- 1Department of Forestry and Wildland Resources, Humboldt State University, Arcata, CA, United States
- 2Department of Biological Sciences, Humboldt State University, Arcata, CA, United States
- 3Department of Wildlife, Humboldt State University, Arcata, CA, United States
Reforestation following timber harvests and natural disturbances is an essential component of sustainable forest management. As disturbances such as drought-induced mortality and wildland fires spread across many forests of the western United States, a better understanding of the influences of stand structure on seedling physiology can foster more effective reforestation efforts. Moreover, as climate throughout the West is projected to become hotter and drier, it is important to investigate regeneration under xeric conditions, particularly for species restricted to mesic habitats. To study the influences of stand structure and climate on regeneration success, we monitored physiology [water potential (Ψ) and stomatal conductance (gs)], growth (change in basal diameter and biomass accumulation), and mortality rate of planted Douglas-fir (Pseudotsuga menziesii) and coast redwood (Sequoia sempervirens) seedlings for two growing seasons after partial harvesting in inland northern California. Compared to seedlings in the no-cut and moderate-retention treatments, seedlings in the gap (100% cut) treatment had the highest Ψ and gs, greatest growth, and lowest mortality. We also found that compared to Douglas-fir, redwood had higher Ψ and gs, greater growth, and lower mortality. Overall, our study indicates that low-retention silvicultural treatments can minimize water stress and maximize gas exchange, growth, and survival in regenerating seedlings. Our results also demonstrate that redwood, a species generally restricted to mesic coastal habitats, can successfully establish in xeric inland sites when planted after partial harvesting, even during drought conditions.
Introduction
Global climate change is forecast to have widespread impacts on forested ecosystems (Jia et al., 2019). The forecasted increase in drier climates worldwide, with increasing exposure to temperature and rainfall extremes, has the potential to alter forest structure and function (Allen et al., 2010). Many regions can expect warming of extreme temperatures, some regions can expect increases in drought intensity or frequency, and drought-prone regions can expect more severe droughts (Jia et al., 2019). Forest management to foster productive, healthy, resistant, and resilient forests in the face of increasing drought frequency and severity is an important and widespread goal across many land ownerships (Keenan, 2015; Newton and Preest, 1988; Millar and Stephenson, 2015).
Drought-induced forest mortality and subsequent regeneration patterns are major concerns in the western United States. In parts of the central and southern Sierra Nevada mountains of California, epidemic tree mortality has exceeded 50% for some species (Asner et al., 2016; Byer and Jin, 2017; Young et al., 2017; Stephenson et al., 2019), with millions of trees having died in response to the recent 2012 – 2015 drought (Plamboeck et al., 2008; Fettig et al., 2019). As forests are an important national renewable resource that supply valuable timber products and support diverse economies, this loss of resources reduces timber production and carbon sequestration and also increases the risk of catastrophic wildland fires (Jolly et al., 2015). Droughts are therefore a major threat to forest health and perpetuation in the West, as water stress can reduce tree hydraulic function via excessive xylem cavitation and can reduce tree carbon uptake via excessive stomatal regulation to conserve water (McDowell et al., 2008). Following drought-induced tree mortality in a climate predicted to continue warming and drying for decades (Allen et al., 2010, 2015), the successful establishment and regeneration of former forestlands is dubious, as water stress can threaten successful seedling establishment in Mediterranean climates with hot, dry summers (Plamboeck et al., 2008). More specifically, water stress can reduce water potential (Ψ), stomatal conductance (gs), and photosynthetic rates in seedlings (Ritchie and Shula, 1984; Warren et al., 2004). Reduced Ψ risks cavitating xylem tissue, thereby lowering plant hydraulic conductance (Tyree and Sperry, 1989), while reduced photosynthesis potentially lowers growth rates. Furthermore, seedling growth rates during establishment can strongly influence later growth rates in older trees (Newton and Preest, 1988), such that the establishment phase of a seedling’s life can have lasting impacts on forest productivity.
Management of western forests is needed to improve forest health and productivity and can produce variable responses depending on treatment prescription, species, climate, and location (O’Hara et al., 2010; Ryan et al., 2013; Sohn et al., 2016). Due in part to fire exclusion over the past century, many forests are overstocked and require thinning and/or prescribed fire to reduce competition among residual trees. There are many treatment options available, including prescriptions that enhance variability and complexity of forest structure such as variable-density thinning (O’Hara et al., 2010; Dagley et al., 2018) and variable retention (Berrill and O’Hara, 2007). Through these types of treatments, trees are retained in a mosaic of patches with different densities to foster the regeneration of a new cohort (Berrill et al., 2018). Removing varying numbers of overstory trees manipulates the understory environment for regenerating seedlings in numerous ways. Typical shifts include increased light, throughfall precipitation, understory vegetation growth, and evapotranspiration (Simonin et al., 2007). While increased light and precipitation potentially improve seedling success, increased understory competition, soil water evaporation, and over- and understory transpiration potentially reduce seedling success. Understanding how these novel variable-density prescriptions influence establishment success and development of regenerating seedlings is important to improve reforestation efforts and enhance forest productivity and resilience.
Given the trends of warming and drying climate, how new cohorts will regenerate after various degrees of overstory removal remains an open question for many forest types. We investigated seedling success of two important California timber species after partial harvesting under drought conditions. Coast Douglas-fir [Pseudotsuga menziesii var. menziesii (Mirbel) Franco] is a widespread conifer that ranges from British Columbia, Canada to California, United States and tolerates mesic to dry environments, while coast redwood [Sequoia sempervirens (D. Don) Endl.] is a conifer restricted to the mesic fog belt of the Pacific coast in northern California and southern Oregon (Stuart and Sawyer, 2001). Using Ψ, gs, basal diameter growth, biomass accumulation, and mortality to evaluate seedling success in interior northern California during drought, we investigated the following questions and corresponding hypotheses: (1) How does thinning treatment influence physiology, growth, and mortality? We hypothesized that seedlings in moderate treatments would be the most vigorous in these metrics due to an optimal increase in light availability buffered with partial shading from the residual overstory to minimize excessive evapotranspirational water losses. (2) How do physiology, growth, and mortality differ between Douglas-fir and redwood seedlings? We hypothesized that Douglas-fir, a species with an expansive range across many habitat types, would be more vigorous in these metrics than redwood, a species with a narrow range restricted to the fog belt of coastal northern California and Southern Oregon. And (3) How does seedling physiology vary seasonally? We hypothesized that Ψ and gs would decrease across the growing season as winter water inputs exhausted and temperatures increased.
Materials and Methods
Study Site
Our study site was the L.W. Schatz Demonstration Tree Farm in Maple Creek, CA (N 40° 46′ 30.306″ N, W 123° 51′ 58.036″ W). The soils have an average depth of 2 m, are classified as sandstone and mudstone and are well-drained gravelly clay loams, very gravelly loam, or loams that form on mountain slopes (Soil Survey Staff, 2017). This site is approximately 160 m elevation above sea level and 27 km from the Pacific Ocean. This area receives the majority of annual precipitation during the winter months and experiences hot, dry summers. Of the 1640 mm of mean annual rainfall (based on 2013–2018 data), only 30 mm (2%) fall in the summer months (June, July, August); mean maximum monthly temperature ranges annually between 19°C in December and 39°C in September1. Field-based measurements occurred in 2015 and 2016. At this location, 2015 was a relatively dry year (1200 mm precipitation) compared to 2016 (1810 mm precipitation), with both years being considered drought [Palmer Drought Severity Indices (PDSI) of −2.7 and −1.6, respectively)2. We note that these two dry years were likely particularly stressful for establishing seedlings, as 2012–2015 represents one of the most severe droughts in California’s history (Asner et al., 2016). This site is located outside of the Pacific Coast’s fog belt and the natural range of coast redwood. After the old-growth Douglas-fir-dominated forest was clear-cut circa 1960, a dense second-growth forest regenerated with an overstory largely composed of Douglas-fir, grand fir [Abies grandis (Douglas ex D. Don) Lindl.], tanoak (Notholithocarpus densiflorus Manos), madrone (Arbutus menziesii Pursh), California bay (Umbellularia californica Nuttall), and bigleaf maple (Acer macrophyllum Pursh). Common understory plants include western sword fern (Polystichum munitum (Kaulf.) C. Presl), poison-oak [Toxicodendron diversilobum (Torr. and A. Gray) Greene], evergreen huckleberry (Vaccinium ovatum Pursh), red huckleberry (Vaccinium parvifolium Sm.), and salal (Gaultheria shallon Pursh), among many others (Berrill et al., 2018).
