- 1Hawkesbury Institute for The Environment, Western Sydney University, Richmond, NSW, Australia
- 2Université Clermont-Auvergne, INRA, PIAF, Clermont-Ferrand, France
- 3Urban Studies, School of Social Science, Western Sydney University, Parramatta, NSW, Australia
Elevated atmospheric carbon dioxide (eCO2) often enhances rates of photosynthesis leading to increased productivity in trees. In their native habitats in Australia, eucalypts display considerable phenotypic plasticity in response to changes in environmental conditions. Little is known whether this plasticity can be harnessed effectively under future atmospheric eCO2 conditions and be used to identify provenances with superior growth. Here, we report two experiments that assessed the physiological and growth responses of Eucalyptus grandis—one of the world's most important hardwood plantation species—to eCO2. We used 11 provenances from contrasting climates. Our selection was based on site-specific information of long-term temperature and water availability. In Experiment 1, four provenances exhibited significant variation in light-saturated photosynthetic rates (Asat), stomatal conductance (gs), and concentrations of non-structural carbohydrates in leaves, stems and roots when grown under ambient CO2 (aCO2). Biomass of leaves, stems and roots varied significantly and were negatively correlated with mean annual temperature (MAT) at seed origin, indicating that provenances from cooler, wetter climates generally produced greater biomass. Yet, stem growth of these provenances was not stimulated by eCO2. Given the vast environmental gradient covered by provenances of E. grandis, we expanded the selection from four to nine provenances in Experiment 2. This allowed us to validate results from Experiment 1 with its small selection and detailed measurements of various physiological parameters by focusing on growth responses to eCO2 across a wider environmental gradient in Experiment 2. In Experiment 2, nine provenances also exhibited intraspecific differences in growth, but these were not related to climate of origin, and eCO2 had little effect on growth traits. Growth responses under eCO2 varied widely across provenances in both experiments, confirming phenotypic plasticity in E. grandis, though responses were not systematically correlated with climate of origin. These results indicate that selection of provenances for improved stem growth of E. grandis under future eCO2 cannot be based solely on climate of origin, as is common practice for other planted tree species.
Introduction
Elevated atmospheric CO2 (eCO2) may affect tree growth by increasing photosynthesis (Way et al., 2015). Empirical and theoretical work for a range of eucalypt species has shown that eCO2 will increase biomass production if access to water and nutrients is not limited (e.g., Wong et al., 1992; Ghannoum et al., 2010; Battaglia and Bruce, 2017; Ellsworth et al., 2017). This response is commonly observed due to the expansion of leaf area (LA), improved photosynthesis (A) and water use efficiency (WUE) under eCO2 (McCarthy et al., 2007; Drake et al., 2011; Norby and Zak, 2011; Resco de Dios et al., 2016). However, it is also well-known that genotypes and provenances of a single tree species may differ in their response to eCO2 (Aspinwall et al., 2015). High genetic variability across (and within) populations may result in intraspecific differences of phenotypic responses to environmental cues, commonly termed “genotype-by-environment interaction,” or G × E (Matheson and Raymond, 1986; Josephs, 2018). This intraspecific variation may be due to heritability of specific trait characteristics and due to phenotypic plasticity (Moran et al., 2016).
For decades tree breeding programs have been used to identify species and provenances that display fast stem growth (e.g., Melville, 1940; Lavigne, 1996; Pan et al., 2020) and increase productivity of commercial plantations (e.g., Cornelius, 1994; Erskine et al., 2006; Calderia et al., 2020). However, provenances selected for high rates of stem growth today may not necessarily display these favorable traits under future environmental conditions. Higher rates of growth under eCO2 can lead to higher respiratory C loss (Dusenge et al., 2019) and metabolic activity due to higher rates of photosynthetic C assimilation and subsequent increases in non-structural carbohydrates in leaves (e.g., Moore et al., 1999; Poorter et al., 2009; Smith et al., 2012; Schmidt et al., 2017). Under such conditions, the carbon use efficiency (CUE, ratio of net primary production to gross primary production, sensu Gifford, 2003) may result in zero growth stimulation under eCO2. If CUE is improved under eCO2, carbon allocation patterns may change such that increased rates of C assimilation stimulate growth of roots rather than stems (Finzi et al., 2007; De Kauwe et al., 2014; Zaehle et al., 2014), which would be undesirable for the timber growing industry, yet potentially improve resilience during dry conditions (Grote et al., 2016). However, identifying provenances that show net-positive stem growth under eCO2 is critical to meeting a rising global demand for wood and wood products (Wang et al., 2010; Booth et al., 2015; Loik et al., 2017).
Nine Eucalyptus species dominate hardwood plantations around the world due to their fast growth under a wide range of environmental conditions (Harwood, 2011; Müller da Silva et al., 2019), providing considerable capacity as sinks for atmospheric C (e.g., Binkley et al., 2017; Viera and Rodríguez-Saolliero, 2019). One of the most important eucalypt species used in plantations is E. grandis Hill ex Maid (comm. Flooded Gum or Rose Gum). Across its natural habitat this tall, fast-growing species displays considerable plasticity in tolerating a range of climates, from temperate to tropical along the coast of eastern Australia (Figure 1). Mean annual temperature (MAT) can vary from 12 to 25°C and mean annual precipitation (MAP) from 750 to 3,500 mm across its distributional range (Aspinwall et al., 2017). At least 40 seed provenances are listed for E. grandis by the Australian Tree Seed Centre and progeny trials have been operated for some time to identify seed sources of E. grandis and other eucalypt species that produce trees with favorable timber properties (Bamber and Humphreys, 1963; Wang et al., 1984; Booth, 2013).
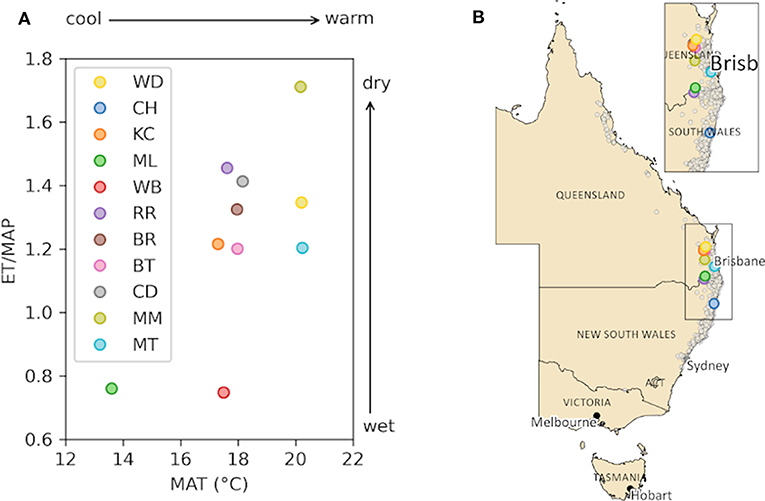
Figure 1. Climate parameters and location for the 11 selected E. grandis sites (each provenance with a unique color). (A) Relationship between evapotranspiration/mean annual precipitation (ET/MAP) and mean annual air temperature (MAT). (B) Records of E. grandis from Atlass of Living Australia (2020) (light gray dots) and provenances location on a map of Eastern Australia (area of interested enlarged in inlet). Note that higher ET/MAP signifies greater water limitation.
High levels of plasticity in E. grandis have been demonstrated in response to nutrient addition, and water deficit (Grassi et al., 2002; Battie-Laclau et al., 2013, 2014). Drake et al. (2015) demonstrated high growth plasticity in E. grandis in response to increasing temperatures. Another recent study showed that eCO2 increased leaf dry mass per unit area, but not respiration, in 15 provenances of E. grandis (Aspinwall et al., 2017). Yet, these studies did not report how stem growth was affected by eCO2. In absence of this information, valuable insight can be drawn from another study that used a widely distributed eucalypt species. Eucalyptus camaldulensis was exposed to eCO2, which stimulated biomass production, although stem biomass increased the least and root biomass the most (Aspinwall et al., 2018). Moreover, the study found no clear trend indicating that the positive growth response to eCO2 was related to climate of origin of the different provenances tested.
Here we report two experiments that assessed phenotypic plasticity in response to growth under eCO2 of E. grandis provenances originating from different climates. Our selection was informed by results from Drake et al. (2015) who showed that productivity of E. grandis provenances generally increased from cool to warm temperate climates when grown under aCO2. Based on this observation, we tested if superior growth performance in provenances from warmer climates would persist under eCO2, using two individual experiments. In Experiment 1, we examined the response to eCO2 for a range of physiological and growth traits in four provenances of E. grandis. The provenances originated from the southern distribution cluster of the species (Figure 1) and varied in climate of origin (precipitation and temperature). Given the vast environmental gradient covered by provenances of E. grandis, we expanded the geographical range of provenances in Experiment 2, where we used nine provenances to exclusively assess the effect of eCO2 on growth traits. Two provenances of Experiment 1 were included in Experiment 2 to allow cross-validation of results in both experiments and their relevance across wider environmental gradients.
Materials and Methods
This study reports two experiments on Eucalyptus grandis seedlings, grown in pots in a glasshouse, originating from a variety of climates at seed origin. Experiment 1 included four provenances (four climates) with five individuals per provenance in order to examine the intraspecific response of leaf physiology traits, non-structural carbohydrates and growth traits to aCO2 and eCO2. Following observation of intraspecific variation, we extended the selection to nine provenances in Experiment 2 to assess phenotypic plasticity in growth enhancement and correlations with climate of origin using 104 seedlings. Experiment 2 allowed validation of results from Experiment 1 by focusing on growth responses to eCO2 across wider environmental gradients compared to more detailed physiological measurements conducted in Experiment 1. For both experiments, seed from E. grandis mother trees were collected at the 11 locations by personnel of the Australian Tree Seed Centre (Commonwealth Scientific and Industrial Research Organization, Government of Australia).
Experiment 1
Plant Material
The objective for Experiment 1 was to (a) identify if temperature or water availability at seed origin affected plant physiology and growth in aCO2 and (b) if the observed trends remain under eCO2. Experiment 1 lasted from March to June in 2015. Four provenances of E. grandis were selected (Table 1, Figure 1). Detailed climate-of-origin data were obtained from eMAST (www.emast.org.au) to calculate MAT, MAP and evapotranspiration (ET) between 1971 and 2010 for each location (see Aspinwall et al., 2017). Final selection for Experiment 1 was based on forming three pairs of MAT and water availability regimes (ratio of ET and MAP). Pair 1: Mt Lindsay and Coffs Harbour had the same low ET/MAP (wet) but different MAT (cool/warm). Pair 2: Coffs Harbour and Kilcoy Creek had similar MAT (warm) but different ET/MAP (wet/dry). Pair 3: Kilcoy Creek and Woondum had similar ET/MAP (dry) but different MAT (warm/hot). Seed was obtained from five mother trees (family) within each provenance of Experiment 1. Seeds were germinated under ambient atmospheric conditions and individuals grown at a plant nursery to enable collection of vegetative cuttings. Randomly selected seedlings from each provenance were coppiced to produce hedges for rooted cutting propagation. After development of sufficiently strong roots, cuttings were transported into temperature and CO2 controlled glasshouse rooms (12 March 2015) at the Hawkesbury campus of Western Sydney University (Richmond, NSW, Australia). There, seedlings were transplanted into pots made of PVC pipe (15 cm in diameter, 40 cm high; 7 L volume) and filled with a customized soil, which consisted of 90% coarse washed river sand, 10% diatomaceous earth (intermediate grade: 0.9–2 mm from Mt Sylvia, QLD) and mineral soil (Zeogreen, premium turf grade 0.5–1.6 mm from ZeoGreen, VIC, Australia). Pots were watered daily to saturation. Liquid fertilizer (500 mL Aquasol, at 1.6 g l−1; 23% N, 4% P, 18% K, 0.05% Zn, 0.06% Cu, 0.013% Mo, 0.15% Mn, 0.06% Fe, 0.011% B; Yates Australia, NSW, Australia) was added to pots every 14 days to minimize potential nutrient limitation.
