- 1Carbone Boréal, Département des Sciences Fondamentales, Université du Québec à Chicoutimi, Chicoutimi, QC, Canada
- 2Laboratoire d’Écologie Végétale et Animale, Département des Sciences Fondamentales, Université du Québec à Chicoutimi, Chicoutimi, QC, Canada
- 3Canadian Wood Fibre Centre, Natural Resources Canada, Québec, QC, Canada
- 4Science and Technology Branch, Environment and Climate Change Canada, Montréal, QC, Canada
- 5Ouranos, Montréal, QC, Canada
- 6Département de Biologie, Université de Sherbrooke, Sherbrooke, QC, Canada
- 7Direction de la Recherche Forestière, Ministère des Forêts, de la Faune et des Parcs du Québec, Québec, QC, Canada
- 8Environmental NMR Centre and Department of Physical and Environmental Sciences, University of Toronto Scarborough, Toronto, ON, Canada
The increase in soil organic matter mineralization rate in boreal forests that may result from global warming is a major concern as it could release large amounts of C to the atmosphere. On the other hand, this may also release N to the soil and stimulate tree growth, which could partly offset the C losses from the soil. The long-term interaction between increased N deposition and soil warming on soil organic N mineralization and tree growth is still uncertain in the boreal zone. In this study, soil temperature was increased by +2–4°C from April to July with heating cables for a period of 9 years and N was applied above the canopy of mature stands from June to September for 7–9 years in two eastern Canada boreal forests [Bernatchez (BER) and Simoncouche (SIM)]. We assessed the effects of these treatments on organic N mineralization rate and on the growth, phenology and foliar N of Abies balsamea (L.) Mill. (balsam fir; BF) and Picea mariana (Mill.) BSP (black spruce; BS) seedlings. The soil warming (SW) treatment had no significant effect on N mineralization rates, whereas canopy N addition (CNA) decreased gross N mineralization rate by 23% and forest floor’s alkyl/O-alkyl C ratio by ∼15% relative to unfertilized plots. Foliar δ15N in the control plots was markedly lower in BS than in BF and at BER than at SIM (–4.8 and –2.9‰ in BS at BER and SIM, respectively; 1.6 and 3.8‰ in BF at BER and SIM, respectively) likely due to a higher contribution of 15N-depleted N derived from mycorrhizal fungi in BS and at BER, the colder and the more N-depleted site. The treatments had non-significant effects on seedling growth and foliar chemistry but SW caused a premature bud outbreak and faster bud development for both species at both sites. Overall, our results show that increased soil temperature and N deposition in boreal forests may not impact soil fertility and vegetation growth as much as previously thought, but climate warming, by initiating earlier and faster bud development, may however expose seedlings to late spring frosts in the future.
Introduction
Nitrogen mineralization is crucial in determining soil fertility and forest productivity because plant N nutrition largely relies upon NH4+, especially in cold ecosystems such as taiga and boreal forests (Schimel and Chapin, 1996; Aerts and Chapin, 2000; McFarland et al., 2002; Persson et al., 2003; Schimel and Bennett, 2004; Shenoy et al., 2013), although several plants of these habitats can absorb small organic-N compounds such as amino acids (Persson and Näsholm, 2001; McFarland et al., 2002; Persson et al., 2003). Since the mineralization of organic N is an enzymatic process and that N can be a limiting factor for soil microbial activity, it is thought that increased N deposition and soil temperature will affect soil OM decomposition and N mineralization (D’Orangeville et al., 2014). A recent meta-analysis compiling 528 observations from 51 articles reports that, on average, experimental warming treatments increased N mineralization rate by 52% although the direction and the magnitude of the effect strongly vary among ecosystems and warming methods and duration (Bai et al., 2013). Similarly, several studies have shown that experimental N addition impacts soil microorganisms, enzymatic activity, and reduces litter or soil OM decomposition (Allison et al., 2008; Frey et al., 2014; Pisani et al., 2015), but the direction and the magnitude of the effect depend on the level of experimental N inputs relative to natural rates of N deposition (Knorr et al., 2005). It is expected that increased soil temperature and changes in N cycling will particularly impact boreal forests because these habitats are characterized by low temperatures, low N availability and high C:N ratio litter (Marty et al., 2017). These changes may increase N availability with subsequent effects on forest regeneration and growth (Hobbie et al., 2002; D’Orangeville et al., 2013a; Blaško et al., 2015). It is important to anticipate the impact of these perturbations on boreal forests because these ecosystems are a major C store at the global scale and provide many services to human societies (e.g., timber and food production, wildlife refuge) (Dixon et al., 1994; Batjes, 1996; Chapin et al., 2004; Lal, 2005). Significant shifts in tree species performances (e.g., survival, regeneration, photosynthetic and growth rates) under a warmer climate may, for instance, impact forest productivity and require adapting silvicultural and management practices (e.g., assisted migration) (Benomar et al., 2018; Otis Prud’Homme et al., 2018).
In Quebec, Canada, the mean annual air temperature (MAAT) has increased by 1–3°C between 1950 and 2011 and is expected to rise by another 2.5–10°C in its northern ecosystems by 2080, depending on the representative concentration pathway (RCP) scenarios (Ouranos, 2015). These increases in air temperature will likely increase soil temperature by up to 2°C by the middle of the century and result in earlier snowmelt (Houle et al., 2012). Large decreasing trends in nitrate deposition have been observed throughout North America (Ackerman et al., 2018; Zhang et al., 2018) and Europe (Vuorenmaa et al., 2018) in the last decades as a response of emission regulations, particularly in remote areas such as boreal forests (Gilliam et al., 2019). The deposition of ammonium has however remained stable and now represents about 50% of total N atmospheric inputs in eastern Canadian boreal forests (Houle et al., 2015). Although the long-term effects of soil warming and N addition on soil processes and OM composition have been studied (Pisani et al., 2015; Melillo et al., 2017; Marty et al., 2019a), how these treatments interactively and simultaneously impact both soil N mineralization and tree regeneration is poorly documented for boreal forests. In addition, whether these treatments affect soil processes differently among sites with contrasting temperature and soil OM composition, and how different conifer species respond to these potential changes is still unclear in these habitats.
In this study, two boreal forests of eastern Canada with contrasting mean annual temperature (MAT) and soil characteristics, were subjected to soil warming (SW) for a period of 9 years and canopy N addition (CNA) for 7–9 years in order to assess the effects of projected environmental changes on N mineralization and on the growth and phenology of Abies balsamea (L.) Mill. (balsam fir; BF) and Picea mariana (Mill.) (black spruce; BS) seedlings, two commercially important species in boreal Canada that have contrasting growth dynamics and resource use strategies with respect to environmental conditions (Chen et al., 2019). Large areas of the eastern Canadian boreal forests are managed for timber and wood fibers, which plays a significant economic role both locally and nationally (Natural Resources Canada, 2019). It is therefore insightful to study how planted seedlings react to changes in soil temperature and N addition in order to adapt sylvicultural practices and species or seed sources selection. Both the applied SW treatment (+ 2–4°C during the growing season) and the CNA rate (+0.30–0.35 kg N ha–1 yr–1) were in agreement with regional projections for 2050 (Houle et al., 2012). Seedling growth, phenology, foliar chemistry and δ15N as well as N mineralization rates were measured for each experimental treatment over the eighth and ninth years of the experiment. The goal of this study was to investigate the impact of increased soil temperature and N deposition on N cycling and on seedling growth, phenology and N sources in the two species. We hypothesized that (i) the SW treatment would increase net and gross N mineralization rates; (ii) the CNA treatment would decrease both N mineralization rates and the relative degree of degradation of forest floor OM due to a decrease in soil microorganisms mining activity; and (iii) both soil warming and CNA would stimulate seedling growth and trigger bud break earlier at both sites.
Materials and Methods
Study Sites
The study was carried out in two boreal forest sites located within the balsam fir–paper birch (Betula papyrifera Marsh.) bioclimatic domain of Québec, Canada. The first site (abbreviated SIM) is located within the Simoncouche research station (48° 13′N, 71° 15′W; 350 m asl.) in the Laurentides Wildlife Reserve, Québec, Canada. The second site (abbreviated BER) is located at higher altitude in the Monts-Valin near Lake Bernatchez (48°51′N, 70° 20′W; 611 m asl.). At both sites, the forest is mostly composed of even-aged mature black spruce. Both sites were affected by major wildfires, in 1922 for SIM (De Barba et al., 2016) and around 1865–1870 for BER. The two sites are characterized by thick undifferentiated glacial tills as parental material and slopes ranging between 8 and 17% (Lupi et al., 2012). The soils are Podzols with a mor-humus. The thickness of the organic soil (LFH) averages 10 and 18 cm at SIM and BER, respectively (Rossi et al., 2013). In the LFH, total N concentration and C:N ratio respectively averaged 8.5 g kg–1 and 50.2 at BER, and 9.7 g kg–1 and 39.1 at SIM. Similar low pH values of 2.5–3.6 were observed at both sites (Lupi et al., 2012).
The climate at both sites is continental, with long cold winters and short cool summers. Mean annual air temperature averages 0.2 and 1.9°C at BER and SIM, respectively (Rossi et al., 2011a), whereas the mean air temperature during the growing season (from May to September) is 11.4°C at BER and 13.3°C at SIM (Rossi et al., 2011a). Snow cover lasts from November to May and complete snowmelt occurs 20 days earlier at SIM [day of year (DOY): 120] than at BER (DOY: 140) (De Barba et al., 2016). Maximum snow cover reaches 132 and 108 cm at BER and SIM, respectively (Rossi et al., 2011b). The growing season at BER is ∼15 days shorter than at SIM (Boulouf Lugo et al., 2012).
Experimental Design
The field experiment combined soil warming (SW) and canopy N addition (CNA) and was conducted for a period of 9 years between 2008 and 2016. Twelve 7.5 m × 7.5 m square plots were delimited within a square area of 60 m × 60 m and distributed across three blocks at each site. Four treatments were randomly assigned to these plots in a split-plot design: warming with N addition (WN), warming with no N addition (W), no warming with N addition (N), and no warming and no N addition (C).
Sprinklers were installed in each experimental plot types (N, W, WN, and C) above the canopy of one selected tree located within each plot in order to simulate N deposition. Once a week, during the frost-safe period (June to September), the equivalent of 2 mm of rainfall was applied over a circular area of 3 m radius centered on the stem of each selected tree. We used a 15N-enriched ammonium-nitrate solution (NO3NH4; 10% 15N) to irrigate the trees. Nitrogen addition-free plots (W and C) received a solution reproducing the chemical composition of natural rainfall (14.93 μmol L–1 of both NO3– and NH4+), while N-enriched plots (N and WN) received a solution with 44.78 μmol L–1 of both NO3– and NH4+ in order to simulate a threefold increase in inorganic N concentration. This is equivalent to a ∼50% increase in N deposition in forests that naturally receive 0.7–1 kg N ha–1 yr–1 (Rossi et al., 2013). Artificial rainfall was applied from 2008 to 2016 at SIM and from 2008 to 2014 at BER.
The W and WN plots were heated using heating cables at an approximate depth of 15 cm beneath the forest floor (FF) surface. The heating system aimed to simulate a 2–4°C increase in soil temperature. The cables were installed in the fall of 2007, in circles (following a spiral pattern) around the same selected tree’s stem collar, leaving 30 cm between cables’ coils. To account for potential soil disturbance and root damage during cable laying, non-heating cables were installed in the same way in non-heated plots (C and N). Soil warming was conducted from April to July between 2008 and 2016, simulating an early snowmelt and soil thawing. Soil warming started 2 weeks later at BER (higher altitude) than at SIM to reflect the natural difference in temperature between the two sites (Lupi et al., 2012). This treatment resulted in an increased FF temperature by up to 4°C in summer months in warmed plots (W and WN) relative to C plots at SIM (Marty et al., 2019a).
At the beginning of the summer 2015, i.e., after 7 years of treatments, each experimental plot was split in two subplots. Five three-year-old black spruce (BS; Picea mariana) seedlings were planted in monoculture in one of these subplots and five three-year-old balsam firs (BF; Abies balsamea) seedlings in the other (Supplementary Material 1). Seedlings were planted with their 110 cm3 soil carrot from the tree nursery, where they were grown from local seed sources. The phenology and the growth of the seedlings were monitored during the second year only (the first year was an acclimatization period) before we harvested them at the end of September 2016, i.e., 2 year after they were planted.
Forest Floor Sampling
After 9 years of treatment (October 2016), we collected four cores (depth of 5–10 cm and diameter of 8 cm) from the F horizon (Fibric) of the organic layer (beneath the litter layer) in each of the four experimental plots (C, W, N, and WN) where the seedlings were planted (two soil cores in the portion with BS seedlings and two soil cores in the portion with the BF seedlings) with a hammer drill. We then mixed and homogenized the samples (4 treatments × 3 blocks × 2 sites = 24 samples) in polyethylene bags that were taken to the lab and kept at 4°C in the dark for 5 months until preparation for analyses. Forest floor samples were then dried at 55°C until reaching a constant weight, ground to fine powder and sent to the lab for analyses.
Forest Floor Chemical Analyses
Subsamples of the air-dry forest floor were analyzed for remaining humidity, organic matter (OM) by loss on ignition, pH (soil:water 1:2.5 ratio wt/vol), and total C and N determination by dry combustion (LECO CR-412, LECO Corporation, St-Joseph, MI, United States). Total P, K, Ca, Mg, Mn, Cu, Zn, Al, and Fe concentrations were analyzed by digestion in concentrated H2SO4 (Parkinson and Allen, 1975) and total S by digestion in hot concentrated HNO3; measurements were made by inductively coupled plasma emission spectrophotometry (ICP-AES).
Solid-State 13C Nuclear Magnetic Resonance (NMR) Analysis
Solid-state 13C NMR analyses were performed as described in Marty et al. (2019a). We packed ∼250 mg of dried and ground soil subsamples into 4 mm zirconium rotors and sealed with a Kel-F cap. Solid-state 13C cross polarization magic angle spinning (CP-MAS) spectra were measured using a 500 MHz Bruker BioSpin Avance III spectrometer having a 4 mm H-X MAS probe. A MAS rate of 11 kHz was used with a 1 ms ramp-CP contact time and a 1 s recycle delay (Conte et al., 2004). NMR spectra were processed using a zero-filling factor of 2 and line broadening of 50 Hz. Spectra were baseline-corrected manually and phased using TopSpin (v3.5). NMR spectra were integrated into four main regions using TopSpin (v3.5), which included: alkyl C (0–50 ppm); O-alkyl C (50–110 ppm), aromatic and phenolic C (110–165 ppm) and carboxylic and carbonyl C (165–210 ppm) (Preston et al., 1997). Alkyl C to O-alkyl C ratios were calculated to compare the relative stage of degradation between samples (Baldock et al., 1992).
