- Department of Botany, DSB Campus, Kumaun University, Nainital, Uttarakhand, India
Coriaria nepalensis, a nitrogen-fixing actinorhizal shrub, is a prominent and successful colonizer of bare rocks and landslide affected degraded lands. Field experiments were conducted to determine the differences in biomass decomposition and nutrient release pattern of different plant parts of C. nepalensis using litter bag technique. Results showed that the leaves decomposed completely within 6 months while only 46.55% of the lateral roots were decomposed with slowest decomposition rate of 0.14% day–1. The decomposition rate was in the order: Leaves > Reproductive parts > Twigs (< 5 mm) > Twigs (> 5 mm) > Bark > Fine roots > Lateral roots. The decay rate coefficient was highest (0.003–0.014) for leaves and lowest (0.001–0.002) for lateral roots. During the decomposition cycle (364 days), overall increase was reported in nitrogen and phosphorus concentration, while potassium concentration decreased continuously in residual litters. The nutrient mobility was in the order: K > P > N. Climatic factors like temperature, relative humidity and rainfall significantly affected the decomposition process and among these factors, rainfall pattern emerged as a most effective environmental driver. Thus, taking into account initial nutrient content, nutrient release and decay rates, the leaves and reproductive parts of C. nepalensis proved to be more important as nutrient source than other components.
Introduction
Decomposition of plant litter is a fundamental biogeochemical process and plays vital role in soil organic matter accumulation and nutrient cycling (Bargali et al., 2015a; Li et al., 2022). It plays major role in regeneration, conserving biological diversity, ensuring plant nutrition, maintaining air and water quality, and preserving soil resilience (Steffen et al., 2015; Turmel et al., 2015), thus any disturbance that affects the decomposition can have pervasive effects on ecosystem functioning. Nutrient release pattern varies with nutrient types and exhibits the different immobilizing patterns which pose different impacts on forest ecosystem functions (Bargali, 1996).
According to the prevalent conceptual model of litter decomposition, climate, litter quality and decomposer species are the key influencers that regulate the rate of decomposition (Bradford et al., 2017; Manral et al., 2022). These controls are thought to work in a hierarchical manner in space, with climate and litter quality co-dominant at regional to global dimensions but decomposers acting solely as the local control with minor effects at larger scales (Makkonen et al., 2012; Santonja et al., 2015; Guo et al., 2019). Over the last three decades, it has been demonstrated that litter decay is influenced by a variety of chemical properties of the litter, including the litter N (Hobbie et al., 2012; Karki et al., 2022; Li et al., 2022), P and K concentrations and lignin content (Liu et al., 2022). However, Monroy et al. (2022) suggested that in temperate regions, chemical properties may not be a good predictor of litter decomposability. Climatic factors like temperature, humidity and rainfall regulate the decomposition processes (Sanderman and Amundson, 2005; Kumar et al., 2021) and among these drivers rainfall pattern assert decomposition more than any other environmental drivers (Bradford et al., 2016; Steidinger et al., 2019).
Coriaria nepalensis (Coriariaceae) is commonly known as Masuri berry, Rikhola, Makhaul, Tanner’s tree, or Masuri shrub (Awasthi et al., 2022a). It is evergreen non-leguminous nitrogen fixing actinorhizal shrub with root nodules formed by Frankia and arbuscular mycorrhizal fungi (Santi et al., 2013). It is prominent and successful colonizer of bare rocks and landslide affected areas. Being a nodulated species (actinorhizal), it is very helpful in nitrogen fixation in eroded soil and able to replenish the deficiency of nitrogen for associate nearby growing species (Bargali et al., 2015a; Mourya et al., 2019).
Active restoration of degraded lands can be achieved through reactivation of biogeochemical nutrient cycles via litter decomposition. Lanuza et al. (2019) reported that the litter decomposition of nitrogen-fixing plants improve the nutrient release and nutrient deposition to support vegetation development and recover soil properties signifying that C. nepalensis should be used for recovering nutrient cycling in retrieval of degraded hills. Most of the studies on decomposition of litter generally consider leaf component only because it represent 50–90% of the total litter fall (Farooq et al., 2022), but in an ecosystem where leaf litter represents only 50% of the total litter fall, it is very vital to consider rest 50% of the litter for decomposition as well (Awasthi et al., 2022b). This study was conducted with the hypothesis that (i) the responses of different litter components of same species differ in weight loss and nutrient release pattern due to differences in initial nutrient content and (ii) in relatively short periods of time C. nepalensis litter can improve soil nutrient (N, P, and K) availability through decomposition of nutrient rich litter and therefore, suitable for the restoration of degraded hills.
Assessment of the periodic monitoring of nutrient cycling in the present climate changing scenario could help in better understanding the alteration in litter decomposition processes and nutrient recycling, if any. However, the available literature about the decomposition of C. nepalensis litter is very old and has not been assessed with respect to the present rising temperature and changing rainfall pattern, which modify the interconnected environmental variables of any region. The nitrogen fixing potential of C. nepalensis possesses nursing effect which encourages other associated species to grow and stabilize the degraded lands for longer period of time and reduces the probability of land degradation, biodiversity loss and landslides in those areas. So, a complete account of information about the contribution of C. nepalensis in nutrients (N, P, and K) recycling would be essential for the proper management and restoration of degraded lands in current climate changing circumstances. Therefore, the objective of this study was to analyse the decomposition rate and associated nutrient dynamics of different plant components of C. nepalensis as a key strategy to reactivate biogeochemical nutrient cycles and thus, improve soil quality in degraded lands of Kumaun Himalaya, India.
Materials and methods
The climate of the study area is monsoon warm temperate (Singh and Singh, 1992). It is characterized by summer (March to mid-June), rainy (mid-June to October) and very cold winter from November to February or sometimes till mid-March. Climatic data of the study site was obtained from the ARIES (Aryabhatta Research Institute of Observational Sciences, Manora peak, Nainital), which is within the aerial distance of 2 km from the study site. During the study period (September 2017 to March 2019), minimum temperature ranged between 7 and 23°C while maximum temperature ranged between 19 and 32°C. The lowest annual rainfall in the region was recorded as 0 mm (November 2017), whereas, highest rainfall was observed as 944.7 mm (August 2018) (Figure 1). About 75% of the total rainfall occurs during the 3 months, i.e., mid-June to mid-September.
