttingen, Germany
- 12Forestry Faculty, University of Jambi, Jambi, Indonesia
- 13Chair of Statistics, University of Göttingen, Göttingen, Germany
- 14Forest Botany and Tree Physiology, University of Göttingen, Göttingen, Germany
- 15Centre of Biodiversity and Sustainable Land Use, University of Göttingen, Göttingen, Germany
- 16Agriculture Faculty, Khairun University, Ternate, Indonesia
- 17Botanical Garden of the University of Bern, University of Bern, Bern, Switzerland
- 18Bioclimatology, University of Göttingen, Göttingen, Germany
- 19Department of Soil Sciences and Land Resources Management, IPB University, Bogor, Indonesia
Oil palm plantations are intensively managed agricultural systems that increasingly dominate certain tropical regions. Oil palm monocultures have been criticized because of their reduced biodiversity compared to the forests they historically replaced, and because of their negative impact on soils, water, and climate. We experimentally test whether less intensive management schemes may enhance biodiversity and lessen detrimental effects on the environment while maintaining high yields. We compare reduced vs. conventional fertilization, as well as mechanical vs. chemical weed control (with herbicides) in a long-term, full-factorial, multidisciplinary experiment. We conducted the experiment in an oil palm company estate in Sumatra, Indonesia, and report the results of the first 2 years. We measured soil nutrients and functions, surveyed above- and below-ground organisms, tracked oil palm condition and productivity, and calculated plantation gross margins. Plants, aboveground arthropods, and belowground animals were positively affected by mechanical vs. chemical weed control, but we could not detect effects on birds and bats. There were no detectable negative effects of reduced fertilization or mechanical weeding on oil palm yields, fine roots, or leaf area index. Also, we could not detect detrimental effects of the reduced fertilization and mechanical weeding on soil nutrients and functions (mineral nitrogen, bulk density, and litter decomposition), but water infiltration and base saturation tended to be higher under mechanical weeding, while soil moisture, and microbial biomass varied with treatment. Economic performance, measured as gross margins, was higher under reduced fertilization. There might be a delayed response of oil palm to the different management schemes applied, so results of future years may confirm whether this is a sustainable management strategy. Nevertheless, the initial effects of the experiment are encouraging to consider less intensive management practices as economically and ecologically viable options for oil palm plantations.
Introduction
Currently, oil palm plantations are widespread in all tropical regions. Oil palm is the most productive oil crop, and it is used in a variety of food and consumer products (Byerlee et al., 2016). On the one hand, oil palm generates considerable economic returns and has a transforming impact on rural development by improving living standards (Euler et al., 2017). On the other hand, the loss of biodiversity and ecosystem functions that occurs when oil palm plantations replace land that was historically covered by forest is considerable (Foster et al., 2011; Clough et al., 2016). These concerns have not halted the rampant growth and intensification of oil palm plantations because of their distinctive attractiveness for short-term economic profits compared to other agricultural land use options (Clough et al., 2016).
Indonesia is the largest producer of palm oil worldwide (Food and Agriculture Organization, 2018). At the same time, it is the country with the highest deforestation rate (Margono et al., 2014). Sixty-five percent of the palm oil is produced within large company estates, which make up 59% of the total area under oil palm cultivation, while the rest is managed by smallholders (Direktorat Jenderal Perkebunan, 2015). Given that most of the area is managed by relatively few stakeholders (companies, compared to smallholders), there is potential to effectively improve environmental and ecological issues at large spatial scales while preserving economic interests.
Oil palm plantations are managed in a variety of ways, which determine their productivity and profitability (Corley and Tinker, 2016). Ecosystem functions of oil palm plantations, with reference to forests, and agroecological practices have been assessed recently (Bessou et al., 2017; Dislich et al., 2017). The Roundtable of Sustainable Oil Palm also formulates criteria for certified oil palm producers to reduced negative impacts on the environment and people (RSPO, 2018). However, harvesting, pruning, weeding and fertilizing activities dominate most of the life cycle of commercially used oil palms, and evidence on how they affect ecosystem functions and biodiversity is scant. Two of those management strategies—weeding and fertilization—are arguably the most important as there is scope for optimizing them. In the following, we review the limited evidence available about how weeding and fertilization practices affect agricultural productivity and economic profitability vs. biodiversity and ecosystem functions, and highlight the unknowns.
Generally, fertilization increases the growth rate and productivity of oil palm. Specifically, assimilated nutrients are allocated first to vegetative growth: the leaf area index increases (Corley and Mok, 1972; Breure, 1985). Oil palm root biomass grows faster after empty fruit bunch application, but yields only increase 2 years later (Kheong et al., 2010). Indeed, effects on oil palm yield can take years to be measurable (Sidhu et al., 2009) because of the long maturation of fruit bunches (6 months from pollination to harvest) and the early determination of the flower sex ratio (Corley et al., 1995). Fertilization, however, is associated with deleterious environmental effects, such as greenhouse gas emissions, nutrient leaching losses (Hassler et al., 2017; Kurniawan et al., 2018).
Weeding balances the positive and potential negative effects of the understory plant cover. Plant communities in oil palm plantations consist of many alien and weedy species (Rembold et al., 2017) which may compete for resources with the crop. Effects of weeding practices on the plant cover have only recently been investigated (Luke et al., 2019). Clean-weeding practices (leaving no plant cover) may compact the soil and reduce its porosity (Yahya et al., 2013), whereas moderate weeding can maintain plant cover, which protects the soil against erosion (Corley and Tinker, 2016). The plants also increase soil biological activity, which can enhance soil porosity, and thus water infiltration (Haruna et al., 2018). Enhanced understory complexity may favor large soil invertebrates and promote litter decomposition (Ashton-Butt et al., 2018). The effect of the weeding method, however, such as mechanical vs. chemical control, seems undocumented (Corley and Tinker, 2016). On the one hand, mechanical weeding leaves underground plant parts intact, which might lead to increases in nutrient cycling and soil fauna, and overall favorable soil conditions. On the other hand, herbicide spraying is more effective in eradicating understory vegetation because underground plant parts are also killed. Usually, both methods are used in oil palm company estates, as herbicide spraying is not possible when it rains, and mechanical weeding is necessary to eliminate woody plant encroachment. Similarly, effects of weeding management on biodiversity are not well documented. Teuscher et al. (2016) suggest that with less intensive oil palm inputs (i.e., no fertilizer, pesticide, or herbicide application), more micro-habitats can increase invertebrate abundance and richness. Other taxa benefit from understory vegetation, as bird species richness can increase with vegetation height (Jambari et al., 2012), and terrestrial mammals' occurrence increases with understory vegetation presence (Pardo et al., 2019). However, little is known about how flying mammals (e.g., bats) respond to weeding management, except that oil palm plantations are deemed inhospitable to them (Fukuda et al., 2009).
We need to understand better how different fertilization and weeding management strategies are related to ecosystem functions (biological, geochemical, or physical processes occurring within an ecosystem) and biodiversity (richness, activity, and occurrence of taxonomic groups), in interaction with oil palm productivity. We argue that owing to the large-scale coverage of oil palm plantations and the diminishing coverage of forests, it is important to investigate the potential of oil palm plantations to bolster biodiversity. In the present paper, we assume that there is a trade-off between higher intensity management and ecosystem function provision and biodiversity, but we need to establish the exact relationships with an empirical approach. To date, plausible trade-offs between intensive management activities and impacts on ecosystem functions and biodiversity have not been investigated thoroughly. Quantifying these trade-offs can help to identify optimal management strategies for oil palm production to mitigate detrimental effects on the environment and biodiversity.
Fertilization strategies can be optimized. With increasing fertilization rates, yield curves saturate, but fertilizer costs linearly increase, so that net return (i.e., income generated by the investment in fertilizer after deducting the expenses) is highest before maximal yield levels are reached (Corley and Tinker, 2016). As observed in other crops, above the economically optimum fertilization level, nutrient losses increase and detrimental environmental impacts (e.g., soil acidification, groundwater pollution) become evident. Chronic application of nitrogen fertilizer like urea reduces base cation availability (Lungu and Dynoodt, 2008; Corre et al., 2014; Cusack et al., 2016); it also reduces microbial biomass (Corre et al., 2010; Baldos et al., 2015) and leads to large changes in soil fungal communities (Brinkmann et al., 2019). However, common nutrient fertilization rates in company estates (e.g., on average 230-260 kg N ha−1 yr−1) exceed the nutrient export from fruit harvest (e.g., 136 kg N ha−1 yr−1; Supplementary Data 2.1), suggesting that oil palm is commonly excessively fertilized and thus economically inefficient. We postulate that a reduction in the fertilization rate decreases soil acidification that follows chronic application of nitrogen (Corre et al., 2010) and thus the required lime application. We expect that efficient retention and recycling of nitrogen in the soil under reduced fertilization will foster nitrogen availability to the palms while reducing gaseous emissions and leaching losses. Reduced fertilizer application may also increase profitability in the short term, because soils and palm trunks in mature oil palm monocultures already accumulated high amounts of nutrients (Nazeeb et al., 1993; Hanum et al., 2016). After this transition, profitability could still be high due to fertilizer cost savings even if yields were lower under reduced fertilization.
Mechanical weeding may be a more viable weeding method due to the environmental costs of chemical weed control (e.g., glyphosate, Van Bruggen et al., 2018). Understory vegetation can improve nutrient retention and cycling (Bigelow et al., 2004; Klinge et al., 2004), which could promote nitrogen availability to the palms and reduce the risk of nitrogen leaching losses (Kurniawan et al., 2018). Ground vegetation cover may also alleviate soil acidification (Ewel and Putz, 2004) through uptake of base cations and return through litter input. Nutrient input from decomposition of senesced fronds can replenish soil nutrient stocks: the frond-piled area has lower leaching losses of dissolved base cations, nitrogen and organic carbon than the fertilized area (Kurniawan et al., 2018). In addition, ground vegetation cover can provide organic matter to augment microbial biomass growth which, in turn, drives soil nitrogen cycling and nitrogen availability in oil palm plantations (Allen et al., 2015).
We established a multidisciplinary experiment in which we vary fertilization levels and weeding methods in a full factorial design. We used conventional vs. reduced fertilization rates as well as chemical (i.e., herbicide spraying) vs. mechanical weed control (i.e., using brush cutters). Our experiment is situated in a mature, productive oil palm company estate of the province of Jambi, Sumatra, Indonesia. We present data from the first and second year of our experiment on oil palm condition and productivity, soil nutrients and functions, above- and belowground organisms, and gross margins. We hypothesize that (1) reducing the fertilization rate will not decrease economic profits, but will increase soil nutrients and functions, (2) mechanical weeding will increase biodiversity compared to herbicide spraying and increase soil nutrients and functions (detailed hypotheses in Supplementary Data 2.2). We identify possible management regimes that could be the most economically profitable and the least detrimental for the environment and biodiversity.