Net N Mineralization
Net N mineralization rates were assessed during the 8th and 9th years of treatments using the buried bag incubations technique (Hart et al., 1994). In each plot, a sample was taken from the F horizon (fibric), manually sorted (i.e., rocks, cones, branches, fresh leaves, and coarse roots were removed) and homogenized. A subsample (∼5 g of dry weight) was taken for NH4+ extraction (see below). The remaining sample was put in a closed polyethylene bag (∼0.030 mm thick) and buried under the surface (L) horizon. The bag was left in situ and collected about 30 days later to extract NH4+ (time t). The same procedure was repeated for each plot six times from May to late October in 2015 and five times from May to late September in 2016. In addition, one incubation of about 210 days (from late October 2015 to late May 2016) was carried out to cover the winter period.
Soil NH4+ extractions were conducted as follows: soil subsamples (∼5 g of dry weight) were mixed with 100 ml of 2 M KCl in glass bottles and shaken (∼130 rpm) for 60 min. All soil extracts were then filtered (No. 1 filter paper; Whatman Inc., Little Chalfont, United Kingdom) and filtered extracts were stored at –19°C until analysis. Ammonium concentrations from the filtered extracts were determined by colorimetry following the nitroprusside-hypochlorite-salicylate method (Mulvaney, 1996) using a Technicon AutoAnalyzer II (Pulse Instrumentation Ltd., Saskatoon, CAN) in 2015 and a QuikChem 8500 (Lachat Instruments, Milwaukee, United States) in 2016. The net N mineralization rate was then calculated for each incubation period as follows (Hart et al., 1994):
where mN is the net N mineralization rate (in mg N-NH4+ kg–1 soil day–1), t is the incubation time (in days), [N-NH4+]t0 is the total N-NH4+ concentration (in mg kg–1 soil) at time 0, and [N-NH4+]t is the total N-NH4+ concentration (in mg kg–1 soil) at time t.
Gross N Mineralization
Gross N mineralization rates were estimated during the 8th and 9th years of treatments using the N isotope dilution technique (Davidson et al., 1991; Hart et al., 1994). Soil samples (150–200 g) were taken from the F horizon, sorted and homogenized as described above, then put in polyethylene bags (∼0.044 mm thick). Using a syringe and a needle 30G1/2 (Becton Dickinson & Co., Franklin Lakes, United States), 4 ml of (15NH4)2SO4 solution (15 mg N L–1 at 98% 15N) was spread uniformly onto the soil sample. The sample was then homogenized and 15 min later, a subsample (∼5 g of dry weight) was transferred into a bottle for NH4+ extraction (time t = 0). The soil bag was closed, buried beneath the L-horizon and the moss layer and left to incubate in situ for about 24 h before another soil subsample was taken for NH4+ extraction (time t). All soil extracts were kept at ∼2°C until filtration. Gravimetric water content was determined like previously and volumetric water content of the F horizon was measured using a GS3 probe (Decagon Devices, Inc., Pullman, United States). The same steps (15N dilution) were repeated twice a year both in 2015 and in 2016 during the soil warming period, i.e., one incubation in June and one in July. These procedures were done 1–2 weeks later at BER than at SIM due to delayed growing season.
Soil extracts were filtered as described previously and stored at –19°C until analysis. A diffusion method was used to measure the atom% 15N as previously described (Davidson et al., 1991; Paré and Bedard-Haughn, 2012). Approximately 0.3 g of magnesium oxide (MgO) was added to a 20 ml subsample of the filtered extract to convert N-NH4+ into N-NH3. Ammonia was then trapped on a 7 mm in diameter paper disk (No. 3; Whatman Inc., Little Chalfont, United Kingdom) previously acidified with 10 μl of 2.5 M KHSO4. The acidified disk was sealed between two Teflon strips (Rona X-Pert; Rona Inc., Boucherville, CAN). The 20 ml subsample, MgO and sealed paper disk were gently shaken (∼45 rpm) in an airtight 60 ml Nalgene bottle (High-Density polyethylene; VWR International, Mississauga, CAN) for 7 days. Thereafter, the paper disk was removed from its Teflon casing, dried (50°C for 1 h) and inserted into a Tin capsule (D1008; Elemental Microanalysis Ltd., Okehampton, United Kingdom). The atom% 15N and N-NH4+ concentration was determined using an elemental analyzer Costech ECS 4010 (Costech Analytical Technologies, Inc., Valencia, United States) coupled to an isotope ratio mass spectrometer Delta V with a ConFlo IV interface (Thermo Fisher Scientific, Bremen, GER). For 2015 samples, N-NH4+ concentration was assessed by colorimetry (Mulvaney, 1996) using a Technicon AutoAnalyzer II (Pulse Instrumentation Ltd., Saskatoon, CAN). Gross N mineralization rate calculated according to Eq. 2 (Hart et al., 1994), respectively:
where mB is the gross N mineralization rates (in mg N-NH4+ kg–1 soil day–1), and APE is the atom% 15N enrichment of a N pool enriched with 15N minus the atom% 15N enrichment of that pool prior to 15N addition.
Seedlings Phenology and Growth
Seedling buds were surveyed throughout the second growing season following planting (2016) between May and late June, twice a week at SIM and once a week at BER. The phenological stages of the buds (0–7; Supplementary Material 2) were determined based on the Numainville and Desponts (2004) procedure. The survey was ended when all trees had reached the last stage of phenological development (stage 7).
Seedling stem diameter at the base and height (from the base to the top of the apical shoot) were measured before planting and at the end of the first and second growing seasons (August 2015 and 2016, respectively) to calculate radial and apical growth rates. The apical shoots of BF seedlings were grazed by herbivores during the first growing season. As a consequence, we show apical shoot growth for BS only. At the end of the second growing season, all seedlings were harvested (4 treatments × 3 blocks × 2 sites × 2 species × 5 seedlings = 240 seedlings). The roots, stems and needles were separated, dried at 65°C for 48 h and weighted.
Foliar Chemistry and δ15N
The foliar biomass of the five BS and BF seedlings per plot (n = 240) was dried at 65°C for 48 h, ground to a fine powder and sent to the lab for analyses. Total N concentrations (g N g–1) were determined through the Kjeldhal digestion method (Kjeltec Tecator 1030). Total P, K, Ca, Mg, Mn, Cu, Zn, Al, Fe, and Mo were determined by ICP-AES after sample digestion with concentrated H2SO4 (Parkinson and Allen, 1975).
Foliar biomass subsamples were pooled in order to obtain one sample for each species in each experimental plot at each site (4 treatments × 3 blocks × 2 sites × 2 species = 48 samples) for δ15N measurements. Analyses were performed at the Centre de recherche en géochimie et géodynamique (GEOTOP; UQÀM, Québec, Montréal). N isotopic ratios were measured using an elemental analyzer in continuous flow mode, coupled to an isotope ratio mass spectrometer (IRMS; Micromass Isoprime Isoprime 100, Cheadle, United Kingdom). Values of δ15N are expressed in ‰ vs. air (± 0.2‰ at 1σ). Raw values were corrected with a calibration line obtained from two reference materials: urea and dogfish tissue (δ15N = −0.22 and +14.36‰, respectively). Internal reference materials were normalized to IAEA-N1, N-2, and N-3 scales for δ15N. A third internal reference material was used to verify the exactitude of the calibration (leucine δ15N = −0.06‰).
Statistical Analyses
Gross mineralization data were log-transformed to meet the assumptions of normality and homogeneity of variance prior to statistical analyses. Net N mineralization data could not be log-transformed because of the presence of several negative values (34 values out of 264). We assessed the impact of soil warming (two levels: “W+” and “W-”), CNA (two levels: “N−” and “N−”), site (two levels: “BER” and “SIM”) and species (two levels: “BF” and “BS”) with blocks as random effect on inorganic N concentrations, N mineralization rates, seedling growth and foliar chemistry by using mixed-model analyses. We used the lmerTest package in R, which uses the Satterthwaite’s degrees of freedom method (Kuznetsova et al., 2017) as in Marty et al. (2019a). A paired t-test was used to assess the impact of the soil warming treatment on foliar δ15N in both N− and N + plots by comparing the foliar δ15N values in W and C plots, and WN and N plots, respectively. Wilcoxon’s rank-sum tests were performed on the mean phenological stage of the buds at each survey date to assess the differences between treatments and species within each site. We performed principal component analyses (PCA) on both forest floor and foliar chemistry by using the ade4 package in R (R Core Team, 2019). For all analyses, P-values ≤0.05 were considered significant.
Results
Soil Chemistry at Initial Conditions
A previous study conducted at the SIM site showed that the presence of warming cables did not affect the soil conditions (Marty et al., 2019a). The control (C) plots can thus be used to characterize the chemical composition of the forest floor at initial conditions. In these C plots, the O-alkyl functional group represented about half of the organic C in the soil samples at both sites (Figure 1). The second most represented functional group was the alkyl C group, which accounted for 23–30% of forest floor organic C. Aromatic and phenolic C together accounted for 18–20%. Finally, carboxylic and carbonyl C represented the smaller fraction of soil C (8–9%).
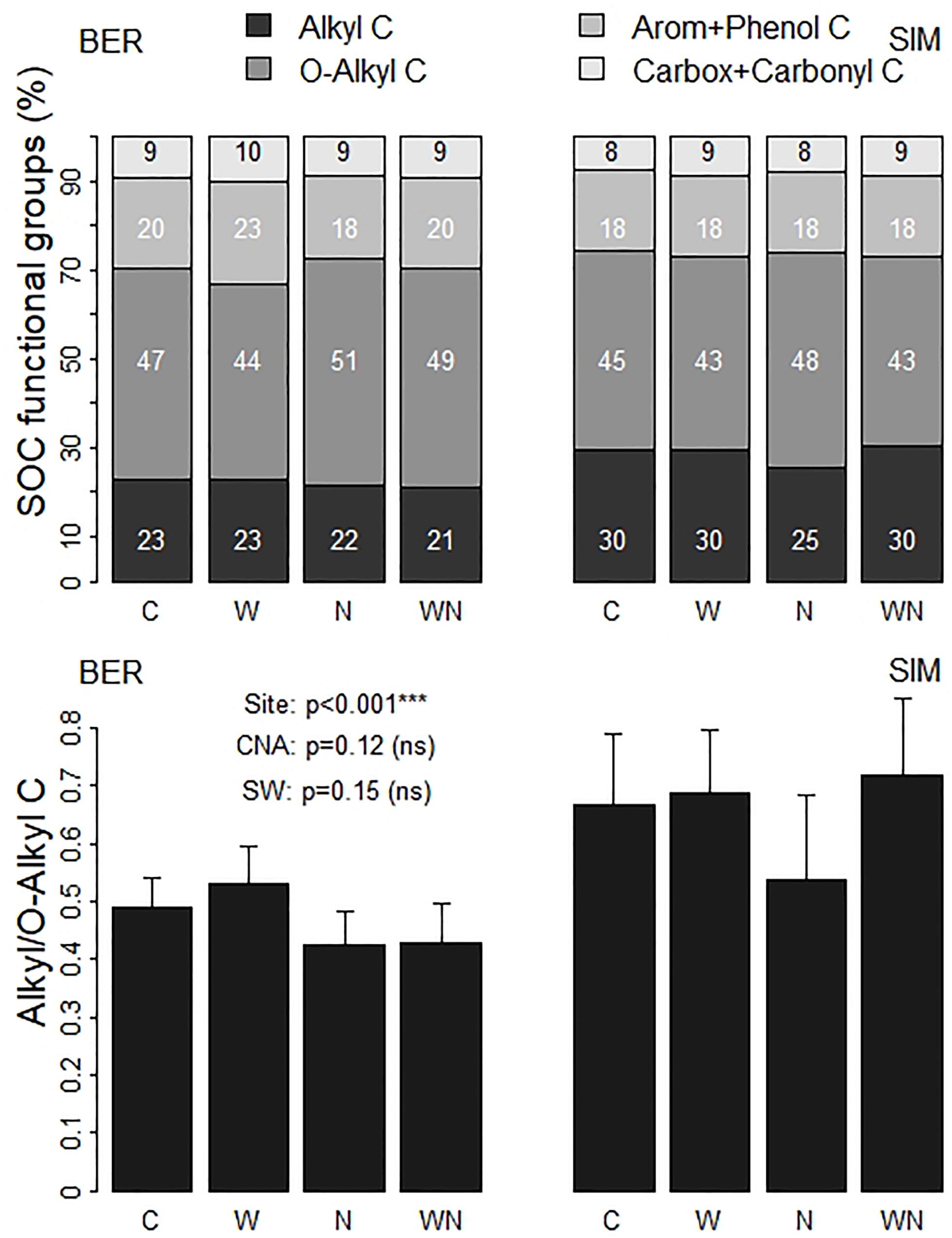
Figure 1. Mean percentages of the four assessed organic C functional groups, and alkyl: O-alkyl C in the C (control), N (fertilized), W (warmed), and WN (warmed and fertilized) plots of both sites at the end of the 9-year experiment. CNA, Canopy N addition; SW, Soil Warming.
The forest floor at BER had a lower proportion of alkyl C as well as a lower alkyl/O-alkyl C ratio, and a slightly higher proportion of aromatic + phenolic C functional groups than at SIM (Figure 1). Forest floor OM content and C:N ratio were higher at BER than at SIM, whereas Cu, Zn, Al, Fe, and N concentrations were much higher at SIM than at BER (Table 1).