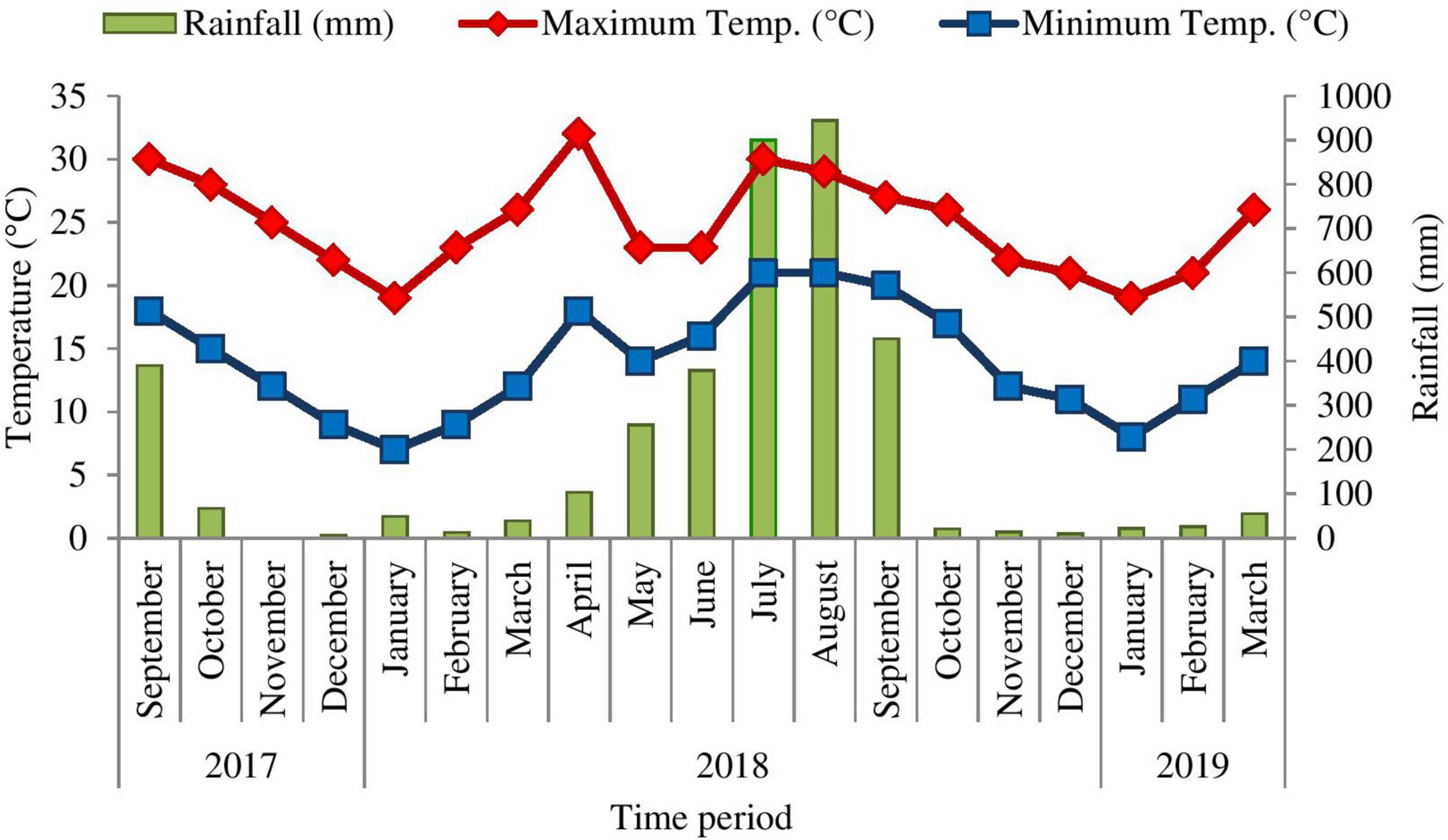
Figure 1. Meteorological data of Nainital (study sites) during September 2017 to March 2019 (Source: ARIES, Nainital).
Decomposition processes
The detailed description of the litter fall estimation of the C. nepalensis dominated sites is given in Awasthi et al. (2022b). For the assessment of the decomposition rate and nutrient dynamics of the different components of the same, litter bag technique, which is a direct method of measuring decomposition rate, was used to observe the litter decomposition (Awasthi et al., 2022c). Nearly senesced but attached plant leaves, twigs (twigs < 5 mm and twigs > 5 mm), bark, fine roots, lateral roots, and reproductive parts were collected from the plant and air dried till the constant weight was not achieved. Nylon litter bags (20 cm × 20 cm with 1 mm mesh size) were prepared and filled with 10 g air dried litter samples, separately. To permit the movement of microbes and other litter decomposing agents, 1 mm mesh size was considered sufficient (Robertson and Paul, 1999). Before deciding on the necessary number of duplicates, variations in the microenvironment of the stand, the diversity of the overstory and understory, and the quality of the litter were taken into account (the phytodiversity of all the sites is given in Awasthi et al., 2022a). A total of 378 litter bags (7 components × 3 replicates × 18 months) were randomly placed on the forest floor in the month of August 2017 in such a way that they were very close to soil without disturbing the forest floor vegetation. This made it possible to characterize the decay curve in a more reliable way. To compare the sample mass of the pre and post decomposition stages, collected litter bags were oven dried as well. All the samples in the litter bags for decomposition were placed at Rusi (1,756 m asl) which is located between 29°21′57′′N latitude 79°27′23′′E longitude. As the site was very steep in nature, small plain surfaces under C. nepalensis plant had been chosen to put litter bags with different litter components after removing the pre-existing debris. No disturbance rather than removing the debris had been made. To protect the litter bags from washing out from the steep site, a boundary of large gravels around the litter bags had been created. Further details about the study site were given in Awasthi et al. (2022b).
Three litter bags of each sample for 18 months (544 days) were monthly recovered. The recovered litter samples were kept in polyethylene bags individually and brought to laboratory, where the samples after separating from the litter bags were carefully washed with water to remove soil particles and dried at 60°C to constant weight and weighed.
Rate of decomposition (% R)
The weight loss of litter was expressed as the percentage remaining after a given time and was calculated as (Petersen and Cummins, 1974; Smeti et al., 2019):
Where, W(tx) is the dry weight (g) of the litter after time (tx), and W(ti) is the initial weight of the litter. In the present study, % R was calculated monthly as well as for the entire period.
Decay rate coefficient (k) was computed following Ndagurwa et al. (2015) as:
Where, t is the time in days.
Relative decomposition rate (RDR) was calculated following Bargali et al. (2015b) as:
Where, W0 = litter mass at time t0, W1 = litter mass at time t1, t1 − t0 = sampling interval (days).
The mass loss over time was fitted to a simple negative exponential model (Olson, 1963; Tan et al., 2021):
Where, X0 is the original litter mass, Xt the amount of litter remaining after time t, t the time (days), and k is the decomposition rate (day–1). The time required for 50% and 95% mass loss was calculated as t50 = 0.693/k and t95 = 3/k, respectively.
Chemical analysis of litter
Collected litter material was grounded and passed through a 0.5 mm sieve for chemical analysis. All analyses were carried out in triplicate. Total Nitrogen (N) concentration was determined by the micro-Kjeldahl method (Jackson, 1958; Kumar et al., 2022). Phosphorous (P) concentration was estimated by the Olsen’s method (Olsen et al., 1954; Wieczorek et al., 2022) using spectrophotometer and the potassium, i.e., exchangeable potassium was determined by flame photometer (Black, 1965; Banerjee and Prasad, 2020) in Soil testing laboratory, Tea Development Board, Bhowali, Nainital.
Nutrient release pattern of decomposing litter
The release pattern of nutrients (N, P, and K) from the litter was calculated monthly by determining the differences in nutrient quantities between two successive months and the net release was calculated by summing nutrient release for the entire period (Wang et al., 2014).
Where, Wt2 is the dry weight of component litter at time t2, Xt2 is the nitrogen/phosphorus/potassium concentration of components at time t2, Wt1 is the weight of component at time t1 and Xt1 is the concentration of nitrogen/phosphorus/potassium of component at time t1.