Materials and Methods
As per the authors' institutions' guidelines as well as applicable national regulations, no ethics approval was required or obtained for the present study. This study was carried out on a state-owned oil palm estate. We obtained approval to access the site and sample animals therein by establishing a cooperation with PTPN VI, the estate owner. We only collected invertebrates that were not protected by Indonesian law, and vertebrates were passively detected without any disturbance. All research groups involved in the experiment obtained research permits from the Ministry of Research, Technology and Higher Education and sample collection and/or sample export permits from the Ministry of Environment and Forestry of the Republic Indonesia.
Study Design
Site Description
The oil palm management experiment is part of the EFForTS project (Ecological and socioeconomic Functions of tropical lowland rainForest Transformation Systems) in Jambi, Sumatra, Indonesia (Drescher et al., 2016). Our experimental site is situated in the state-owned oil palm plantation PTPN VI, in the regency of Batanghari. To characterize its climatic conditions, we measured air temperature and humidity above the oil palm canopy at a height of 22 m with a thermohygrometer (type 1.1025.55.000, Thies Clima, Göttingen, Germany). Precipitation above the oil palm canopy was measured with two precipitation gauges (Thies Clima, Göttingen, Germany). Global radiation above the oil palm canopy was measured with a sunshine sensor (BF5, Delta T, Cambridge, UK). All meteorological variables were measured every 15 s, averaged and stored in a DL16 Pro data logger (Thies Clima, Göttingen, Germany) as 10 min mean, minimum and maximum values. The climate at the PTPN VI oil palm plantation is tropical humid (Supplementary Figure 1). During the period from March 2014 to May 2019, average air temperature was 26.7 ± 0.4 °C and annual precipitation was 2075.4 ± 94.0 mm, with two precipitation peaks during the rainy season (in November and March). Atmospheric vapor pressure deficit, an important determinant of water vapor and CO2 exchange between the land surface and the atmosphere, was on average 5.7 ± 0.87 hPa and reached its annual maximum at the end of the dry season in September (Supplementary Figure 1). Global radiation was, on average, 194.4 ± 11.1 W m−2 (Supplementary Figure 1) and is reduced during the rainy season due to increased cloud cover. The soil type is an Acrisol, common in the lowland areas of Jambi province, Indonesia (based on the soil map from the International Center for Research in Agroforestry).
Oil palm (African oil palm, Elaeis guineensis, Jacq.) is a vegetable oil-yielding crop native to west and southwest Africa. Oil palm company estates are typically divided into “afdeling” (Dutch word meaning “division”), which are assigned to different afdeling managers who supervise the harvest, fertilizing, and weeding activities. Empty fruit bunches are applied on the plantations, which helps to reduce the need for chemical fertilizer (Singh et al., 2010). First-generation oil palms were planted in the experiment's afdelings in 1999–2002, at a density of 144 to 160 palms/ha, spaced 8 m apart within a row. The management practices in oil palm plantations commonly result in three distinct management zones (Supplementary Figure 2): (1) The palm circle is a 2 m radius disk around each palm base; in our case, it is raked before each application of nutrients (macro- and micro-nutrients as well as lime). (2) The frond piles consist of pruned senesced fronds; in our case, they are linear stacks occurring every two rows, where the next generation of oil palms will be planted. (3) The interrows are the remaining area (sometimes referred to as “harvest paths”); in our case, understory plant cover and harvest paths are in this zone.
Experimental Design
Starting in November 2016, we established a cross-factorial design to vary fertilizer and weed control treatments across 16 plots grouped in four sites (Figure 1). Each plot was 50 × 50 m with similar elevation (plot corners from 63 to 85 m elevation) and flat topography. They contained 36 to 40 palms at a minimal distance of 2 oil palm rows from roads, and were oriented to the north. The fertilizer treatment consists of two levels: the conventional rates at PTPN VI and other oil palm plantation estates of 260 kg nitrogen, 50 kg phosphorus, and 220 kg potassium ha−1 yr−1, and the reduced treatment based on quantified harvest export of fruit bunches, 136 kg nitrogen, 17 kg phosphorus, and 187 kg potassium ha−1 yr−1 (Supplementary Data 2.1); these amounts were split in two applications per year, commonly in November and May. Together with these fertilizers, lime (426 kg dolomite ha−1 yr−1) and micronutrients (142 kg micromag ha−1 yr−1) were applied in the same rates in all treatments; application of lime is necessary in highly weathered Acrisol soils to improve pH, base cations, and phosphorus availability (Schlesinger and Bernhardt, 2013). The weeding treatment consists of two levels: the herbicide treatment used 1,500 cm3 glyphosate ha−1 yr−1 sprayed within the palm circle and divided into four applications per year, and 750 cm3 glyphosate ha−1 yr−1 for the interrows split in two applications per year; the mechanical weeding treatment consists of cutting the ground vegetation using a brush cutter, four times per year inside the palm circles, and twice per year in the interrows, at the same times as the herbicide application.
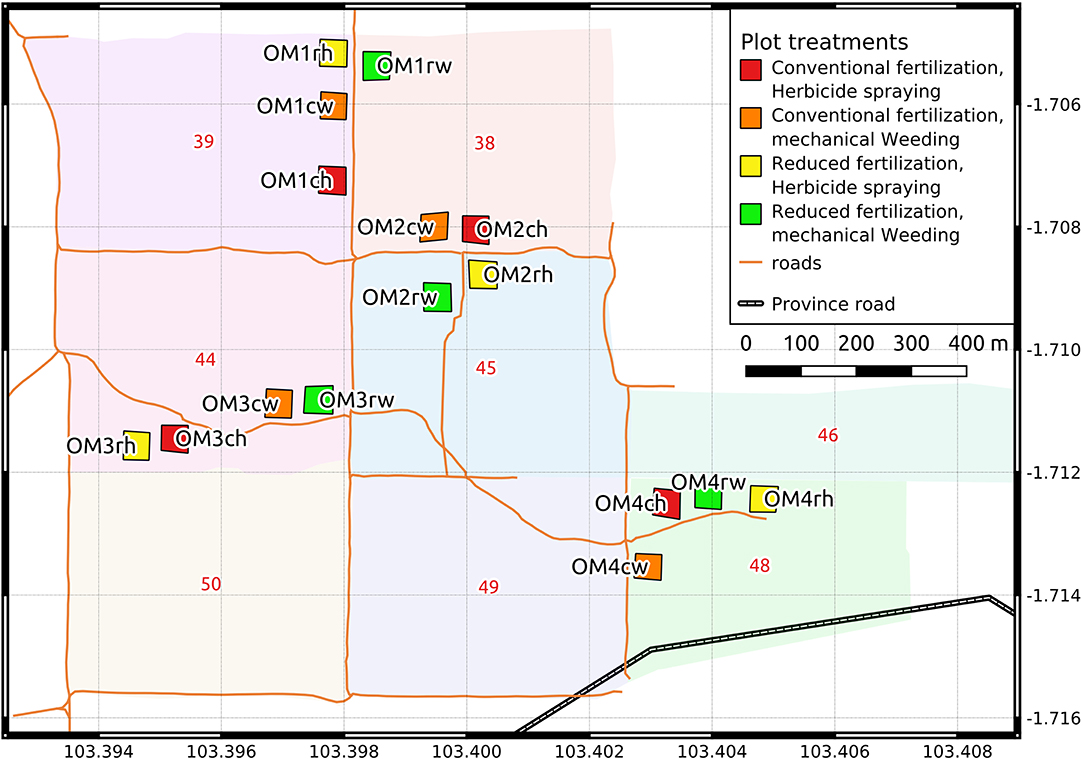
Figure 1. Map of the 16 experimental plots, grouped in four sites. Plots are situated in different afdelings. Afdeling numbers are written in red and distinguished with different fill colors. Plot codes are written in black and indicate the following: “OM” stands for “Oil palm Management experiment”, sites are coded with numbers, treatments are coded with small letters. “c” corresponds to conventional, “r” to reduced fertilization. “h” corresponds to herbicide spraying, “w” to mechanical weeding.
The cross-factorial design resulted in four different, randomly assigned treatment combinations that we abbreviated for reference in figures:
• conventional fertilization, herbicide spraying (“ch”)—so-called high-intensity management;
• conventional fertilization, mechanical weeding (“cw”);
• reduced fertilization, herbicide spraying (“rh”);
• reduced fertilization, mechanical weeding (“rw”)—so-called low-intensity management.
Data were collected at spatial scales that were adapted to each research group (Supplementary Figure 3), and the plots are structured in different sections along a coordinate grid (Supplementary Figure 4). Spatially large scale variables (e.g., bird activity) were collected over the entire plot. Variables that are potentially influenced by the area outside the plot (e.g., yield) were restricted to the central area of the plots, delimited by the inner 30 × 30 m area, which contained 10 to 15 palms per plot. Soil variables (e.g., mineral N) were measured at each management zone per plot. Five marked and labeled 5 × 5 m subplots were used for measurements of spatially fine scale variables (e.g., plants, litter decomposition) in each plot. Palms were individually labeled to be able to link palm-specific variables (e.g., yield, height).
Oil Palms Condition and Productivity
Yield
We measured harvested fresh fruit bunch number and mass of each palm within the central area during 2017 and 2018. We followed the PTPN VI harvesting schedule of one harvest every 10 days and used the company's standard harvesting practice, where fronds are pruned to access the fruit bunches that grow in their axils. We measured the total weight of all bunches (± 0.1 kg) and noted the number of bunches for each palm. We used the mean yield increment per palm of each plot between 2017 and 2018 for the analysis.
Stem Growth
We used a vertex (IV-GS, Haglöf, Långsele, Sweden) to measure the height between the ground and the meristem of all central palms (contained within the central 30 × 30 m square). We defined the meristem as the point to which all the younger fronds (those that are horizontal or pointing higher up) converge. The eye height was entered into the device and it was pointed at the meristem while standing at a spot that was at the same height as the base of the oil palm. We measured stem height before the experiment started (May 2017) and thereafter (June 2019). We computed the stem growth as the difference between the heights measured in 2017 and 2019 and used the mean growth per palm of each plot for the analysis.