Seedlings
Prior to planting, seedlings were grown by a local commercial nursery from local seed zone (092) seeds. Seedlings were planted within a 0.6 ha study area on a north-facing slope. This study area was divided into 15 0.04 ha (20 × 20 m) neighboring treatment squares. Across these 15 squares, one of five treatments was randomly applied to each square in the Fall of 2014, such that each treatment had three replicates. The treatments included: no-cut (control), high-density retention, moderate-density retention, low-density retention, and gaps (100% cut). Post-treatment, target stand density index (SDI) was 675, 450, and 225 in the high-, moderate-, and low-density retention treatments, respectively, with no-cut treatments naturally having SDI values of 1000, 1050, and 1600. For more details of the study layout, see Berrill et al. (2018). Forest structure based on all treated plots at this site was highly variable, with basal area ranging from 0 to 110 m2 ha–1 (mean = 22 m2 ha–1), canopy openness ranging from 0 to 100% (mean = 30%), leaf area index ranging from 0.75 to 1.90 (mean = 1.28), understory vegetation cover ranging from 0 to 100% (mean = 30%), and fern cover ranging from 0 to 100% (mean = 17%) (Berrill et al., 2018). In early spring 2015, 1-year-old styro-15 containerized Douglas-fir and redwood seedlings were hand planted in one no-cut treatment, one moderate-density retention treatment, and one gap treatment. In each of these three treatments, 15 seedlings were planted per species, for a total of 90 seedlings. On average, Douglas-fir seedlings were 51 ± 1 cm tall with a basal diameter of 3.6 ± 0.1 mm and redwood seedlings were 33 ± 1 cm tall with a basal diameter of 2.6 ± 0.2 mm.
Physiology and Light Measurements
Physiology was measured during the 2015 and 2016 growing seasons (May through October). Throughout each growing season, field measurements were taken approximately twice a month. We measured predawn (ΨPD) and midday Ψ (ΨMD) water potential to quantify the least and greatest daily water stress, respectively. To evaluate leaf-level carbon uptake, we measured leaf-level stomatal conductance of water vapor (gs) as a proxy, as both gases (water and carbon dioxide) are exchanged between the leaf and atmosphere through stomata and the two rates are positively correlated (Skov et al., 2004). In 2015, we measured ΨPD and ΨMD, and in 2016 we measured ΨPD, ΨMD, and gs. To compensate for seedling mortality in 2015, replacement seedlings were recruited from within each treatment plot for 2016 physiology measurements; these replacements were from the same nursery and planted at the same time as the original cohort of seedlings. Predawn measurements were taken approximately 2 h before sunrise and midday measurements were taken approximately between 1100 and 1300. A pressure chamber (Model 600, PMS Instruments, Corvalis, OR, United States) was used to measure Ψ. Due to the destructive sampling required for pressure chamber readings, on each sampling day, only ten (randomly selected) of the 15 seedlings per species were measured per treatment; this approach minimized the negative impacts of sampling on seedlings and also ensured that there was sufficient plant material for bi-weekly sampling across two growing seasons. On a given sampling day, gs was measured on the same ten seedlings used for Ψ measurements. For gs measurements, a leaf porometer (Model SC-1, Decagon Devices, Pullman, WA, United States) was used to take three midday readings per seedling (on different leaves) per sampling day; these three measurements were averaged into a single value for that plant on that day. For each gs measurement, multiple needles were carefully laid in parallel to ensure that the porometer aperture was fully covered. On each field measurement day, maximum light availability was measured in the center of each treatment at midday using a light meter (Model LT300, Extech Instruments, Nashua, NH, United States); for these measurements, the light meter was left on for ten minutes to record the highest light-level measured.
Growth and Mortality Measurements
In April 2015, shortly after planting, the basal diameter of each seedling was measured near ground level above any swelling using calipers. In October 2016, these measurements were repeated to quantify growth during the 2-year study as a function of percent increase in basal diameter:
where D2016 is basal diameter in October 2016 and Di is initial basal diameter in April 2015 after planting. We used change in basal diameter, rather than height, to quantify growth due to the fact that animal browse and/or destructive sampling for pressure chamber readings may have influenced seedling heights. In November 2016, all seedlings were harvested to quantify biomass accumulation. Seedling mortality within each treatment was quantified for both species in November of 2015 and 2016 to evaluate mortality in the first and second years, respectively, following planting. Seedlings were classified as dead when all foliage was brown and branch xylem was brittle and dry. When harvesting seedlings, as much of each seedling’s root system as possible was collected; each seedling was divided into above- (stem, branches, and leaves) and belowground (roots) components. Seedling components were oven-dried for 72 h at 60°C and then weighed to determine biomass. These final biomass values were used to evaluate trends in biomass accumulation. Biomass was evaluated as the percent aboveground biomass accumulated relative to the total biomass accumulated to control for the unknown starting dry mass of each seedling, which was impossible to measure. Additionally, although animal browse potentially decreased measurable aboveground biomass accumulation, there were very few (<5) notable instances of animal (e.g., deer, elk, and rodents) browse on seedlings observed throughout the study. Basal diameter growth and biomass calculations only used living seedlings from the original 2015 cohort and are therefore based on a reduced sample size due to mortality.
Statistical Analyses
All statistical analyses were done using JMP (SAS Institute, Cary, NC, United States). To evaluate response variables (light, Ψ, gs, biomass, mortality), one- and two-way ANOVAs were done using treatment, species, month, year, and their interactions as effects. Repeated measures ANOVA were used to evaluate monthly light, Ψ, and gs measurements. Shapiro-Wilk goodness of fit tests were used to test the assumption that data were normally distributed; if this assumption was violated, Kruskal-Wallis tests were used to detect significant differences among groups. Levine and Bartlett tests were used to test the assumption of equal variance among groups; if this assumption was violated, Welch tests were used to detect significant differences among groups. If significant differences among groups were found, Tukey’s HSD multiple means comparisons were used to determine how groups differed. For all statistical analyses, we used α = 0.05.
Results
All results are reported as mean ± standard error and are summarized in Table 1. There was no difference in light availability between 2015 and 2016 in plot centers (X2 = 3.5, df = 1, p = 0.06); data from both years were therefore pooled for a better estimate of monthly light levels. Neither treatment (F = 1.4, df = 2,6, p = 0.32) nor month (F = 4.1, df = 5,2, p = 0.21) were significant effects on light availability. Across the two growing seasons, mean light (photosynthetic photon flux density, PPFD) was greatest in the gap (561 ± 99 μmol m–2 s–1) compared to the moderate-retention (326 ± 74 μmol m–2 s–1) and no-cut (302 ± 82 μmol m–2 s–1) treatments, although these differences were not significant (X2 = 4.2, df = 2, p = 0.13). Across all treatments, monthly mean light between May and August was significantly reduced in September and October (X2 = 12.5, df = 5, p = 0.03), with the highest and lowest mean light levels occurring in July (546 ± 137 μmol m–2 s–1) and October (111 ± 33 μmol m–2 s–1), respectively.