Results
Weight loss pattern
There is no benchmark for the beginning of decomposition of litter that is detached from the living plant. During decomposition, litter samples lost their natural color and turned black by the time, got fragmented into various pieces except twigs > 5 mm (544 days), which may be because of the formation of hardwood or secondary growth in twigs > 5 mm. For all the litter components, higher decomposition was recorded during the period between the placement of the litter bags (August) and first two collections (After 30 and 61 days). Among all the components, leaves decomposed within 6 months of the placement on the site because of its high surface area and thinness of leaves (0.54% day–1), whereas lateral roots decomposed only 46.55% with the slowest decomposition rate (0.14% day–1) (Table 1). The order of decomposition rate of the different components was: Leaves > Reproductive parts > Twigs (<5 mm) > Twigs (>5 mm) > Bark > Fine roots > Lateral roots.
The decay coefficient of all components is given in Table 2. It was highest for leaves (0.003–0.014) and lowest for lateral roots (0.001–0.002). Relative decomposition rate for leaves, reproductive parts, twigs < 5 mm, twigs > 5 mm, bark, lateral roots and fine roots was ranged from 0.001 to 0.017, 0.000–0.024, 0.000–0.0227, 0.001–0.011, 0.000–0.011, 0.000–0.037, and 0.001–0.026 g g–1 day–1, respectively (Table 3). t50 and t95 of respective components is given in Table 4.
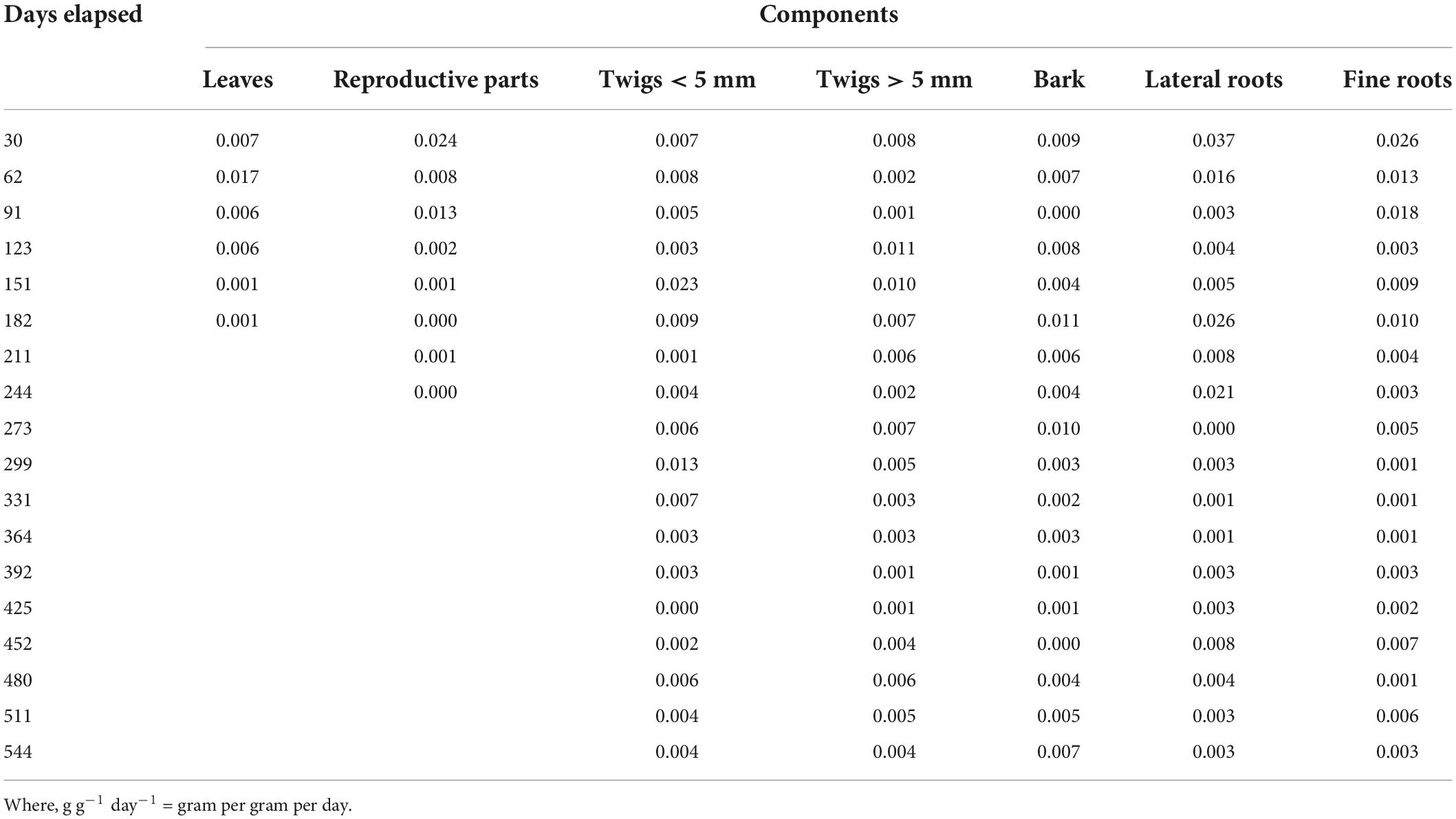
Table 3. Relative decomposition rate (g g–1day–1) for different components of Coriaria nepalensis (n = 3).
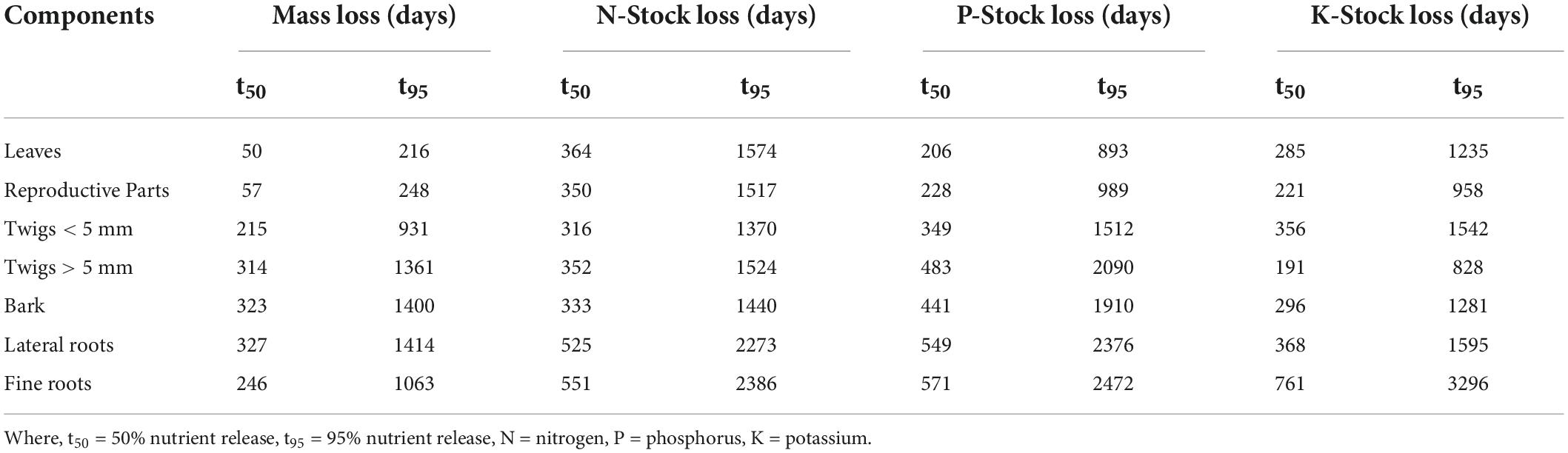
Table 4. Decomposition parameters for nutrients and time required for various levels of nutrient release (n = 3).