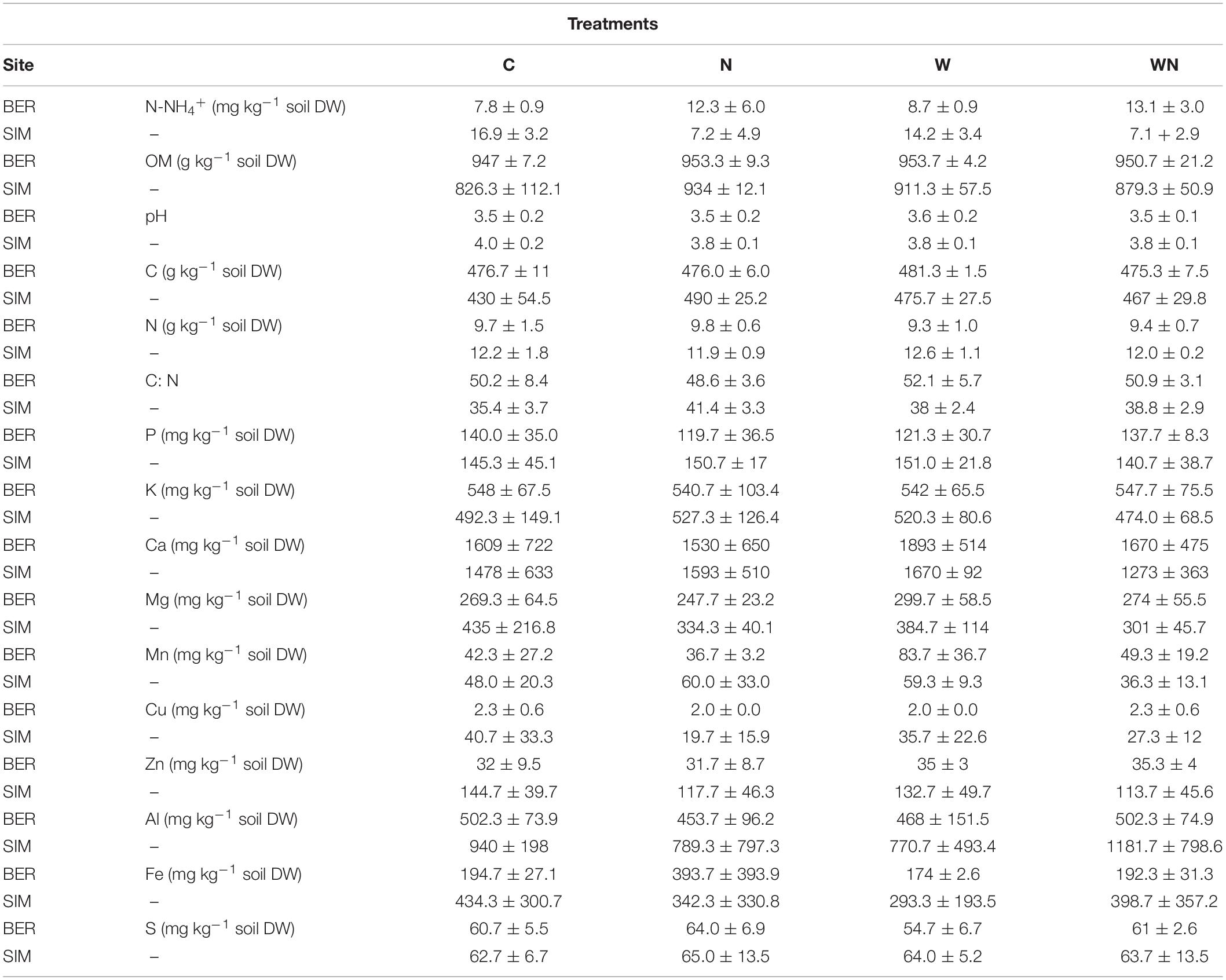
Table 1. Forest floor chemical composition in the C (control), N (fertilized), W (warmed), and WN (warmed and fertilized) plots of both sites at the end of the 9-year experiment.
Effects of the Treatments on Soil Chemistry
The PCA graphical representation (Figure 2A) shows an obvious separation of the two sites on the PC1 axis, all BER samples having negative PC1 values and all but one SIM samples having positive PC1 values. This separation on the PC1 axis reflected the higher OM, Ca, Mn concentrations, as well as higher C:N ratio and contents of carboxyl + carbonyl and aromatic + phenolic functional groups at BER than at SIM (Table 1 and Figure 1). Conversely, SIM samples were more associated with high P, N and Fe concentrations and a higher alkyl/O-alkyl C ratio.
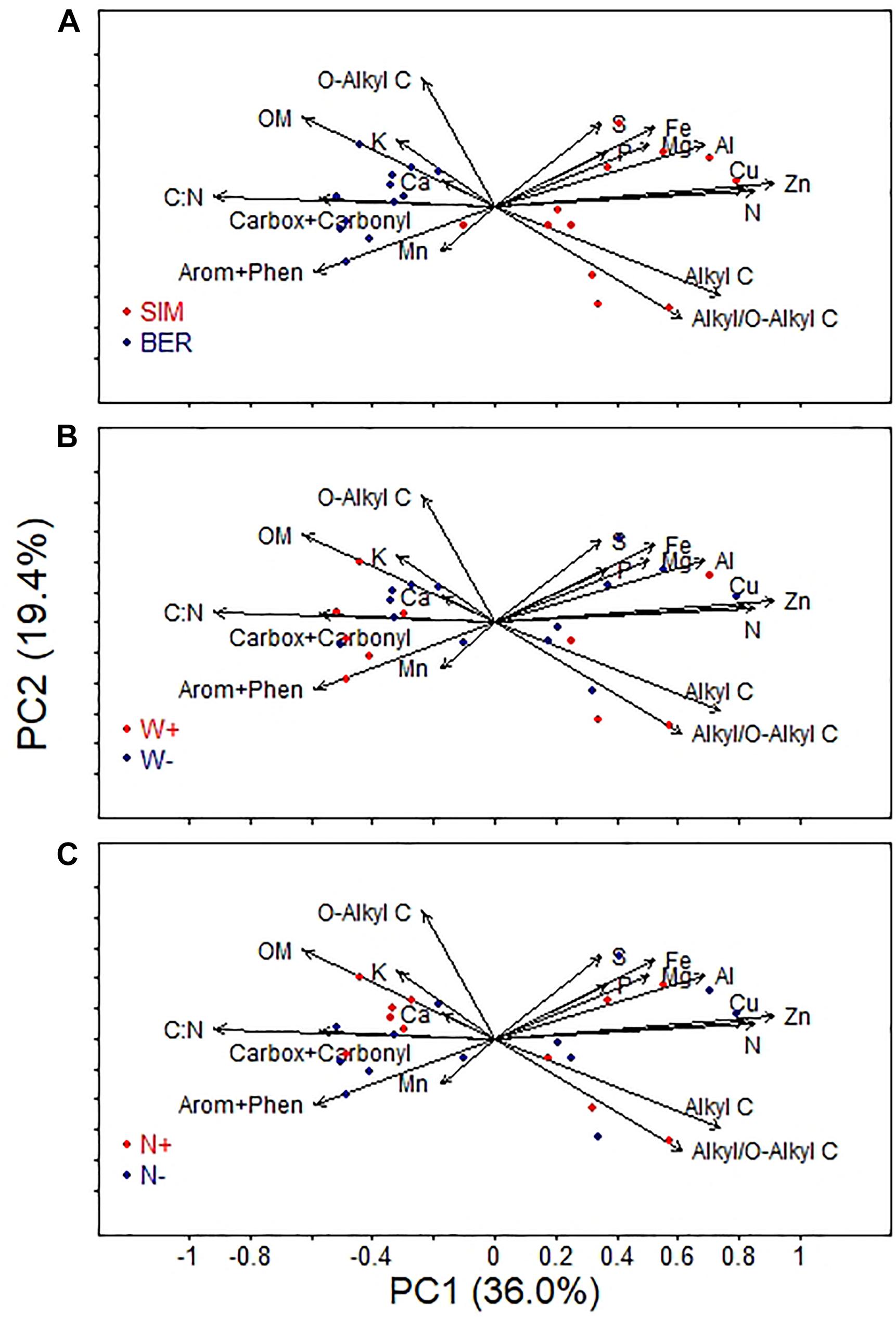
Figure 2. Results of the principal component analysis (PCA). The 18 forest floor chemical variables are projected along with forest floor sample (n = 24) scores as a function of (A) site, (B) soil warming treatment, and (C) canopy N addition treatment.
In contrast, the PCA did not reveal clear differences in forest floor’s chemical characteristics between warmed (W+) and unwarmed (W-) samples (Figure 2B), or between fertilized (N+) and unfertilized (N-) samples (Figure 2C). Although not statistically significant, the alkyl/O-alkyl C ratio was slightly reduced by the N+ treatment relative to control at both sites. At SIM, this ratio was on average 25% lower in the N plots (0.54 ± 0.15) than in the WN plots (0.72 ± 0.13), whereas it was similarly lower in both N and WN plots (0.43) than in the other plots (0.49–0.53) at BER (Figure 1).
Differences in Soil Inorganic N Concentrations Among Sites and Treatments
On May 19 2015, before the first incubation of the soil (buried bag method), N-NH4+ represented about 100% of total soil inorganic N (N-NO3– + N-NH4+) as N-NO3– concentrations were below the detection limit at both sites, which is common in boreal soils. In C plots (i.e., initial conditions), soil N-NH4+ concentration was on average more than twice higher at SIM (16.9 mg kg–1) than at BER (7.8 mg kg–1; Table 1). At SIM, N-NH4+ concentrations were significantly lower in N+ (N and WN plots) than in N- plots (C and W plots; Table 1).
Differences in Net and Gross N Mineralization Rates Among Sites and Treatments
Net N mineralization rate was on average about three times higher at SIM than at BER across experimental treatments (Figure 3A). The CNA and soil warming treatments had no significant effect on net N mineralization rate (Figures 3B,C).
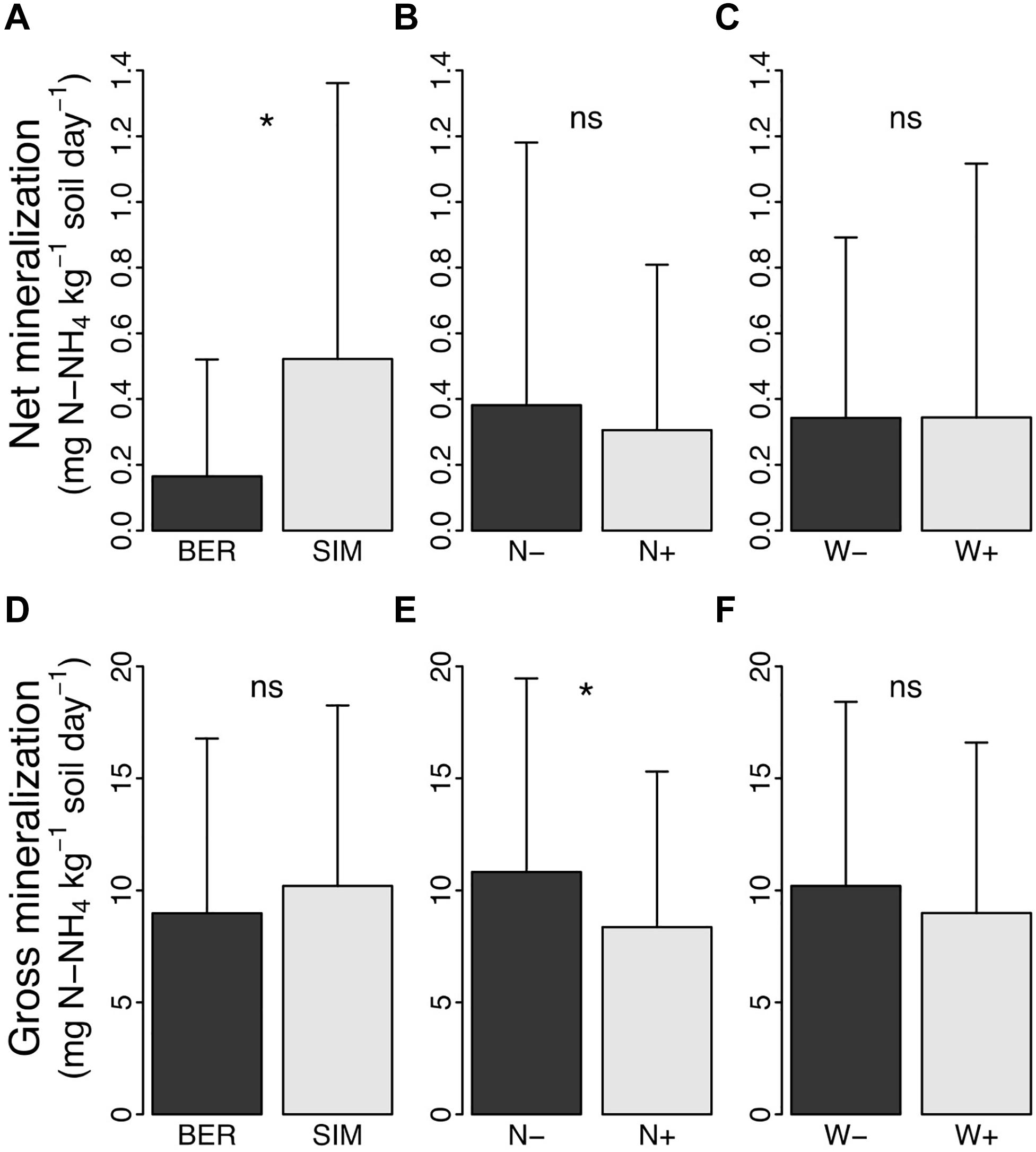
Figure 3. Net and gross mineralization rates (mg N-NH4+ kg–1 soil day–1) in (A–D) BER vs. SIM sites, (B–E) fertilized (N + ) vs. unfertilized (N−) plots and (C–F) warmed (W+) vs. unwarmed (W-) plots. * and ns indicate a significant (P < 0.05) and not significant difference, respectively.
The rate of gross N mineralization was similar at both sites (Figure 3D) but 23% lower in N + than in N− plots (Figure 3E). In contrast, the soil warming treatment had no significant effect on gross nitrogen mineralization rates (Figure 3F).