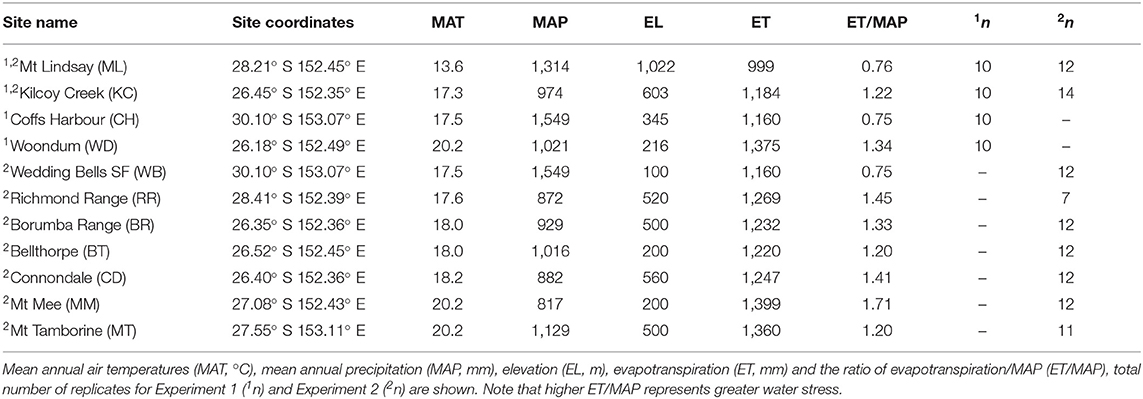
Table 1. Location and climate variables characterizing the origin of E. grandis seed provenance for Experiment 1 (n = 40) and Experiment 2 (n = 104); see superscript numbers.
Seedlings were grown for 14 weeks (12 March to 15 Jun 2015) under natural light in four adjacent temperature and CO2 controlled glasshouse rooms. Temperature (T) in all glasshouse rooms was programmed to represent the 30 year average of November to May conditions in Richmond NSW and diel variation (0600–1,000 h: 22°C; 1,000–1,600 h: 26°C; 1,600–2,000 h: 24°C; 2,000–0600 h: 15°C). Ambient and elevated CO2 concentrations were set at 400 and 640 μmol mol−1 in two rooms per treatment, respectively, and controlled automatically (Lambda T, ADC BioScientific Ltd., Herts, UK). Environmental conditions inside the glasshouse bays were logged every 15 min. Ten plants of each provenance (40 seedlings total) were randomly assigned to the four glasshouse rooms and rotated between and within rooms every 14 days. Initially, 20 seedlings were grown in aCO2 and 20 seedlings in eCO2. At the end of the experiment, seedlings were destructively harvested. Leaf number was determined for each plant and leaf area (LA) of the canopy of each seedling was measured by placing all leaves into a leaf area meter (LI-3100C, Li-Cor Inc., NE, USA). Roots were carefully washed free of soil medium. Leaves, stems and roots were dried at 70°C to constant mass to determine dry weight.
Leaf Gas Exchange
Light-saturated photosynthetic rates of CO2 assimilation (Asat, μmol m−2 s−1) and stomatal conductance of water vapor (gs, mol m−2 s−1) of fully expanded leaves were measured during the day (0930–1,530 h, sunny days) using an infrared gas analyzer (LI-6400XT, Li-Cor Inc., NE, USA). Measurement conditions for Asat were set as: saturating light = 1600 μmol m−2 s−1; block T = 26°C; CO2 concentration = 400 or 640 μmol mol−1; and flow rate = 500 μmol s−1. Leaf vapor pressure deficit (VPD) was regulated by manipulating relative humidity inside the cuvette to maintain VPD between 1.2–1.6 kPa. Leaves were allowed to equilibrate to environmental conditions inside the cuvette for at least 5 min prior to measurement. Gas analysers were always matched before logging data. A minimum of five measurements per seedling were logged at 5 s intervals and later averaged. Care was taken that leaves covered the full 2 × 3 cm measurement chamber.
Leaf δ13C
Relative abundance of 13C to 12C in leaf material can be used as an integrated measurement of water use efficiency (WUE) over a longer timespan (Farquhar et al., 1989). For this purpose, one fully developed leaf per seedling was collected on days of leaf gas exchange measurements during Experiment 1. Leaves were snap-frozen in liquid nitrogen, dried at 60°C for 3 days and ground to a fine powder. Only leaves that were used for gas exchange measurements were collected. Analyses of leaf bulk tissue (δ13Cleaf) were completed at the Australian National University using a continuous-flow stable isotope ratio mass spectrometer (Fison Isochrom CF-IRMS, former Fisons Instruments, UK). The photosynthetic discrimination rate (Δ13C), which relates to WUE for the hours of 0900–1600, was calculated from leaf material (δ13Cleaf) and known atmospheric values (δ13Cair), expressed in ‰ (Farquhar et al., 1982):
Since glasshouse air was artificially enriched with 13C depleted CO2, the δ13C fraction of glasshouse room air (δ13Cair) was also determined to calculate Δ13C. This was achieved by collecting measurements of air of all four glasshouse rooms separately using a Tuneable Diode Laser (TGA100; Campbell Scientific, UT, USA), repeated for each room three times during a diel cycle to account for diurnal variation of CO2. Mean Δ13C was calculated for each glasshouse room. After determining Δ13C, WUE was calculated as:
where gs is stomatal conductance of water vapor, a is the average fractionation constant (4.4‰, O'Leary, 1981) for the diffusion of gases through stomata and b' represents the carboxylation fractionation by Rubisco and PEP carboxylase (27‰, Farquhar and Richards, 1984). The factor 1.6 accounts for the greater diffusivity of water vapor vs. CO2 in air and ca represents the partial pressure of CO2 in the outer atmosphere.
Mean response ratios (RR) for Asat, gs, and Δ13C between eCO2 and aCO2 were calculated by dividing the mean value of the parameter in eCO2 by the mean value in aCO2. Variance of these RR was calculated according to Hedges et al. (1999).
Non-structural Carbohydrates
Plant tissues (leaves, stem, and roots) were sampled during morning hours of the final harvest of Experiment 1 to assess concentration of non-structural carbohydrates (NSC). Tissues were microwaved for 10 s to stop metabolic activity and then dried at 70°C for 3 days. A detailed protocol for NSC extraction can be found in Tissue and Wright (1995). In short, subsamples of plant material were dried and ground to a fine powder before extracting NSC. Starch was separated from soluble sugars, lipids and amino acids using a methanol/chloroform/water solution. Water soluble sugars were partitioned from remaining cell components by phase separation. Starch was digested into ss with perchloric acid. Sugar content of both fractions was quantified colorimetrically in a spectrophotometer (DU800, Beckman Coulter Australia Pty Ltd, NSW, Australia) via a phenol-sulfuric acid reaction (Tissue and Wright, 1995).
Biomass
At harvest (15–18 June 2015, 14 weeks since repotting), leaf area (LA) of the whole canopy was measured by placing all leaves into a leaf area meter (LI-3100C, Li-Cor Inc., NE, USA); leaf size was estimated by dividing LA by leaf number. Leaf mass per area (LMA) was calculated by dividing dry weight of its leaves (DWleaf) by LA. Measurements of height (h, cm) and diameter (d, mm; measured 2 cm above soil surface) commenced at week 2 since repotting into the glasshouse and were subsequently conducted on a weekly basis. Stem volume (vstem, cm3) was estimated using h and d, assuming the shape of a cone using the following formula:
Total plant dry mass (DWplant) was calculated by summing DWleaf, DWstem, and DWroot. Biomass fraction was calculated by dividing DW of plant organs by DWplant as leaf-mass-fraction (LMF), stem-mass-fraction (SMF) and root-mass-fraction (RMF).
Data Analyses
Data were analyzed using R-3.6.1 software (R Core Team, 2019). Analysis of variance (ANOVA) was used to test if the [CO2] treatment as well as the origin of the four provenances had a significant effect on leaf gas exchange, growth-related traits (DW, vstem, LMF, SMF, RMF) leaf specific traits (leaf number, leaf size, LA, LMA) and NSC. Statistical models were tested with all interactions between factors ([CO2] and provenance). Assumptions of data normality and equal variance were tested using Shapiro-Wilk and Levene's test, as well as a quantile-quantile plot for visual assessment. The “car” package (Fox and Weisberg, 2019) was used for Anova() and leveneTest() functions with Type III sums of squares and Levene's test for equal variance among test groups, respectively. Post-hoc tests were conducted when plant traits were significantly different across provenances. To establish whether Asat or gs was the driver for changes in Δ13C correlations were analyzed with Pearson's correlation coefficient using the cor.test() function of the base package. All means are presented with one standard error (SE).
Experiment 2
Plant Material
The objective of Experiment 2 was to validate results of Experiment 1 recorded for a narrow climate of seed origin gradient and be able to identify a generalized growth response strategy of E. grandis provenances. Experiment 2 lasted from February to May 2017. The range of climate at seed origin was extended to nine provenances in Experiment 2 including two provenances from Experiment 1 (Table 1, Figure 1). Detailed selection criteria for the provenances used in Experiment 2 can be found in Aspinwall et al. (2017). Seed was obtained from 2 to 5 mother trees within each provenance. Seeds were germinated as described for Experiment 1 and were transported into temperature and CO2 controlled glasshouse rooms on 27 February 2017 using similar pots and soil as in Experiment 1.
Seedlings of Experiment 2 were grown at the same location as Experiment 1 for 10 weeks (27 February to 11 May 2017). Glasshouse environmental conditions were comparable to Experiment 1. Experiment 2 included 3–7 plants per provenance, of which 52 were randomly assigned and grown in aCO2 and 52 plants in eCO2. Seedlings were destructively harvested in the same way as Experiment 1.
Biomass
Harvest was conducted on 10 and 11 May 2017, 10 weeks since repotting. The same measurements were taken as in Experiment 1. Measurements of h and d commenced during the first week following replanting into the glasshouse.
Data Analyses
Data were analyzed using R-3.6.1 software (R Core Team, 2019). Analysis of variance (ANOVA) was used to test if the [CO2] treatment as well as climate origin of the nine provenances had a significant effect on growth-related traits (DW, vstem) and leaf specific traits (LA, LMA). See Experiment 1 for further details. Correlations between DWshoot and DWstem, respectively, and climate variables (MAT, MAP, ET/MAP) were analyzed with Pearson's correlation coefficient using the cor.test() function of the base package. The same approach was used for determining the correlation between DWstem and LMA. All figures were drawn using Python (Phyton Software Foundation)1.
All figures were plotted using Python (Phyton Software Foundation).
Results
Experiment 1
Environmental Conditions
Throughout Experiment 1, relative humidity ranged from 57–85%, T averaged 26.9 ± 0.1(±1SE) °C during the photoperiod, and excellent [CO2] control was achieved (aCO2: 401 ± 0.2 μmol mol−1; eCO2: 645 ± 0.1 μmol mol−1). From March to June 2015, mean photon flux density during mid-day was 950 μmol m−2 s−1, ranging from 115 μmol m−2 s−1 on a cloudy day to 1,900 μmol m−2 s−1 on a sunny day.
Physiological Responses to eCO2
Asat differed significantly by provenance and [CO2] treatment. However, there were no provenance by [CO2] interactions statistically confirming these observed trends. In aCO2, Asat of seedlings originating from the warmest climate (provenance Woondum) was significantly lower (18.2 ± 1.4 μmol m−2 s−1, p = 0.002; Figure 2) than Asat of seedlings in the other three provenances, which were similar to each other (22.1 ± 0.6 μmol m−2 s−1). Across all provenances, Asat was on average 23% greater in eCO2 (23.3 ± 0.9 μmol m−2 s−1) compared to those grown in aCO2 (19.0 ± 0.6 μmol m−2 s−1, p = 0.003). In eCO2, Asat of seedlings from Woondum was 26% lower than seedlings originating from cooler climates (Figure 2). Notably, seedlings from the coolest climate (Mt Lindsay) showed on average the largest response to eCO2, increasing Asat by 40% in eCO2 compared to aCO2, yet also displayed high variability within this provenance (Figure 3).