Changes in nutrient concentration in decomposing litter
N and P concentration in the different litter component was generally increased throughout the study period but concentration of K decreased in residual litter in the entire decomposition cycle (364 days). N concentration in bark and fine roots and P concentration in bark and lateral roots slightly decreases sometimes in the later stage of decomposition. Concentration of N was more than twice of initial concentration during the study period in both types of twigs and bark (Figures 2A–C). t50 and t95 for N, P, and K stock loss for all respective components is given in Table 4.
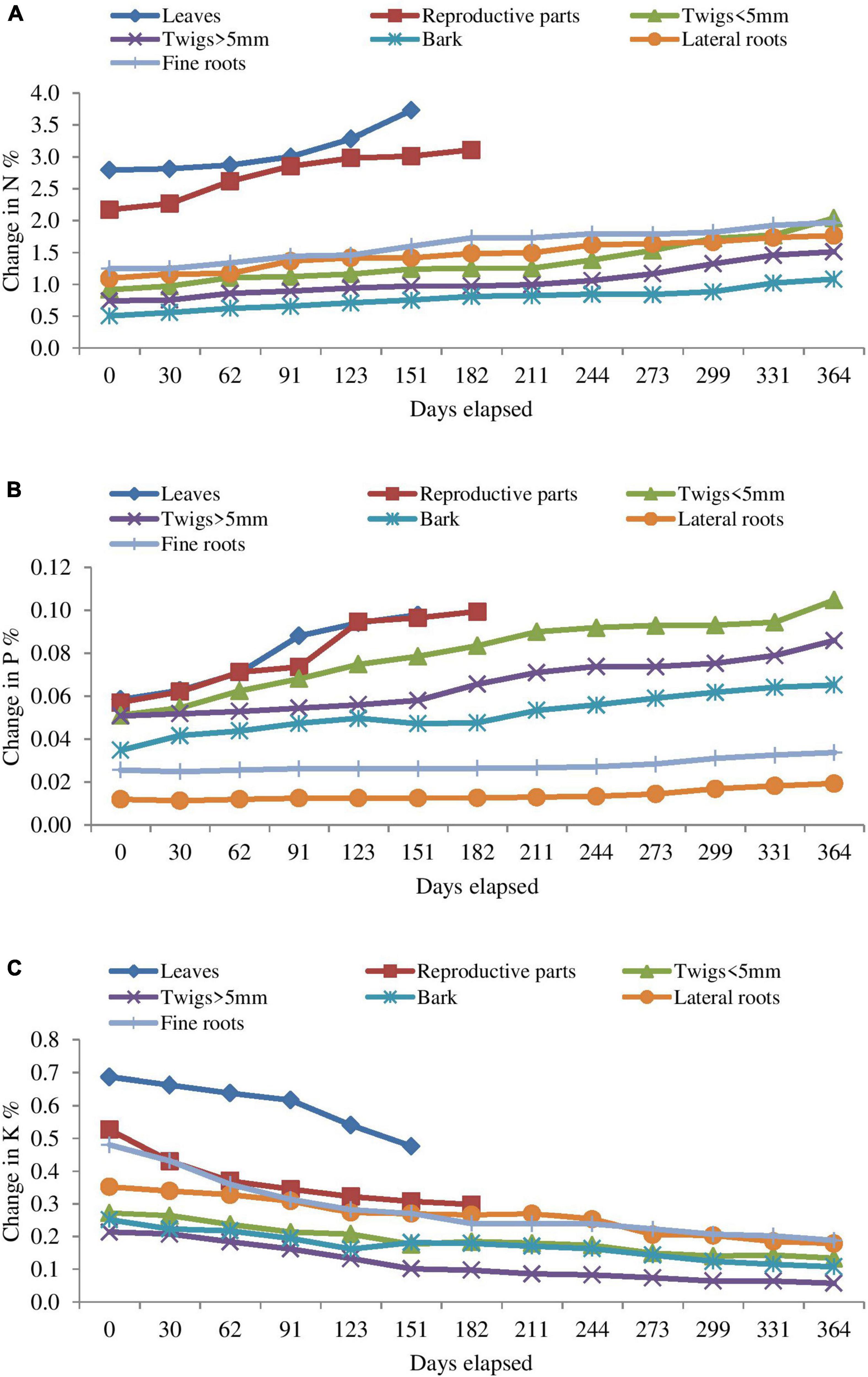
Figure 2. Changes in (A) Nitrogen, (B) Phosphorus, and (C) Potassium concentration in different components of decomposing Coriaria nepalensis litter (n = 3).
Nutrient release pattern
During first 123 days, about 71% of nitrogen was released from leaves followed by reproductive parts (50.36%) and twigs < 5 mm (27.74%). The slowest release of nitrogen in the first 123 days was in lateral roots (0.35%) followed by fine roots (2.70%). In case of bark the absolute amount of nitrogen increased by 1.61% within 123 days of the study. In the first month of the study (0–30 days) the maximum release in nitrogen was in reproductive parts (37.60%), whereas, minimum was in twigs > 5 mm (7.55%). Except leaf litter, no other component continuously decreased in absolute amount of nitrogen throughout the study. At the end of the study (364 days), percent N release ranged from 14.97% (Twigs < 5 mm) to 83.05% (Leaves) (Figure 3).