Seedling Growth
Soil warming and CNA had no effect on BS annual apical shoot growth, which significantly varied only among sites (Table 2). Stem radial growth (mm) was higher at BER than at SIM (Figure 4A) and for BS than for BF (Figure 4B). There was a significant interaction between species and CNA for stem radial growth (Table 2). Stem radial growth was 20% higher in N- than in N + plots for BS but not for BF (Figure 4C). Annual apical shoot growth was significantly higher at BER than at SIM in both 2015 and 2016 (Supplementary Material 3).
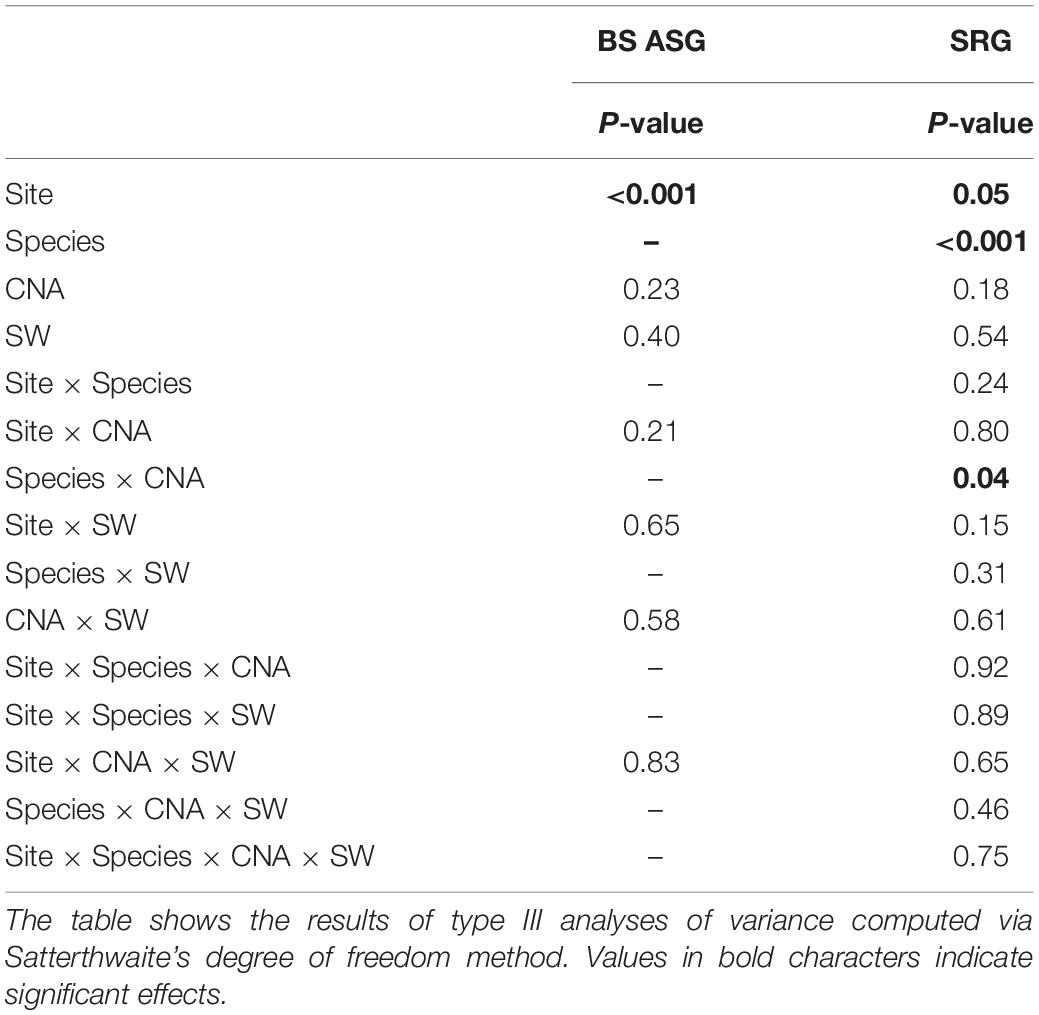
Table 2. Result of the mixed model analysis conducted on black spruce annual shoot growth (BS ASG) and stem radial growth of black spruce and balsam fir (SRG) between June 2015 and August 2016 (two growing seasons) with site, species, canopy N addition (CNA), and soil warming (SW) as fixed effects and blocks as random effect.
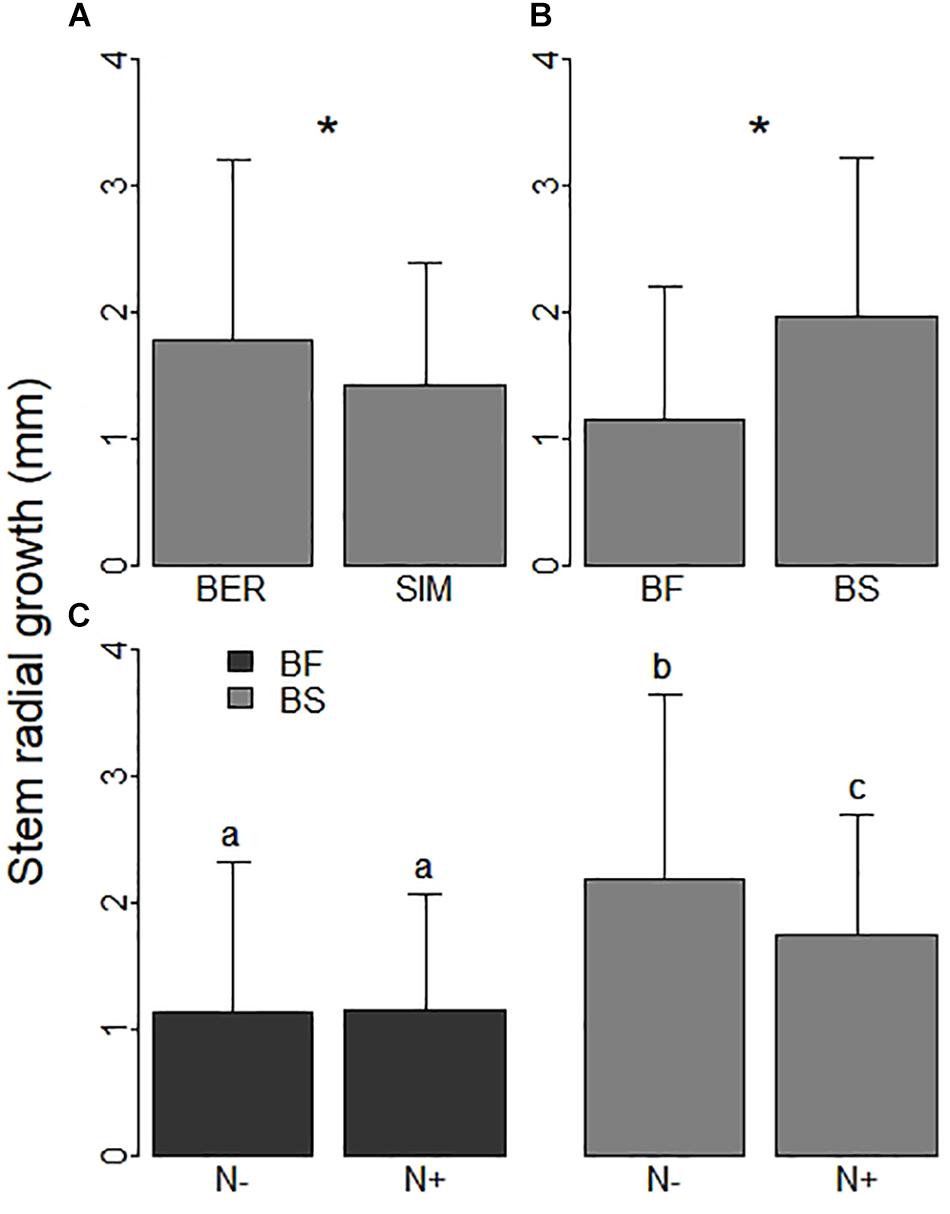
Figure 4. Seedlings’ stem radial growth (mm) (A) at the BER and SIM sites; (B) for balsam fir (BF) and black spruce (BS); and (C) in fertilized (N + ) and unfertilized (N−) plots in both species. * indicates significant differences (P < 0.05). Values not sharing the same letter are significantly different (P < 0.05).
Effects of Site and Treatments on Seedling Growth and Phenology
Seedling budbreak occurred earlier and was more rapid i) at SIM than at BER; and ii) for BF than for BS (Supplementary Material 4). The SW treatment significantly accelerated BF bud development at both sites (Figures 5A,B). The impact was not as pronounced for BS. The effect of CNA was non-significant for both species at BER, although the mean phenological stage was lower in N + than in N− plots at the beginning of the growing season (Figure 5C). In contrast, CNA decreased BS bud development rate at SIM, particularly at the very beginning and at the end of the growing season (Figure 5D).
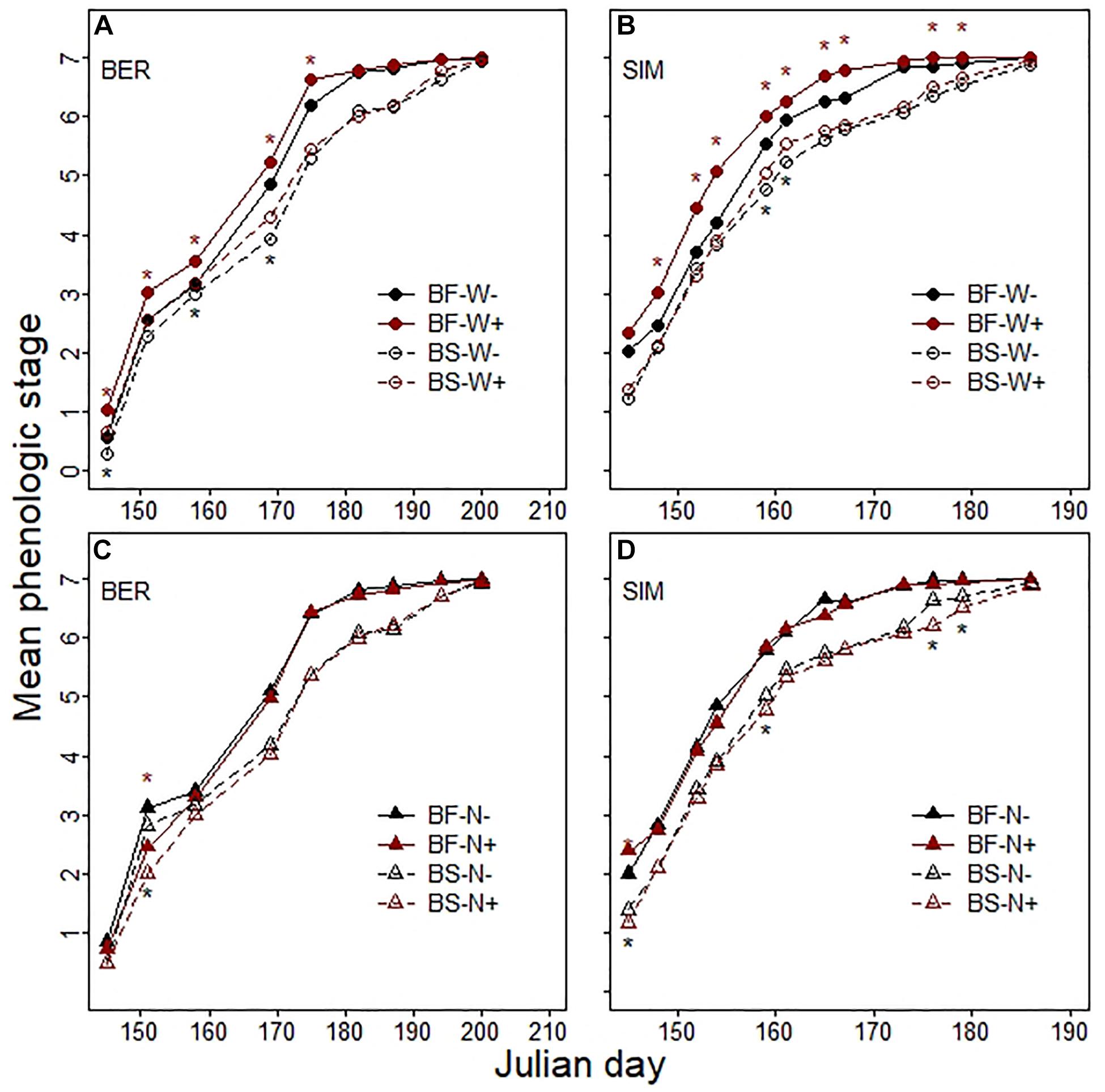
Figure 5. Change in the mean phenologic stage for balsam fir (BF) and black spruce (BS) in (A,B) warmed (W+) and unwarmed (W-) plots and (C,D) in fertilized (N+) and unfertilized (N-) plots across the 2016 growing season at the BER and SIM sites. * indicate significant differences between treatments for BF (red symbols) and BS (black symbols) (Wilcoxon’s rank-sum test; P < 0.05).
Seedling Foliar Chemistry and δ15N
The PCA shows a contrast in foliar chemical composition between the two sites (Figure 6A) and seedling species (Figure 6D), reflecting the significant effects of these variables on most elements (Table 3). BF seedlings were associated with P and N concentrations on the PC1 axis (Figure 6D), reflecting higher foliar N and P concentrations in BF than in BS (Table 4). All other nutrients were more concentrated in BS than in BF (Table 4). Foliar concentrations of N, P, K, and Fe were significantly higher at SIM than at BER. The N + and N− plots (Figure 6B) as well as the SW- and SW+ plots were scattered through the graphical representation of the PCA (Figures 6B,C), reflecting the absence of CNA and SW effects on foliar chemistry (Table 4).