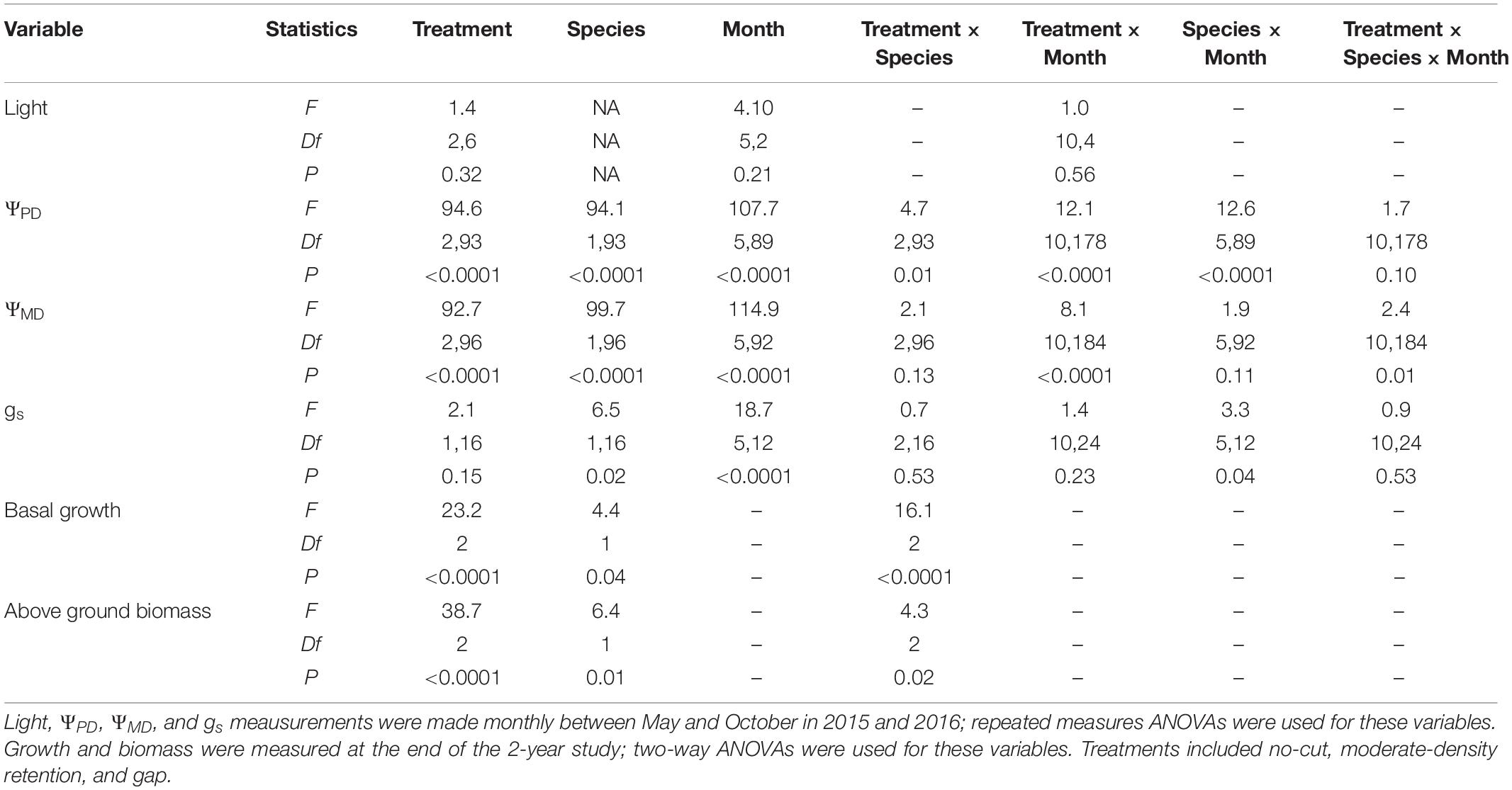
Table 1. ANOVA Statistics for light, predawn water potential (ΨPD), midday water potential (ΨMD), stomatal conductance (gs), basal diameter growth, and aboveground biomasss accumulation in Douglas-fir and redwood seedlings after partial harvesting treatments in Maple Creek, CA.
Physiology varied with treatment, species, and month. For ΨPD, treatment (F = 94.6, df = 2,93, p < 0.0001), species (F = 94.1, df = 1,93, p < 0.0001), and month (F = 107.7, df = 5,89, p < 0.0001) were all significant effects. In almost all sampling months, ΨPD was greatest in the gap treatment and lowest in the no-cut treatment (Figure 1A). Between species, ΨPD was lower in Douglas-fir compared to redwood in almost all sampling months (Figure 1B). In 2015 and 2016, the lowest ΨPD values were measured in August and September, respectively. Midday Ψ followed similar trends as ΨPD, with treatment (F = 92.7, df = 2,96, p < 0.0001), species (F = 99.7, df = 1,96, p < 0.0001), and month (F = 114.9, df = 5,92, p < 0.0001) all being significant effects. In almost all sampling months, ΨMD was lowest in the no-cut treatment and was generally highest in the gap treatment (Figure 1C). Between species, ΨMD was lower in Douglas-fir compared to redwood in most sampling months (Figure 1D). In 2015 and 2016, the lowest ΨMD values were measured in August and September, respectively. For gs, species (F = 6.5, df = 1,16, p = 0.02) and month (F = 3.3, df = 5,12, p = 0.04) were significant effects, but treatment was not (F = 2.1, df = 1,16, p = 0.15). Among treatments, gs was generally highest in the gap plot, although this was only significant in August (X2 = 12.7, df = 2, p = 0.002) and September (X2 = 15.0, df = 2, p = 0.001, Figure 2A). Between species, gs was almost always higher in redwood compared to Douglas-fir, although this was only significant in July (X2 = 7.3, df = 1, p = 0.01) and August (X2 = 4.1, df = 1, p = 0.04, Figure 2B). Generally, gs decreased across the growing season between May and October.