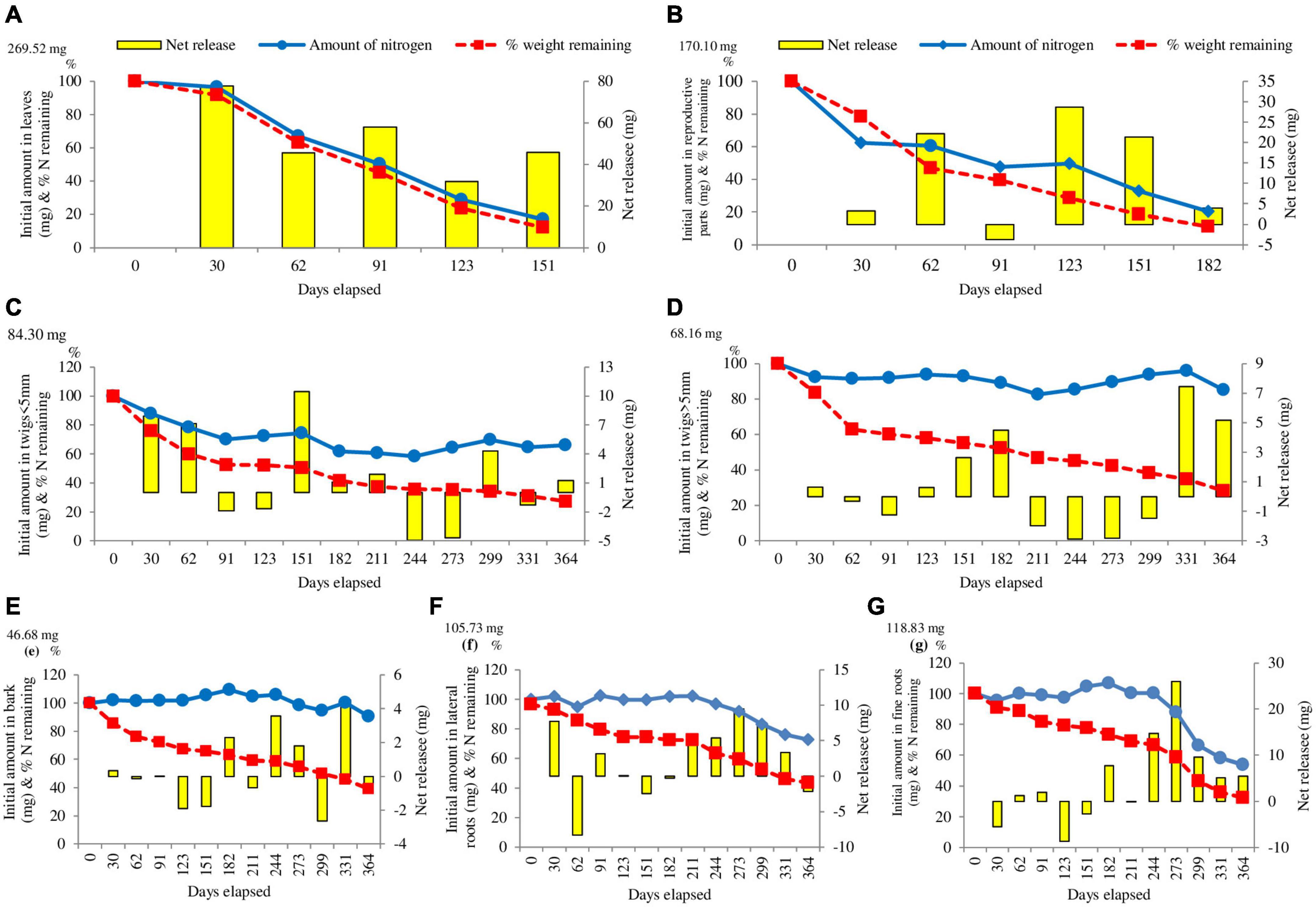
Figure 3. Changes in the absolute amount of nitrogen in (A) leaves, (B) reproductive parts, (C) twigs < 5 mm, (D) twigs > 5 mm, (E) bark, (F) lateral roots, (G) fine roots mass in litter. The initial mass of nitrogen is given on the left side of the Y-axis. The columns indicate the net change between the measurements (right Y-axis). The broken lines indicate the percentage weight remaining of litter (n = 3).
The release of phosphorus was faster in comparison to nitrogen except in the case of leaf litter. The pattern of phosphorus release was fastest in leaves (78.92%) in the first 151 days of the study, followed by reproductive parts (67.13%) and lateral roots (19.61%). None of the selected components surpassed the initial absolute amount of phosphorus except bark that surpassed the absolute amount of phosphorus by maximum 11.01%. At the end of the study, release of phosphorus was observed between 24.73% (Bark) and 79.92% (Leaves). Except leaf and reproductive parts, no other component continuously decreased in absolute amount of phosphorus throughout the study period (Figure 4).
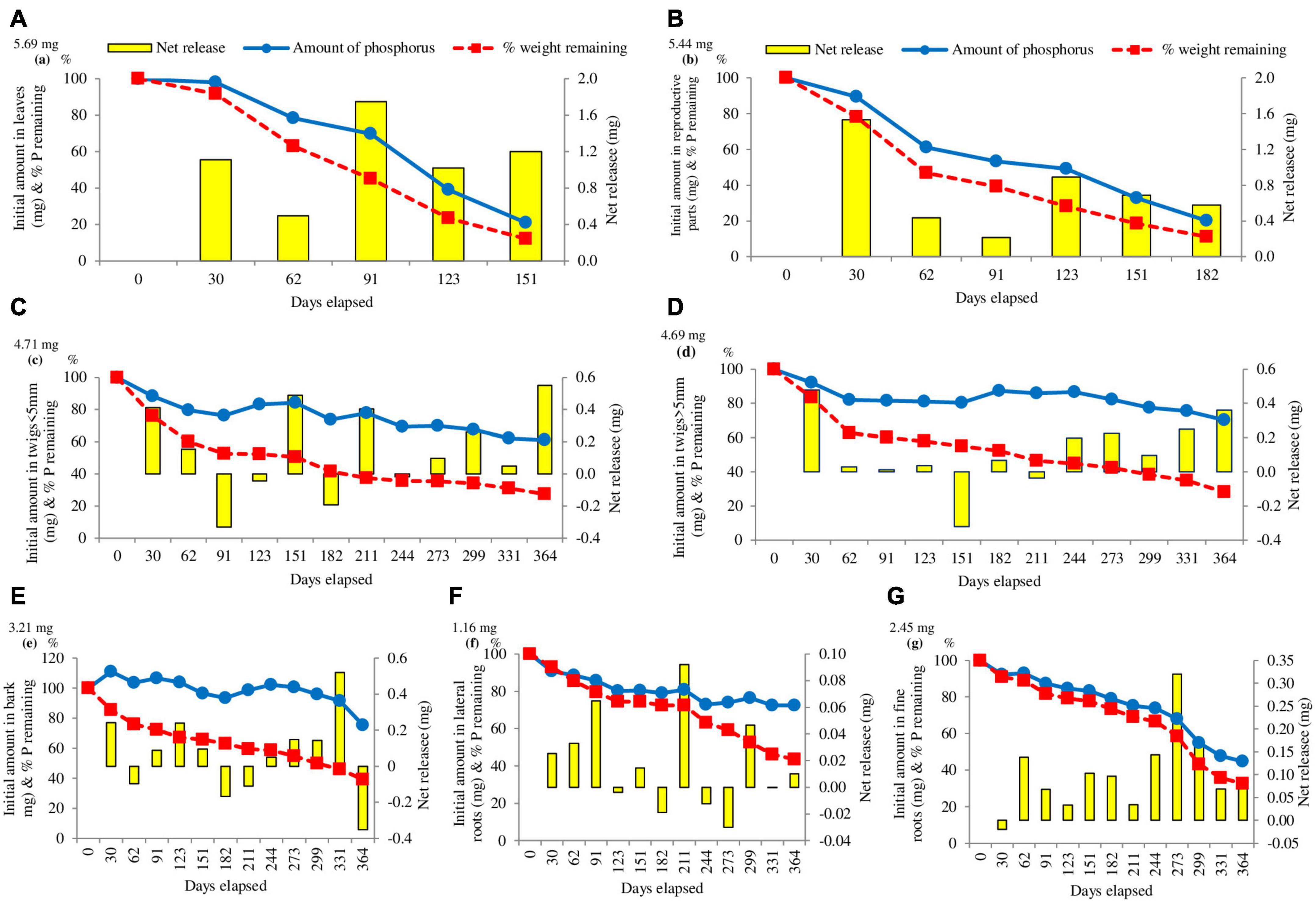
Figure 4. Changes in the absolute amount of phosphorus in (A) leaves, (B) reproductive parts, (C) twigs < 5 mm, (D) twigs > 5 mm, (E) bark, (F) lateral roots, (G) fine roots mass enclosed in litter. The initial mass of phosphorus is given on the left side of the Y-axis. The columns indicate the net change between the measurements (right Y-axis). The broken lines indicate the percentage weight remaining of litter (n = 3).