Leaf Area Index
In April 2018, leaf area index (LAI) was measured in each subplot using the LAI-2200 Plant Canopy Analyzer (LI-COR, Biosciences Inc., Lincoln, NE, USA, 2014). The instrument calculates LAI from readings made above and below canopy, which are used to determine canopy light interception at five angles. The above canopy readings were taken in a nearby open area and all measurements were taken at 1.3 m height, avoiding partly cloudy skies, and holding the sensor parallel to the ground with the help of the bubble level. Due to the small size of the subplots, a 90° view cap was used to make readings in each corner facing the plot; then a single value was obtained by averaging. We computed the mean LAI over all subplots of each plot for the analysis.
Oil Palm Roots
We extracted soil cores during November-December 2017 from the central 30 × 30 m area, in the interrows, approximately 2.5 m from the next oil palm trunk. Litter was removed, and 5 cm diameter, 10 cm deep soil cores were extracted. In each plot, three interrows were sampled; 5 soil cores were collected per interrow, spaced 5 meters from each other; samples from one interrow were pooled. All 48 samples were cooled and transported to the laboratory, where they were sieved through two layers of 5 mm mesh to extract roots, which were washed, quickly dried on tissue paper and weighed after sorting oil palm roots and other roots based on the visual appearance. We used the mean biomass of fresh fine roots per square meter of each plot for the analysis.
Soil Nutrients and Functions
Soil Parameters
Soil mineral nitrogen in the top 5 cm depth was measured monthly for 1 year from March 2017 to February 2018. In each plot, we selected two palms in the central area, and we measured at the three management zones: palm circle, inter-row and frond-piled area. Mineral nitrogen was extracted on-site right after soil sampling using previously prepared extraction bottles containing 150 mL of 0.5 M K2SO4 solution. The extraction bottles were transported to the laboratory in a cooling box, shaken for 1 h and filtered. Extracts were frozen immediately and kept frozen during the transport to Germany for analysis. The remaining part of the soil sample was used to determine gravimetric moisture content by oven-drying it for 1 day at 105°C. Ammonium and nitrate concentrations in the extracts were determined by continuous flow injection colorimetry (SEAL Analytical AA3, SEAL Analytical GmbH, Norderstedt, Germany). Mineral nitrogen is the sum of ammonium and nitrate, and we used its median per management zone of each plot for the analysis.
In February 2018, bulk density, microbial biomass nitrogen and base saturation were determined at each management zone and plot in the top 5 cm of soil. Bulk density was determined using the core method (Blake and Hartge, 1986). Microbial biomass nitrogen was measured with the fumigation-extraction method (Brookes et al., 1985) the soil was sampled at the same sampling location as the mineral nitrogen measurements and a subsample (about 25 g) was fumigated with CHCl3 for 6 days. The fumigated and unfumigated soils were extracted with 0.5 M K2SO4 as described above, and total nitrogen concentration in the extracts were measured by ultraviolet-persulfate digestion followed by hydrazine sulfate reduction using a continuous flow injection colorimetry mentioned above. We calculated microbial biomass nitrogen as the difference in total nitrogen between the fumigated and unfumigated samples, divided by kN = 0.68 for a 6-day fumigation (Brookes et al., 1985). To measure base saturation, the soil samples from the two palms, each with the three management zones, were pooled to have one sample per management zone in each plot. Base saturation was calculated as the percentage of exchangeable base cations of the effective cation exchange capacity, which was determined by percolating the soils with unbuffered 1 M NH4Cl and measuring the cations in percolates with an inductively coupled plasma-atomic emission spectrometer (ICP-AES; iCAP 6300 Duo VIEW ICP Spectrometer, Thermo Fischer Scientific GmbH, Dreieich, Germany). We computed mean bulk density, microbial biomass nitrogen, and base saturation per management zone of each plot for the analysis.
Water Infiltration
From December 2017 until February 2018, we used custom-built, constant head double ring infiltrometers with inner and outer ring dimensions of 15 and 30 cm respectively, to measure the water infiltration capacity. Infiltrometers were installed in the middle of the interrows with 2 replications per plot (total of 32 point measurements), using 30 L of water (Gregory et al., 2005). The mean infiltration capacity—reflecting the constant infiltration rate after soil is saturated—was computed for each plot and used for the analysis.
Leaf Litter Decomposition
Litterbags (20 × 20 cm with 4 mm mesh size), containing 10 g of dry oil palm leaf litter were incubated (exposed to field conditions) in all plots at the end of December 2016. Leaflets without petioles were taken from oil palm fronds and cut to a length of ca. 15 cm. One litterbag was left for 8 months in each management zone of each of 3 subplots (a,b,c) in each plot, resulting in 144 litterbags in total (3 management zones × 3 subplots × 16 plots). Decomposition was defined as the difference between the initial litter dry mass and litter dry mass remaining after 8 months of incubation. We used the mean mass loss for each plot in the analysis.
Above- and Belowground Organisms
Soil Animals
During October-November 2017, in each plot, five soil samples of 16 × 16 cm were taken randomly with a spade down to a depth of 5 cm, mostly in the interrows. Animals were extracted using a gradient heat extractor (Kempson et al., 1963) and collected in dimethyleneglycol:water solution (1:1) and thereafter transferred into 70 % ethanol (Klarner et al., 2017). All animals, except nematodes, were counted and sorted to taxonomic groups (Supplementary Table 1). We calculated community metabolism in a sample as a proxy of animal decomposer activity by using mean group- and ecosystem-specific estimates derived from (Potapov et al., 2019). The estimates are based on a survey of ca. 30,000 individuals of soil animals across eight different oil palm plantations in the same region; all individuals were measured and individual body masses were recalculated to metabolic rates using group-specific regressions from Ehnes et al. (2011). Metabolic rates for Sternorrhyncha and Gastropoda were not available due to low numbers of sampled individuals. Community metabolism was calculated by summing up metabolic rates of all individuals (Supplementary Table 1); we used the mean per plot for the analysis. We also computed group richness as the number of taxonomic groups (in most of cases orders) present in each plot for the analysis.
Understorey Plants
In September 2016 and 2017, we recorded vascular plant species richness per subplot in all five subplots of each plot, excluding oil palm, and estimated the percentage cover of the vegetation for the entire subplot and for the areas occupied by the palm circle, frond piles, and interrow areas. We computed the differences in species richness and plant cover between 2016 and 2017 to account for differing plot-specific baseline levels at the start of the experiment. We used the mean percent change in cover per management zone for each plot in the analysis.
Aboveground Arthropods
In November 2016 and September 2017, we sampled arthropods with 18 fluorescent yellow pan traps placed in six clusters of three traps, arranged in a 2 × 3 grid in the central area of the plot in the interrows. We attached traps to a platform raised to the height of the surrounding vegetation. We placed the traps in the field for 48 h, after which we collected and preserved arthropods in 70% ethanol. Due to time constraints, we identified and counted only Coleoptera, Hemiptera and Hymenoptera to the family level (CSIRO, 1991; Goulet and Huber, 1993; Johnson and Triplehorn, 2004). We analyzed total insect arthropod abundance and family richness (of Coleoptera, Hemiptera, and Hymenoptera). For both measures, we computed the difference between 2016 and 2017 to account for differing baseline levels between plots at the start of the experiment. We used the mean change in number of individuals for each plot in the analysis.
Birds and Insectivorous Bats
We sampled birds and bats using sound recorders in September 2017. We used SM2Bat+ recorders (Wildlife acoustics, Massachusetts, USA) with two microphones (one SMX-II and one SMX-US or Bio-SM2 microphone) strapped to wooden poles in the middle of the plot at a height of 1.5 m. For birds (during the day), we recorded stereo sound at 22.05 kHz; for bats (during the night), we recorded mono sound at 384 kHz. We sampled half of the plots (OM1 and OM2 sites) simultaneously first, and the other half (OM3 and OM4 sites) afterward. On 2 consecutive days, we extracted, for birds, 15-min recordings starting 15 min before sunset and at sunrise, and for bats, 20-min recordings starting 20 min after sunset. On consecutive days, we extracted, for birds, 15-minute recordings starting at sunrise and 15-minute recordings starting 15 minutes before sunset, and for bats, 20-minute recordings starting 20 minutes after sunset. Recordings were uploaded to an online platform (http://soundefforts.uni-goettingen.de/biosounds/) for analysis. The bird vocalizations were assigned to species (del Hoyo et al., 2014, 2016), using Xeno-Canto and the Macaulay library (Macaulay Library; Xeno-canto Foundation, 2012) to verify species identifications. Bird detection distances were estimated using a reference sound transmission sequence (Darras et al., 2018) to exclude birds detected outside the plots (>35 m) from the data analysis. We computed the total vocalization activity of all birds, as well as the species richness. Similarly, all night recordings were scanned for bat calls by visualizing 10-s long, 870 pixel wide spectrograms, and tagged to extract the duration of their passes. We interpreted the total duration of bat passes as the insectivorous bat activity for each plot in the analysis. We distinguished bat morphospecies based on the characteristics of the calls (call frequency, duration, shape) to compute insectivorous bat species richness of each plot for the analysis.
Economics
To evaluate and compare the economic performance of the management techniques, we conducted a gross margin analysis, also called gross profit analysis. Gross margin is often used to compare the economic performance of farms and management techniques (Clough et al., 2016). We calculated gross margin per ha per year as follows:
Gross Margin = Total Revenue–Variable Cost
where total revenue is total yield in kg per ha multiplied by the associated market prices in each year. Variable cost includes the cost of variable inputs, namely fertilizers, pesticides, spray labor, and weeding labor. We focus only on these variable inputs because they are the main farm inputs over which plantation companies and farmers have control. Total variable cost varies among farms and management techniques based on the application intensity of the associated variable inputs. To calculate the gross margin, we used the recorded yields (weight of fruit bunches in kg, as explained earlier), obtained weekly market prices for Jambi (“Jambi Estate Crops Agency—Dinas Perkebunan Jambi”), recorded the amount and prices of fertilizers, micronutrients, and herbicides applied, and the labor wages incurred in PTPN VI for harvesting, pruning, fertilizer application, mechanical weeding, and herbicide spraying.
In general, gross margin represents the portion of revenue plantation companies and farmers retain as gross profit after covering the variable costs. The higher the gross profit proportion is, the higher the economic performance of a given farm or management technique. We report gross margin expressed both in monetary values (the difference between total revenue and variable cost, the gross profit) and in percentages (gross profit as a proportion of the total revenue). The monetary value is useful to evaluate economic performance of a given farm or a management technique over time, assuming other factors stay constant. The percentage value is suitable to evaluate and compare economic performance of different farms and management techniques, that is, which ones retain higher profit for every dollar generated as a revenue. Here, we focus on and explain the gross margin percentages as we are interested in evaluating the economic performance of the different management schemes that we applied in our experimental plots. We used the mean percent gross margin and mean monetary gross margin for each plot in the analysis.