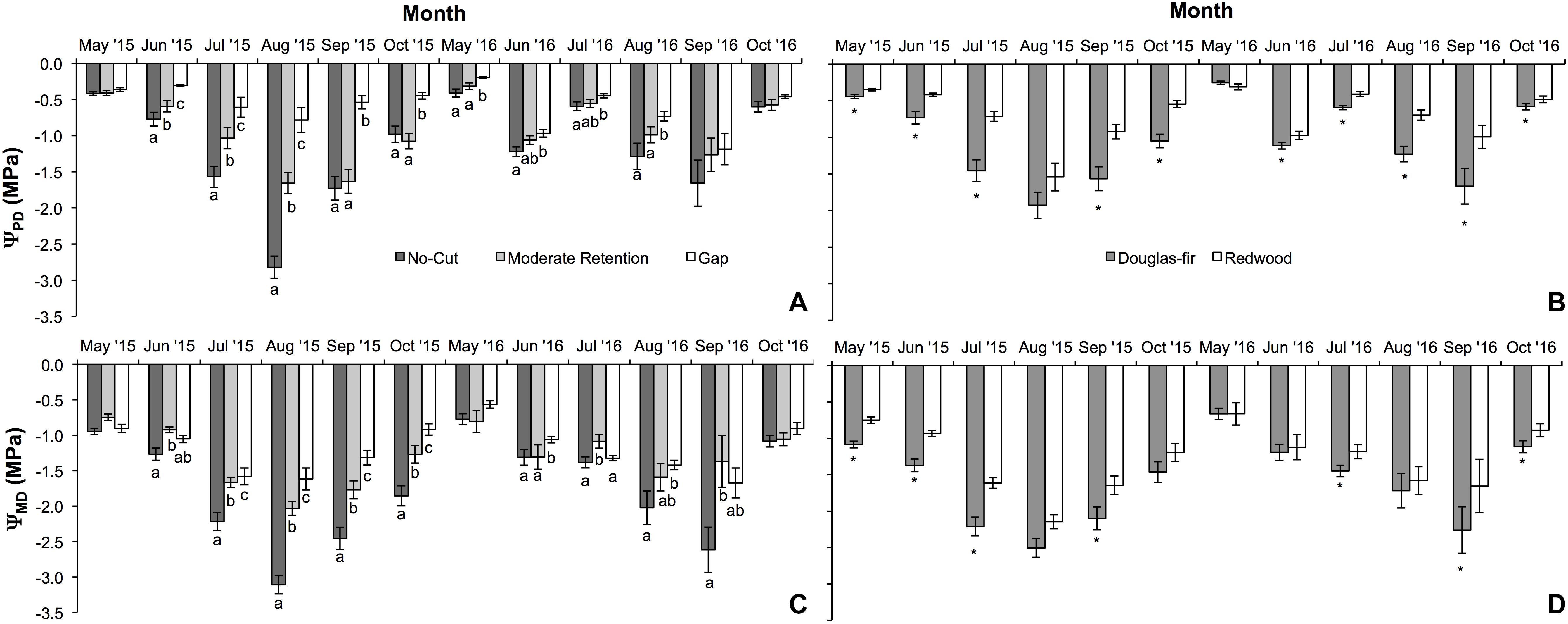
Figure 1. Mean (±SE) predawn (ΨPD) and midday (ΨMD) water potential measurements during the first two growing seasons (2015 and 2016) for Douglas-fir and redwood seedlings planted after partial harvesting in Maple Creek, CA. (A) Predawn Ψ among treatments for both species pooled, (B) predawn Ψ between species for all treatments pooled, (C) midday Ψ among treatments for both species pooled, and (D) midday Ψ between species for all treatments pooled. In panels (A,C), within a month, treatments not sharing the same letter are significantly different; in panels (B,D), asterisks represent a significant difference between species.
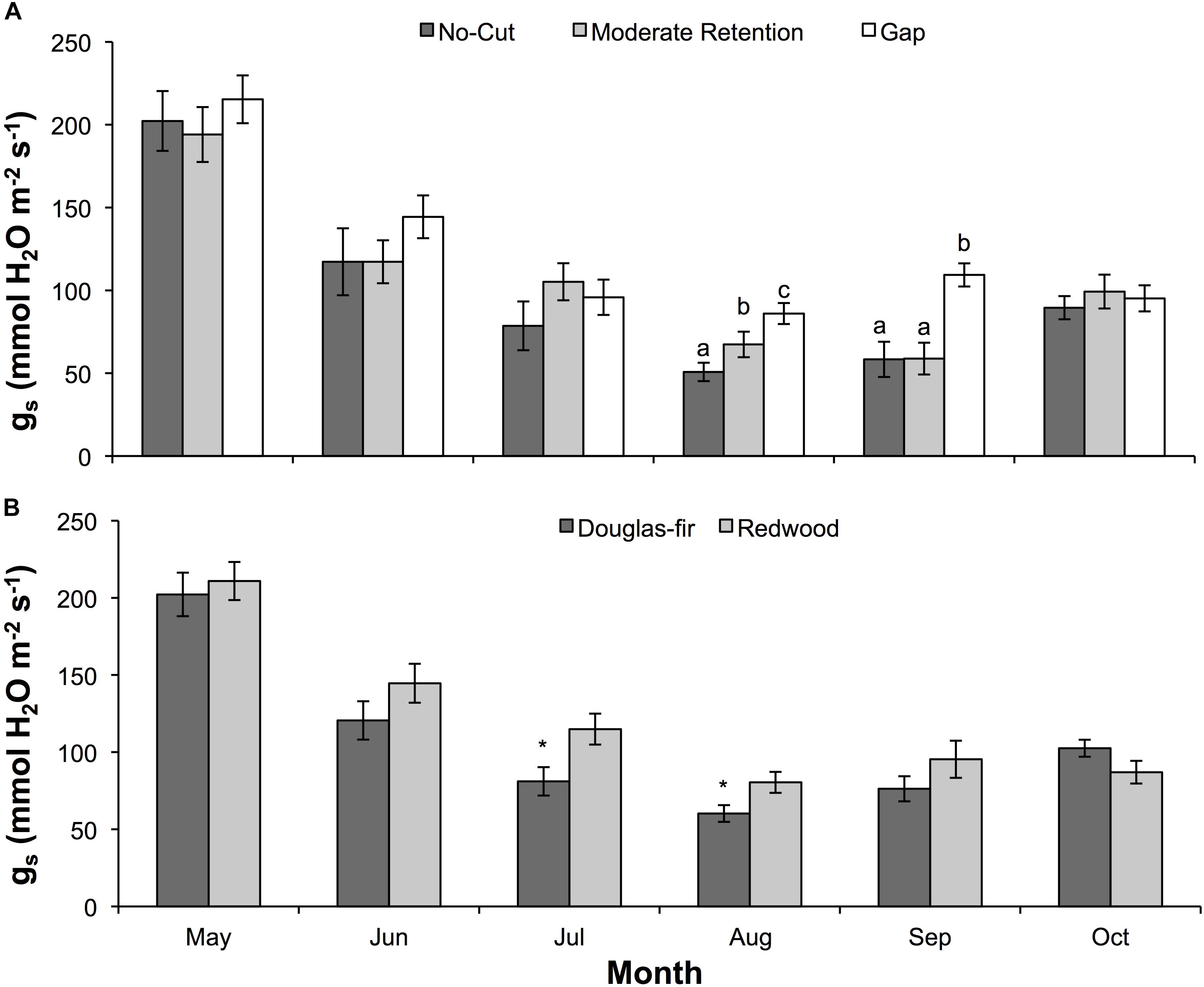
Figure 2. Mean (±SE) stomatal conductance of water vapor (gs) during the 2016 (second) growing season for Douglas-fir and redwood seedlings planted after partial harvesting in Maple Creek, CA. (A) Stomatal conductance among treatments for both species pooled, and (B) stomatal conductance between species for all treatments pooled. In panel (A), within a month, treatments not sharing the same letter are significantly different; in panel (B), asterisks represent a significant difference between species.
Seedling growth and mortality between April 2015 and October 2016 varied among treatments and between species. Seedling growth was evaluated in two ways: as the percent increase in basal diameter (Figure 3A) and as aboveground biomass accumulation relative to total biomass accumulation (Figure 3B). Both treatment (F = 23.2, df = 2, p < 0.0001) and species (F = 4.4, df = 1, p = 0.04) were significant effects on basal diameter growth. For Douglas-fir, diameter growth did not differ among treatments (F = 1.8, df = 26, p = 0.19), while for redwood, diameter growth was significantly greatest in the gap treatment (F = 23.3, df = 28, p < 0.0001). In the no-cut treatment, diameter growth was greater in Douglas-fir than in redwood (F = 13.4, df = 12, p = 0.004) and in the gap treatment, diameter growth was greater in redwood than in Douglas-fir (X2 = 11.5, df = 1, p = 0.001). Similar to these growth trends, treatment (F = 38.7, df = 2, p < 0.0001) and species (F = 6.4, df = 1, p = 0.014) both influenced aboveground biomass accumulation. The percent of aboveground biomass accumulation (relative to total biomass accumulation) was greatest in the gap treatment for Douglas-fir (X2 = 6.1, df = 2, p = 0.047) and redwood (X2 = 21.6, df = 2, p < 0.0001). In the gap treatment, aboveground biomass accumulation was greater in redwood compared to Douglas-fir (X2 = 11.9, df = 1, p = 0.001). Overall, 32 of the 90 planted seedlings (36%) died (Figure 3C). By species, 18 of the 45 Douglas-fir seedlings died (40%), and 14 of the 45 redwood seedlings died (31%). For both species, seedling mortality was greatest in the no-cut treatment and lowest in the gap treatment. Douglas-fir had greater mortality than redwood in the moderate-retention and no-cut treatments. Because we only had one plot for each treatment, there are no statistics to report for mortality, just raw counts. To determine if mortality was possibly due to drought, we compared ΨPD in August 2015 (the month with the lowest Ψ values during the first growing season) between seedlings that survived the 2-year study and seedlings that died during the study; surviving seedlings had higher ΨPD (−1.24 ± 0.18 MPa) than seedlings that died (−2.20 ± 0.16 MPa; X2 = 14.8, df = 1, p = 0.0001).