The release of potassium was faster in comparison to nitrogen and phosphorus. Continuous release occurred in all the components except in bark and lateral roots. The pattern of potassium release was fastest in leaves (91.19%) in the first 151 days of the study, followed by reproductive parts (88.62%) and twigs < 5 mm (64.52%). At the end of the study, release of potassium was observed between 93.41% (Reproductive parts) and 77.21% (Lateral roots) (Figure 5).
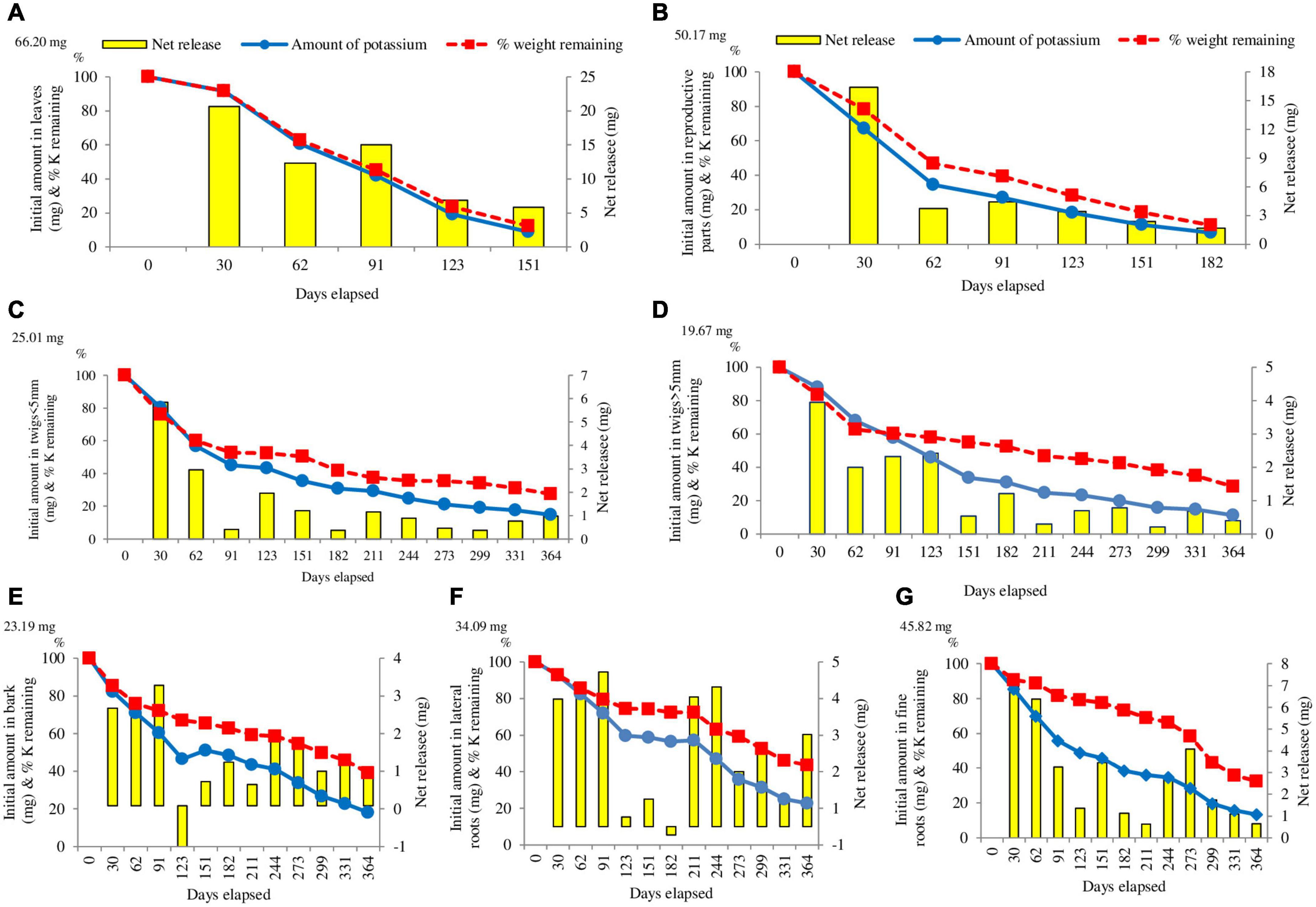
Figure 5. Changes in the absolute amount of potassium in (A) leaves, (B) reproductive parts, (C) twigs < 5 mm, (D) twigs > 5 mm, (E) bark, (F) lateral roots, (G) fine roots mass enclosed in litter. The initial mass of Potassium is given on the left side of the Y-axis. The columns indicate the net change between the measurements (right Y-axis). The broken lines indicate the percentage weight remaining of litter (n = 3).
Discussion
The order of the different components on the basis of decomposition rate was: Leaves > Reproductive parts > Twigs (< 5 mm) > Twigs (> 5 mm) > Bark > Fine roots > Lateral roots. Among all the components, leaves decomposed within 6 months of the sample placement on site because of its high surface area and thinness of leaves (0.54% day–1), whereas lateral roots only decomposed 46.55% with the slowest decomposition rate (0.14% day–1). High value of decay rate coefficient (k) indicates high rate of decomposition. The higher values of k for leaves (0.003–0.014) and reproductive parts (0.002–0.010) indicated that these components decomposed faster while lowest values of k reported for lateral roots (0.001–0.002) indicated its slow decomposition.
Decomposition and mass loss
It has been shown that the labile fractions of litter provide a readily available source of energy for the decomposer; moreover, the nutrients are easily leached. Nutrient concentration should therefore, be most influential in determining the rate of decomposition in the initial days (Siqueira et al., 2022). On the sites where understory vegetation is available in ample amount, it helps soil to retain moisture for longer period of time and eventually helps microbes responsible for decomposition to flourish (Su et al., 2022). Differences in the rate of decomposition of leaf have been reported by several authors, e.g., Bargali S. S. et al. (2015) and Joshi and Garkoti (2020) (Table 5).
In conformity to earlier studies among all the components (Marchante et al., 2019; Akoto et al., 2022), the higher weight loss was recorded during rainy season followed by summer and winter season. The greater decomposition in rainy season may be due to the presence of pronounced microbial activity under favorable conditions, i.e., availability of ample moisture and optimum temperature that accentuated due to rainfall (Krishna and Mohan, 2017; Tamura et al., 2017). They also stated that indirectly, climate could affect the litter decomposition by interacting with plant physiology and nutrients re-absorption, thus, modulating the chemical composition of plant litter at its formative stages. Decomposition of litter continued throughout the study period because of microbial activity depending on the conditions available for them. Actinomycetes, bacteria, algae and fungi may be the major soil fauna and microbes which directly affect the litter decomposition (Berg and McClaugherty, 2014) and their abundance and hierarchy in the soil would be very vital for their role on decomposition rate (Bargali S. S. et al., 2015).