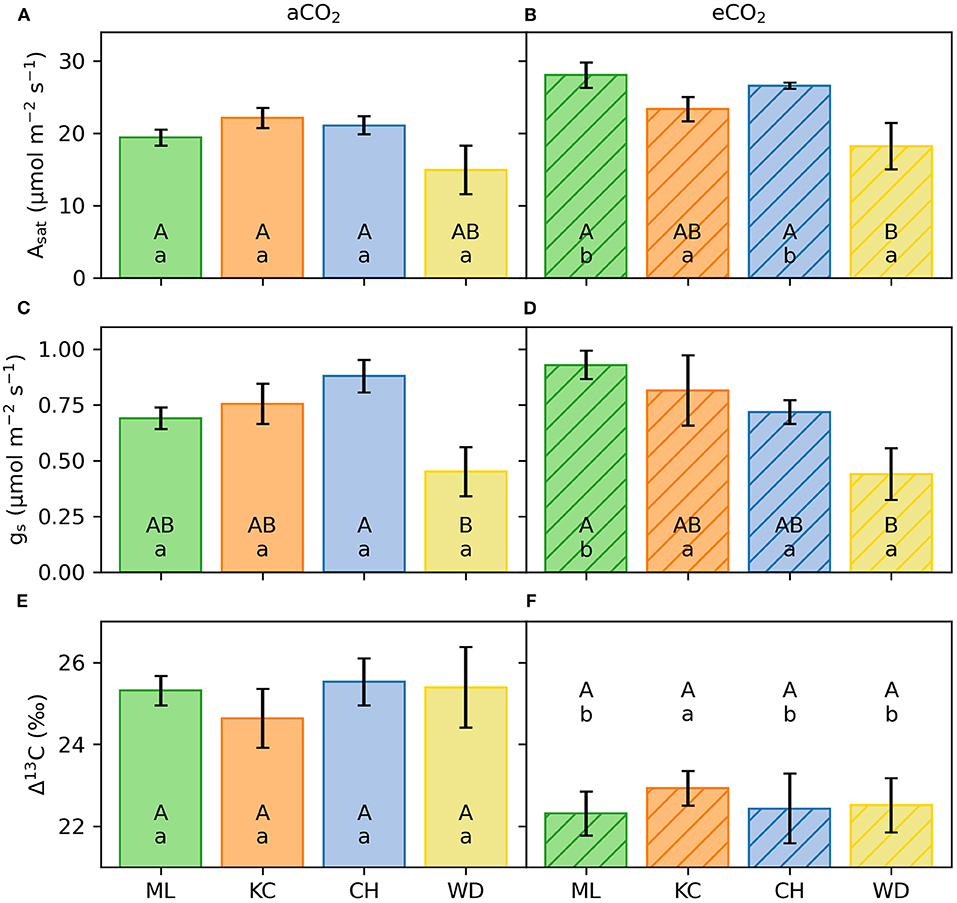
Figure 2. Light-saturated photosynthesis (Asat; A,B) stomatal conductance (gs; C,D) and carbon isotope discrimination (Δ13C; E,F) of four provenances of E. grandis selected for Experiment 1. Panels on the left show seedlings grown in ambient [CO2] (aCO2), panels on the right show seedlings grown in elevated [CO2] (eCO2; n = 5 per provenance). Error bars represent 1SE. Different capital case letters represent statistical significance of traits among provenances within the same [CO2] treatment. Different lower-case letters represent statistical significance of traits between [CO2] treatments per provenance.
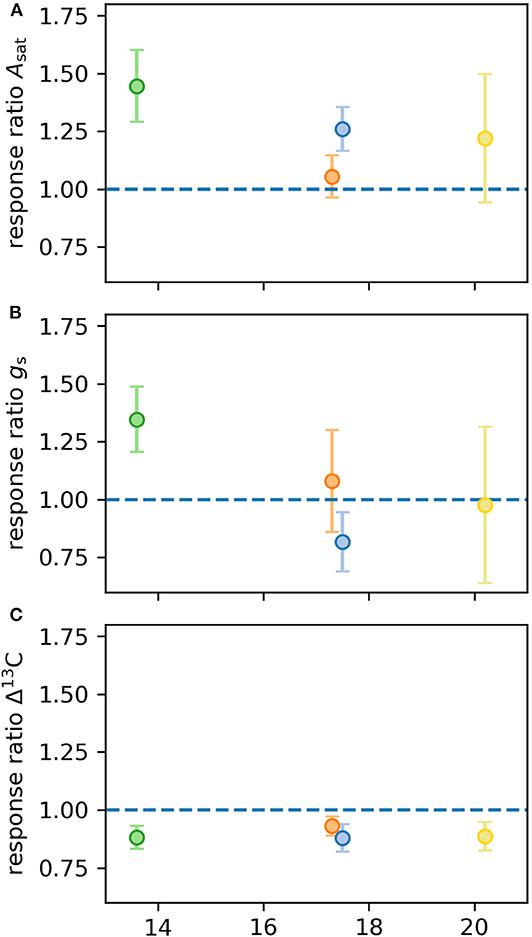
Figure 3. Response ratios of leaf physiological traits calculated for Experiment 1. Response ratios represent differences in four different provenances of E. grandis grown under eCO2 compared to aCO2 (n = 5 per provenance and [CO2] treatment). Traits: light-saturated photosynthetic carbon assimilation (Asat; A), stomatal conductance (gs; B), carbon isotope discrimination (Δ13C; C). Error bars represent 1SE.
[CO2] treatment did not affect gs, but values differed across provenances. In May 2015, seedlings growing in aCO2 and originating from provenance Woondum had 49% lower gs (0.45 ± 0.11 mol m−2 s−1) than seedlings from provenance Coffs Harbour, averaging 0.88 ± 0.07 mol m−2 s−1 (p = 0.001; Figures 2, 3). Seedlings from provenances Mt Lindsay and Kilcoy Creek had intermediate mean values of 0.69 ± 0.05 mol m−2 s−1 and 0.75 ± 0.09 mol m−2 s−1, respectively. In eCO2, seedlings from provenance Woondum had similar low gs averaging 0.44 ± 0.12 mol m−2 s−1 while seedlings from provenance Mt Lindsay had the highest values with 0.93 ± 0.06 mol m−2 s−1 (35% increase from aCO2 to eCO2).
Provenances did not differ in Δ13C when grown in aCO2. Seedlings grown in eCO2 had an improved WUE, indicated by Δ13C being significantly lower compared to seedlings grown in aCO2 (22.5 ± 0.3o vs. 25.2 ± 0.3o, p < 0.001; Figures 2, 3). Asat was negatively correlated with Δ13C (Pearson's r = −0.59, p > 0.001) whereas gs was not (p = 0.99).
Non-structural Carbohydrates
eCO2 did not change concentrations of soluble sugars or starch in any provenance or tissue. For instance, soluble sugars in leaves averaged 55.0 ± 2.1 mg g−1 dry weight in aCO2 and 53.8 ± 1.6 mg g−1 dry weight in eCO2. Leaf starch averaged 68.0 ± 1.7 mg g−1 dry weight in aCO2 and 64.5 ± 1.4 mg g−1 dry weight in eCO2. Data were pooled for [CO2] treatments to assess variation of NSC concentration in plant organs among provenances. Concentrations of soluble sugars at harvest differed significantly in leaves, stem and roots among provenances. Seedlings originating from Woondum had the greatest concentrations of soluble sugars in leaves (60.1 ± 2.5 mg g−1 dry weight, p = 0.02) and stem tissue (11.4 ± 1.0 mg g−1 dry weight, p < 0.001), respectively. Concentrations in leaves were 21% greater than soluble sugars in seedlings from Coffs Harbour and 64% greater than soluble sugars in stem tissue of Kilcoy Creek. In roots, concentration of soluble sugars differed significantly among provenances (p = 0.02), where seedlings originating from Coffs Harbour contained the greatest amount of soluble sugars (18.3 ± 0.9 mg g−1 dry weight) followed by those from Woondum (16.7 ± 1.8 mg g−1 dry weight). Concentrations of starch at harvest only differed among provenances in leaves (p = 0.05; Figure 4). Seedlings from Kilcoy Creek had the greatest starch concentrations in leaves (69.7 ± 2.0 mg g−1 dry weight), whereas those from Woondum had the lowest (61.3 ± 2.0 mg g−1 dry weight; Figure 4).
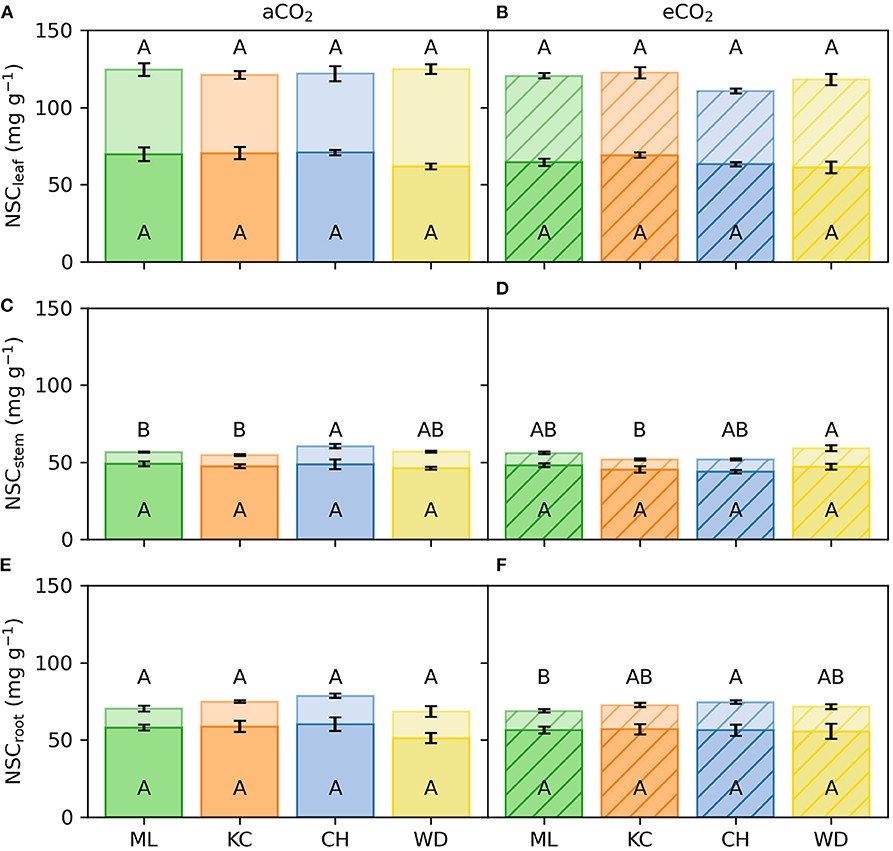
Figure 4. Non-structural carbohydrates (NSC) in three tissues of E. grandis. Samples were collected at the final harvest. Data are shown for ambient [CO2] treatment (aCO2; left; n = 5) and elevated [CO2] treatment (eCO2; right; n = 5). NSC are presented for two fractions: soluble sugars (ss; light colored area) and starch (st; full colored area) in leaves (NSCleaf; A,B), stem (NSCstem; C,D) and roots (NSCroot; E,F). Error bars represent 1SE. Colors and abbreviations represent different provenances. Different capital case letters represent statistical significance of ss or st, respectively, among provenances within the same [CO2] treatment.