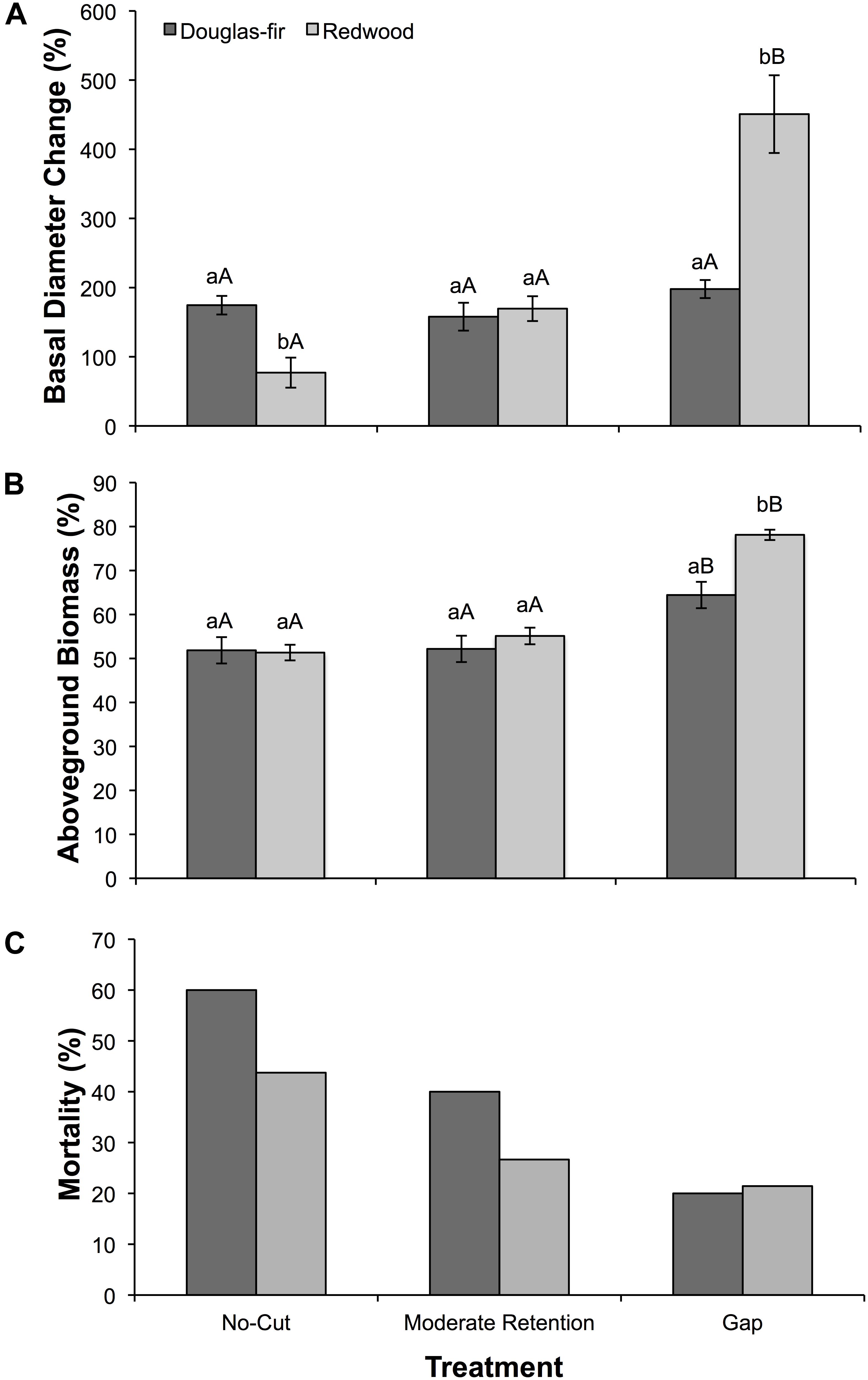
Figure 3. (A) Mean (±SE) change in basal diameter as a percent of initial basal diameter, (B) mean (±SE) aboveground biomass accumulation as a percent of total biomass (above- plus belowground), and (C) total mortality as a percent of initial cohort after 2 years of growth for Douglas-fir and redwood seedlings planted after partial harvesting in Maple Creek, CA. Seedlings were planted (15 seedlings per species per treatment) in early spring 2015 and were harvested, including root systems, in November 2016 for biomass quantification. The mortality percentages shown here are cumulative for 2015 and 2016. Within a treatment, species not sharing the same lowercase letters are significantly different; within a species, treatments not sharing the same uppercase letters are significantly different.
Discussion
To answer this study’s research questions, our findings indicate that (1) seedlings in the gap treatment had the most vigorous physiology (Ψ and gs) and growth and showed the lowest mortality, (2) redwood seedlings had more vigorous physiology and growth and lower mortality compared to Douglas-fir seedlings, and (3) Ψ was lowest in July/August/September and gs was lowest in August. While it is well known that gaps are important for forest regeneration (Gray et al., 2002; Blair et al., 2010; York et al., 2011; O’Hara et al., 2017), this demonstration that gap-grown seedlings have high survivability despite increased amounts of direct solar radiation and soil water evaporative losses, even during drought, is encouraging for future forest regeneration and sustainability, as water stress can be a common cause of seedling mortality (McDowell et al., 2008; Plamboeck et al., 2008). Further, our demonstration that redwood seedlings can successfully establish at a relatively hot, dry site outside of their native range is also encouraging for forest regeneration and assisted migration efforts. Our documentation of Ψ and gs decreasing across the growing season to reach a seasonal low around August is likely driven by the depletion of winter water inputs and increasing temperatures in this Mediterranean climate. These findings collectively suggest that forest regeneration following drought-induced mortality and timber harvests has the potential to be successful, even under hot, dry conditions. Related to land management, these results show that reductions in stand density by thinning or patch cutting to create small gaps can improve seedling establishment and forest regeneration during drought.
The 2 years of this study, 2015 and 2016, were both dry, with PDSI values of −2.7 and −1.62, respectively. This drought was the most severe in both observational and reconstructed records (Griffin and Anchukaitis, 2014; Swain et al., 2014; Allen et al., 2015; Diffenbaugh et al., 2015; Williams et al., 2015), constituting a drought emergency in the State of California (California Department of Water Resources, United States, Drought Monitor). Thus, the high amount of observed mortality (36% of all planted seedlings) could be in part due to water stress associated with these severe drought conditions, particularly as seedlings that did not survive the 2-year study had lower ΨPD values in the first year compared to surviving seedlings. In the gap treatment, there developed a remarkable amount of understory vegetation. While it would seem this vegetation would inhibit conifer seedlings via increased competition, seedlings in this treatment had the greatest success by all measures (Ψ, gs, growth, biomass accumulation, and mortality rate). A previous study at this site (Berrill et al., 2018) also did not detect negative effects of understory vegetation cover on seedling performance. As plants will often allocate more resources to root production when belowground resources are limiting (Kerr et al., 2015; Hansen and Turner, 2019), the fact that gap seedlings allocated proportionally less biomass to belowground structures compared to seedlings in other treatments further suggests that understory vegetation was not likely a significant source of competition.