Data Analysis
We used two different approaches to analyze the data collected within the oil palm management experiment: a simple categorical analysis that directly shows the impact of the treatments on all our measured response variables, and an explorative structural equation model to reveal the mechanisms underlying the responses we observed, taking into account the main hypothesized links between our measured variables. We established hypothesized links before conducting the data analysis. We use R 3.5.1 (R Core Team, 2019) and R package ggplot2 (Wickham, 2009) for the entire data analysis and generation of figures (except Figure 1).
Categorical Analysis
In a first approach, we conducted a categorical analysis of the experimental treatment effects on the measured variables. We used linear mixed-effects models for most response variables, with sites as random intercepts in package lme4 (version 1.1-20, Bolker et al., 2009). We did not use afdelings as random intercepts because the fertilization and weeding management has been identical in all of them since 2014, except for 2015, when nitrogen fertilization was adapted, presumably following a routine leaf analysis. We switched to simple linear models (without random effects) when we obtained singular fits, which typically is an indication that the random effects are estimated to be irrelevant such that mixed models and linear models coincide. Taxonomic richness variables were count variables, and activity of organisms variables were also considered as such since they were derived from them (they are essentially weighted counts) and followed Poisson distributions. Belowground animals group richness data, however, did not follow a poison distribution as they were severely under-dispersed; a normal distribution was used instead with a linear mixed effects model. Both were initially modeled using generalized linear mixed effects models with a Poisson family. We switched to simple generalized models when we obtained singular fits, and we switched to negative binomial models when Poisson models were overdispersed. Richness and abundance differences were modeled using linear models, because they could be negative numbers. Response variables in the form of proportions (bounded between 0 and 1) were modeled using beta regressions with the package betareg (version 3.1-1 Cribari-Neto and Zeileis, 2010). We assessed the significant differences among treatments using Tukey multiple comparison of means with the multcomp package (version 1.4–8, Hothorn et al., 2008), considering uncorrected P-values smaller than 0.05 as significant.
Structural Equation Modeling
In a second approach, we investigated the hypothesized links (Supplementary Data 2.2) between our measured variables to uncover intermediate effects and understand through which pathways the experimental treatments were acting. We used a Bayesian structural equation model using mixed-effects models with sites as a random intercepts for a more explorative and mechanistic approach. We log-transformed count data and logit-transformed proportion data when used as a response variable, to use only linear models so that we obtained comparable effect sizes. We used the actual arthropod abundance instead of its change to link it better to bird and bat measures, which were not expressed as changes. We constructed the Bayesian model using the brms package in R (version 2.7.0, Bürkner, 2017). Compared to frequentist approaches, Bayesian models allow the computation of effect probabilities. We considered our hypothesized links as likely when their probability of being either bigger or smaller than zero was at least 0.75. In other words, the structural equation model paths were deemed likely when they were at least three times more probable than their opposite paths (effects of the opposite sign).
Results
Categorical Analysis
All the results from the categorical analysis are reported in Supplementary Table 2 and Figure 2. We give details about individual variables in the following.
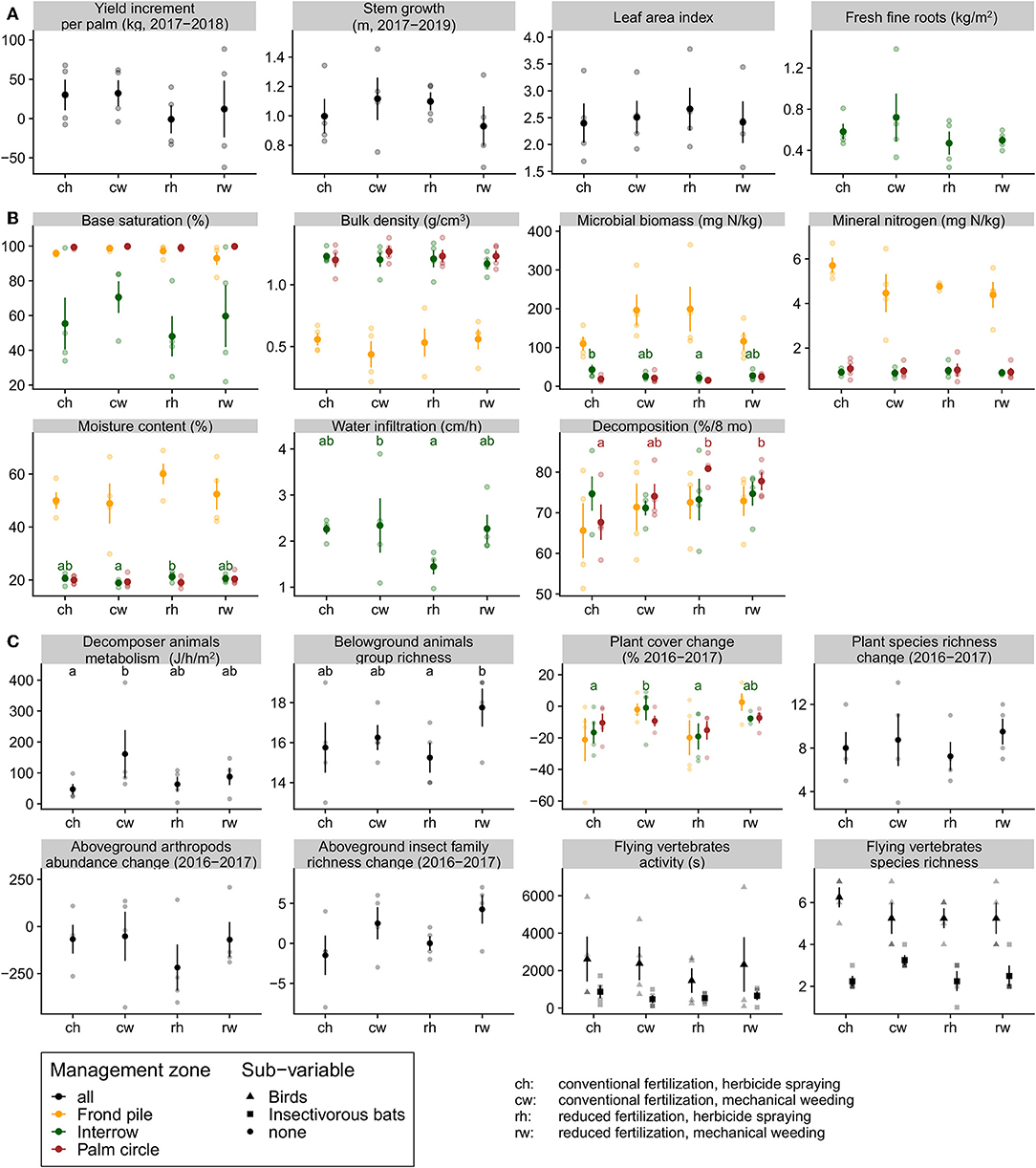
Figure 2. Responses of (A) oil palms condition and productivity, (B) soil nutrients and functions, (C) above- and belowground organisms to the fertilizing and weed control treatments. Data points are indicated with semi-transparent dots. Mean values are indicated with bigger, opaque dots. Error bars show standard errors of the mean, and statistically significant differences between means are shown with distinct letters, color-coded according to the management zone.
Oil Palms Condition and Productivity
We did not detect a significant effect of treatment on the oil palm yield increment between 2017 and 2018 (Figure 2A). Even though the difference in average yield increment between conventional and reduced fertilization was 25.62 kg (corresponding to 11% of the yield under conventional fertilization in 2018), we could not reject the null hypothesis that yield increments are unaffected by our experimental treatments. Similarly, we did not detect a significant treatment effect on leaf area index, stem growth, or fresh fine root biomass.
Soil Nutrients and Functions
Some soil parameters responded significantly to the management treatments, for the others we did not detect an effect (Figure 2B). Oil palm leaf litter decomposition inside palm circles increased by 20% under reduced fertilization with mechanical weeding compared to its lowest level under conventional fertilization with herbicide spraying. However, we refrain from interpreting these changes that we observed in incubated litterbags, as palm circles are normally devoid of oil palm leaf litter (fronds are accumulated in the frond piles). Water infiltration in the interrows decreased by 37% under reduced fertilization with herbicide spraying. On average, soil moisture in the interrows increased by 12% under reduced fertilization with herbicide spraying compared with the other treatments. Microbial biomass nitrogen decreased by 50% in the interrows under reduced fertilization with herbicide spraying compared to the conventional fertilization treatment. On average, base saturation increased by 26% under mechanical weeding in the frond-piled area, but we detected no significant difference.
Above- and Belowground Organisms
Measures of fauna and flora varied between experimental treatments (Figure 2C). Plant cover in interrows decreased 5 times more strongly under herbicide spraying. On average, the community metabolism of decomposer soil fauna increased by 125% under mechanical weeding, but this result was strongly driven by an outlier. Belowground animals group richness also increased by 16% under mechanical weeding compared to herbicide spraying but only under reduced fertilization. We did not detect changes in other richness, abundance, or activity measures in response to our four experimental treatments. However, when comparing aboveground biodiversity measures between weed control treatments—according to our hypotheses (Supplementary Data 2.2), we observe increases under mechanical weeding (except for bird species richness and bat activity) which are statistically significant in the case of aboveground insect richness.
Economic Analysis
In general, economic performance was higher under low-intensity management than conventional management in both years (Figure 3). In particular, gross margin was highest at 79% under reduced fertilization with herbicide spraying, and lowest at 72% under conventional fertilization with mechanical weeding in 2017. Similarly, in 2018, the average gross margins were maximal at 76%, under reduced fertilization with mechanical weeding and minimal at 69% under conventional fertilization with herbicide spraying. In 2018, gross monetary margin was higher under conventional fertilization and mechanical weeding than under the two herbicide spraying treatments.