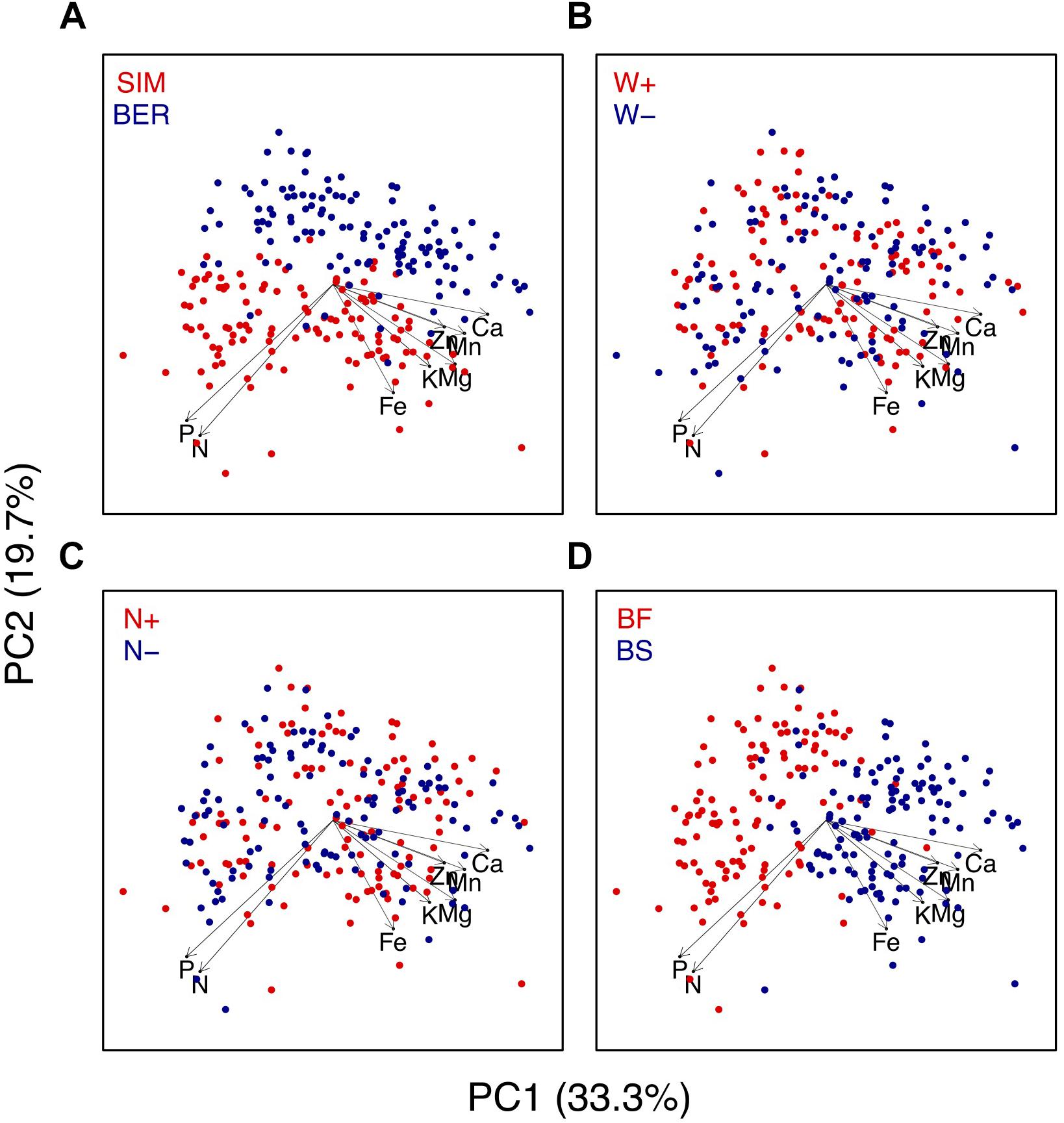
Figure 6. Results of the principal component analysis (PCA). The seven foliar chemical variables (elemental concentrations) are projected along with foliage sample (n = 240) scores as a function of (A) site, (B) soil warming treatment, (C) canopy N addition treatment, and (D) species.
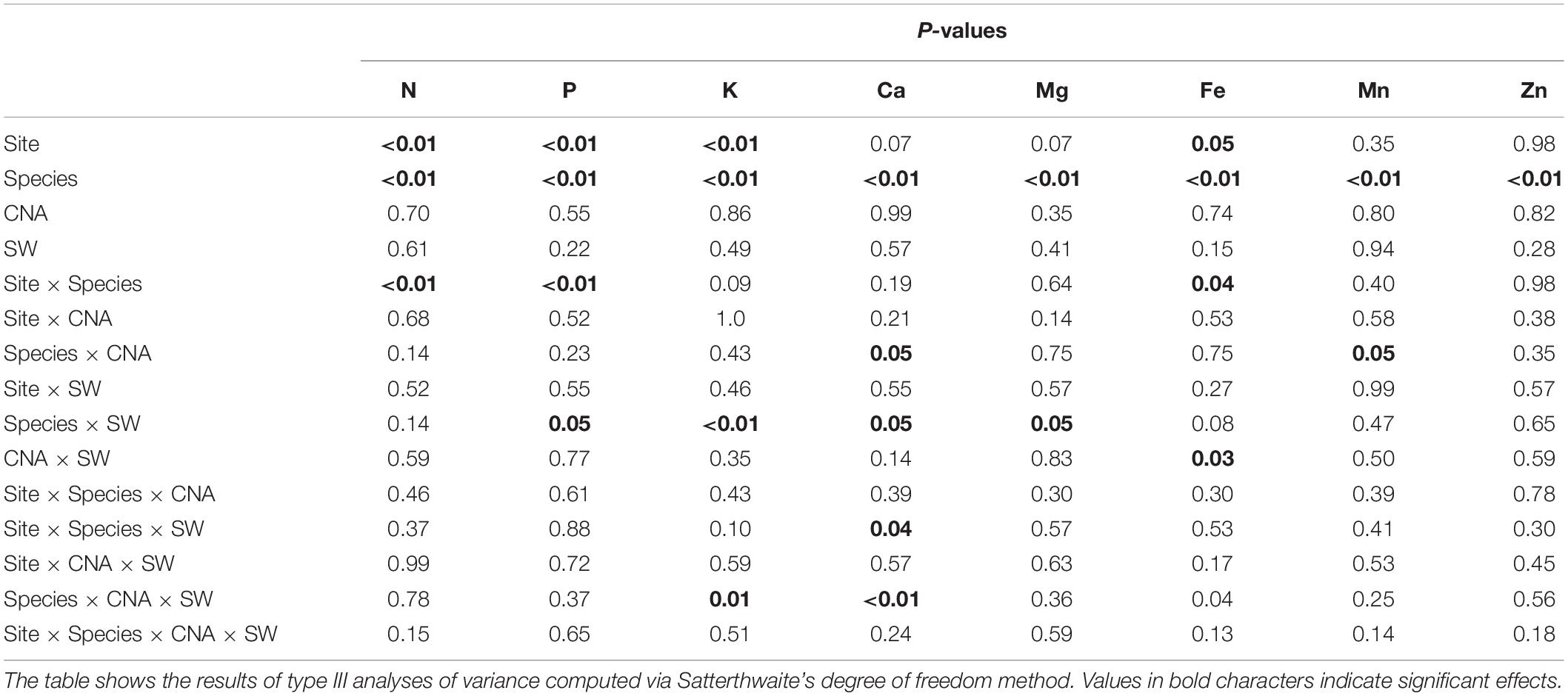
Table 3. Result of the mixed model analysis conducted on seedlings’ foliar chemistry with site, species, canopy N addition (CNA) and soil warming (SW) as fixed effects and blocks as random effect.
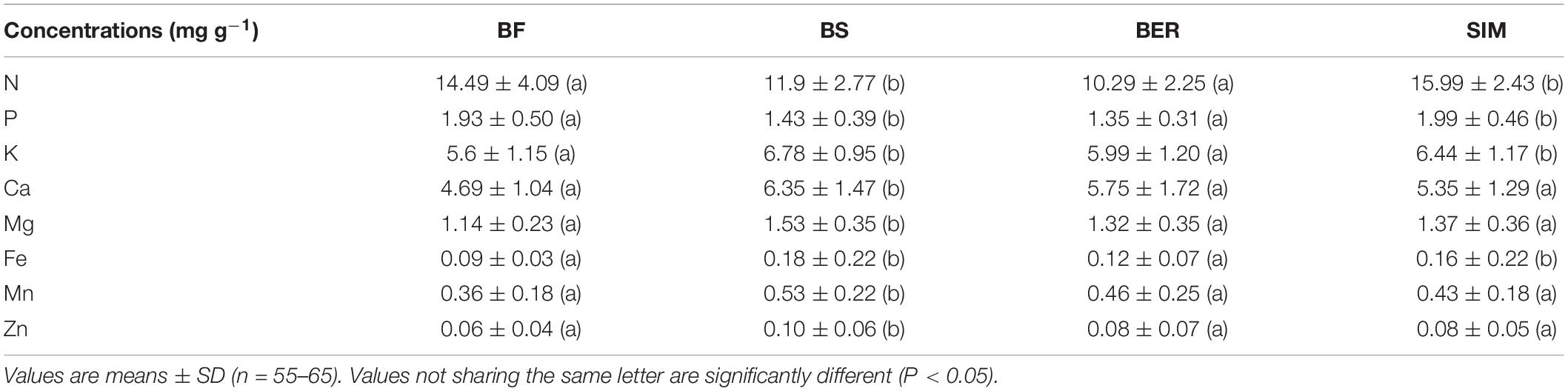
Table 4. Seedlings’ foliar chemistry for balsam fir (BF) and black spruce (BF) at the BER and SIM sites.
BS and BF seedlings from the C plots had distinct foliar N isotopic signatures. δ15N values were markedly higher in BF (range from 0.2 to 4.9‰) than in BS (range from –5.3 to –2.6‰) at both sites (Figure 7). Foliar δ15N was also higher at SIM than at BER for both species, reflecting the higher δ15N in the forest floor at SIM (7.6‰) than at BER (0.8‰). The foliar δ15N average values were similar to those of the seedlings prior to planting for both species (+3.3 and –2.8‰ for BF and BS, respectively) at SIM, and markedly lower at BER (decrease of 1.7 and 2.0‰ for BF and BS, respectively). Foliar δ15N appeared slightly higher in warmed than in unwarmed plots both in N− and N + treatments (except for BF at SIM in N–) but these differences were not statistically significant (P > 0.05; Table 5).
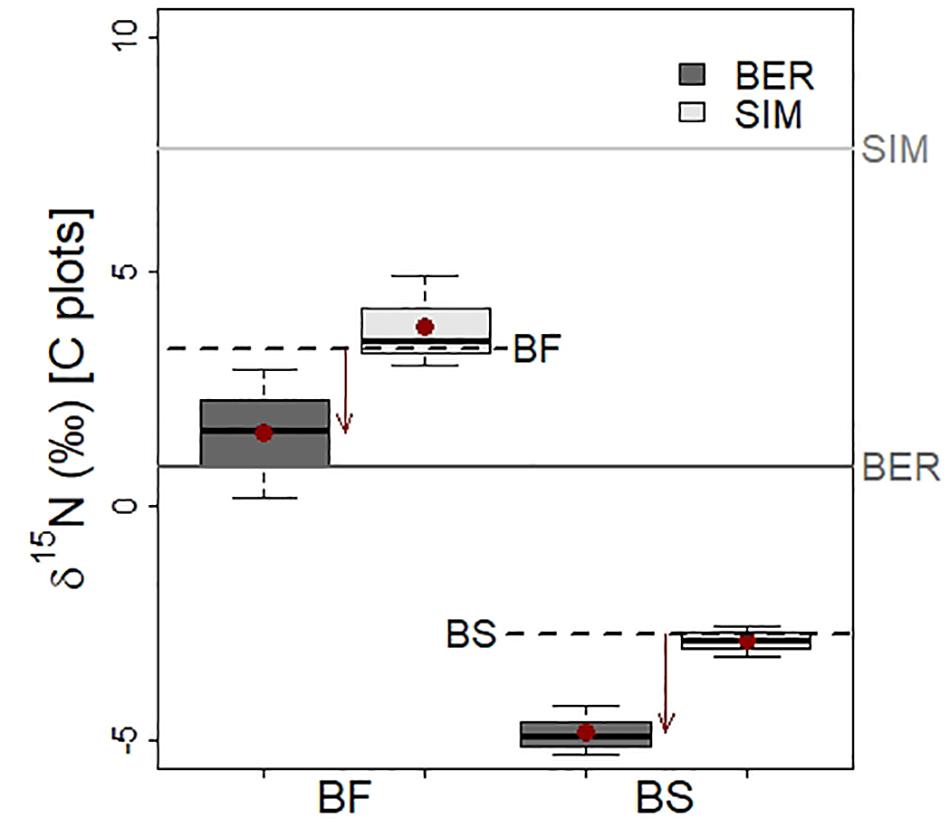
Figure 7. Foliar δ15N (‰) in balsam fir (BF) and black spruce (BS) seedlings harvested from the control plots (no warming and no N addition) 2 years after planting at the BER and SIM sites. The red circles are mean δ15N values and the horizontal dotted lines show the foliar δ15N average values for BF and BS seedlings prior to planting in the field. Horizontal full lines show the average forest floor δ15N values at BER and at SIM. Arrows show the changes in mean foliar δ15N after 2 years in the field.
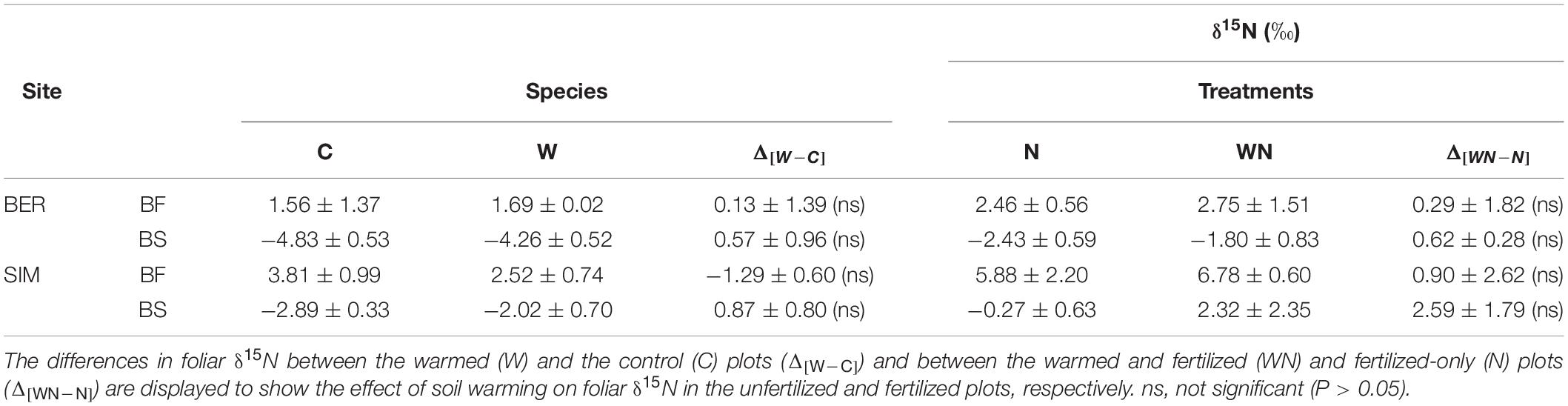
Table 5. Foliar δ15N (‰) (mean ± SD; n = 3) in balsam fir (BF) and black spruce (BS) seedlings in the four experimental plots at the BER and SIM sites.