It is possible that the success of gap seedlings during drought could be due to positive interactions between seedlings and understory vegetation under high levels of environmental stress (Gómez-Aparicio et al., 2004). In forest ecosystems around the globe, this type of facilitation has been fairly well documented (Bertness and Callaway, 1994). In Montana, ponderosa pine (Pinus ponderosa Douglas ex Lawson and C. Lawson) seedlings appeared to facilitate Douglas-fir seedling success under dry conditions (Fajardo et al., 2006). In Argentina, medium cover of understory did not negatively impact Douglas-fir regeneration (Caccia and Ballaré, 1998). In Spain, Spanish juniper (Juniperus thurifera L.) facilitated holm oak [Quercus ilex L. subsp. Ballota (Desf.) Samp.] establishment under dry conditions (Gimeno et al., 2015). It is likely that partial shade cast by understory plants at our study site reduced soil temperature and soil water evaporation (Wang et al., 2011), thereby improving seedling water availability and resulting vigor. Our finding of superior seedling performance in the gap treatment also could indicate that in this system, increased evapotranspirational water loss in heavily thinned stands may be less than competitive stress from densely spaced residual trees in less aggressive treatments.
The differences in performance between Douglas-fir, a species native to this site, and redwood, a species not naturally occurring at this site, were pronounced and interesting. The Ψ values experienced in our study were on par with moderate to severe drought Ψ values for Douglas-fir (Khan et al., 1996) and redwood (Ambrose et al., 2015). Redwood generally had higher Ψ, gs, basal diameter growth, aboveground biomass allocation, and survivorship compared to Douglas-fir. This was surprising, as Douglas-fir is native to this site and redwood is not. These inter-specific differences in responses to drought and management likely relate to functional characteristics such as drought tolerance, shade tolerance, and growth rate. Generally, water stress reduces trees’ hydraulic function due to excessive xylem cavitation, carbon uptake due to excessive stomatal regulation to conserve water, and capacity to translocate photosynthate in the phloem tissue (McDowell et al., 2008; Choat et al., 2012; Sevanto et al., 2014). Ultimately, these stressors can decrease tree growth rates and resistance to pathogens (Stephenson et al., 2019). Compared to redwood, reduced Ψ in Douglas-fir likely limited growth in this species, as Douglas-fir stops photosynthesizing at xylem water potential values of −2 MPa (Newton and Preest, 1988) and redwood maintains photosynthesis under much lower Ψ values (Ambrose et al., 2015). This relatively isohydric stomatal regulation in Douglas-fir is surprising, as its xylem anatomy with small lumen diameters suggests that stems should be relatively resistant to cavitation (Miller and Johnson, 2017). While the higher Ψ measured in redwood compared to Douglas-fir could possibly be explained by greater stomatal regulation to conserve water, we measured greater gs in redwood than in Douglas-fir. Further, stomatal regulation in redwood is relatively anisohydric, maintaining gs even under very low Ψ values (Ambrose et al., 2015). Thus, the combination of higher Ψ and higher gs in redwood compared to Douglas-fir suggests that the former somehow had access to more water than the latter. We speculate that perhaps redwood more quickly acquired mycorrhizal symbionts such that it was better able to mine soil for water and nutrients to support greater vigor (Plamboeck et al., 2007).
Following similar trends as measured in seedling physiology, growth and survival were also lower in Douglas-fir compared to redwood. Although another study at this site found that Douglas-fir seedlings were taller than redwood seedlings and that the two species had comparable basal diameters, this study did not consider initial seedling size metrics (Berrill et al., 2018). Our evaluation of seedling growth relative to measured starting dimensions provides a more accurate assessment of growth. The lack of difference in Douglas-fir basal diameter growth among treatments could indicate that the gap treatment was too shaded due to the northern aspect and shading from adjacent treatments to support variable growth responses in this shade-intolerant species that exhibits some tolerance to shade when young (Caccia and Ballaré, 1998; Stuart and Sawyer, 2001). Our light measurements confirm that although light availability was greatest in the gap treatment, overall levels were still relatively low (PPFD <1000 μmol m–2 s–1). The fact that we measured reduced basal diameter growth, reduced proportional allocation of biomass aboveground, and greater water stress in Douglas-fir compared to redwood makes sense, as the former likely invested more resources into root production to increase water uptake capacity, given its consistently lower Ψ values. Additionally, although we observed almost no evidence of animal browse on seedlings, it is possible that undetected animal browse may have reduced aboveground seedling biomass; preferential browsing could have contributed to the reduced aboveground biomass allocation measured in Douglas-fir. While our measured 64% seedling survivorship is low, we note that this establishment success rate occurred during a notably severe drought (Asner et al., 2016) and that under more typical conditions, the success rate would likely be higher. As such, this type of partial harvesting likely represents an economically viable combination of treatments and species for forest regeneration in this region (Fleming et al., 1998; Talbert and Marshall, 2005).
There are two noteworthy limitations with this small study. First, the small treatment plot size (0.04 ha) and the immediate adjacency of plots make it difficult to isolate specific treatment effects, as neighboring plots were surely influencing one another. The relatively low light levels measured in the gap treatment likely provide confirmation of this confounding issue, although these low light levels in the gap could also be due to the site’s northern aspect. We highlight that due to the close proximity of treatments, there were almost assuredly interactions (e.g., shading and competition for water and nutrients) between seedlings and neighbor trees in other treatments; this was a design feature of the partial harvesting study to test spatial variability of tree spacing at small spatial scales. Future studies with larger plots and greater inter-plot spacing are needed to more effectively assess seedling responses to different levels of thinning and patterns of overstory tree retention. Nevertheless, we feel that this study provides important preliminary findings about the dynamic relationships between partial harvesting silvicultural prescriptions and the regenerating cohort. Second, the north-facing slope of this study may have dampened stressors (e.g., increased solar radiation and evapotranspiration) typical of open stands. As such, if this study were expanded to include a south-facing slope, our findings may have differed. We therefore note that these findings are specific to this location, these treatments, and these species, and that due to the lack of treatment replication in this study and treatment proximity, these findings may not hold true if extended to larger scales or other ecosystems. These inherent limitations in study design and statistical analysis need to be considered when evaluating our findings.
In summary, findings from this study show that the removal of competitor trees to create small openings (20 × 20 m) creates an environment conducive to the successful establishment of planted seedlings, even during drought. Our results also demonstrate the potential to successfully establish species threatened by climate change in locations outside of their current range. More broadly, this work highlights the efficacy of active management to foster forest persistence in the face of warming and drying climate. Our combined measurements of physiological performance, morphological performance, and mortality to better understand seedling establishment success during drought provide valuable information for future reforestation plans in western North America and in Mediterranean climates and other drought-prone locations worldwide. Globally, widespread drought-induced forest mortality (Allen et al., 2010, 2015) reduces carbon sequestration and timber production and increases the likelihood of catastrophic wildland fires (Jolly et al., 2015; Abatzoglou and Williams, 2016; Hessburg et al., 2019). In a climate predicted to continue warming and drying for decades to come (Mastrandrea et al., 2011), the successful establishment and regeneration of forests is not guaranteed (Hansen and Turner, 2019). Through a combined evaluation of physiology, growth, and mortality, this study demonstrates that active forest management can increase seedling establishment success during drought, and that tree species originating from elsewhere can be less water-stressed and outperform locally-adapted species.
Data Availability Statement
The datasets generated for this study are available on request to the corresponding author.
Author Contributions
LK designed the study, in collaboration on a larger study with J-PB. LK, WP, and NK contributed to fieldwork and data analysis. All authors contributed to writing.
Funding
This research was supported by the McIntire-Stennis Cooperative Forestry Research Program and the L.W. Schatz Demonstration Tree Farm.
Conflict of Interest
The authors declare that the research was conducted in the absence of any commercial or financial relationships that could be construed as a potential conflict of interest.