Growth
At harvest, seedlings were 62–118 cm tall. Throughout the experiment, stem volume (vstem) was not significant across [CO2] treatments (p = 0.15) nor [CO2] x provenance interaction (p = 0.91) but varied across provenances (p < 0.001; Figures 5A,B). Specifically, seedlings originating from the warmest climate (Woondum) had continuously lower stem volume than seedlings from the coolest climate (Mt Lindsay). Trends were detected for relative responses of vstem from aCO2 to eCO2 with seedlings from Coffs Harbour indicated the greatest increase (+33%). At harvest, DWplant of individual seedlings varied from 7.3 to 59.5 g. Leaf, stem and root biomass was only significant across provenances, but not [CO2] nor their interactions (Table 7). Under aCO2, seedlings from Woondum had the lowest DWleaf (p = 0.004), DWstem (p < 0.001; Figure 6A), and DWroot (p < 0.001) followed by Coffs Harbour. Seedlings from Mt Lindsay had the greatest DWplant (39.8 ± 3 g; Table 3; Figure 6A). DWroot was essentially statistically significant (p = 0.07) increasing from 4.9 ± 0.6 g in aCO2 to 6.3 ± 0.8 g in eCO2 (Table 3). Responses of DWplant from aCO2 to eCO2 widely differed across provenance for biomass of all plant tissues (DWstem as example shown in Figures 6B, 7A). As a trend, seedlings from Coffs Harbour increased mass in all tissues resulting in a mean DWplant of 34.7 ± 4.4 g (+44%). DWstem was correlated to climate parameters MAT, MAP, ET/MAP, mean annual minimum and maximum temperature. The best fit was found for MAT (r2 = 0.38, p = 0.004; Figures 8A,B) where biomass decreased with greater MAT. In aCO2, this linear fit had a negative slope of −1.54, whereas the slope in eCO2 was slightly steeper with −1.74. Biomass partitioning calculated as LMF, SMF and RMF did not differ among provenances, but LMF was statistically significant and RMF essentially significant, respectively between [CO2] treatments (Table 7). In aCO2, LMF averaged 0.40 ± 0.008 compared to 0.37 ± 0.009 in eCO2 (p = 0.01; Table 3). RMF showed an opposite trend increasing from 0.17 ± 0.006 in aCO2 to 0.19 ± 0.01 in eCO2 (p = 0.09; Table 3).
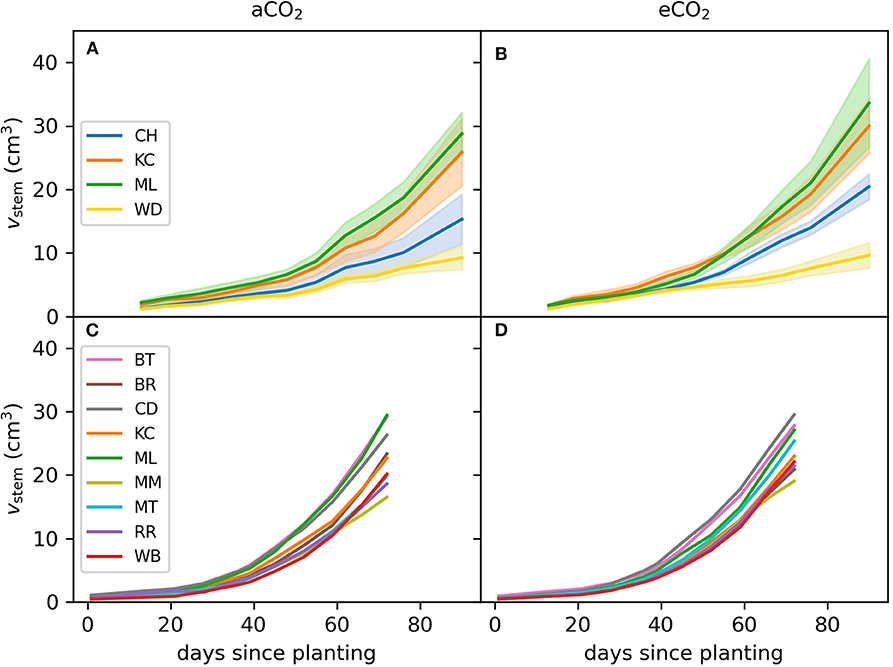
Figure 5. Stem volume of E. grandis during Experiment 1 and 2. Seedlings (n = 3–7 per provenance) were grown in ambient (aCO2; left) and ambient [CO2] (eCO2; right). Colors in (A,B) represent the four selected provenances of Experiment 1. The nine selected provenances of Experiment 2 are shown in (C,D). Shaded bands represent 1SE.
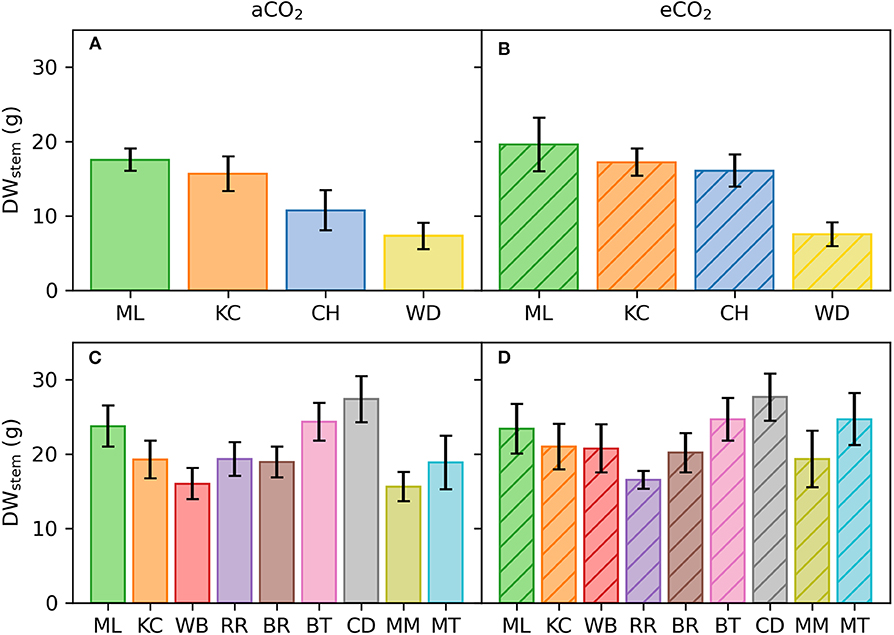
Figure 6. Mean stem dry weight (g) of E. grandis at the end of Experiment 1 and 2. Seedlings (n = 3–7 per provenance) were grown in ambient (aCO2; left) and elevated CO2 (eCO2; right). Colors in (A,B) represent the four selected provenances of Experiment 1. The nine selected provenances of Experiment 2 are shown in (C,D). Error bars represent 1SE.
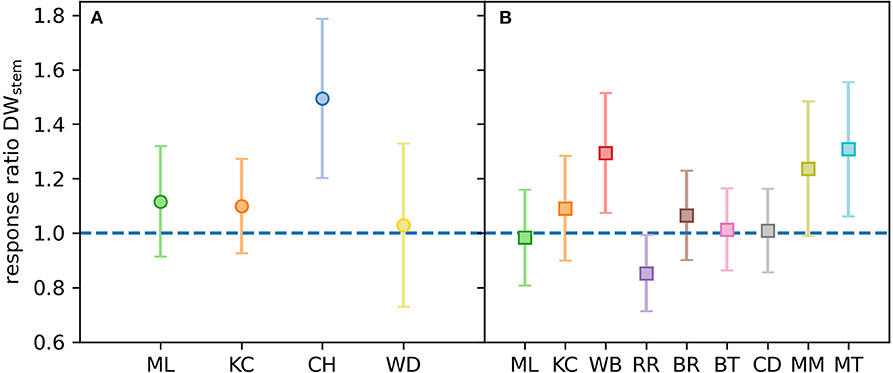
Figure 7. Response ratios of stem dry weight at harvest (DWstem) for Experiment 1 and 2. Response ratios represent differences in the different provenances of E. grandis grown under elevated [CO2] (B) compared to ambient [CO2] (A; n = 3–7 per provenance and [CO2] treatment). Colors in (A) represent the four selected provenances of Experiment 1 and colors in (B) represent the nine selected provenances of Experiment 2. Error bars represent variance.
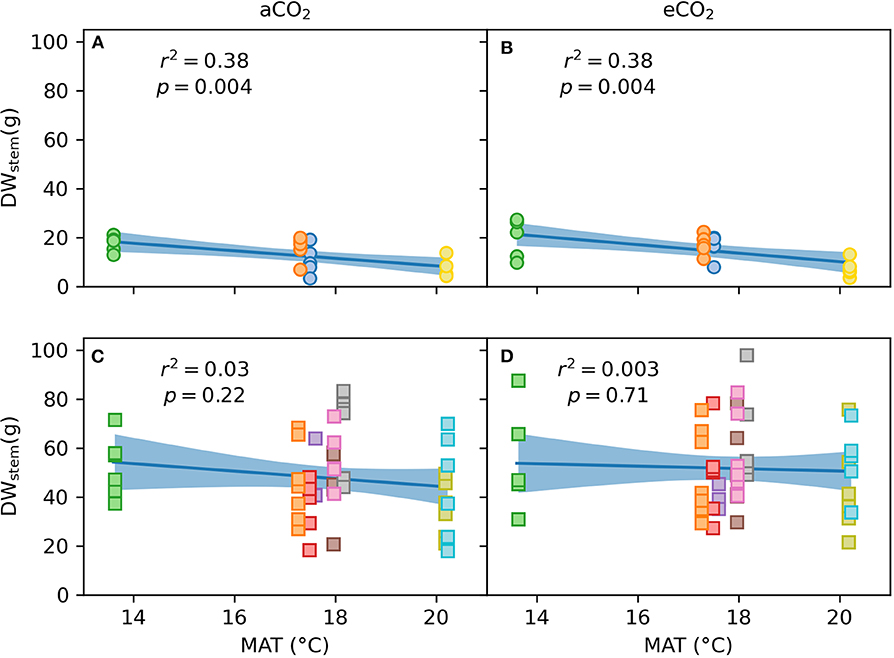
Figure 8. Correlation between stem dry weight at harvest (DWstem) and mean annual temperature (MAT) at seed origin for Experiment 1 and 2 for ambient (aCO2; A,B) and elevated [CO2] (eCO2; n = 3–7 per provenance and [CO2] treatment; C,D). Circles in (A,B) represent data of the four provenances in Experiment 1, squares in (C,D) represent data of the nine provenances in Experiment 2. Dark blue line shows linear fit of the data with light blue areas representing confidence intervals.
At final harvest, leaf number and LA differed significantly among the four provenances, while leaf size and LMA did not. Leaf traits did not significantly respond to [CO2] nor [CO2] x provenance interaction. Under aCO2, seedlings from Mt Lindsay had developed the most leaves (236 ± 24 plant−1, p = 0.01) and had also the largest leaf area (4249 ± 143 cm2 plant−1, p = 0.003; Table 2). Seedlings from provenance Woondum had developed about half of the number and area of leaves compared to those from Mt Lindsay. Seedlings from Kilcoy Creek had the largest leaf size (19.0 ± 3.0 cm2) and those from Coffs Harbour the smallest (15.1 ± 1.9 cm2; Table 2). LMA under aCO2 was similar among provenances, averaging 36.5 to 40.0 g m−2. Although a trend toward increased leaf size and area was apparent in all seedlings when grown in eCO2, large intra-specific variation resulted in non-significant changes compared to seedlings grown in aCO2.
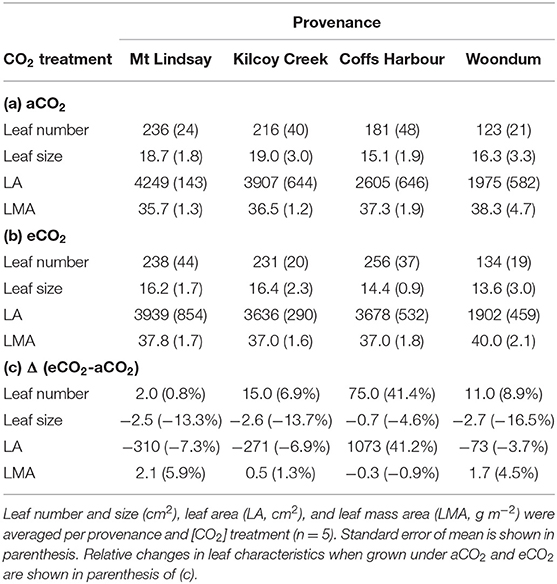
Table 2. Leaf characteristics of E. grandis seedlings of Experiment 1 grown in ambient [aCO2; (a)] and elevated [CO2] [eCO2; (b)].
Experiment 2
Environmental Conditions
Relative humidity during Experiment 2 ranged from 62 to 88% between the hours of 10:00 and 16:00. T averaged 27.6 ± 0.2°C and mean [CO2] were for aCO2 396 ± 0.2 μmol mol−1 and for eCO2 643 ± 0.2 μmol mol−1.