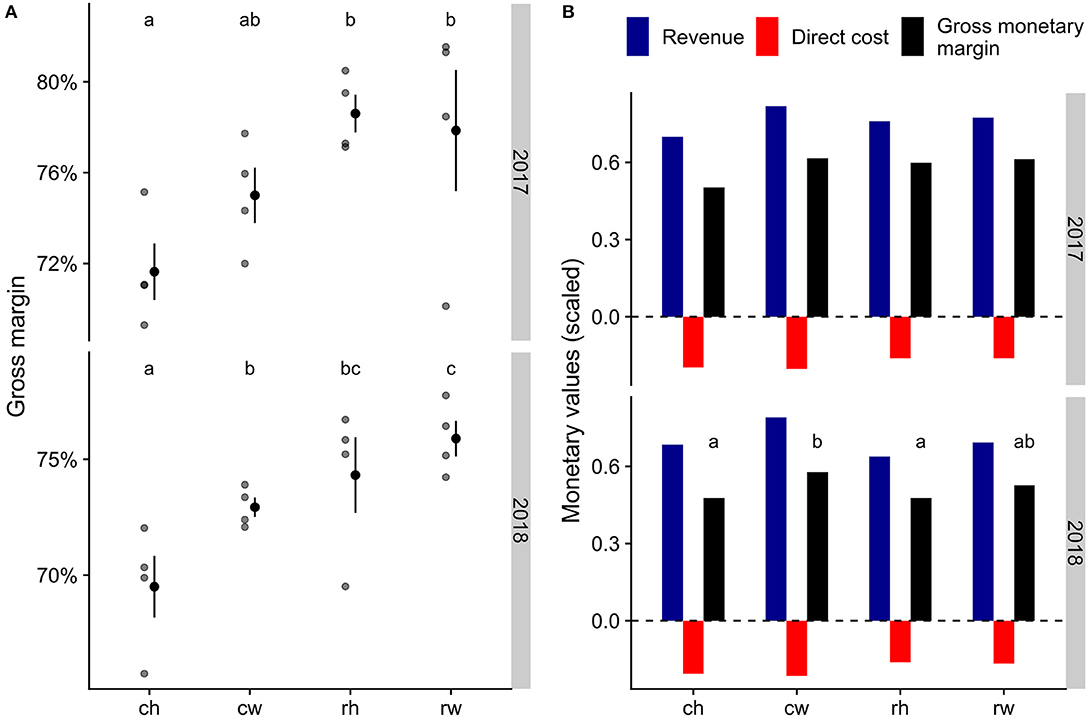
Figure 3. Mean gross margins in percentages (A) and money values (B) are portrayed with the associated cost of inputs and value of outputs for each experimental treatment. Data points are indicated with semi-transparent dots. Mean values are indicated with bigger, opaque dots. Error bars show standard errors of the mean. Revenue and direct costs are shown scaled to the maximum of their values; the difference between these two is the scaled gross margin in money values. Statistically significant differences between means are shown with distinct letters. ch, conventional fertilization, herbicide spraying; cw, conventional fertilization, mechanical weeding; rh, reduced fertilization, herbicide spraying; rw, reduced fertilization, mechanical weeding.
Structural Equation Model
The explorative null structural equation model revealed effects between the measured variables that could not be assessed using the categorical analysis of treatment effects (Figure 4). Some treatment effects became apparent because of the lower threshold we use for considering hypothesized links as likely in the structural equation model. For some variables, there was not enough variation to detect links, partly due to the lack of effects of the experimental treatment. When we did not detect likely links, we could not conclude that there is no link, only that we did not have enough statistical power to detect one. The analysis of trace plots revealed that the estimation of all parameters had succeeded, albeit with low effect sizes of random intercepts.
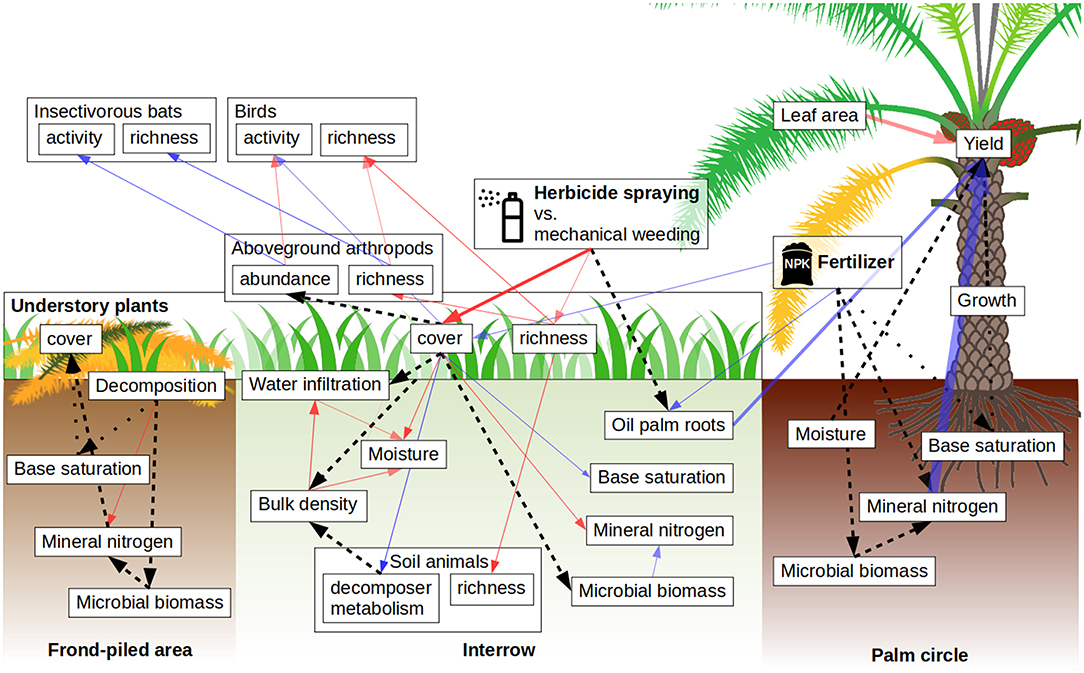
Figure 4. Bayesian structural equation modeling results. All hypothesized paths are indicated with arrows. Full arrows correspond to paths that are three times more likely than their opposite paths: Red arrows indicate negative effects, blue arrows indicate positive effects. Black dashed arrows indicate tested, inconclusive links. Black dotted arrows are hypothesized links that could not be tested with our model due to insufficient variability (base cations were saturated in palm circles and the frond-piled area). Arrow opacity scales with the probability, and arrow width scales with the logarithm of the effect size.
Mineral nitrogen positively affected oil palm yields strongly. We could not detect an effect of fertilizer on mineral nitrogen, microbial biomass, and base saturation. There was not enough variation in base saturation of the palm circles to detect any links. Oil palm roots responded positively to fertilization and were positively associated with oil palm yield increment. Leaf area index was negatively associated with oil palm yield increment, and we could not detect an effect of stem growth on yield increment.
Decomposition in the frond-piled area weakly and negatively affected mineral nitrogen. We could not detect effects of decomposition on microbial biomass and in turn on mineral nitrogen. There was not enough variation in base saturation of the frond-piled areas to detect any links. In the interrows, we could not detect effects of plant cover on microbial biomass but microbial biomass weakly affected mineral nitrogen positively, which was negatively affected by plant cover, while base saturation was affected positively. Increasing bulk density reduced water infiltration and soil moisture. However, we could not detect an effect of decomposers or plant cover on bulk density. Plant cover was negatively associated with soil moisture, and soil moisture was inhibited by water infiltration.
We found a strong negative effect of herbicide application and a weak positive effect of fertilizer on plant cover, which had no detectable effect on aboveground arthropods abundance and a weak positive effect on bird activity. Herbicides also weakly decreased plant species richness, which weakly negatively impacted arthropod richness. Aboveground arthropod abundances were weakly negatively correlated with bird activity. Insectivorous bat activity was weakly enhanced by arthropod abundance. We detected weak negative effects of arthropod richness on birds, and weak positive effects for insectivorous bats. Plant cover positively affected decomposer metabolism but plant richness negatively affected soil animals richness.
Discussion
Overall, reduced fertilization did not clearly reduce oil palm productivity, and there was a clear positive effect on gross margins. Soil nutrients and functions did not detectably decrease under low-intensity management. Biodiversity generally benefited from the mechanical weeding treatment.
Reducing Fertilizer
One year after the start of the experiment, we did not detect a statistically significant reduction in yield under reduced fertilization. Fertilizer can increase stem growth in palms that are nutrient-limited (Sidhu et al., 2009), but we observed no change in stem growth between treatments, even though base saturation increased in the interrows under mechanical weeding. The comparable mineral nitrogen levels between conventional and reduced fertilization suggest that mineral nitrogen was possibly recycled more efficiently under reduced fertilization, but this was not due to nitrogen-fixing plants, which typically covered only 0–2% of a subplot. It is possible that the reduced fertilization led to a higher nitrogen and carbon mineralization (Baldos et al., 2015; Cusack et al., 2016), whereas the high fertilization may have stimulated nitrification (Chu et al., 2008; Corre et al., 2010; Zhang et al., 2013), and thus increased nitrate and base cation leaching (Kurniawan et al., 2018), as well as gaseous nitrogen oxide emissions (Davidson et al., 2000; Hassler et al., 2017). Highly-weathered Acrisol soils, as in the present study, are highly susceptible to nitrogen-induced acidification because of their inherently low acid-buffering capacity (Comte et al., 2013; Allen et al., 2016; Kurniawan et al., 2018). However, as lime was concurrently applied with the fertilizers, we did not detect significant changes in base saturation and soil pH within the palm circles among treatments (Supplementary Figure 5). In the interrows, however, where lime was not applied, we found a positive effect of plant cover on base saturation, suggesting that base cations were retained effectively under high plant cover.
As yields remained comparable among treatments, reduced fertilizer expenses led to higher or similar profitability. Soil and leaf nutrient levels can be measured to objectively decide whether particular nutrients should be cut for reducing costs Mohd Tayeb and Ahmad Tarmizi. However, effects of reduced fertilization should be taken into perspective: as of 2019, palms in our studied plantation are 17–21 years old, and hence the oil palms under reduced fertilization may have benefited from the legacy of long-term conventional fertilization regime. Hanum et al. (2016) reported that total nitrogen stocks of closed-canopy oil palm plantations are on a high level in intensively fertilized company estates, and Nazeeb et al. (1993) report that mature oil palm trunks contain considerable nutrient reserves. There can be an additional delayed response to reduced fertilization as fruits need 6 months to ripen and flower sex ratios are determined 2 to 3 years before harvest (Corley and Tinker, 2016). Generally, it is advised not to reduce fertilizer to cut costs during short-term oil price lows (Goh and Teo, 2011; Corley and Tinker, 2016).