Acknowledgments
We thank the Humboldt State University L.W. Schatz Demonstration Tree Farm manager Gordon Schatz, Christa Dagley, and Humboldt State University students enrolled in numerous forestry classes for help with study site selection, silvicultural treatment implementation, and seedling planting. We are grateful to the Green Diamond Resource Company for supplying conifer seedlings. We thank Gabriel Goff and Nicholas Kilgore for help with field-based physiology measurements, seedling harvest, and seedling dissection into above- and belowground components. We also thank three reviewers and editor for providing helpful comments on the manuscript.
Footnotes
References
Abatzoglou, J. T., and Williams, A. P. (2016). Impact of anthropogenic climate change on wildfire across western US forests. Proc. Natl. Acad. Sci. U.S.A. 113, 11770–11775. doi: 10.1007/s10584-011-0148-z
Allen, C. D., Breshears, D. D., and McDowell, N. G. (2015). On underestimation of global vulnerability to tree mortality and forest die-off from hotter drought in the Anthropocene. Ecosphere 6, 1–55.
Allen, C. D., Macalady, A. K., Chenchouni, H., Bachelet, D., McDowell, N., Vennetier, M., et al. (2010). A global overview of drought and heat-induced tree mortality reveals emerging climate change risks for forests. For. Ecol. Manag. 259, 660–684. doi: 10.1016/j.foreco.2009.09.001
Ambrose, A. R., Baxter, W. L., Wong, C. S., Naesborg, R. R., Williams, C. B., and Dawson, T. E. (2015). Contrasting drought-response strategies in California redwoods. Tree Physiol. 35, 453–469. doi: 10.1046/j.1365-3040.1998.00342.x
Asner, G. P., Brodrick, P. G., Anderson, C. B., Vaughn, N., Knapp, D. E., and Martin, R. E. (2016). Progressive forest canopy water loss during the 2012–2015 California drought. Proc. Natl. Acad. Sci. U.S.A. 113, e249–e255. doi: 10.1016/j.rse.2008.10.018
Berrill, A. J.-P., Dagley, C. M., Gorman, A. J., Obeidy, C. S., Powell, H. K., and Wright, J. C. (2018). Variable-density retention promotes spatial heterogeneity and structural complexity in a Douglas-fir/tanoak stand. Curr. Trends For. Res. 2018, 1–9. doi: 10.29011/CTFR-108
Berrill, J.-P., and O’Hara, K. L. (2007). “Modeling coast redwood variable retention management regimes,” in Proceedings of the Redwood Region Forest Science Symposium: What does the future hold? March 15-17, 2004, Rohnert Park, California, eds R. B. Standiford, G. A. Giusti, Y. Valachovic, W. J. Zielinkski, and M. J. Furniss (Albany, CA: USDA), 261–269.
Bertness, M. D., and Callaway, R. M. (1994). Positive interactions in communities. Trends Ecol. Evol. 9, 187–191.
Blair, B. C., Letourneau, D. K., Bothwell, S. G., and Hayes, G. F. (2010). Disturbance, resources, and exotic plant invasion: gap size effects in a redwood forest. Madroño 57, 11–19. doi: 10.3120/0024-9637-57.1.11
Byer, S., and Jin, Y. (2017). Detecting drought-induced tree mortality in Sierra Nevada forests with time series of satellite data. Remote Sens. 9, 929–952. doi: 10.1641/B580607
Caccia, F. D., and Ballaré, C. L. (1998). Effects of tree cover, understory vegetation, and litter on regeneration of Douglas-fir (Pseudotsuga menziesii) in southwestern Argentina. Can. J. For. Res. 28, 683–692. doi: 10.1139/cjfr-28-5-683
Choat, B., Jansen, S., Brodribb, T. J., Cochard, H., Delzon, S., Bhaskar, R., et al. (2012). Global convergence in the vulnerability of forests to drought. Nature 491, 752–755. doi: 10.1038/nature11688
Dagley, C. M., Berrill, J.-P., Leonard, L. P., and Kim, Y. G. (2018). Restoration thinning enhances growth and diversity in mixed redwood/Douglas-fir stands in northern California, U.S.A. Restorat. Ecol. 26, 1170–1179. doi: 10.1139/x01-085
Diffenbaugh, N. S., Swain, D. L., and Touma, D. (2015). Anthropogenic warming has increased drought risk in California. Proc. Natl. Acad. Sci. U.S.A. 112, 3931–3936. doi: 10.1073/pnas.1422385112/-/DCSupplemental/pnas.201422385SI.pdf
Fajardo, A., Goodburn, J. M., and Graham, J. (2006). Spatial patterns of regeneration in managed uneven-aged ponderosa pine/Douglas-fir forests of Western Montana, USA. For. Ecol. Manag. 223, 255–266. doi: 10.1016/j.foreco.2005.11.022
Fettig, C. J., Mortenson, L. A., Bulaon, B. M., and Foulk, P. B. (2019). Tree mortality following drought in the central and southern Sierra Nevada, California, U.S. For. Ecol. Manag. 432, 164–178. doi: 10.1016/j.foreco.2018.09.006
Fleming, R. L., Black, T. A., Adams, R. S., and Slathers, R. J. (1998). Silvicultural treatments, microclimatic conditions and seedling response in Southern Interior clearcuts. Can. J. Soil Sci. 78, 115–126. doi: 10.4141/s97-042
Gimeno, T. E., Escudero, A., and Valladares, F. (2015). Different intra- and interspecific facilitation mechanisms between two Mediterranean trees under a climate change scenario. Oecologia 177, 159–169. doi: 10.1007/s00442-014-3115-3
Gómez-Aparicio, L., Zamora, R., Gómez, J. M., Hódar, J. A., Castro, J., and Baraza, E. (2004). Applying plant facilitation to forest restoration: a meta-analysis of the use of shrubs as nurse plants. Ecol. Appl. 14, 1128–1138. doi: 10.1890/03-5084
Gray, A. N., Spies, T. A., and Easter, M. J. (2002). Microclimatic and soil moisture responses to gap formation in coastal Douglas-fir forests. Can. J. For. Res. 32, 332–343. doi: 10.1029/JZ069i004p00615
Griffin, D., and Anchukaitis, K. J. (2014). How unusual is the 2012-2014 California drought? Geophys. Res. Lett. 41, 9017–9023.
Hansen, W. D., and Turner, M. G. (2019). Origins of abrupt change? Postfire subalpine conifer regeneration declines nonlinearly with warming and drying. Ecol. Monogr. 32:772. doi: 10.1038/nclimate1693
Hessburg, P. F., Miller, C. L., Parks, S. A., Povak, N. A., Taylor, A. H., Higuera, P. E., et al. (2019). Climate, environment, and disturbance history govern resilience of western North American forests. Front. Ecol. Evol. 7:239. doi: 10.3389/fevo.2019.00239
Jia, G., Shevliakova, E., Artaxo, P., De Noblet-Ducoudré, N., Houghton, R., House, J., et al. (2019). “Land–climate interactions,” in Climate Change and Land: An IPCC Special Report on Climate Change, Desertification, Land Degradation, Sustainable Land Management, Food Security, and Greenhouse Gas Fluxes in Terrestrial Ecosystems, eds P. R. Shukla, J. Skea, E. Calvo Buendia, V. Masson-Delmotte, H.-O. Pörtner, D. C. Roberts, et al. (Geneva: IPCC).