Growth
At harvest, seedlings were 58–109 cm tall. Throughout Experiment 2, vstem was not significant across [CO2] treatments nor [CO2] x provenance interaction yet varied across provenances (p < 0.001; Figures 5C,D). Seedlings originating from provenance Bellthorpe had continuously greater stem volume than seedlings from provenance Mt Mee (Figure 5). Leaf and stem biomass were not significant across [CO2] treatments (p =) nor [CO2] x provenance interaction but differed among the nine provenances (Table 5). Under aCO2, seedlings from provenance Mt Mee of the warmest and wettest climate had the lowest DWleaf (p = 0.008) and DWstem (p = 0.01; Figure 6C). Seedlings from provenance Connondale had the greatest DWshoot (67.3 ± 6.9 g; Table 5), 91% greater than seedlings from Mt Mee. Similar to Experiment 1, trends for intraspecific variability in growth response to eCO2 were noticeable (Table 5; Figures 6D, 7B). Seedlings from provenance Wedding Bells SF, similar to seedlings from Coffs Harbour in Experiment 1, had the greatest increase of DWshoot resulting in a mean of 49.1 ± 7.1 g (+31% from aCO2 to eCO2). Yet, dry weight of shoot and stem was, contrary to Experiment 1, not correlated to any climate parameters of seed origin in aCO2 or eCO2 (Table 6; Figures 8C,D).
LA and LMA of the nine selected provenances only differed significantly among provenances at the end of Experiment 2 (Table 4). Under aCO2, seedlings from Connondale originating from warmer and wetter climate had the largest LA (8212 ± 779 cm2, p = 0.002; Table 4). Seedlings from provenance Mt Mee, originating from the wettest climate, had only half the LA compared to Connondale. LMA under aCO2 differed among the nine provenances. Seedlings from provenance Richmond Range with relatively wet climate had the greatest LMA averaging 55.9 ± 3.0 g m−2. Similar to Experiment 1, seedlings from provenance Mt Lindsay had the lowest LMA (41.9 ± 1.2 g m−2). Trends for intraspecific variation in the response of leaf traits to eCO2 were noticeable. Six of the nine provenances had increased LA under eCO2 with the largest increase in seedlings from provenance Wedding Bells SF (+28%; Table 4), resembling similar climate to seedlings from provenance Coffs Harbour in Experiment 1. In aCO2, LA was negatively correlated to MAT (Pearson's r = −0.27, p = 0.05) and ET of seed origin (Pearson's r = −0.27, p = 0.05; Table 6). LMA was positively correlated to DWstem (Pearson's r = 0.29, p = 0.04 in aCO2 and Pearson's r = 0.44, p = 0.001 in eCO2, respectively).
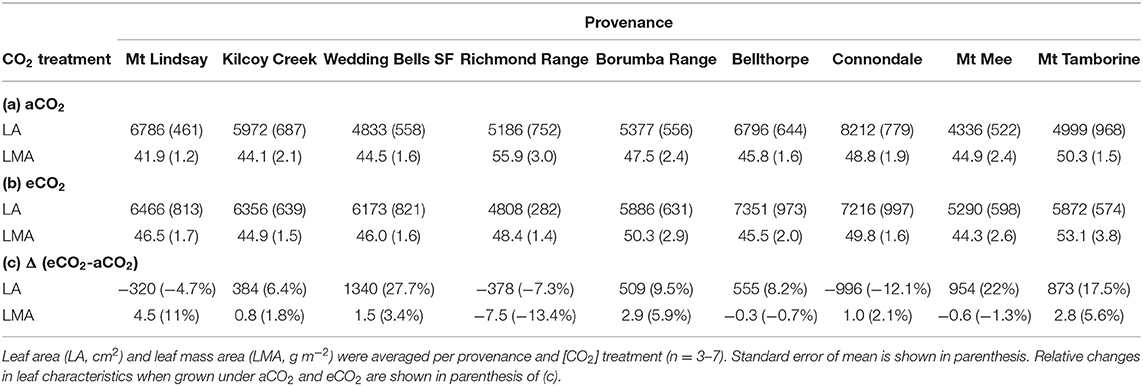
Table 4. Leaf characteristics of E. grandis seedlings of Experiment 2 grown in ambient [aCO2; (a)] and elevated [CO2] [eCO2; (b)].
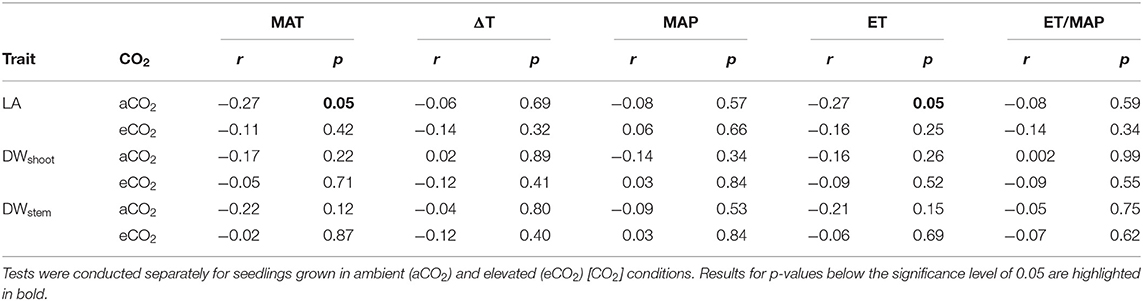
Table 6. Pearson's correlation coefficient (Pearson's r) between leaf area (LA), shoot dry weight (DWshoot) and stem dry weight (DWstem), and climate variables of seed origin of the nine provenances selected for Experiment 2: mean annual temperature (MAT, °C), difference between mean annual maximum and minimum temperature (ΔT, °C), mean annual precipitation (MAP, mm), mean annual evapotranspiration (ET, mm), and evapotranspiration/MAP (ET/MAP).
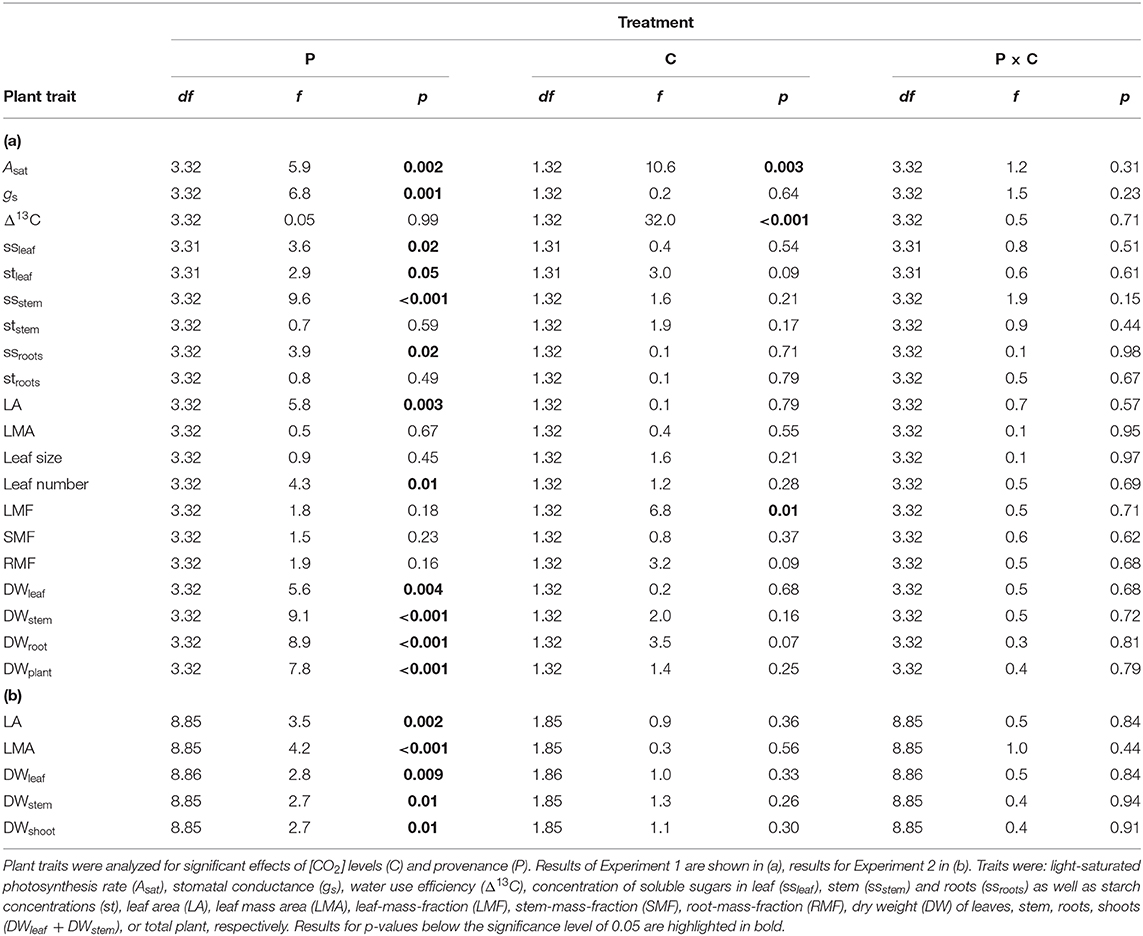
Table 7. Statistical results with degrees of freedom (df), f-values (f), and p-values (p) for Analysis of Variance (ANOVA) for E. grandis.
Discussion
Two experiments on E. grandis were used to investigate phenotypic plasticity in this important hardwood plantation species. These experiments revealed significant variability regarding leaf physiology traits, concentrations of non-structural carbohydrates and growth parameters among provenances grown in aCO2. Yet, contrary to our expectation, variation in biomass was not systematically related to climate at seed origin in E. grandis seedlings.
Although eCO2 increased Asat and WUE (calculated from leaf carbon isotope discrimination and reflecting the lifespan of the leaf), this did not result in a systematic stimulation of growth and increased biomass among provenances. Systematic stimulation of growth by eCO2 was not apparent, possibly due to the limited number of selected provenances and high intraspecific plant-to-plant variation. However, even by increasing the number of tested provenances to improve statistical power in Experiment 2, we did not observe a uniform growth stimulation and biomass (stem, leaves, roots) increase in eCO2 nor a significant provenance by CO2 effect. However, some provenances did show greater responses of physiology (Asat and gs) or growth in eCO2 than others, potentially related to environmental conditions of seed origin—as seen with provenances Coffs Harbour and Wedding Bells SF with strong positive biomass increases under eCO2. Yet, unidirectional phenotypic plasticity among provenances as result of eCO2 was not observed due to great variability within groups.
Physiological Differences Among Provenances
Greater carbon assimilation, due to greater Asat and a larger canopy, enabled seedlings from regions with low ET/MAP to also produce more stem biomass (i.e., provenance Coffs Harbour) relative to seedlings from drier climates (i.e., provenance Woondum and Mt Mee). Variability in LMA among provenances can also influence plant growth (Poorter et al., 2009) as shown with our LMA data correlating with stem biomass. As LMA has a strong positive relationship with leaf density, our results suggest that provenances MT and RR have greater leaf density than provenances KC and ML. Higher leaf density may be caused by a greater proportion of mesophyll tissue or lignified tissue resulting in greater leaf toughness and consequently plant survival (Poorter et al., 2009). Despite these observed trends, stem biomass of E. grandis was not correlated to climate of origin in Experiment 2. We thus had to reject the notion that results from Experiment 1 could be used to extrapolate growth responses to eCO2 across wider environmental gradients. However, intraspecific differences across provenances or families are frequently reported for trees in response to their local climate conditions. European pine and beech species (Correia et al., 2008; Rose et al., 2009; Bachofen et al., 2018) as well as North American poplars (Kaluthota et al., 2015) as well as eucalypts (Blackman et al., 2016; Aspinwall et al., 2018) have been shown to display considerable intraspecific variation in physiological and growth-related traits when grown as potted plants or in common gardens. These variations were correlated to temperature, water availability and the length of the growing season at seed origin. Seedlings from provenances originating either from areas with wet and cool or wet and warm climate produced the greatest biomass when grown at aCO2. In support of this trend of adaptation to local climate conditions, biomass production in E. grandis was greater in trees from cooler and wetter climates (Drake et al., 2015). Thus, E. grandis does show signs of a systematic adaption to environmental conditions at seed origin, yet climate conditions alone are insufficient to explain observed responses for every provenance.