Avoiding Herbicides
Overall, mechanical weeding bolstered animals in different taxa: richness, activity, and abundance tended to be positively affected for all response variables except bird species richness and insectivorous bat activity. When analyzing only the differences between the weed control treatments for the aboveground organisms (in line with our hypotheses), we found that mechanical weeding promotes insect family richness (Supplementary Figure 6). Arthropods supported insectivorous bats, and plants bolstered birds and decomposer animals. Arthropods, however, had a weak negative association with bird activity, which could be explained by top-down control by birds on arthropods. Surprisingly, we found negative effects of plant species richness on bird and arthropod richness. Future in depth-analyses of the composition of the animal and plant communities could detect which species drive these responses.
Mechanical weeding had mild positive effects on soil functions. We postulated that plant cover has wide-ranging positive effects on soil functions, but we could not confirm or refute some of our hypotheses. For instance, we could not detect the effects of plant cover on water infiltration, microbial biomass, or bulk density. However, the higher plant cover under mechanical weeding presumably led to higher transpiration: this could account for the slight observed reduction in soil moisture (6%) in the first 5 cm, while deeper layers could have had higher soil moisture. Changes in water infiltration and soil moisture might become crucial in the face of extreme weather events such as the El Niño of 2015–2016 (Thirumalai et al., 2017), which are projected to affect agricultural production considerably with climate change (Rosenzweig et al., 2001), but oil palms in the lowland areas of Jambi seem less affected by water supply, as suggested by their shallow root distribution (i.e., 90% of fine roots measured within 1 m depth are located in the top 0.5 m, Kurniawan et al., 2018). Mechanical weeding, through increases in plant cover, tended to increase base saturation in the interrows, which can have positive feedback effects on plant cover. In the frond-piled area, we could not detect effects of decomposition on microbial biomass or base saturation. However, the latter was due to the fact that base cations were already saturated.
Overall, the lack of strong contrasts and measurable effects—or the presence of neutral responses—shows that mechanical-only weeding is a tenable plant cover management strategy that maintains and sometimes enhances soil functions compared to herbicide spraying. Mechanical weeding avoids the use of herbicides such as glyphosate, which is possibly harmful to humans and animals (Van Bruggen et al., 2018). Implementing it on larger scales should be unproblematic as even commercial plantations routinely use both chemical and mechanical weed control methods. Even in herbicide-sprayed plantations, periodical cuts are necessary to prevent the establishment of woody plants (such as the invasive Clidemia hirta).
We chose to compare treatments differing only in method (the weeding frequency stayed the same) because excessively high plant cover hampers management activities. Nonetheless, from a theoretical point of view, it could be interesting to compare more contrasting treatments, such as clean-weeding practices or enrichment planting (Teuscher et al., 2016), or low- and high-intensity weeding practices (Luke et al., accepted) against business-as-usual weeding methods. Such comparisons could show the trade-offs between weeding management intensity and ecosystem functions and biodiversity, and may detect non-linear patterns to reveal optimal management intensity levels. Our experiment, however, focuses on weeding management options that are in use or could be adopted by the oil palm industry. With the data that will be collected in the coming years, our results regarding soil processes and even oil palm productivity might change. We will assess the response change to our experimental treatments from short-term (within the first 2 years) vs. long-term effects (4–5 years) on nutrient leaching losses, soil greenhouse gas fluxes, nutrient response efficiency, and nutrient retention efficiency.
Outlook
Overall, the results of the first two years of our experiment suggest that more economically profitable plantations could be designed for enhancing biodiversity levels and natural ecosystem functions while reducing potential adverse environmental effects from excessive use of agro-chemicals. Future research campaigns will gather more data and synthesize findings about environmental footprints—together with indicators of below- and aboveground biodiversity and cost-benefit analysis—by analyzing the trade-offs between ecosystem functions and profit in order to holistically evaluate whether reduced management can abate negative impacts of palm oil production without sacrificing profit. These data will also be used in ecological-economic optimization modeling, which will aid stakeholders in their choices of management for sustainable oil palm production. Furthermore, these findings will also be used in evaluation of effects of policy, such as RSPO, using a tool like the Environmental and Social Accounting Matrices at a scale of local rural economy.
Most of our findings can be directly related to the RSPO's 17th principle to “Protect, conserve, and enhance ecosystems and the Environment,” which were recently updated (RSPO, 2018). RSPO requires Integrated Pest Management and regulates the use of pesticides (insecticides and herbicides). Integrated Pest Management systems were advocated decades ago when broad-spectrum insecticides were recognized as disruptive to the services of natural enemies of oil palm pests (Wood, 1971). Formally, RSPO criteria require that the application of pesticides—classed as pollutants—needs to be justified, monitored, and publicly reported. Workers have to be trained, prophylactic use is prohibited, and application is reduced to a minimum. However, critics of RSPO may argue that these criteria can be easily fulfilled as RSPO does not mention quantitative thresholds of pesticide application. Our mechanical weeding treatment results clearly show that a milder, entirely non-chemical weed control method is a viable management strategy. This is encouraged by RSPO criteria, and also required for organic farming certification.
Surrounding natural vegetation can also enhance biological pest control by acting as sources of arthropod predators (Nurdiansyah et al., 2016; Ganser et al., 2017). This emphasizes that in addition to the local effects of understory vegetation addressed here, the surrounding habitats in the landscape need to be considered too (Koh, 2008). Thus, High Conservation Value areas (Yaap, 2010)—which are a RSPO certification requirement—could also support biological pest control as part of an Integrated Pest Management strategy. The latest RSPO criteria update from 2018 not only requires closer monitoring and assessment of High Conservation Value areas, it also recommends the establishment of buffers around and corridors between them (Koh et al., 2009), which would support plant and animal populations. From a conservation point of view, however, large organisms need considerably bigger conservation areas to subsist (Turner, 1996; Edwards et al., 2012).
Further RSPO criteria that require the maintenance of soil fertility, the minimization of soil erosion, the improvement of surface and groundwater quality, and the reduction of greenhouse gases are also directly linked to our experimental treatments. Our findings indicate that reduced, compensatory fertilization levels in a mature plantation can maintain optimal yield at least during the first 2 years of management, and did not change the nutrient levels in the soil. These results also suggest that the conventional high fertilization rates were in excess and thus promote leaching (Kurniawan et al., 2018), which could diminish water quality and cause soil greenhouse gas emissions (Hassler et al., 2015, 2017). Mechanical weeding practices should also help to control soil erosion; even though we did not directly measure it, plant cover protects soil against erosion in oil palm plantations (Guillaume et al., 2016). Mechanical weeding, together with reduced fertilization, may minimize leaching of nutrients into surface and ground waters, which are water sources that are used by rural communities in our study region and are becoming increasingly scarce (Merten et al., 2016). Long-term management experiments are vital for science-based recommendations for sustainability of land use and policy implementation. Our findings from these initial years of this management experiment should be strengthened by continuous measurements with a long-term perspective.
Data Availability Statement
All datasets analyzed for this study are included in the manuscript/Supplementary Files.
Ethics Statement
As per the authors' institutions' guidelines as well as applicable national regulations, no ethics approval was required or obtained for the present study. This study was carried out on a state-owned oil palm estate. We obtained approval to access the site and sample animals therein by establishing a cooperation with PTPN VI, the estate owner. We only collected invertebrates that were not protected by Indonesian law, and vertebrates were passively detected without any disturbance. All research groups involved in the experiment obtained research permits from the Ministry of Research, Technology and Higher Education and sample collection and/or sample export permits from the Ministry of Environment and Forestry of the Republic Indonesia.
Author Contributions
KD was the scientific coordinator of the experiment, led the manuscript writing, and analyzed the data. MC, TT, and EV designed the experiment. MC, IG, TT, and EV were scientific supervisors of the experiment. KD, AT, and SY coordinated the experiment's field activities. JD, BI, SS, AT, and SY supported the establishment of the experiment. TK validated the statistical analysis. AAR, DB, FB, MC, JD, RF, GF, IG, TK, VK, HK, KL, MM, APol, APot, ARR, KR, CS, SS, KS, ST, AT, TT, AV-U, and EV contributed to the manuscript writing. AAR, FB, MC, RF, GF, DH, VK, AK, KL, APot, ARR, KR, CS, SS, KS, ST, AT, AV-U, and EV contributed data.
Funding
This study was funded by the Deutsche Forschungsgemeinschaft (DFG, German Research Foundation)–project number 192626868–SFB 990 in the framework of the collaborative German-Indonesian research project CRC990. AR holds a Ph.D. scholarship from the Indonesia Endowment Fund for Education (LPDP), the Ministry of Finance (KEMENKEU) and the Ministry of Research, Technology and Higher Education (RISTEKDIKTI) of the Republic of Indonesia under the BUDI-LN 2016 scholarship scheme. The PTPN VI company provided no funding and did not have any influence on the study design, data collection, analysis, or interpretation.
Conflict of Interest
The authors declare that the research was conducted in the absence of any commercial or financial relationships that could be construed as a potential conflict of interest.
Acknowledgments
We acknowledge the support by PT Perkebunan Nusantara VI (PTPN VI) for granting us access to and use of their oil palm plantation and for helping with the harvest. We are grateful to RISTEKDIKTI for providing research permits.
Supplementary Material
The Supplementary Material for this article can be found online at: https://www.frontiersin.org/articles/10.3389/ffgc.2019.00065/full#supplementary-material
References
Allen, K., Corre, M. D., Kurniawan, S., Utami, S. R., and Veldkamp, E. (2016). Spatial variability surpasses land-use change effects on soil biochemical properties of converted lowland landscapes in Sumatra, Indonesia. Geoderma 284, 42–50. doi: 10.1016/j.geoderma.2016.08.010
Allen, K., Corre, M. D., Tjoa, A., and Veldkamp, E. (2015). Soil nitrogen-cycling responses to conversion of lowland forests to oil palm and rubber plantations in Sumatra, Indonesia. PLoS ONE. 10:e0133325. doi: 10.1371/journal.pone.0133325
Ashton-Butt, A., Aryawan, A. A., Hood, A. S. C, Naim, M., Purnomo, D., Suhardi, et al. (2018). Understory vegetation in oil palm plantations benefits soil biodiversity and decomposition rates. Fron. For. Glob. Change. 1:10. doi: 10.3389/ffgc.2018.00010
Baldos, A. P., Corre, M. D., and Veldkamp, E. (2015). Response of N cycling to nutrient inputs in forest soils across a 1000–3000 m elevation gradient in the Ecuadorian Andes. Ecology. 96, 749–761. doi: 10.1890/14-0295.1
Bessou, C., Verwilghen, A., Beaudoin-Ollivier, L., Marichal, R., Ollivier, J., Baron, V., et al. (2017). Agroecological practices in oil palm plantations: examples from the field. OCL. 24:D305. doi: 10.1051/ocl/2017024
Bigelow, S. W., Ewel, J. J., and Haggar, J. P. (2004). Enhancing nutrient retention in tropical tree plantations: no short cuts. Ecol. Appl. 14, 28–46. doi: 10.1890/02-5389
Blake, G. R., and Hartge, K. H. (1986). “Bulk density,” in Methods of Soil Analysis (American Society of Agronomy and Soil Science Society of America).