Discussion
Soil Inorganic N at the Two Boreal Sites
Although soil organic matter of Canadian temperate and boreal forest soils store large amounts of N (up to 1.2 kg N m–2), particularly in the mineral horizons of the soil (Marty et al., 2017), N is one of the main factors limiting the growth of trees in boreal forests (Schulte-Uebbing and de Vries, 2018), because most of this N is in its organic forms due to low mineralization rates, and thus not directly and readily available to support plant development and growth. The very low NO3– concentrations we measured in the soil of both study sites agree with what is generally observed in boreal forests of the region, where N-NH4+ is the main inorganic N form in the soil solution (Ste-Marie and Houle, 2006). At the beginning of the growing season, NH4+ concentration in the forest floor was more than twice higher at SIM than at BER (Table 1), which is consistent with the higher net N mineralization rate observed at SIM (Figure 3A). These differences were likely due to a higher immobilization rate at BER, as suggested by the similar gross mineralization rates at both sites (Figure 3D) and the much higher soil C:N ratio at BER than at SIM (50.2 vs. 35 at SIM and BER, respectively; Table 1), a condition known to stimulate inorganic N immobilization. The forest floor organic matter at SIM also had a higher alkyl/O-alkyl C ratio (Figure 1C), which is consistent with the generally observed negative relationship between OM C:N ratio and alkyl/O-alkyl C ratio (Simpson and Simpson, 2012). As O-alkyl C corresponds to more labile and easily degraded OM constituents, a higher alkyl/O-alkyl C ratio generally indicates a higher degree of soil OM degradation (Simpson and Simpson, 2012; Marty et al., 2019a). The sometimes large variability in NH4+ concentrations (large standard deviation values; Table 1) sometimes observed within experimental treatments at each site was certainly caused by a variation over time and space in factors controlling OM mineralization, such as soil temperature, soil moisture, resources availability (e.g., labile organic C) as well as microbial activity (Moorhead and Sinsabaugh, 2006).
Effect of N Addition and Soil Warming on Soil N
In contrast with our hypothesis, the CNA treatment did not impact soil NH4+ concentrations and net N mineralization rates. This absence of effect agrees with a meta-analysis reporting an overall negligible effect of N addition on litter decomposition (Knorr et al., 2005) but contrasts with other studies reporting either a decrease (Berg and Matzner, 1997; Magill and Aber, 1998; Craine et al., 2007; Frey et al., 2014) or an increase (Aber et al., 1998; Knorr et al., 2005; Contosta et al., 2011; Ma et al., 2011; Zhang et al., 2012) in soil OM decomposition in response to increased N addition, either directly onto the forest floor or on top of the forest canopy. The absence of effect of CNA in our experimental setting may result from the low absolute amount of N actually added to the soil-plant system. Although we doubled the N concentration in precipitation relative to natural rainfall, our treatment corresponded to a total input of only 0.3–0.5 kg N ha–1 yr–1, which is much lower than in other studies which added up to 50–100 kg N ha–1 yr–1 (Frey et al., 2014; Maaroufi et al., 2015). Such N inputs are nevertheless unrealistic in eastern Canadian boreal forests which receive low and decreasing N deposition rates (Houle et al., 2014). Moreover, a significant fraction of CNA may have been immobilized in the canopy (Dail et al., 2009; Adriaenssens et al., 2012; Fenn et al., 2013; Houle et al., 2014) or in the upper moss layers (Gundale et al., 2011; Houle et al., 2014), and may thus have not reached the sampled forest floor.
Nevertheless, the 23% lower gross N mineralization in N+ plots as compared to plots that received no additional N as well as the higher foliar δ15N in N+ plots (Figure 7) show that at least a fraction of the added N reached the forest floor. A decrease in the N mineralization rate following inorganic N addition is generally ascribed to a decrease in soil microorganism mining effort (Moorhead and Sinsabaugh, 2006; Craine et al., 2007), which preferentially use inorganic N rather than alternative N sources such as organic compounds (Geisseler et al., 2010). This phenomenon is illustrated by an increase in soil OM decomposition with N limitation across a wide range of soil types (Craine et al., 2007). Several studies have also shown that chronic N fertilization reduces soil microbial and fungal biomass, activity and diversity (Lilleskov et al., 2001; Frey et al., 2004, 2014; Maaroufi et al., 2015), but that the effect depends on the amount of N added and on the context. Litter decomposition is for instance inhibited when N inputs are 2–20 times the N deposition rate and when litter quality is low, i.e., high lignin litter (Knorr et al., 2005). Also, inorganic N addition to fresh litter generally stimulates the decomposition of cellulose and soluble compounds, whereas inorganic N addition to stabilized soil OM may reduce microbial decomposition activity (Berg and Matzner, 1997). In early successional boreal forests, N addition has been shown to increase litter mass loss as well as the activity of cellulose- and chitin-degrading enzymes (Allison et al., 2010). In our study, we observed slightly lower alkyl/O-alkyl C ratios with N at both sites relative to the control. The alkyl/O-alkyl C ratio typically increases with soil OM degradation and as such, N addition appears to have suppressed biodegradation at both BER and SIM (Figure 1), which is consistent with the decreased gross mineralization rate associated with the CNA treatment (Figure 3E).
The observed decrease in gross mineralization rate caused by N addition was not accompanied by a significant decrease in net mineralization rate, suggesting that N immobilization by soil microorganisms was also decreased with the same magnitude by the CNA treatment. However, we cannot exclude a methodological bias. Gross mineralization was indeed measured over a short time scale (24 h-long incubation), whereas net mineralization was measured over several incubation periods of 30 days. This may explain a part of the discrepancy between the observed effect of N addition on net and gross mineralization rates.
Contrary to our hypothesis, soil warming did not stimulate net and gross N mineralization rates. This also contrasts with a recent meta-analysis showing that soil warming increases the rate of several variables related to N fluxes, such as net N mineralization, net nitrification, denitrification and N fixation, especially in experiments where the soil was warmed with heating cables (Bai et al., 2013). A recent study also reports a stimulation of microbial activity and cutin degradation by in situ soil warming (Pisani et al., 2015). This also contrasts with a previous study conducted at the SIM site which showed that soil warming had a significant impact on the temperature sensitivity and the basal respiration rate of the forest floor organic C (Marty et al., 2019a). Our results are however in agreement with other studies reporting no significant effect of soil warming on N mineralization rate and inorganic N fluxes in boreal forests. For instance, 3 years of soil warming (+4°C) in a boreal forest of Quebec did not increase soil inorganic N fluxes (Houle et al., 2014) nor tree growth and N status in a mature balsam fir boreal forest (D’Orangeville et al., 2013b). A recent study also reported that soil N mineralization and inorganic N pools in the soil solution were not affected by experimental warming in northern agro-ecosystems of eastern China (Fu et al., 2019). This treatment also did not significantly affect the alkyl/O-alkyl C ratio relative to the control, indicating an absence of significant impact on the degree of soil OM degradation. Several factors may explain the absence of effect of soil warming on N mineralization in the long term. First, a reduction in microbial biomass and/or changes at the individual and community levels, i.e., replacement by a microbial community with lower temperature sensitivity, have been observed following in situ long-term soil warming (Zogg et al., 1997; Luo et al., 2001; Bradford et al., 2008; Craine et al., 2013). At the BER site, Rossi et al. (2013) have for instance observed a reduction in the proportion of EcM fungi in response to increased soil temperature. Second, increased soil temperature may have reduced soil moisture resulting in decreased microbial activity and nutrient diffusion throughout the soil matrix, or enhanced the magnitude and the frequency of freeze/thaw cycles by removing the snow cover earlier in spring. This phenomenon (freeze/thaw cycle) is known to have large detrimental effects on tree N uptake (Templer et al., 2007), nutritional status (Comerford et al., 2013) and growth (Reinmann et al., 2019), as well as on soil organic N mineralization rates (Durán et al., 2016). Interestingly, the combination of soil warming and N addition had a different impact on the alkyl/O-alkyl C ratio depending on the site. The alkyl/O-alkyl C ratio of WN plots was similar to that of the N plots at BER, whereas it was similar to that of the W plots at SIM (Figure 1). This discrepancy is difficult to interpret but shows that specific ecosystem properties (e.g., temperature, soil OM properties, soil microbial communities) influence soil OM stability and may control the net output of N addition and soil warming on soil OM degradation (Schmidt et al., 2011). The greater similarity between WN and N plots at BER corroborates observations from the Harvard Forest, MA, United States after 4 years of similar treatments (Pisani et al., 2015), suggesting a predominant influence of the N treatment at this site, at least over the duration of the study. Given the dynamic nature of soil processes and microbial communities (Melillo et al., 2017), the relative influence of these treatments may change over time, which could explain the difference between the two sites.
Seedling Phenology and Growth
Our data showed an earlier budbreak and faster bud development at SIM than at BER, which is consistent with latitudinal and climate differences between the two sites. We also observed differences between the two seedling species. The phenological development was faster for BF than for BS seedlings at both sites (Supplementary Material 4). The phenology seemed to be decoupled with the growth of seedlings, as our data point toward higher growth rates for BS than for BF and at BER than at SIM.
Despite the higher NH4+ pool and net mineralization rate at SIM, the growth of both BF and BS seedlings was higher at BER, suggesting that the seedlings could not access this N pool at SIM, or that soil net mineralization and NH4+ concentration at the beginning of the growing season did not represent the actual N availability for seedlings in boreal forests where immobilization rates are high. In these systems, N availability may rather be more precisely estimated by the gross mineralization rate as suggested by the positive (but not significant) correlation between the growth mineralization rate and BS radial growth at BER (R2 = 0.30; P = 0.06; Supplementary Material 5) and the coupled decreases in both stem radial growth of BS seedlings (Figure 4C) and gross mineralization consecutive to canopy N addition (Figure 3E).
Our data also suggest that the contribution of ectomycorrhizae (EcM) to seedling N nutrition contributed to the observed growth differences among sites and seedling species. Foliar δ15N was much lower for BS than for BF at both sites, which likely resulted from a different degree of EcM association or different N fertilization treatments in the tree nursery, as their isotopic signatures were already much different prior to planting in the field (–2.7 and 3.3‰ for BS and BF, respectively; Figure 7). The particularly low foliar δ15N of BS as compared to other tree species has been reported in other studies (Marty et al., 2011; Houle et al., 2014) and may result from its high reliance on N derived from EcM fungi (Mayor et al., 2012), which provide trees with 15N-depleted N (Hobbie and Hobbie, 2008; Hobbie et al., 2009; Mayor et al., 2015). The lower foliar δ15N at BER than at SIM for both seedling species is consistent with the much higher forest floor δ15N at SIM (Figure 7). Interestingly, foliar δ15N of both species decreased at BER, whereas it remained unchanged after 2 years in the field at SIM. This indicates the use of a more 15N-depleted N source, such as EcM-derived N, or in larger amount at BER than at SIM. The first hypothesis is consistent with the lower inorganic N availability and organic N mineralization rate as well as the higher C:N ratio at BER, which generally promotes mycorrhizal associations and increases the relative contribution of EcM fungi to trees’ N demand (Hobbie and Ouimette, 2009; Marty et al., 2019b). Thus, the decline in foliar δ15N at BER may have resulted from a rapid infection by EcM fungi and from a greater dependence of seedlings upon N derived from EcM fungi at this site. This N source may explain the higher growth rate of seedlings at BER than at SIM and for BS than for BF. By contrast, the absence of a significant change in foliar δ15N and the lower growth rate at SIM suggests that the seedlings’ root system did not develop enough to access soil N pools (yet larger at SIM than at BER) and that the seedlings mainly have relied on internal recycling of N or N resources contained in the soil carrot to support annual growth. This hypothesis is supported by the lower root biomass at SIM than at BER (Supplementary Material 6). The lower root development at SIM may have been caused by various local factors such as soil texture, moisture or temperature, and likely resulted in the lower growth rate at this site.
Effect of N Addition and Soil Warming on Seedling Growth, Foliar δ15N and Phenology
Our hypothesis that N addition and soil warming would result in higher seedling growth was not supported by the data. Our results also indicate that N addition may not have the same effect on all tree species. Whereas the CNA treatment had no effect on BF seedling stem radial growth, it decreased it by about 20% for BS (Figure 4D). This may be due to the observed decrease in gross mineralization rate consecutive to the CNA treatment. Seedling growth, particularly for BS, may be related more closely to the gross mineralization rate and EcM association than to soil NH4+ concentration in the long term. Although not statistically significant, the soil warming treatment slightly increased BS foliar δ15N in both N + and N− treatments (Table 5), which may be the result of a decrease in the contribution of N derived from EcM to the seedling N nutrition. This hypothesis is consistent with the reported decrease in the proportion of EcM fungi consecutive to soil warming at the BER site (Rossi et al., 2013).
The most significant effect of soil warming was on seedling phenology. We clearly observed an earlier and more rapid budbreak in W+ than in W- plots at both sites and for both seedling species. This likely resulted from a ∼2-week earlier snow melting in warmed plots, which mimicked an earlier initiation of the growing season. This also reflects the commonly reported influence of soil temperature on the triggering of budbreak in trees and confirms the potential of climate warming to impact the phenology of trees (Hugues, 2000; Hannah, 2015). The induction of budbreak earlier in the spring due to climate warming is not without risks for the trees as it exposes them to late frosts (Silvestro et al., 2019).