Decomposition rate is markedly affected by water soluble compounds and leachable substances and nutrients in fresh litter. In the present study, components like leaves and reproductive parts showed similar pattern while twigs and roots follow almost different pattern. Overall this species showed higher decomposition rate as compared to the other species of the region.
t50 and t95 for different components ranged from 50 to 327 and 216–1,414 days, respectively (Table 4) as it depends on various factors such as biochemistry of components, behavior of soil and edaphic factors. The higher t50 and t95 reported by Bohara et al. (2019) were 456–569 and 3,289–4,125, respectively, in shrubland of Taihang Mountain, North China; Yang et al. (2021) reported 880–1,529 for t50 and 3,938–6,563 days for t95 and Huang et al. (2011) reported 324–511 days for t50 and 1,405–2,119 days for t95. Caliman et al. (2020) reported 84–284 days for t50 days and 372–1,230 days for t95 for multipurpose trees in Central India, which was lower than reported in the present study. Presence of favorable condition for decomposition may reduce the t50 and t95 time and lower value of t50 and t95 depicts that the site has a very active cycling of nutrients and dry matter.
To determine the temporal pattern of weight loss, the percent weight remaining was regressed against the time (days) elapsed (Table 6). A negative relationship between percent weight remaining and time elapsed was recorded for all the components.
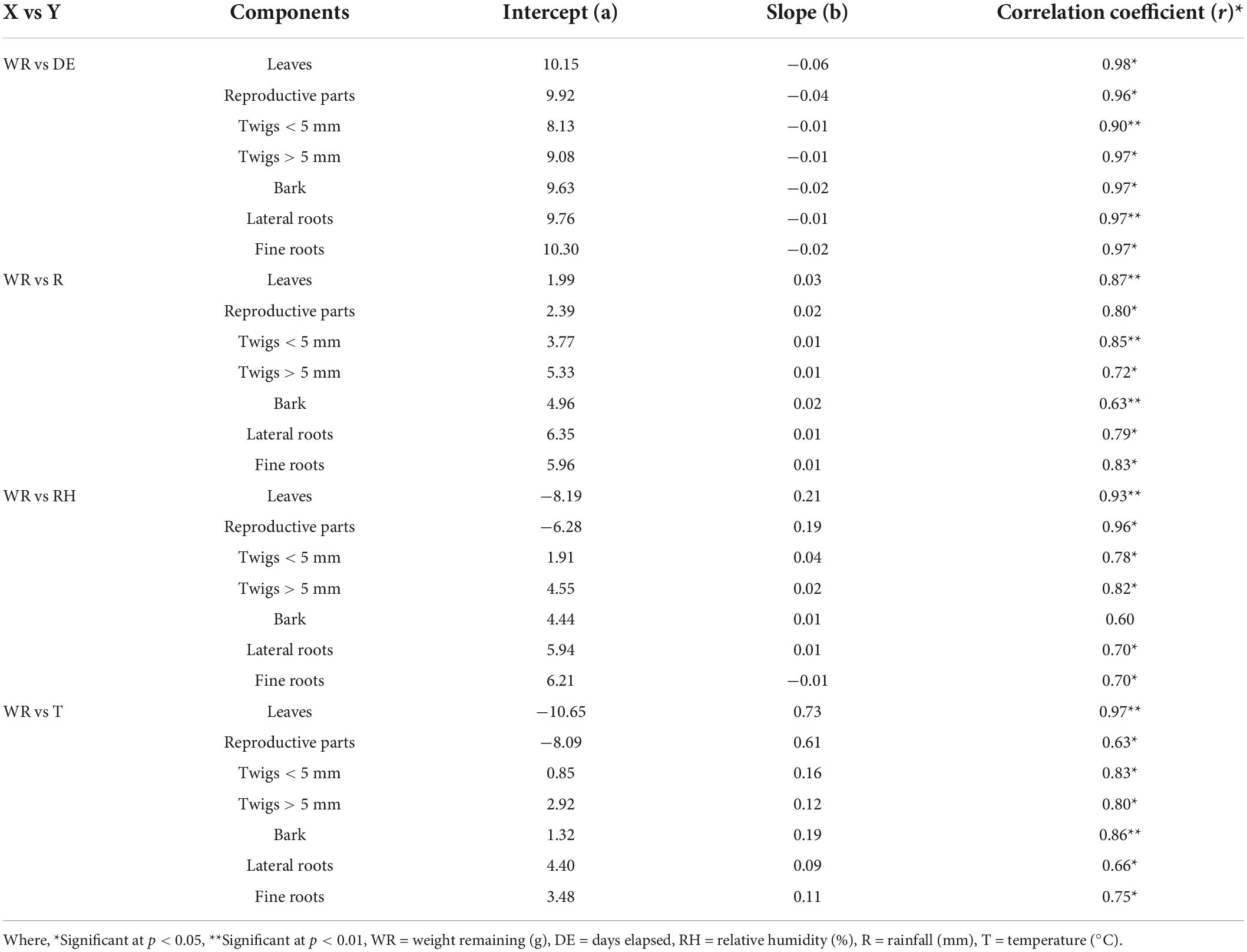
Table 6. Correlation coefficient between weight remaining, days elapsed, relative humidity, temperature, and rainfall.
Nutrient dynamics of decomposing litter
N and P concentrations (%) generally increased during different months till the termination of the experiment while the N and P content (mg) decreased due to release of nutrients during decomposition process (Figure 2). About 13–85% N and 22–75% P remained at the end of experiment for all litter types with highest release observed in the leaves. During the litter decomposition, K is released in accordance with the gradual process of leaching. The release of nutrients may contribute to nutrient conservation in degraded nutrient-poor sites (Manral et al., 2020). The observed pattern of initial N accumulation led to a net N release after 330 days, suggesting that immobilization of N was needed in this shrub until a critical concentration for decomposition was reached. The N and P immobilization indicate that leaching is not important for these two nutrients, whereas K has the significant leaching ability. By analyzing the nutrients remaining at the end of the decomposition process, the mobility of K was high followed by P and N in different components as also reported by Li et al. (2007) and Yang et al. (2022). Akoto et al. (2022) observation on a wide-ranging pattern of nutrient release from decomposing litter components includes an early immobilization of N and P often followed by net nutrient discharge. In contrast to our findings, an initial release phase followed by net accumulation for N and P was reported by Gaisie et al. (2016) in multipurpose trees.
Controlling factors
The decomposition during first 2 months was rapid because the molecules are easy to breakdown and rich in energy and the period of incubation was monsoon season (August) and the rainfall influenced the rate of decomposition by enhancing the activities of microorganisms. The greater weight loss recorded during rainy season may be due to optimum moisture, soil temperature and leaching of water soluble substances from the litter mass. Lower weight loss during winter season may be due to cool and dry conditions. However, in the later stages of litter decomposition, weight loss was decreased because the breakdown of lignin was much slower (due to very large and complex molecules) and the period of decomposition was post monsoon, i.e., winter and summer seasons where precipitation was not enough to support decomposition. The positive correlation between the weight remaining with rainfall temperature, humidity and rainfall also supported these finding (Table 6). Among the three environmental drivers, the litter decomposition was most affected by rainfall as compared to the other two drivers, i.e., relative humidity and temperature.