Bolker, B. M., Brooks, M. E., Clark, C. J., Geange, S. W., Poulsen, J. R., Stevens, M. H., et al. (2009). Generalized linear mixed models: a practical guide for ecology and evolution. Trends Ecol. Evol. 24, 127–135. doi: 10.1016/j.tree.2008.10.008
Breure, C. J. (1985). Relevant factors associated with crown expansion in oil palm (Elaeis guineensisJacq.). Euphytica 34, 161–75. doi: 10.1007/BF00022876
Brinkmann, N., Schneider, D., Sahner, J., Ballauff, J., Edy, N., Barus, H., et al. (2019). Intensive tropical land use massively shifts soil fungal communities. Sci. Rep. 9:3403. doi: 10.1038/s41598-019-39829-4
Brookes, P. C., Landman, A., Pruden, G., and Jenkinson, D. S. (1985). Chloroform fumigation and the release of soil nitrogen: a rapid direct extraction method to measure microbial biomass nitrogen in soil. Soil Biol. Biochem. 17, 837–842. doi: 10.1016/0038-0717(85)90144-0
Bürkner P-C. (2017). brms: an R Package for Bayesian Multilevel Models Using Stan. J. Stat. Softw. 80, 1–28. doi: 10.18637/jss.v080.i01
Byerlee, D., Falcon, W. P., and Naylor, R. L. (2016). The Tropical Oil Crop Revolution: Food, Feed, Fuel, and Forests. Oxford University Press. doi: 10.1093/acprof:oso/9780190222987.001.0001
Chu, H., Fujii, T., Morimoto, S., Lin, X., and Yagi, K. (2008). Population size and specific nitrification potential of soil ammonia-oxidizing bacteria under long-term fertilizer management. Soil Biol. Biochem. 40, 1960–1963. doi: 10.1016/j.soilbio.2008.01.006
Clough, Y., Krishna, V. V., Corre, M. D., Darras, K., Denmead, L. H., Meijide, A., et al. (2016). Land-use choices follow profitability at the expense of ecological functions in Indonesian smallholder landscapes. Nat. Commun. 7:13137. doi: 10.1038/ncomms13137
Comte, I., Colin, F., Gr?nberger, O., Follainc, S., Whalena, J. K., and Calimane, J. P. (2013). Landscape-scale assessment of soil response to long-term organic and mineral fertilizer application in an industrial oil palm plantation, Indonesia. Agric. Ecosyst. Environ. 169, 58–68. doi: 10.1016/j.agee.2013.02.010
Corley, R. H. V., and Mok, C. K. (1972). Effects of nitrogen, phosphorus, potassium and magnesium on growth of the oil palm. Exp. Agric. 8, 347–353. doi: 10.1017/S0014479700005470
Corley, R. H. V., Ng, M., and Donough, C. R. (1995). Effects of defoliation on sex differentiation in oil palm clones. Exp. Agric. 31, 177–190. doi: 10.1017/S0014479700025266
Corley, R. H. V., and Tinker, P. B. (2016). The Oil Palm. Hoboken, NJ: John Wiley & Sons. doi: 10.1002/9781118953297
Corre, M. D., Sueta, J. P., and Veldkamp, E. (2014). Nitrogen-oxide emissions from tropical forest soils exposed to elevated nitrogen input strongly interact with rainfall quantity and seasonality. Biogeochemistry. 118, 103–20. doi: 10.1007/s10533-013-9908-3
Corre, M. D., Veldkamp, E., Arnold, J., and Wright, S. J. (2010). Impact of elevated N input on soil N cycling and losses in old-growth lowland and montane forests in Panama. Ecology. 91, 1715–1729. doi: 10.1890/09-0274.1
Cribari-Neto, F., and Zeileis, A. (2010). Beta regression in R. J. Stat. Softw. 34, 1–24. doi: 10.18637/jss.v034.i02
CSIRO (Ed). (1991). The Insects of Australia: A Textbook for Students and Research Workers. Carlton South, VIC: Melbourne University Press.
Cusack, D. F., Macy, J., and McDowell, W. H. (2016). Nitrogen additions mobilize soil base cations in two tropical forests. Biogeochemistry. 128, 67–88. doi: 10.1007/s10533-016-0195-7
Darras, K., Furnas, B., Fitriawan, I., Mulyani, Y., and Tscharntke, T. (2018). Estimating bird detection distances in sound recordings for standardizing detection ranges and distance sampling. Methods Ecol. Evol. 9, 1928–1938. doi: 10.1111/2041-210X.13031
Davidson, E. A., Keller, M., Erickson, H. E., Verchot, L. V., and Veldkamp, E. (2000). Testing a Conceptual Model of Soil Emissions of Nitrous and Nitric OxidesUsing two functions based on soil nitrogen availability and soil water content, the hole-in-the-pipe model characterizes a large fraction of the observed variation of nitric oxide and nitrous oxide emissions from soils. BioScience. 50, 667–680. doi: 10.1641/0006-3568(2000)050[0667:TACMOS]2.0.CO;2
del Hoyo, J., Collar, N, A., Christie, D, Elliott, A., and Fishpool, L. D. C. (2014). HBW and BirdLife International Illustrated Checklist of the Birds of the World, Vol. 1: Non-passerines. Barcelona: Lynx Edicions in association with Birdlife International.
del Hoyo, J., Collar, N., Christie, D. A., Elliott, A., Fishpool, L. D. C., Boesman, P., et al. (2016). HBW and BirdLife International Illustrated Checklist of the Birds of the World. Vol. 2. Barcelona: Lynx Edicions in association with Birdlife International.
Direktorat Jenderal Perkebunan (2015). Statistic Perkebunan Indonesia 2014–2016 Kelapa Sawit. Jakarta: Direktorat Jenderal Perkebunan.
Dislich, C., Keyel, A. C., Salecker, J., Kisel, Y., Meyer, K. M., Auliya, M., et al. (2017). A review of the ecosystem functions in oil palm plantations, using forests as a reference system. Biol. Rev. 92, 1539–1569. doi: 10.1111/brv.12295
Drescher, J., Rembold, K., Allen, K., Beckschäfer, P., Buchori, D., Clough, Y., et al. (2016). Ecological and socio-economic functions across tropical land use systems after rainforest conversion. Phil. Trans. R. Soc. B. 371:20150275. doi: 10.1098/rstb.2015.0275
Edwards, D. P., Fisher, B., and Wilcove, D. S. (2012). High conservation value or high confusion value? Sustainable agriculture and biodiversity conservation in the tropics. Conserv. Lett. 5, 20–27. doi: 10.1111/j.1755-263X.2011.00209.x
Ehnes, R. B., Rall, B. C., and Brose, U. (2011). Phylogenetic grouping, curvature and metabolic scaling in terrestrial invertebrates. Ecol. Lett. 14, 993–1000. doi: 10.1111/j.1461-0248.2011.01660.x
Euler, M., Krishna, V., Schwarze, S., Siregar, H., and Qaim, M. (2017). Oil palm adoption, household welfare, and nutrition among smallholder farmers in Indonesia. World Develop. 93, 219–235. doi: 10.1016/j.worlddev.2016.12.019
Ewel, J. J., and Putz, F. E. (2004). A place for alien species in ecosystem restoration. Front. Ecol. Environ. 2, 354–60. doi: 10.1890/1540-9295(2004)002[0354:APFASI]2.0.CO;2
Food Agriculture Organization (2018). FAOSTAT. Available online at: http://faostat.fao.org/site/339/default.aspx (accessed February 25, 2019).
Foster, W. A., Snaddon, J. L., Turner, E. C., Fayle, T. M., Cockerill, T. D., Ellwood, M. D., et al. (2011). Establishing the evidence base for maintaining biodiversity and ecosystem function in the oil palm landscapes of South East Asia. Philos. Trans. R. Soc. Lond. B Biol. Sci. 366, 3277–3291. doi: 10.1098/rstb.2011.0041
Fukuda, D., Tisen, O. B., Momose, K., and Sakai, S. (2009). Bat diversity in the vegetation mosaic around a lowland dipterocarp forest of Borneo. Raffles Bull. Zool. 57, 213–221.
Ganser, D., Denmead, L. H., Clough, Y., Buchori, D., and Tscharntke, T. (2017). Local and landscape drivers of arthropod diversity and decomposition processes in oil palm leaf axils. Agr. For. Entomol. 19, 60–69. doi: 10.1111/afe.12181
Goh, K. J., and Teo, C. B. (2011). “Agronomic principles and practices of fertilizer management of oil palm,” in Agronomic Principles and Practices of Oil Palm Cultivation, 241–3181.
Gregory, J. H., Dukes, M. D., Miller, G. L., and Jones, P. H. (2005). Analysis of double-ring infiltration techniques and development of a simple automatic water delivery system. Appl. Turfgrass Sci. 2. doi: 10.1094/ATS-2005-0531-01-MG
Guillaume, T., Holtkamp, A. M., Damris, M., Brümmer, B., and Kuzyakov, Y. (2016). Soil degradation in oil palm and rubber plantations under land resource scarcity. Agric. Ecosyst. Environ. 232, 110–118. doi: 10.1016/j.agee.2016.07.002
Hanum, C., Rauf, A., Fazrin, D. A., and Habibi, A. R. (2016). Nitrogen, phosphor, and potassium level in soil and oil palm tree at various composition of plant species mixtures grown. IOP Conf. Ser. Earth Environ. Sci. 41:012008. doi: 10.1088/1755-1315/41/1/012008
Haruna, S. I., Nkongolo, N. V., Anderson, S. H., Eivazi, F., and Zaibon, S. (2018). In situ infiltration as influenced by cover crop and tillage management. J. Soil Water Conserv. 73, 164–172. doi: 10.2489/jswc.73.2.164
Hassler, E., Corre, M. D., Kurniawan, S., and Veldkamp, E. (2017). Soil nitrogen oxide fluxes from lowland forests converted to smallholder rubber and oil palm plantations in Sumatra, Indonesia. Biogeosciences. 14, 2781–2798. doi: 10.5194/bg-14-2781-2017
Hassler, E., Corre, M. D., Tjoa, A., Utami, S. N. H., and Veldkamp, E. (2015). Soil fertility controls soil?atmosphere carbon dioxide and methane fluxes in a tropical landscape converted from lowland forest to rubber and oil palm plantations. Biogeosciences. 12, 5831–5852. doi: 10.5194/bg-12-5831-2015
Hothorn, T., Bretz, F., and Westfall, P. (2008). Simultaneous inference in general parametric models. Biom J. 50, 346–363. doi: 10.1002/bimj.200810425
Jambari, A., Azhar, B., Ibrahim, N. L., Jamian, S., Hussint, A., Puan, C. L., et al. (2012). Avian biodiversity and conservation in Malaysian oil palm production areas. J. Oil Palm Res. 24, 1277–1286.