Jolly, W. M., Cochrane, M. A., Freeborn, P. H., Holden, Z. A., Brown, T. J., Williamson, G. J., et al. (2015). Climate-induced variations in global wildfire danger from 1979 to 2013. Nat. Commun. 6, 7537–7548. doi: 10.1038/ncomms8537
Keenan, R. J. (2015). Climate change impacts and adaptation in forest management: a review. Ann. For. Sci. 72, 145–167. doi: 10.1073/pnas.0901643106
Kerr, K. L., Meinzer, F. C., McCulloh, K. A., Woodruff, D. R., and Marias, D. E. (2015). Expression of functional traits during seedling establishment in two populations of Pinus ponderosa from contrasting climates. Tree Physiol. 35, 535–548. doi: 10.1093/treephys/17.7.461
Khan, S. R., Rose, R., Haase, D. L., and Sabin, T. E. (1996). Soil water stress: its effects on phenology, physiology, and morphology of containerized Douglas-fir seedlings. New For. 12, 19–39.
Mastrandrea, M. D., Tebaldi, C., Snyder, C. W., and Schneider, S. H. (2011). Current and future impacts of extreme events in California. Clim. Change 109, 43–70. doi: 10.1175/JCLI3366.1
McDowell, N., Pockman, W., Allen, C., Breshears, D., Cobb, N., Kolb, T., et al. (2008). Mechanisms of plant survival and mortality during drought: why do some plants survive while others succumb to drought? New Phytol. 178, 719–739. doi: 10.1111/j.1469-8137.2008.02436.x
Millar, C. J., and Stephenson, N. L. (2015). Temperate forest health in an era of emerging megadisturbance. Science 349, 823–826. doi: 10.1126/science.aaa9933
Miller, M. L., and Johnson, D. M. (2017). Vascular development in very young conifer seedlings: theoretical hydraulic capacities and potential resistance to embolism. Am. J. Bot. 104, 979–992. doi: 10.3732/ajb.1700161
Newton, M., and Preest, D. S. (1988). Growth and water relations of Douglas fir (Pseudotsuga menziesii) seedlings under different weed control regimes. Weed Sci. 36, 653–662.
O’Hara, K., Cox, L., Nikolaeva, S., Bauer, J., and Hedges, R. (2017). Regeneration dynamics of coast redwood, a sprouting conifer species: a review with implications for management and restoration. Forests 8, 144–163. doi: 10.3732/ajb.1600110
O’Hara, K. L., Nesmith, J. C. B., Leonard, L., and Porter, D. J. (2010). Restoration of old forest features in coast redwood forests using early-stage variable-density thinning. Restorat. Ecol. 18, 125–135. doi: 10.1111/j.1526-100X.2010.00655.x
Plamboeck, A. H., Dawson, T. E., Egerton-Warburton, L. M., North, M., Bruns, T. D., and Querejeta, J. I. (2007). Water transfer via ectomycorrhizal fungal hyphae to conifer seedlings. Mycorrhiza 17, 439–447. doi: 10.1016/B978-0-12-372180-8.50042-1
Plamboeck, A. H., North, M., and Dawson, T. E. (2008). Conifer seedling survival under closed-canopy and manzanita patches in the Sierra Nevada. Madrono 55, 191–201. doi: 10.3120/0024-9637-55.3.191
Ritchie, G. A., and Shula, R. G. (1984). Seasonal changes of tissue-water relations in shoots and root systems of Douglas-fir seedlings. For. Sci. 30, 538–548.
Ryan, K. C., Knapp, E. E., and Varner, J. M. (2013). Prescribed fire in North American forests and woodlands: history, current practice, and challenges. Front. Ecol. Environ. 11, e15–e24. doi: 10.1890/120329
Sevanto, S., Mcdowell, N. G., Dickman, L. T., Pangle, R., and Pockman, W. T. (2014). How do trees die? A test of the hydraulic failure and carbon starvation hypotheses. Plant Cell Environ. 37, 153–161. doi: 10.1111/pce.12141
Simonin, K., Kolb, T., Montes-Helu, M., and Koch, G. (2007). The influence of thinning on components of stand water balance in a ponderosa pine forest stand during and after extreme drought. Agric. For. Meteorol. 143, 266–276.
Skov, K., Kolb, T., and Wallin, K. (2004). Tree size and drought affect ponderosa pine physiological response to thinning and burning treatments. For. Sci. 50, 81–91.
Sohn, J. A., Saha, S., and Bauhus, J. (2016). Potential of forest thinning to mitigate drought stress: a meta-analysis. For. Ecol. Manag. 380, 261–273. doi: 10.1016/j.foreco.2016.07.046
Soil Survey Staff (2017). Natural Resources Conservation Service, United States Department of Agriculture. Available online at: https://websoilsurvey.sc.egov.esda.gov/. (accessed October 23, 2017).
Stephenson, N. L., Das, A. J., Ampersee, N. J., Bulaon, B. M., and Yee, J. L. (2019). Which trees die during drought? The key role of insect host-tree selection. J. Ecol. 146, 271–290. doi: 10.1371/journal.pone.0169770
Stuart, J. D., and Sawyer, J. O. (2001). Trees and Shrubs of California. Berkeley, CA: University of California Press.
Swain, D. L., Tsiang, M., Matz, H., Singh, D., Charland, A., Rajaratnam, B., et al. (2014). The extraordinary California drought of 2013/2014: character, context, and the role of climate change. Bull. Am. Meteorol. Soc. 95, S3–S20.
Talbert, C., and Marshall, D. (2005). Plantation producitivity in the Douglas-fir region under intensive silvicultural practices: results from research and operations. J. Forest. 103, 65–70.
Tyree, M., and Sperry, J. (1989). Vulnerability of xylem to cavitation and embolism. Annu. Rev. Plant Physiol. Plant Mol. Biol. 40, 19–38.
Wang, X., Zhao, J., Wu, J., Chen, H., Lin, Y., Zhou, L., et al. (2011). Impacts of understory species removal and/or addition on soil respiration in a mixed forest plantation with native species in southern China. For. Ecol. Manag. 261, 1053–1060. doi: 10.1016/j.foreco.2010.12.027
Warren, C., Livingston, N., and Turpin, D. (2004). Water stress decreases the transfer conductance of Douglas-fir (Pseudotsuga menziesii) seedlings. Tree Physiol. 24, 971–979.
Williams, A. P., Seager, R., Abatzoglou, J., Cook, B., Smerdon, J. E., and Cook, E. R. (2015). Contribution of anthropogenic warming to California drought during 2012–2014. Geophys. Res. Lett. 42, 6819–6828. doi: 10.1002/(ISSN)1944-8007
York, R. A., Battles, J. J., Eschtruth, A. K., and Schurr, F. G. (2011). Giant sequoia (Sequoiadendron giganteum) regeneration in experimental canopy gaps. Restorat. Ecol. 19, 14–23. doi: 10.1139/x03-222
Keywords: biomass, carbon allocation, forest restoration, silviculture, stomatal conductance, tree mortality, variable retention, water potential
Citation: Kerhoulas L, Polda W, Kerhoulas N and Berrill J-P (2020) Physiology and Growth of Douglas-Fir and Redwood Seedlings Planted After Partial Harvesting. Front. For. Glob. Change 3:49. doi: 10.3389/ffgc.2020.00049
Received: 28 December 2019; Accepted: 06 April 2020;
Published: 30 April 2020.
Edited by:
Teresa E. Gimeno, Basque Centre for Climate Change, SpainReviewed by:
John T. Van Stan, Georgia Southern University, United StatesEnrique Andivia, Complutense University of Madrid, Spain
Callie Ashton Oldfield, University of Georgia, United States
Copyright © 2020 Kerhoulas, Polda, Kerhoulas and Berrill. This is an open-access article distributed under the terms of the Creative Commons Attribution License (CC BY). The use, distribution or reproduction in other forums is permitted, provided the original author(s) and the copyright owner(s) are credited and that the original publication in this journal is cited, in accordance with accepted academic practice. No use, distribution or reproduction is permitted which does not comply with these terms.
*Correspondence: Lucy Kerhoulas, bHVjeS5rZXJob3VsYXNAaHVtYm9sZHQuZWR1