Physiological Responses of Provenances to eCO2
Intraspecific variation across provenances was more prominent than responses to eCO2. Hence, ranking among provenances for physiology and growth traits persisted, supporting Hypothesis 2. Asat increased by 24% on average, similar to the stimulation of Asat under eCO2 in other trees (e.g., Ainsworth and Rogers, 2007; Ghannoum et al., 2010; Leakey et al., 2012; Resco de Dios et al., 2016), which predominately results from increased rates of carboxylation by Rubisco and reduced rates of photorespiration (Drake et al., 1997; Long et al., 2004). Additionally, eCO2 increased WUE in all provenances (i.e., Δ13C declined), an effect that has been attributed to decreases in gs (Saxe et al., 1998; Medlyn et al., 2001) and subsequent decline in conductance of water vapor by leaves (e.g., Ainsworth and Rogers, 2007; Adair et al., 2011; Keenan et al., 2013; Reich et al., 2014; Frank et al., 2015; Dekker et al., 2016). Yet, in E. grandis, improved WUE was due to increasing carbon uptake (increases in Asat) under non-limiting water supply rather than decreases in gs, a strategy also confirmed for Fagus sylvatica (Aranda et al., 2017). Under eCO2, gs is commonly reported to decline (e.g., Ainsworth and Long, 2005). However, a lack of downregulation of gs under eCO2 was also found in Larix and Pinus trees (Streit et al., 2014). Furthermore, the strong influence of leaf tissue structure on gas exchange in E. camaldulensis (Blackman et al., 2016) may explain why gs response to eCO2 was not significant given that LMA was also not affected in E. grandis. Regrettably, we did not investigate stomatal traits, which might have helped differentiate anatomical from biochemical constraints on WUE (Blackman et al., 2016). Limited information suggests changes in stomatal density of broadleaved trees may occur as an interactive response to [CO2], water availability and origin of provenance (Pyakurel and Wang, 2014). In agreement with the current study, most leaf physiological traits in 14 genotypes within six provenances of E. camaldulensis showed extensive intraspecific variation, but a limited growth response to eCO2 (Blackman et al., 2016).
When trees are grown under eCO2, they often exhibit increased carbon assimilation rates. However, these increases sometimes are not reflected in simultaneous increases in stem biomass, indicating that additional carbon gained is not necessarily allocated to stem growth (Resco de Dios et al., 2016). For example, net carbon assimilation of E. camaldulensis was greatly stimulated by eCO2, yet no increase in stem biomass or LMA (leaf structure) was detected (Blackman et al., 2016). In the present study, concentration of starch in leaves of E. grandis was greater in eCO2 compared to aCO2, suggesting that leaves may serve as temporary storage organs under eCO2 rather than building new leaf structure (e.g., larger or thicker leaves).
Another possible fate of additional carbon gained in eCO2 can be its loss through elevated rates of cell respiration and/or root exudation. We did not examine either of the two processes, but studies have shown that large variability exists in the effects of eCO2 on plant mitochondrial respiration rates (see Dusenge et al., 2019 for review). Studies have found respiration to remain stable, increase or decline when plants are exposed to eCO2 (Curtis, 1996; Wang et al., 2001; Tissue et al., 2002; Ayub et al., 2011; Crous et al., 2012; Li et al., 2013; Gauthier et al., 2014). Increased rates of respiration would explain the absence of greater allocation of assimilated carbon into aboveground biomass in E. grandis. However, higher respiration rates have also been linked to greater carbohydrate accumulation in pine (Tjoelker et al., 2009; Li et al., 2013), but it was not observed here in E. grandis.
Growth is often enhanced in other species of Eucalyptus grown under eCO2 (Ghannoum et al., 2010; Ayub et al., 2011; Smith et al., 2012; Duan et al., 2013; Aspinwall et al., 2018), but was not statistically significant here for stem and leaf biomass of E. grandis. However, the lack of significant aboveground growth stimulation in eCO2 has been observed in other species of Eucalpytus (Duff et al., 1994) and Populus (Liberloo et al., 2005) despite increased carbon assimilation (Cantin et al., 1997; Atwell et al., 2007; Lawson et al., 2017; Bachofen et al., 2018; Killi et al., 2018). It has also been observed that carbon may be allocated belowground to facilitate increased nutrient uptake when photosynthesis is increased in eCO2 (Hättenschwiler and Körner, 1997; Iverson et al., 2008; Pritchard et al., 2008; Souza et al., 2016; Aspinwall et al., 2018). Here, 13% more biomass was on average allocated to roots rather than leaves (−8%) in Experiment 1. Thus, biomass responses of E. grandis to eCO2 may be due to carbon allocation to roots rather than aboveground biomass allocation.
Environmental Drivers
Cooler climate and low ET/MAP at seed origin of trees from Mt Linsday may represent contributing factors to the pronounced increase of Asat in the different provenances when grown in eCO2. A common garden study on European Picea abies found that populations from cooler climates at high-altitudes had higher leaf nitrogen concentrations, greater Amax and higher concentrations of chlorophyll than those from lower elevations (Oleksyn et al., 1998). In E. camaldulensis, genotypes with the largest growth response to eCO2 had large increases in root mass fraction and photosynthetic nitrogen-use efficiency (Aspinwall et al., 2018). The Coffs Harbour (Experiment 1) and Wedding Bells (Experiment 2) provenances had the largest relative increase of leaf area and biomass when grown in eCO2. Both locations are in very close proximity to each other and experience a similarly low ET/MAP compared to Mt Lindsey. Shifting such species from cooler to warmer growth conditions could influence temperature optima for photosynthesis. This physiological optimization due to warming while maintaining optimal access to water is in agreement with previous temperature-shift experiments using E. grandis (Drake et al., 2015).
In addition to climate, soil characteristics are known to influence productivity of eucalypt trees (Cavalli et al., 2020). Eucalyptus grandis, however, tolerates a wide range of soil conditions, reflected in high growth rates of provenances in their native habitats in Australia as well as plantations around the world. Here we controlled the influence of soil on growth by providing all provenances with the same customized, non-nutrient limited soil. The impact of eCO2 on stem growth of individual provenances, when grown in soil from their native habitat, should be investigated in future glasshouse experiments.
Conclusions
It now seems well-established that tree genotypes and provenances respond differentially to eCO2 (Ceulemans et al., 1996; Dickson et al., 1998; Isebrands et al., 2001; Mohan et al., 2004). While individual species may show increased stem growth and biomass accumulation under eCO2, this is not a widely observed trend (Resco de Dios et al., 2016). Here we added one of the most important hardwood plantation species to the growing list of tree species that seem to accelerate photosynthetic carbon assimilation under eCO2 yet do not use surplus photosynthates to increase stem growth. Selection of high performing tree provenances for the next generation of hardwood plantations in a higher CO2 world must account for this effect to remain economically profitable.
Data Availability Statement
The raw data supporting the conclusions of this article will be made available by the authors, without undue reservation, to any qualified researcher.
Author Contributions
AW, SP, and DT designed Experiment. AW, CB, DT, and SP designed Experiment. RS managed the controlled environment facilities, including CO2 treatments. AW analyzed the data and wrote the complete first draft. All authors collected plant physiological measurements, harvested data, and assisted in developing the final version of the manuscript.
Funding
This study was supported by the Science and Industry Endowment Fund (SIEF grant RP04-122) and the Higher Degree Research Scholarship from the Hawkesbury Institute for the Environment.
Conflict of Interest
The authors declare that the research was conducted in the absence of any commercial or financial relationships that could be construed as a potential conflict of interest.
Acknowledgments
The authors thank Carrie Drake, Wen Shi, and Craig Barton for technical support and helpful advice for development of this manuscript by Mark Tjoelker and Victor Resco de Dios is acknowledged.
Footnotes
1. ^Python Software Foundation. Python Language Reference, version 3.6. Available online at http://www.python.org.
References
Adair, E. C., Reich, P. B., Trost, J., and Hobbie, S. E. (2011). Elevated CO2 stimulates grassland soil respiration by increasing carbon inputs rather than by enhancing soil moisture. Glob. Change Biol. 17, 3546–3563. doi: 10.1111/j.1365-2486.2011.02484.x
Ainsworth, E. A., and Long, S. P. (2005). What have we learned from 15 years of free-air CO2 enrichment (FACE)? A meta-analytic review of the responses of photosynthesis, canopy properties and plant production to rising CO2. New Phytol. 165, 351–372. doi: 10.1111/j.1469-8137.2004.01224.x
Ainsworth, E. A., and Rogers, A. (2007). The response of photosynthesis and stomatal conductance to rising [CO2]: mechanisms and environmental interactions. Plant Cell Environ. 30, 258–270. doi: 10.1111/j.1365-3040.2007.01641.x
Aranda, I., Bahamonde, H. A., and Sánchez-Gómez, D. (2017). Intra-population variability in the drought response of a beech (Fagus sylvatica L.) population in the southwest of Europe. Tree Physiol. 37, 938–949. doi: 10.1093/treephys/tpx058
Aspinwall, M. J., Blackman, C. J., de Dios, V. R., Busch, F. A., Rymer, P. D., Loik, M. E., et al. (2018). Photosynthesis and carbon allocation are both important predictors of genotype productivity responses to elevated CO2 in Eucalyptus camaldulensis. Tree Physiol. 38, 1286–1301. doi: 10.1093/treephys/tpy045
Aspinwall, M. J., Jacob, V. K., Blackman, C. J., Smith, R. A., Tjoelker, M. G., and Tissue, D. T. (2017). The temperature response of leaf dark respiration in 15 provenances of Eucalyptus grandis grown in ambient and elevated CO2. Funct. Plant Biol. 44, 1075–1086. doi: 10.1071/FP17110
Aspinwall, M. J., Loik, M. E., Resco de Dios, V., Tjoelker, M. G., Payton, P. R., and Tissue, D. T. (2015). Utilizing intraspecific variation in phenotypic plasticity to bolster agricultural and forest productivity under climate change. Plant Cell Environ. 38, 1752–1764. doi: 10.1111/pce.12424
Atwell, B. J., Henery, M. L., Rogers, G. S., Seneweera, S. P., Treadwell, M., and Conroy, J. P. (2007). Canopy development and hydraulic function in eucalyptus tereticornis grown in drought in CO2-enriched atmospheres. Funct. Plant Biol. 34, 1137–1149. doi: 10.1071/FP06338
Ayub, G., Smith, R. A., Tissue, D. T., and Atkin, O. K. (2011). Impacts of drought on leaf respiration in darkness and light in eucalyptus saligna exposed to industrial-age atmospheric CO2 and growth temperature. New Phytol. 190, 1003–1018. doi: 10.1111/j.1469-8137.2011.03673.x
Bachofen, C., Moser, B., Hoch, G., Ghazoul, J., and Wohlgemuth, T. (2018). No carbon “bet hedging” in pine seedlings under prolonged summer drought and elevated CO2. J. Ecol. 106, 31–46. doi: 10.1111/1365-2745.12822
Bamber, R. K., and Humphreys, F. R. (1963). A preliminary study of some wood properties of Eucalyptus grandis (Hill) Maiden. J. Inst. Wood Sci. 11, 63–70.