Johnson, N. F., and Triplehorn, C. A. (2004). Borror and DeLong's Introduction to the Study of Insects. Cengage Learning.
Kempson, D., Lloyd, M., and Ghelardi, R. (1963). A new extractor for woodland litter. Pedobiologia. 3, 1–21.
Kheong, L., Rahman, A. Z., Hanafi, M., and Hussein, A. (2010). Empty fruit bunch application and oil palm root proliferation. J. Oil Palm Res. 22, 750–757.
Klarner, B., Winkelmann, H., Krashevska, V., Maraun, M., Widyastuti, R., and Scheu, S. (2017). Trophic niches, diversity and community composition of invertebrate top predators (Chilopoda) as affected by conversion of tropical lowland rainforest in Sumatra (Indonesia). PLoS ONE. 12:e0180915. doi: 10.1371/journal.pone.0180915
Klinge, R., Martins, A. R. A., Mackensen, J., and Fölster, H. (2004). Element loss on rain forest conversion in East Amazonia: comparison of balances of stores and fluxes. Biogeochemistry. 69, 63–82. doi: 10.1023/B:BIOG.0000031040.38388.9b
Koh, L. P. (2008). Can oil palm plantations be made more hospitable for forest butterflies and birds? J. Appl. Ecol. 45, 1002–1009. doi: 10.1111/j.1365-2664.2008.01491.x
Koh, L. P., Levang, P., and Ghazoul, J. (2009). Designer landscapes for sustainable biofuels. Trends Ecol. Evol. 24, 431–438. doi: 10.1016/j.tree.2009.03.012
Kurniawan, S., Corre, M. D., Matson, A. L., Schulte-Bisping, H., Rahayu Utami, S. N. H., van Straaten, O., et al. (2018). Conversion of tropical forests to smallholder rubber and oil palm plantations impacts nutrient leaching losses and nutrient retention efficiency in highly weathered soils. Biogeosciences. 15, 5131–5154. doi: 10.5194/bg-15-5131-2018
Luke, S., Purnomo, D., Advento, A., Aryawan, A. A. K., Naim, M., Pikstein, R. N., et al. (2019). Effects of understory vegetation management on plant communities in oil palm plantations in Sumatra, Indonesia. Front. For. Glob. Change. 2:33. doi: 10.3389/ffgc.2019.00033
Lungu, O. I., and Dynoodt, R. F. (2008). Acidification from long-term use of urea and its effect on selected soil properties. Afr. J. Food Agric. Nutr. Develop. 8, 63–76. doi: 10.4314/ajfand.v8i1.19180
Macaulay Library. Macaulay Library at the Cornell Lab of Ornithology (ML529861). Available online at: https://www.macaulaylibrary.org/ (accessed July 4, 2019).,
Margono, B. A., Potapov, P. V., Turubanova, S., Stolle, F., and Hansen, M. C. (2014). Primary forest cover loss in Indonesia over 2000–2012. Nat. Clim. Change. 4, 730–735. doi: 10.1038/nclimate2277
Merten, J., Röll, A., Guillaume, T., Meijide, A., Tarigan, S., Agusta, H., et al. (2016). Water scarcity and oil palm expansion: social views and environmental processes. Ecol. Soc. 21:5. doi: 10.5751/ES-08214-210205
Nazeeb, M., Tang, M. K., Letchumanan, A., and Loong, S. G. (1993). “Trials on cessation of manuring before replanting,” in Proceedings of the International Palm Oil Congress (Pipoc).
Nurdiansyah, F., Denmead, L. H., Clough, Y., Wiegand, K., and Tscharntke, T. (2016). Biological control in Indonesian oil palm potentially enhanced by landscape context. Agric. Ecosyst. Environ. 232, 141–149. doi: 10.1016/j.agee.2016.08.006
Pardo, L. E., Campbell, M. J., Cove, M. V., Edwards, W., Clements, G. R., and Laurance, W. F. (2019). Land management strategies can increase oil palm plantation use by some terrestrial mammals in Colombia. Sci. Rep. 9:7812. doi: 10.1038/s41598-019-44288-y
Potapov, A. M., Klarner, B., Sandmann, D., Widyastuti, R., and Scheu, S. (2019). Linking size spectrum, energy flux and trophic multifunctionality in soil food webs of tropical land-use systems. J. Anim. Ecol. doi: 10.1111/1365-2656.13027
R Core Team (2019). R: A Language and Environment for Statistical Computing. Vienna: R Foundation for Statistical Computing.
Rembold, K., Mangopo, H., Tjitrosoedirdjo, S. S., and Kreft, H. (2017). Plant diversity, forest dependency, and alien plant invasions in tropical agricultural landscapes. Biol. Conserv. 213, 234–242. doi: 10.1016/j.biocon.2017.07.020
Rosenzweig, C., Iglesias, A., Yang, X. B., Epstein, P. R., and Chivian, E. (2001). Climate change and extreme weather events; implications for food production, plant diseases, and pests. Glob. Change Hum. Health. 2, 90–104. doi: 10.1023/A:1015086831467
Schlesinger, W. H., and Bernhardt, E. S. (2013). Biogeochemistry: An Analysis of Global Change, 3rd Edn. Amsterdam; Boston, MA: Academic Press.
Sidhu, M., CheongKeng, K., Sinuraya, Z., Kurniawan, M., and Hasyim, A. (2009). Resumption of manuring and its impact on the nutrient status, growth and yield of unfertilised oil palm. Planter. 85, 675–689.
Singh, R. P., Ibrahim, M. H., Esa, N., and Iliyana, M. S. (2010). Composting of waste from palm oil mill: a sustainable waste management practice. Rev. Environ. Sci. Biotechnol. 9, 331–344. doi: 10.1007/s11157-010-9199-2
Teuscher, M., Gérard, A., Brose, U., Buchori, D., Clough, Y., Ehbrecht, M., et al. (2016). Experimental biodiversity enrichment in oil-palm-dominated landscapes in Indonesia. Front. Plant Sci. 7:1538. doi: 10.3389/fpls.2016.01538
Thirumalai, K., DiNezio, P. N., Okumura, Y., and Deser, C. (2017). Extreme temperatures in Southeast Asia caused by El Niño and worsened by global warming. Nat. Commun. 8:15531. doi: 10.1038/ncomms15531
Turner, I. M. (1996). Species loss in fragments of tropical rain forest: a review of the evidence. J. Appl. Ecol. 33, 200–209. doi: 10.2307/2404743
Van Bruggen, A. H. C., He, M. M., Shin, K., Mai, V., Jeong, K. C., Finckh, M. R., et al. (2018). Environmental and health effects of the herbicide glyphosate. Sci. Total Environ. 616–617, 255–268. doi: 10.1016/j.scitotenv.2017.10.309
Wickham, H. (2009). Ggplot2: Elegant Graphics for Data Analysis. New York, NY: Springer. doi: 10.1007/978-0-387-98141-3
Wood, B. J. (1971). “Development of integrated control programs for pests of tropical perennial crops in Malaysia” in Biological Control, ed C. B. Huffaker (Boston, MA: Springer US), 422–457. doi: 10.1007/978-1-4615-6531-4_19
Xeno-canto Foundation (2012). Xeno-canto: Sharing Bird Sounds from Around the World. Xeno-canto Foundation Amsterdam.
Yaap, B. (2010). Mitigating the biodiversity impacts of oil palm development. CAB Rev. Perspect. Agric. Vet. Sci. Nutr. Natural Resour. 5, 1–11. doi: 10.1079/PAVSNNR20105019
Yahya, Z., Tarmizi Mohammed, A., Harun, M. H., and Shuib, R. A. (2013). Oil palm adaptation to compacted alluvial soil (Typic Endoaquepts) in Malaysia. J. Oil Palm Res. 24, 1533–1541.
Keywords: fertilizer, weeding, land use change, oil palm, gross margin, biodiversity, ecosystem functions, monoculture
Citation: Darras KFA, Corre MD, Formaglio G, Tjoa A, Potapov A, Brambach F, Sibhatu KT, Grass I, Rubiano AA, Buchori D, Drescher J, Fardiansah R, Hölscher D, Irawan B, Kneib T, Krashevska V, Krause A, Kreft H, Li K, Maraun M, Polle A, Ryadin AR, Rembold K, Stiegler C, Scheu S, Tarigan S, Valdés-Uribe A, Yadi S, Tscharntke T and Veldkamp E (2019) Reducing Fertilizer and Avoiding Herbicides in Oil Palm Plantations—Ecological and Economic Valuations. Front. For. Glob. Change 2:65. doi: 10.3389/ffgc.2019.00065
Received: 22 March 2019; Accepted: 04 October 2019;
Published: 05 November 2019.
Edited by:
Janice Ser Huay Lee, Nanyang Technological University, SingaporeReviewed by:
Frances Claire Manning, University of Aberdeen, United KingdomLoren P. Albert, Brown University, United States
Copyright © 2019 Darras, Corre, Formaglio, Tjoa, Potapov, Brambach, Sibhatu, Grass, Rubiano, Buchori, Drescher, Fardiansah, Hölscher, Irawan, Kneib, Krashevska, Krause, Kreft, Li, Maraun, Polle, Ryadin, Rembold, Stiegler, Scheu, Tarigan, Valdés-Uribe, Yadi, Tscharntke and Veldkamp. This is an open-access article distributed under the terms of the Creative Commons Attribution License (CC BY). The use, distribution or reproduction in other forums is permitted, provided the original author(s) and the copyright owner(s) are credited and that the original publication in this journal is cited, in accordance with accepted academic practice. No use, distribution or reproduction is permitted which does not comply with these terms.
*Correspondence: Kevin F. A. Darras, a2RhcnJhc0Bnd2RnLmRl