Conclusion
Our data show the strong stability of the soil in these boreal forests in which N net mineralization rate, soil chemistry and soil OM quality in the forest floor were barely impacted by 9 years of soil warming and canopy N addition. This absence of impact on the soil may explain that the growth and the foliar chemistry of the seedlings were not affected during their 2 first years in the field. The higher growth rate of seedlings at BER—where net N mineralization rate and soil NH4+ pool is lower—suggests that seedlings may not be limited only by inorganic N availability at our sites. Seedling growth may be more tied to the level of EcM association and to gross mineralization rate, particularly for BS. The observed decreased foliar δ15N after 2 years in the field at BER actually points toward a larger use of N derived from EcM fungi at this site. Although not statistically significant, the slightly higher BS foliar δ15N in warmed than in unwarmed plots suggests that this treatment reduced EcM contribution to seedling N nutrition, which corroborates the previously reported decrease in EcM biomass resulting from soil warming at BER. The main effect of soil warming was on seedling phenology. The treatment caused a more precocious and rapid budbreak for both BS and BF at both sites, which confirms the influence of soil temperature and the impact of climate warming on the phenology of trees. Overall, our data showed only a small effect of N addition and soil warming on soil N availability and seedling growth. Climate warming, by initiating earlier and faster bud development, may however expose seedlings to late spring frosts in the future and eventually impact tree growth and forest community establishment and structure.
Data Availability Statement
The raw data supporting the conclusions of this article will be made available by the authors, without undue reservation, to any qualified researcher.
Author Contributions
HM, RB, DH, NT, and MP designed the study. JP and ÉD-C performed field and lab work. MS performed the NMR analyses. CM analyzed the data and drafted the manuscript. MP, HM, RB, DH, NT, RO, and MS reviewed and edited the manuscript. All authors contributed to the article and approved the submitted version.
Funding
This study was supported by funding from the CRSNG/NSERC (Strategic Project Grants), NSERC Discovery Grants, Fonds de Recherche Nature et Technologies du Québec, Ministère des Forêts, de la Faune et des Parcs du Québec, Ouranos, Mitacs, Centre SÈVE, la Fondation UQAC, and Carbone Boréal. MS thanks support from the Canada Research Chairs program.
Conflict of Interest
The authors declare that the research was conducted in the absence of any commercial or financial relationships that could be construed as a potential conflict of interest.
Acknowledgments
We also would like to thank Patrick Nadeau and Claire Fournier for technical assistance, and Xavier Plante and Catherine Tremblay for their help.
Supplementary Material
The Supplementary Material for this article can be found online at: https://www.frontiersin.org/articles/10.3389/ffgc.2020.581363/full#supplementary-material
References
Aber, J., Mcdowell, W., Nadelhoffer, K., Magill, A., Berntson, G., Kamakea, M., et al. (1998). Nitrogen saturation in temperate forest ecosystems: hypotheses revisited. Bioscience 48, 921–934. doi: 10.2307/1313296
Ackerman, D., Millet, D. B., and Chen, X. (2018). Global estimates of inorganic nitrogen deposition across four decades. Glob. Biogeochem. Cycles 33, 100–107. doi: 10.1029/2018GB005990
Adriaenssens, S., Staelens, J., Wuyts, K., Samson, R., Verheyen, K., and Boeckx, P. (2012). Retention of dissolved inorganic nitrogen by foliage and twigs of four temperate tree species. Ecosystems 15, 1093–1107. doi: 10.1007/s10021-012-9568-9565
Aerts, R., and Chapin, F. S. (2000). The mineral nutrition of wild plants revisited: a re-evaluation of processes and patterns. Adv. Ecol. Res. 30, 1–67. doi: 10.1016/s0065-2504(08)60016-1
Allison, S. D., Czimczik, C. I., and Treseder, K. K. (2008). Microbial activity and soil respiration under nitrogen addition in Alaskan boreal forest. Glob. Chang. Biol. 14, 1156–1168. doi: 10.1111/j.1365-2486.2008.01549.x
Allison, S. D., Gartner, T. B., Mack, M. C., McGuire, K., and Treseder, K. (2010). Nitrogen alters carbon dynamics during early succession in boreal forest. Soil Biol. Biochem. 42, 1157–1164. doi: 10.1016/j.soilbio.2010.03.026
Bai, E., Li, S., Xu, W., Li, W., Dai, W., and Jiang, P. (2013). A meta-analysis of experimental warming effects on terrestrial nitrogen pools and dynamics. New Phytol. 199, 441–451. doi: 10.1111/nph.12252
Baldock, J. A., Oades, J. M., Waters, A. G., Peng, X., Vassallo, A. M., and Wilson, M. A. (1992). Aspects of the chemical structure of soil organic materials as revealed by solid-state 13C NMR spectroscopy. Biogeochemistry 6, 1–42. doi: 10.1007/bf02402261
Batjes, N. H. (1996). Total carbon and nitrogen in the soils of the world. Eur. J. Soil Sci. 47, 151–163. doi: 10.1111/j.1365-2389.1996.tb01386.x
Benomar, L., Lamhamedi, M. S., Pepin, S., Rainville, A., Lambert, M. C., Margolis, H. A., et al. (2018). Thermal acclimation of photosynthesis and respiration of southern and northern white spruce seed sources tested along a regional climatic gradient indicates limited potential to cope with temperature warming. Ann. Bot. 121, 443–457. doi: 10.1093/aob/mcx174
Berg, B., and Matzner, E. (1997). Effect of N deposition on decomposition of plant litter and soil organic matter in forest systems. Environ. Rev. 5, 1–25. doi: 10.1139/a96-017
Blaško, R., Holm Bach, L., Yarwood, S. A., Trumbore, S. E., Högberg, P., and Högberg, M. N. (2015). Shifts in soil microbial community structure, nitrogen cycling and the concomitant declining N availability in ageing primary boreal forest ecosystems. Soil Biol. Biochem. 91, 200–211. doi: 10.1016/j.soilbio.2015.08.041
Boulouf Lugo, J., Deslauriers, A., and Rossi, S. (2012). Duration of xylogenesis in black spruce lengthened between 1950 and 2010. Ann. Bot. 110, 1099–1108. doi: 10.1093/aob/mcs175
Bradford, M. A., Davies, C. A., Frey, S. D., Maddox, T. R., Melillo, J. M., Mohan, J. E., et al. (2008). Thermal adaptation of soil microbial respiration to elevated temperature. Ecol. Lett. 11, 1316–1327. doi: 10.1111/j.1461-0248.2008.01251.x
Chapin, F. S., Peterson, G., Berkes, F., Callaghan, T. V., Angelstam, P., Apps, M., et al. (2004). Resilience and vulnerability of northern regions to social and environmental change. Ambio 33, 344–349.
Chen, L., Rossi, S., Deslauriers, A., and Liu, J. (2019). Contrasting strategies of xylem formation between black spruce and balsam fir in Quebec. Canada. Tree Physiol. 39, 747–754. doi: 10.1093/treephys/tpy151
Comerford, D. P., Schaberg, P. G., Templer, P. H., Socci, A. M., Campbell, J. L., and Wallin, K. F. (2013). Influence of experimental snow removal on root and canopy physiology of sugar maple trees in a northern hardwood forest. Oecologia 171, 261–269. doi: 10.1007/s00442-012-2393-x
Conte, P., Spaccini, R., and Piccolo, A. (2004). State of the art of CPMAS 13C-NMR spectroscopy applied to natural organic matter. Prog. Nucl. Magn. Reson. Spectrosc. 44, 215–223. doi: 10.1016/j.pnmrs.2004.02.002
Contosta, A. R., Frey, S. D., and Cooper, A. B. (2011). Seasonal dynamics of soil respiration and N mineralization in chronically warmed and fertilized soils. Ecosphere 2, 1–21.
Craine, J. M., Fierer, N., McLauchlan, K. K., and Elmore, A. J. (2013). Reduction of the temperature sensitivity of soil organic matter decomposition with sustained temperature increase. Biogeochemistry 113, 359–368. doi: 10.1007/s10533-012-9762-9768
Craine, J. M., Morrow, C., and Fierer, N. (2007). Microbial nitrogen limitation increases decomposition. Ecology 88, 2105–2113. doi: 10.1890/06-1847.1
Dail, D. B., Hollinger, D. Y., Davidson, E. A., Fernandez, I., Sievering, H. C., Scott, N. A., et al. (2009). Distribution of nitrogen-15 tracers applied to the canopy of a mature spruce-hemlock stand, Howland, Maine, USA. Oecologia 160, 589–599. doi: 10.1007/s00442-009-1325-x
Davidson, E. A., Hart, S. C., Shankst, C. A., and Firestone, M. K. (1991). Measuring gross nitrogen mineralization, immobilization, and nitrification by 15N isotopic pool dilution in intact soil cores. J. Soil Sci. 42, 335–349. doi: 10.1111/j.1365-2389.1991.tb00413.x
De Barba, D., Rossi, S., Deslauriers, A., and Morin, H. (2016). Effects of soil warming and nitrogen foliar applications on bud burst of black spruce. Trees 30, 87–97. doi: 10.1007/s00468-015-1152-0
Dixon, R. K., Brown, S., Houghton, R. A., Solomon, A. M., Trexler, M. C., and Wisniewski, J. (1994). Carbon pools and flux of global forest ecosystems. Science 263, 185–189. doi: 10.1126/science.263.5144.185
D’Orangeville, L., Côté, B., Houle, D., and Whalen, J. (2013a). Reduced mineralizable carbon in a boreal forest soil after three years of artificial warming. Can. J. Soil Sci. 93, 567–572. doi: 10.4141/cjss2013-046
D’Orangeville, L., Houle, D., Côté, B., Duchesne, L., and Morin, H. (2013b). Increased soil temperature and atmospheric N deposition have no effect on the N status and growth of a mature balsam fir forest. Biogeosciences 10, 4627–4639. doi: 10.5194/bg-10-4627-2013
D’Orangeville, L., Houle, D., Côté, B., and Duchesne, L. (2014). Soil response to a 3-year increase in temperature and nitrogen deposition measured in a mature boreal forest using ion-exchange membranes. Environ. Monit. Assess. 186, 8191–8202. doi: 10.1007/s10661-014-3997-x
Durán, J., Morse, J. L., Groffman, P. M., Campbell, J. L., Christenson, L. M., Driscoll, C. T., et al. (2016). Climate change decreases nitrogen pools and mineralization rates in northern hardwood forests. Ecosphere 7, 1–13. doi: 10.1002/ecs2.1251
Fenn, M. E., Ross, C. S., Schilling, S. L., Baccus, W. D., Larrabee, M. A., and Lofgren, R. A. (2013). Atmospheric deposition of nitrogen and sulfur and preferential canopy consumption of nitrate in forests of the Pacific Northwest. USA. For. Ecol. Manag. 302, 240–253. doi: 10.1016/j.foreco.2013.03.042
Frey, S. D., Knorr, M., Parrent, J. L., and Simpson, R. T. (2004). Chronic nitrogen enrichment affects the structure and function of the soil microbial community in temperate hardwood and pine forests. For. Ecol. Manag. 196, 159–171. doi: 10.1016/j.foreco.2004.03.018
Frey, S. D., Ollinger, S., Nadelhoffer, K., Bowden, R., Brzostek, E., Burton, A., et al. (2014). Chronic nitrogen additions suppress decomposition and sequester soil carbon in temperate forests. Biogeochemistry 121, 305–316. doi: 10.1007/s10533-014-0004-0
Fu, W., Wang, X., and Wei, X. (2019). No response of soil N mineralization to experimental warming in a northern middle-high latitude agro-ecosystem. Sci. Total Environ. 659, 240–248. doi: 10.1016/j.scitotenv.2018.12.315
Geisseler, D., Horwath, W. R., Joergensen, R. G., and Ludwig, B. (2010). Pathways of nitrogen utilization by soil microorganisms - A review. Soil Biol. Biochem. 42, 2058–2067. doi: 10.1016/j.soilbio.2010.08.021
Gilliam, F. S., Burns, D. A., Driscoll, C. T., Frey, S. D., Lovett, G. M., and Watmough, S. A. (2019). Decreased atmospheric nitrogen deposition in eastern North America: predicted responses of forest ecosystems. Environ. Pollut. 244, 560–574. doi: 10.1016/j.envpol.2018.09.135
Gundale, M. J., Deluca, T. H., and Nordin, A. (2011). Bryophytes attenuate anthropogenic nitrogen inputs in boreal forests. Glob. Chang. Biol. 17, 2743–2753. doi: 10.1111/j.1365-2486.2011.02407.x
Hannah, L. (2015). “Chapter 4 - Phenology: changes in timing of biological events due to climate change,” in Climate Change Biology, 2nd Edn, ed. L. Hannah (Boston, MA: Academic Press), 83–102. doi: 10.1016/B978-0-12-420218-4.00004-4
Hart, S. C., Stark, J. M., Davidson, E. A., and Firestone, M. K. (1994). “Nitrogen mineralization, immobilization, and nitridication,” in Methods of Soil Analysis, Part 2. Microbiological and Biochemical Properties, eds P. J. Bottomley, J. S. Angle, and R. W. Weaver (Madison, WI: SSSA Book Series no5).