Battaglia, M., and Bruce, J. (2017). Direct climate change impacts on growth and drought risk in blue gum (Eucalyptus globulus) plantations in Australia. Aust. For. 80, 216–227. doi: 10.1080/00049158.2017.1365403
Battie-Laclau, P., Laclau, J.-P., Beri, C., Mietton, L., Almeida Muniz, M. R., Arenque, B. C., et al. (2013). Photosynthetic and anatomical responses of Eucalyptus grandis leaves to potassium and sodium supply in a field experiment. Plant Cell Environ. 37, 70–81. doi: 10.1111/pce.12131
Battie-Laclau, P., Laclau, J.-P., Domec, J.-C., Christina, M., Bouillet, J.-P., de Cassia Piccolo, M., et al. (2014). Effects of potassium and sodium supply on drought-adaptive mechanisms in Eucalyptus grandis plantations. New Phytol. 203, 401–413. doi: 10.1111/nph.12810
Binkley, D., Campoe, O. C., Alvares, C., Carniero, R. L., Cegatta, I., and Stape, J. L. (2017). The interactions of climate, spacing and genetics on clonal eucalyptus plantations across Brazil and Uruguay. For. Ecol. Managem. 405, 271–283. doi: 10.1016/j.foreco.2017.09.050
Blackman, C. J., Aspinwall, M. J., Resco de Dios, V., Smith, R. A., and Tissue, D. T. (2016). Leaf photosynthetic, economic and hydraulic traits are decoupled among genotypes of a widespread species of eucalypt grown under ambient and elevated CO2. Funct. Ecol. 30, 1491–1500. doi: 10.1111/1365-2435.12661
Booth, T. H. (2013). Eucalypt plantations and climate change. For. Ecol. Manage. 301, 28–34. doi: 10.1016/j.foreco.2012.04.004
Booth, T. H., Broadhurst, L. M., Pinkard, E., Prober, S. M., Dillon, S. K., Bush, D., et al. (2015). Native forests and climate change: Lessons from eucalypts. For. Ecol. Manage. 347, 18–29. doi: 10.1016/j.foreco.2015.03.002
Calderia, D. R. M., Alvares, C. A., Campoe, O. C., Hakamada, R. E., Guerrini, I. A., Cegatta, I. R., et al. (2020). Multisite evaluation of the 3-PG model for the highest phenotypic plasticity Eucalyptus clone in Brazil. For. Ecol. Manage. 462:117989. doi: 10.1016/j.foreco.2020.117989
Cantin, D., Tremblay, M. F., Lechowicz, M. J., and Potvin, C. (1997). Effects of CO2 enrichment, elevated temperature, and nitrogen availability on the growth and gas exchange of different families of jack pine seedlings. Can. J. For. Res. 27, 510–520. doi: 10.1139/x96-221
Cavalli, J. P., Reichert, J. M., Rodrigues, M. F., and de Araújo, E. F. (2020). Composition and functional soil properties of arenosols and acrisols: effects on eucalyptus growth and productivity. Soil Till. Res. 196:104439. doi: 10.1016/j.still.2019.104439
Ceulemans, R., Shao, B. Y., Jiang, X. N., and Kalina, J. (1996). First-and second-year aboveground growth and productivity of two Populus hybrids grown at ambient and elevated CO2. Tree Physiol. 16, 61–68. doi: 10.1093/treephys/16.1-2.61
Cornelius, J. (1994). The effectiveness of plus-tree selection for yield. For. Ecol. Manage. 67, 23–34. doi: 10.1016/0378-1127(94)90004-3
Correia, I., Almeida, M. H., Aguiar, A., Alía, R., David, T. S., and Pereira, J. S. (2008). Variations in growth, survival and carbon isotope composition (δ13C) among Pinus pinaster populations of different geographic origins. Tree Physiol. 28, 1545–1552. doi: 10.1093/treephys/28.10.1545
Crous, K. Y., Zaragoza-Castells, J., Ellsworth, D. S., Duursma, R. A., Loew, M., Tissue, D. T., et al. (2012). Light inhibition of leaf respiration in field-grown eucalyptus saligna in whole-tree chambers under elevated atmospheric CO2 and summer drought. Plant Cell Environ. 35, 966–981. doi: 10.1111/j.1365-3040.2011.02465.x
Curtis, P. S. (1996). A meta-analysis of leaf gas exchange and nitrogen in trees grown under elevated carbon dioxide. Plant Cell Environ. 19, 127–137. doi: 10.1111/j.1365-3040.1996.tb00234.x
De Kauwe, M. G., Medlyn, B. E., Zaehle, S., Walker, A. P., Dietze, C. D., Wang, Y.-P., et al. (2014). Where does all the carbon go? A model-data intercomparison of vegetation carbon allocation and turnover processes at two temperate forest free-air CO2 enrichment sites. New Phytol. 203, 883–899. doi: 10.1111/nph.12847
Dekker, S. C., Groenendijk, M., Booth, B. B., Huntingford, C., and Cox, P. M. (2016). Spatial and temporal variations in plant water use efficiency inferred from tree-ring, eddy covariance and atmospheric observations. Earth Syst. Dynam. Discuss.7, 525–533. doi: 10.5194/esd-7-525-2016
Dickson, R. E., Coleman, M., Riemenschneider, D. E., Isebrands, J. G., Hogan, G., and Karnosky, D. F. (1998). Growth of five hybrid poplar genotypes exposed to interacting elevated CO2 and O3. Can. J. For. Res. 28, 1706–1716. doi: 10.1139/x98-150
Drake, B. G., Gonzàlez-Meler, M. A., and Long, S. P. (1997). More efficient plants: a consequence of rising atmospheric CO2? Annu. Rev. Plant Biol. 48, 609–639. doi: 10.1146/annurev.arplant.48.1.609
Drake, J. E., Aspinwall, M. J., Pfautsch, S., Rymer, P. D., Reich, P. B., Smith, R. A., et al. (2015). The capacity to cope with climate warming declines with from temperate to tropical latitudes in two widely distributed Eucalyptus species. Glob. Change Biol. 21, 459–472. doi: 10.1111/gcb.12729
Drake, J. E., Gallet-Budynek, A., Hofmockel, K. S., Bernhardt, E. S., Billings, S. A., Jackson, R. B., et al. (2011). Increases in the flux of carbon belowground stimulate nitrogen uptake and sustain the long-term enhancement of forest productivity under elevated CO2. Ecol. Lett. 14, 349–357. doi: 10.1111/j.1461-0248.2011.01593.x
Duan, H., Amthor, J. S., Duursma, R. A., O'Grady, A. P., Choat, B., and Tissue, D. T. (2013). Carbon dynamics of eucalypt seedlings exposed to progressive drought in elevated [CO2] and elevated temperature. Tree Physiol. 33, 779–792. doi: 10.1093/treephys/tpt061
Duff, G. A., Berryman, C. A., and Eamus, D. (1994). Growth, biomass allocation and foliar nutrient contents of two Eucalyptus species of the wet-dry tropics of Australia grown under CO2 enrichment. Funct. Ecol. 8, 502–508. doi: 10.2307/2390075
Dusenge, M. E., Duarte, A. G., and Way, D. A. (2019). Plant carbon metabolism and climate change: elevated CO2 and temperature impacts on photosynthesis, photorespiration and respiration. New Phytol. 221, 32–49. doi: 10.1111/nph.15283
Ellsworth, D. S., Anderson, I. C., Crous, K. Y., Cooke, J., Drake, J. E., Gherlenda, A. N., et al. (2017). Elevated CO2 does not increase eucalypt forest productivity on a low-phosphorus soil. Nat. Clim. Change 7, 279–282. doi: 10.1038/nclimate3235
Erskine, P. D., Lamb, D., and Bristow, M. (2006). Tree species diversity and ecosystem function: can tropical multi-diversity plantations generate greater productivity. For. Ecol. Manage. 233, 2005–2210. doi: 10.1016/j.foreco.2006.05.013
Farquhar, G. D., Ehleringer, J. R., and Hubick, K. T. (1989). Carbon isotope discrimination and photosynthesis. Ann. Rev. Plant Physiol. Plant. Mol. Biol. 40, 503–537. doi: 10.1146/annurev.pp.40.060189.002443
Farquhar, G. D., O'Leary, M. H., and Berry, J. A. (1982). On the relationship between carbon isotope discrimination and the intercellular carbon dioxide concentration in leaves. Funct. Plant Biol. 9, 121–137. doi: 10.1071/PP9820121
Farquhar, G. D., and Richards, R. A. (1984). Isotopic composition of plant carbon correlates with water-use efficiency of wheat genotypes. Funct. Plant Biol. 11, 539–552. doi: 10.1071/PP9840539
Finzi, A. C., Norby, R. J., Calfapietra, C., Gallet-Budynek, A., Gielen, B., Holmes, W. E., et al. (2007). Increases in nitrogen uptake rather than nitrogen-use efficiency support higher rates of temperate forest productivity under elevated CO2. Proc. Natl. Acad. Sci. U.S.A. 104, 14014–14019. doi: 10.1073/pnas.0706518104
Fox, J., and Weisberg, S. (2019). An R Companion to Applied Regression, 3rd edn. Thousand Oaks, CA: Sage.
Frank, D. C., Poulter, B., Saurer, M., Esper, J., Huntingford, C., Helle, G., et al. (2015). Water-use efficiency and transpiration across European forests during the Anthropocene. Nat. Clim. Change 5, 579–583. doi: 10.1038/nclimate2614
Gauthier, P. P. G., Crous, K. Y., Ayub, G., Duan, H., Weerasinghe, L. K., Ellsworth, D. S., et al. (2014). Drought increases heat tolerance of leaf respiration in Eucalyptus globulus saplings grown under both ambient and elevated atmospheric [CO2] and temperature. J. Exp. Bot. 65, 6471–6485. doi: 10.1093/jxb/eru367
Ghannoum, O., Phillips, N. G., Conroy, J. P., Smith, R. A., Attard, R. D., Woodfield, R., et al. (2010). Exposure to preindustrial, current and future atmospheric CO2 and temperature differentially affects growth and photosynthesis in Eucalyptus. Glob. Change Biol. 16, 303–319. doi: 10.1111/j.1365-2486.2009.02003.x
Gifford, R. M. (2003). Plant respiration in productivity models: conceptualization, representation and issues for global terrestrial carbon-cycle research. Funct. Plant Biol. 30, 171–186. doi: 10.1071/FP02083
Grassi, G., Meir, P., Cromer, R., Tompkins, D., and Jarvis, P. G. (2002). Photosynthetic parameters in seedlings of eucalyptus grandis as affected by rate of nitrogen supply. Plant Cell Environ. 25, 1677–1688. doi: 10.1046/j.1365-3040.2002.00946.x
Grote, R., Gessler, A., Hommel, R., Poschenrieder, W., and Priesack, E. (2016). Importance of tree height and social position for drought-related stress on tree growth and mortality. Trees 30, 1467–1482. doi: 10.1007/s00468-016-1446-x
Harwood, C. (2011). “New introductions-doing it right,” in Developing a Eucalypt Resource: Learning From Australia and Elsewhere: University of Canterbury, ed J. Walker (Christchurch: Wood Technology Research Centre), 43–54.
Hättenschwiler, S., and Körner, C. (1997). Biomass allocation and canopy development in spruce model ecosystems under elevated CO2 and increased N deposition. Oecologia 113, 104–114. doi: 10.1007/s004420050358
Hedges, L. V., Gurevitch, J., and Curtis, P. S. (1999). The meta-analysis of response ratios in experimental ecology. Ecology 80, 1150–1156. doi: 10.1890/0012-9658(1999)080[1150:TMAORR]2.0.CO;2
Isebrands, J. G., McDonald, E. P., Kruger, E., Hendrey, G., Percy, K., Pregitzer, K., et al. (2001). Growth responses of Populus tremuloides clones to interacting elevated carbon dioxide and tropospheric ozone. Environ. Pollut. 115, 359–371. doi: 10.1016/S0269-7491(01)00227-5
Iverson, L., Prasad, A., and Matthews, S. (2008). Modeling potential climate change impacts on the trees of the northeastern United States. Mitig. Adapt. Strateg. Glob. Change 13, 487–516. doi: 10.1007/s11027-007-9129-y
Josephs, E. B. (2018). Determining the evolutionary forces shaping G × E. New Phytol. 219, 31–36. doi: 10.1111/nph.15103
Kaluthota, S., Pearce, D. W., Evans, L. M., Letts, M. G., Whitham, T. G., and Rood, S. B. (2015). Higher photosynthetic capacity from higher latitude: foliar characteristics and gas exchange of southern, central and northern populations of Populus angustifolia. Tree Physiol. 35, 936–948. doi: 10.1093/treephys/tpv069
Keenan, T. F., Hollinger, D. Y., Bohrer, G., Dragoni, D., Munger, J. W., Schmid, H. P., et al. (2013). Increase in forest water-use efficiency as atmospheric carbon dioxide concentrations rise. Nature 499, 324–327. doi: 10.1038/nature12291
Killi, D., Bussotti, F., Gottardini, E., Pollastrini, M., Mori, J., Tani, C., et al. (2018). Photosynthetic and morphological responses of oak species to temperature and [CO2] increased to levels predicted for 2050. Urb. Forest. Urb. Green. 31, 26–37. doi: 10.1016/j.ufug.2018.01.012
Lavigne, M. B. (1996). Comparing stem respiration and growth of jack pine provenances from northern and southern locations. Tree Physiol. 16, 847–852.