Hobbie, E., and Hobbie, J. (2008). Natural abundance of 15N in nitrogen-limited forests and tundra can estimate nitrogen cycling through mycorrhizal fungi: a review. Ecosystems 11, 815–830. doi: 10.1007/s10021-008-9159-7
Hobbie, E., and Ouimette, A. (2009). Controls of nitrogen isotope patterns in soil profiles. Biogeochemistry 95, 355–371. doi: 10.1007/s10533-009-9328-6
Hobbie, J. E., Hobbie, E. A., Drossman, H., Conte, M., Weber, J. C., Shamhart, J., et al. (2009). Mycorrhizal fungi supply nitrogen to host plants in Arctic tundra and boreal forests: 15N is the key signal. Can. J. Microbiol. 55, 84–94. doi: 10.1139/W08-127
Hobbie, S. E., Nadelhoffer, K. J., and Högberg, P. (2002). A synthesis: the role of nutrients as constraints on carbon balances in boreal and arctic regions. Plant Soil 242, 163–170. doi: 10.1023/A:1019670731128
Houle, D., Bouffard, A., Duchesne, L., Logan, T., and Harvey, R. (2012). Projections of Future Soil Temperature and Water Content for Three Southern Quebec forested sites. J. Clim. 25, 7690–7701. doi: 10.1175/JCLI-D-11-00440.1
Houle, D., Marty, C., and Duschesne, L. (2015). Response of nitrogen canopy uptake to a rapid decrease in bulk nitrate deposition in two eastern Canadian boreal forests. Oecologia 177, 29–37. doi: 10.1007/s00442-014-3118-0
Houle, D., Moore, J., Ouimet, R., and Marty, C. (2014). Tree species partition N uptake by soil depth in boreal forests. Ecology 95, 1127–1133. doi: 10.1890/14-0191.1
Hugues, L. (2000). Biological consequences of global warming: is the signal already. TREE 15, 56–61. doi: 10.1016/s0169-5347(99)01764-4
Knorr, A. M., Frey, S. D., and Curtis, P. S. (2005). Nitrogen additions and litter decomposition: a meta-analysis. Ecology 86, 3252–3257. doi: 10.1890/05-0150
Kuznetsova, A., Brockhoff, P. B., and Christensen, R. H. B. (2017). lmerTest package: tests in linear mixed effects models. J. Stat. Softw. 82, 1–26. doi: 10.18637/jss.v082.i13
Lal, R. (2005). Forest soils and carbon sequestration. For. Ecol. Manag. 220, 242–258. doi: 10.1016/j.foreco.2005.08.015
Lilleskov, E., Fahey, T., and Lovett, G. (2001). Ectomycorrhizal fungal aboveground community change over an atmospheric nitrogen deposition gradient. Ecol. Appl. 11, 397–410. doi: 10.1890/1051-0761(2001)011[0397:efacco]2.0.co;2
Luo, Y., Wan, S., Hui, D., and Wallace, L. L. (2001). Acclimatization of soil respiration to warming in a tall grass prairie. Nature 413, 622–625. doi: 10.1038/35098065
Lupi, C., Morin, H., Deslauriers, A., Rossi, S., and Houle, D. (2012). Increasing nitrogen availability and soil temperature: effects on xylem phenology and anatomy of mature black spruce 1 1 This article is one of a selection of papers from the 7th International Conference on Disturbance Dynamics in Boreal Forests. Can. J. For. Res. 42, 1277–1288. doi: 10.1139/x2012-055
Ma, L., Lu, X., Liu, Y., Guo, J., Zhang, N., Yang, J., et al. (2011). The effects of warming and nitrogen addition on soil nitrogen cycling in a temperate grassland, northeastern China. PLoS One 6:e27645. doi: 10.1371/journal.pone.0027645
Maaroufi, N. I., Nordin, A., Hasselquist, N. J., Bach, L. H., Palmqvist, K., and Gundale, M. J. (2015). Anthropogenic nitrogen deposition enhances carbon sequestration in boreal soils. Glob. Chang. Biol. 21, 3169–3180. doi: 10.1111/gcb.12904
Magill, A. H., and Aber, J. D. (1998). Long-term effects of experimental nitrogen additions on foliar litter decay and humus formation in forest ecosystems. Plant Soil 203, 301–311. doi: 10.1023/A:1004367000041
Marty, C., Houle, D., Courchesne, F., Gagnon, C., (2019b). Soil C:N ratio is the main driver of soil δ15N in cold and N-limited eastern Canadian forests. Catena 172, 285–294. https://doi.org/10.1016/j.catena.2018.08.029
Marty, C., Houle, D., Gagnon, C., and Duchesne, L. (2011). Isotopic compositions of S, N and C in soils and vegetation of three forest types in Québec, Canada. Appl. Geochem. 26, 2181–2190. doi: 10.1016/j.apgeochem.2011.08.002
Marty, C., Houle, D., Gagnon, C., and Courchesne, F. (2017). The relationships of soil total nitrogen concentrations, pools and C: N ratios with climate, vegetation types and nitrate deposition in temperate and boreal forests of eastern Canada. Catena 152, 163–172. doi: 10.1016/j.catena.2017.01.014
Marty, C., Piquette, J., Morin, H., Bussières, D., Thiffault, N., Houle, D., et al. (2019a). Nine years of in situ soil warming and topography impact the temperature sensitivity and basal respiration rate of the forest floor in a Canadian boreal forest. PLoS One 14:e0226909. doi: 10.1371/journal.pone.0226909
Mayor, J., Bahram, M., Henkel, T., Buegger, F., Pritsch, K., and Tedersoo, L. (2015). Ectomycorrhizal impacts on plant nitrogen nutrition: emerging isotopic patterns, latitudinal variation and hidden mechanisms. Ecol. Lett. 18, 96–107. doi: 10.1111/ele.12377
Mayor, J. R., Schuur, E. A. G., Mack, M. C., Hollingsworth, T. N., and Baath, E. (2012). Nitrogen isotope patterns in Alaskan black spruce reflect organic nitrogen sources and the activity of ectomycorrhizal fungi. Ecosystems 15, 819–831. doi: 10.1007/s10021-012-9548-9549
McFarland, J. W., Ruess, R. W., Kielland, K., Doyle, A. P., Mcfarland, J. W., Ruess, R. W., et al. (2002). Cycling dynamics of NH4+ and amino acid nitrogen in soils of a deciduous boreal forest ecosystem. Ecosystems 5, 775–788. doi: 10.1007/s10021-002-0146-0LCIT
Melillo, J. M., Frey, S. D., Deangelis, K. M., Werner, W. J., Bernard, M. J., Bowles, F. P., et al. (2017). Long-term pattern and magnitude of soil carbon feedback to the climate system in a warming world. Science 358, 101–105. doi: 10.1126/science.aan2874
Moorhead, D. L., and Sinsabaugh, R. L. (2006). A theoretical model of litter decay and microbial interaction. Ecol. Monogr. 76, 151–174. doi: 10.1890/0012-9615(2006)076[0151:atmold]2.0.co;2
Mulvaney, R. (1996). “Nitrogen-Inorganic forms,” in Methods of Soil Snalysis: Part 3-Chemical methods, ed. D. Sparks (Madison, WI: Wiley), 1123–1184. doi: 10.2136/sssabookser5.3.c38
Numainville, G., and Desponts, M. (2004). Les Stades de Débourrement des Bourgeons Foliaires de L’épinette Noire. Québec: Gouvernement du Québec, 23.
Otis Prud’Homme, G., Lamhamedi, M. S., Benomar, L., Rainville, A., Deblois, J., Bousquet, J., et al. (2018). Ecophysiology and growth of white spruce seedlings from various seed sources along a climatic gradient support the need for assisted migration. Front. Plant Sci. 8:2214. doi: 10.3389/fpls.2017.02214
Ouranos (2015). Vers l’adaptation. Synthèse des connaissances sur les changements climatiques au Québec. Montréal: Ouranos.
Paré, M. C., and Bedard-Haughn, A. (2012). Landscape-scale N mineralization and greenhouse gas emissions in Canadian Cryosols. Geoderma 189–190, 469–479. doi: 10.1016/j.geoderma.2012.06.002
Parkinson, J. A., and Allen, S. E. (1975). A wet oxidation procedure suitable for the determination of nitrogen and mineral nutrients in biological material. Comm. Soil Sci. Plant Anal. 6, 1–11. doi: 10.1080/00103627509366539
Persson, J., Högberg, P., Ekblad, A., Högberg, M. N., Nordgren, A., and Näsholm, T. (2003). Nitrogen acquisition from inorganic and organic sources by boreal forest plants in the field. Oecologia 137, 252–257. doi: 10.1007/s00442-003-1334-1330
Persson, J., and Näsholm, T. (2001). Amino acid uptake: a widespread ability among boreal forest plants. Ecol. Lett. 4, 434–438. doi: 10.1046/j.1461-0248.2001.00260.x
Pisani, O., Frey, S. D., Simpson, A. J., and Simpson, M. J. (2015). Soil warming and nitrogen deposition alter soil organic matter composition at the molecular-level. Biogeochemistry 123, 391–409. doi: 10.1007/s10533-015-0073-78
Preston, C. M., Trofymow, J. A., Sayer, B. G., and Niu, J. (1997). 13C nuclear magnetic resonance spectroscopy with cross-polarization and magic-angle spinning investigation of the proximate-analysis fractions used to assess litter quality in decomposition studies. Can. J. Bot. 75, 1601–1613. doi: 10.1139/b97-872
R Core Team (2019). R: A Language and Environment for Statistical Computing. Vienna: R Foundation for Statistical Computing. Available online at: https://www.R-project.org/
Reinmann, A. B., Susser, J. R., Demaria, E. M. C., and Templer, P. H. (2019). Declines in northern forest tree growth following snowpack decline and soil freezing. Glob. Chang. Biol. 25, 420–430. doi: 10.1111/gcb.14420
Rossi, S., Bordeleau, A., Morin, H., and Houle, D. (2013). The effects of N-enriched rain and warmer soil on the ectomycorrhizae of black spruce remain inconclusive in the short term. Ann. For. Sci. 70, 825–834. doi: 10.1007/s13595-013-0329-1
Rossi, S., Morin, H., and Deslauriers, A. (2011a). Multi-scale influence of snowmelt on xylogenesis of black spruce. Arctic, Antarct. Alp. Res. 43, 457–464. doi: 10.1657/1938-4246-43.3.457
Rossi, S., Morin, H., Deslauriers, A., and Plourde, P.-Y. (2011b). Predicting xylem phenology in black spruce under climate warming. Glob. Chang. Biol. 17, 614–625. doi: 10.1111/j.1365-2486.2010.02191.x
Schimel, J. P., and Bennett, J. B. (2004). Nitrogen mineralization: challenges of a changing paradigm. Ecology 85, 591–602. doi: 10.1890/03-8024
Schimel, J. P., and Chapin, F. S. (1996). Tundra plant uptake of amino acid and NH4+ nitrogen in situ: plants compete well for amino acid nitrogen. Ecology 77, 2142–2147. doi: 10.2307/2265708
Schmidt, M. W. I, Torn, M. S., Abiven, S., Dittmar, T., Guggenberger, G., Janssens, I. A., et al. (2011). Persistence of soil organic matter as an ecosystem property. Nature 478, 49–56. doi: 10.1038/nature10386
Schulte-Uebbing, L., and de Vries, W. (2018). Global-scale impacts of nitrogen deposition on tree carbon sequestration in tropical, temperate, and boreal forests: a meta-analysis. Glob. Chang. Biol. 24, e416–e431. doi: 10.1111/gcb.13862
Shenoy, A., Kielland, K., and Johnstone, J. F. (2013). Effects of fire severity on plant nutrient uptake reinforce alternate pathways of succession in boreal forests. Plant Ecol. 214, 587–596. doi: 10.1007/s11258-013-0191-190
Silvestro, R., Rossi, S., Zhang, S., Froment, I., Huang, J.-G., and Saracino, A. (2019). From phenology to forest management: ecotypes selection can avoid early or late frosts, but not both. For. Ecol. Manage 436, 21–26. doi: 10.1016/j.foreco.2019.01.005
Simpson, M. J., and Simpson, A. J. (2012). The Chemical Ecology of Soil Organic Matter Molecular Constituents. J. Chem. Ecol. 38, 768–784. doi: 10.1007/s10886-012-0122-x
Ste-Marie, C., and Houle, D. (2006). Forest floor gross and net nitrogen mineralization in three forest types in Quebec. Canada. Soil Biol. Biochem. 38, 2135–2143. doi: 10.1016/j.soilbio.2006.01.017
Templer, P. H., Arthur, M. A., Lovett, G. M., and Weathers, K. C. (2007). Plant and soil natural abundance delta 15N: indicators of relative rates of nitrogen cycling in temperate forest ecosystems. Oecologia 153, 399–406. doi: 10.1007/s00442-007-0746-7
Vuorenmaa, J., Augustaitis, A., Beudert, B., Bochenek, W., Clarke, N., De Wit, H. A., et al. (2018). Long-term changes (1990 – 2015) in the atmospheric deposition and runoff water chemistry of sulphate, inorganic nitrogen and acidity for forested catchments in Europe in relation to changes in emissions and hydrometeorological conditions. Sci. Total Environ. 625, 1129–1145. doi: 10.1016/j.scitotenv.2017.12.245
Zhang, X., Wang, Q., Gilliam, F., Bai, W., Han, X., and Li, L. (2012). Effect of nitrogen fertilization on net nitrogen mineralization in a grassland soil, northern China. Grass Forage Sci. 67, 219–230. doi: 10.1111/j.1365-2494.2011.00836.x
Zhang, Y., Mathur, R., Bash, J. O., Hogrefe, C., Xing, J., and Roselle, S. J. (2018). Long-term trends in total inorganic nitrogen and sulfur deposition in the US from 1990 to 2010. Atmos. Chem. Phys. 18, 9091–9106. doi: 10.5194/acp-18-9091-2018
Keywords: soil warming experiment, nitrogen addition experiment, boreal forest, nitrogen mineralization, conifer regeneration, foliar δ15N, NMR
Citation: Marty C, Piquette J, Dussault-Chouinard É, Morin H, Thiffault N, Houle D, Bradley RL, Ouimet R, Simpson MJ and Paré MC (2020) Canopy Nitrogen Addition and Soil Warming Affect Conifer Seedlings’ Phenology but Have Limited Impact on Growth and Soil N Mineralization in Boreal Forests of Eastern Canada. Front. For. Glob. Change 3:581363. doi: 10.3389/ffgc.2020.581363
Received: 08 July 2020; Accepted: 28 September 2020;
Published: 29 October 2020.
Edited by:
Zuoqiang Yuan, Institute of Applied Ecology (CAS), ChinaReviewed by:
Guiomar Ruiz-Pérez, Swedish University of Agricultural Sciences, SwedenMariangela N. Fotelli, Hellenic Agricultural Organization, Greece
Copyright © 2020 Marty, Piquette, Dussault-Chouinard, Morin, Thiffault, Houle, Bradley, Ouimet, Simpson and Paré. This is an open-access article distributed under the terms of the Creative Commons Attribution License (CC BY). The use, distribution or reproduction in other forums is permitted, provided the original author(s) and the copyright owner(s) are credited and that the original publication in this journal is cited, in accordance with accepted academic practice. No use, distribution or reproduction is permitted which does not comply with these terms.
*Correspondence: Maxime C. Paré, TWF4aW1lX1BhcmVAdXFhYy5jYQ==