Lawson, J. R., Fryirs, K. A., and Leishman, M. R. (2017). Interactive effects of waterlogging and atmospheric CO2 concentration on gas exchange, growth and functional traits of Australian riparian tree seedlings. Ecohydrology 10:e1803. doi: 10.1002/eco.1803
Leakey, A. D., Bishop, K. A., and Ainsworth, E. A. (2012). A multi-biome gap in understanding of crop and ecosystem responses to elevated CO2. Curr. Opin. Plant. Biol. 15, 228–236. doi: 10.1016/j.pbi.2012.01.009
Li, X., Zhang, G., Sun, B., Zhang, S., Zhang, Y., Liao, Y., et al. (2013). Stimulated leaf dark respiration in tomato in an elevated carbon dioxide atmosphere. Sci. Rep. 3:3433. doi: 10.1038/srep03433
Liberloo, M., Dillen, S. Y., Calfapietra, C., Marinari, S., Luo, Z. B., De Angelis, P., et al. (2005). Elevated CO2 concentration, fertilization and their interaction: growth stimulation in a short-rotation poplar coppice (EUROFACE). Tree Physiol. 25, 179–189. doi: 10.1093/treephys/25.2.179
Loik, M. E., Dios, V. R., de Smith, R., and Tissue, D. T. (2017). Relationships between climate of origin and photosynthetic responses to an episodic heatwave depend on growth CO2 concentration for Eucalyptus camaldulensis var. camaldulensis. Funct. Plant Biol. 44, 1053–1062. doi: 10.1071/FP17077
Long, S. P., Ainsworth, E. A., Rogers, A., and Ort, D. R. (2004). Rising atmospheric carbon dioxide: plants face the future. Annu. Rev. Plant Biol. 55, 591–628. doi: 10.1146/annurev.arplant.55.031903.141610
Matheson, A. C., and Raymond, C. A. (1986). A review of provenance × environment interaction: its practical importance and use with particular reference to the tropics. Commonwealth For. Rev. 65, 283–302.
McCarthy, H. R., Oren, R., Finzi, A. C., Ellsworth, D. S., Kim, H.-S., Johnsen, K. H., et al. (2007). Temporal dynamics and spatial variability in the enhancement of canopy leaf area under elevated atmospheric CO2. Glob. Change Biol. 13, 2479–2497. doi: 10.1111/j.1365-2486.2007.01455.x
Medlyn, B. E., Barton, C. V. M., Broadmeadow, M. S. J., Ceulmans, R., De Angelis, P., Forstreuter, M., et al. (2001). Stomatal conductance of forest species after long-term exposure to elevated CO2 concentration: a synthesis. New Phytol. 149, 247–264. doi: 10.1046/j.1469-8137.2001.00028.x
Melville, R. (1940). Intergrading among plants in relation to the provenance of forest trees. Nature 145, 130–132. doi: 10.1038/145130a0
Mohan, J. E., Clark, J. S., and Schlesinger, W. H. (2004). Genetic variation in germination, growth, and survivorship of red maple in response to sub-ambient through elevated atmospheric CO2. Glob. Change Biol. 10, 233–247. doi: 10.1046/j.1365-2486.2004.00726.x
Moore, B. D., Cheng, S.-H., Sims, D., and Seemann, J. R. (1999). The biochemical and molecular basis for photosynthetic acclimation to elevated CO2. Plant Cell Environ. 22, 567–582. doi: 10.1046/j.1365-3040.1999.00432.x
Moran, E. V., Hartig, F., and Bell, D. M. (2016). Intraspecific trait variation across scales: implications for understanding global change responses. Glob. Change Biol. 22, 137–150. doi: 10.1111/gcb.13000
Müller da Silva, P. H., Brune, A., Alvares, C. A., do Amaral, W., de Moares, M. L. T., Grattapaglia, D., et al. (2019). Selecting for stable and productive families of Eucalyptus urophylla across a country wide range of climates in Brazil. Can. J. For. Res. 49, 87–95. doi: 10.1139/cjfr-2018-0052
Norby, R. J., and Zak, D. R. (2011). Ecological lessons from free-air CO2 enrichment (FACE) experiments. Annu. Rev. Ecol. Evol. Syst. 42, 181–203. doi: 10.1146/annurev-ecolsys-102209-144647
O'Leary, M. H. (1981). Carbon isotope fractionation in plants. Phytochem. 20, 553–567. doi: 10.1016/0031-9422(81)85134-5
Oleksyn, J., Modrzýnski, J., Tjoelker, M. G., Zytkowiak, R., Reich, P. B., and Karolewski, P. (1998). Growth and physiology of Picea abies populations from elevational transects: common garden evidence for altitudinal ecotypes and cold adaptation. Funct. Ecol. 12, 573–590. doi: 10.1046/j.1365-2435.1998.00236.x
Pan, Y., Jiang, L., Xu, G., Li, J., Wang, B., Li, Y., et al. (2020). Evaluation and selection analyses of 60 Larix kaempferi clones in four provenances based on growth traits and wood properties. Tree Genet. Genom. 16:27. doi: 10.1007/s11295-020-1420-z
Poorter, H., Niinemets, Ü., Poorter, L., Wright, I. J., and Villar, R. (2009). Causes and consequences of variation in leaf mass per area (LMA): a meta-analysis. New Phytol. 182, 565–588. doi: 10.1111/j.1469-8137.2009.02830.x
Pritchard, S. G., Strand, A. E., McCormack, M. L., Davis, M. A., Finzi, A. C., Jackson, R. B., et al. (2008). Fine root dynamics in a loblolly pine forest are influenced by free-air-CO2-enrichment: a six-year-minirhizotron study. Glob. Change Biol. 14, 588–602. doi: 10.1111/j.1365-2486.2007.01523.x
Pyakurel, A., and Wang, J. R. (2014). Interactive effects of elevated [CO2] and soil water stress on leaf morphological and anatomical characteristics of paper birch populations. Am. J. Plant Sci. 5, 691–703. doi: 10.4236/ajps,.2014.55084
R Core Team (2019). R: A Language and Environment for Statistical Computing. R Foundation for Statistical Computing, Vienna.
Reich, P. B., Hobbie, S. E., and Lee, T. D. (2014). Plant growth enhancement by elevated CO2 eliminated by joint water and nitrogen limitation. Nature Geosci. 7, 920–924. doi: 10.1038/ngeo2284
Resco de Dios, V., Mereed, T. E., Ferrio, J. P., Tissue, D. T., and Voltas, J. (2016). Intraspecific variation in juvenile tree growth under elevated CO2 alone and with O3: a meta-analysis. Tree Physiol. 36, 682–693. doi: 10.1093/treephys/tpw026
Rose, L., Leuschner, C., Köckemann, B., and Buschmann, H. (2009). Are marginal beech (Fagus sylvatica L.) provenances a source for drought tolerant ecotypes? Eur. J. For. Res. 128, 335–343. doi: 10.1007/s10342-009-0268-4
Saxe, H., Ellsworth, D. S., and Heath, J. (1998). Tree and forest functioning in an enriched CO2 atmosphere. New Phytol. 139, 395–436. doi: 10.1046/j.1469-8137.1998.00221.x
Schmidt, S., Palacio, S., and Hoch, G. (2017). Growth reduction after defoliation is independent of CO2 supply in deciduous and evergreen young oaks. New Phytol. 214, 1479–1490. doi: 10.1111/nph.14484
Smith, R. A., Lewis, J. D., Ghannoum, O., and Tissue, D. T. (2012). Leaf structural responses to pre-industrial, current and elevated atmospheric [CO2] and temperature affect leaf function in Eucalyptus sideroxylon. Funct. Plant Biol. 39, 285–296. doi: 10.1071/FP11238
Souza, J. P., Melo, N. M., Pereira, E. G., Halfeld, A. D., Gomes, I. N., and Prado, C. H. B. (2016). Responses of woody Cerrado species to rising atmospheric CO2 concentration and water stress: gains and losses. Funct. Plant Biol. 43, 1183–1193. doi: 10.1071/FP16138
Streit, K., Siegwolf, R. T. W., Hagedorn, F., Schaub, M., and Buchmann, N. (2014). Lack of photosynthetic or stomatal regulation after nine years of elevated [CO2] and four years of soil warming in two conifer species at the alpine treeline. Plant Cell Environ. 37, 315–326. doi: 10.1111/pce.12197
Tissue, D. T., Lewis, J. D., Wullschleger, S. D., Amthor, J. S., Griffin, K. L., and Anderson, O. R. (2002). Leaf respiration at different canopy positions in sweetgum (Liquidambar styraciflua) grown in ambient and elevated concentrations of carbon dioxide in the field. Tree Physiol. 22, 1157–1166. doi: 10.1093/treephys/22.15-16.1157
Tissue, D. T., and Wright, S. J. (1995). Effect of seasonal water availability on phenology and the annual shoot carbohydrate cycle of tropical forest shrubs. Funct. Ecol. 9, 518–527. doi: 10.2307/2390018
Tjoelker, M. G., Oleksyn, J., Lorenc-Plucinska, G., and Reich, P. B. (2009). Acclimation of respiratory temperature responses in northern and southern populations of Pinus banksiana. New Phytol. 181, 218–229. doi: 10.1111/j.1469-8137.2008.02624.x
Viera, M., and Rodríguez-Saolliero, R. (2019). A complete assessment of carbon stocks in above and belowground biomass components of a hybrid eucalyptus plantation in Southern Brazil. Forests 10:536. doi: 10.3390/f10070536
Wang, S., Littell, R. C., and Rockwood, D. L. (1984). Variation in density and moisture content of wood and bark among twenty Eucalyptus grandis progenies. Wood Sci. Technol. 18, 97–102.
Wang, T., O'Neill, G. A., and Aitken, S. N. (2010). Integrating environmental and genetic effects to predict responses of tree populations to climate. Ecol. Appl. 20, 153–163. doi: 10.1890/08-2257.1
Wang, X., Lewis, J. D., Tissue, D. T., Seemann, J. R., and Griffin, K. L. (2001). Effects of elevated atmospheric CO2 concentration on leaf dark respiration of Xanthium strumarium in light and in darkness. Proc. Natl. Acad. Sci. U.S.A. 98, 2479–2484. doi: 10.1073/pnas.051622998
Way, D. A., Oren, R., and Kroner, Y. (2015). The space-time continuum: the effects of elevated CO2 and temperature on trees and the importance of scaling. Plant Cell Environ. 38, 991–1007. doi: 10.1111/pce.12527
Wong, S. C., Kriedemann, P. E., and Farquhar, G. D. (1992). CO2 × nitrogen interaction on seedling growth of four species of eucalypt. Aust. J. Bot. 40, 457–472. doi: 10.1071/BT9920457
Keywords: forestry, tree growth, climate change, gas exchange, carbohydrates, phenotypic plasticity, interspecific variation
Citation: Wesolowski A, Blackman CJ, Smith RA, Tissue DT and Pfautsch S (2020) Elevated CO2 Did Not Stimulate Stem Growth in 11 Provenances of a Globally Important Hardwood Plantation Species. Front. For. Glob. Change 3:66. doi: 10.3389/ffgc.2020.00066
Received: 06 December 2019; Accepted: 05 May 2020;
Published: 04 June 2020.
Edited by:
Heather R. McCarthy, University of Oklahoma, United StatesReviewed by:
Cate Macinnis-Ng, The University of Auckland, New ZealandCameron Ducayet McIntire, University of New Mexico, United States
Ismael Aranda García, Instituto Nacional de Investigación y Tecnología Agraria y Alimentaria (INIA), Spain
Copyright © 2020 Wesolowski, Blackman, Smith, Tissue and Pfautsch. This is an open-access article distributed under the terms of the Creative Commons Attribution License (CC BY). The use, distribution or reproduction in other forums is permitted, provided the original author(s) and the copyright owner(s) are credited and that the original publication in this journal is cited, in accordance with accepted academic practice. No use, distribution or reproduction is permitted which does not comply with these terms.
*Correspondence: Sebastian Pfautsch, Uy5QZmF1dHNjaEB3ZXN0ZXJuc3lkbmV5LmVkdS5hdQ==