The components of C. nepalensis released nutrients (N, P, and K) at different rates, depending on their initial N, P, and K concentration (Figure 2). As compared to other components, leaves and reproductive parts with high N concentration showed fast decomposition. Previous studies (Lv et al., 2013; Zhang et al., 2021) also reported lower initial nitrogen content for slow decomposing litter. However, high N concentration and slow decomposition rate recorded for components like fine and lateral roots suggested that higher N concentration is not the only criteria that determine the rate of decomposition (Tu et al., 2014; Zhu et al., 2016; Zhang et al., 2021). Other decomposition studies (Silveira et al., 2011) also indicated that the decomposition of plant litter was related more to initial C quality, such as lignin concentration and acid-soluble carbohydrates than to the relative availability of N.
Significant (p < 0.01) inverse linear relationship between N and P concentration in residual material and percent weight remaining for all components was observed (Table 7). Galloway et al. (2008) and Allison et al. (2009) have stated that this relationship to be inversely linear in case where physical removal of material from bag is minimized which was also observed in our study.
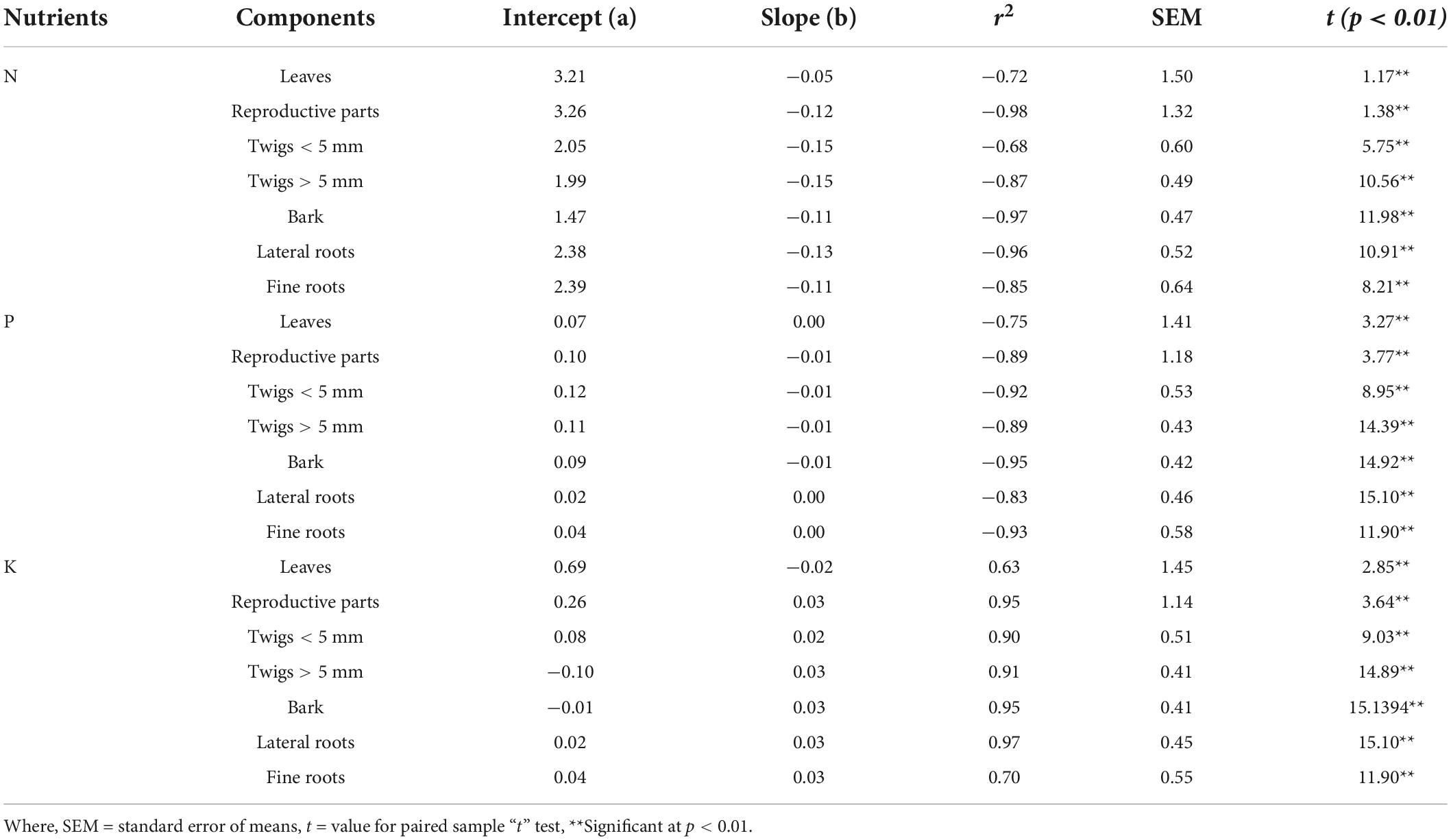
Table 7. Regression coefficient (r2) and t value between weight loss (g) and Nitrogen (N), Phosphorus (P), and Potassium (K) concentration (%).
Conclusion
Litter decomposition is a critically fundamental process that determines the potential return of organic matter and nutrients to the soil. Different litter components of C. nepalensis recorded 57–100% mass loss during 1 year period suggesting that organic matter and nutrients provided by decomposing litter can improve soil conditions in degraded hills. Low soil availability of N and P seems to be the major constraints in reclamation of degraded hills. Through litter decomposition and consequent nutrient release C. nepalensis can ameliorate soil conditions. In addition, the ability of C. nepalensis to form symbiotic association with nitrogen-fixing actinomycetes Frankia and arbuscular mycorhizal fungi makes it suitable for the management of fragile hills specially in regeneration and conservation of plant biodiversity in Kumaun Himalaya, India. Climate change could have positive or negative impact on litter decomposition or mass loss of litter depending on the species composition as well as environmental variables. Optimum conditions are needed for quick or healthy decomposition but conditions like prolong drought or global warming can reduce the litter decomposition because these conditions are not suitable for decomposers to grow in ample amount. In long run, the climate change could also influence the growth and chemical properties of the plant which also ultimately influence the decomposition rate of the plant.
Data availability statement
The original contributions presented in this study are included in the article/supplementary material, further inquiries can be directed to the corresponding author/s.
Author contributions
PA collected the data and prepared the first draft. KK helped in manuscript preparation and analysis. KB and SB guided the research, reviewed, and modified the manuscript. All authors contributed to the article and approved the submitted version.
Acknowledgments
The authors are thankful to the Head, Department of Botany, D.S.B. Campus, Kumaun University, Nainital, Uttarakhand for providing all sorts of facilities. Haripriya Pathak (Associate Professor-English, Kumaun University, Nainital, Uttarakhand, India) is highly acknowledged for the language correction. The authors are also thankful to the editor and the reviewers for their constructive comments which improved the quality of our manuscript.
Conflict of interest
The authors declare that the research was conducted in the absence of any commercial or financial relationships that could be construed as a potential conflict of interest.
Publisher’s note
All claims expressed in this article are solely those of the authors and do not necessarily represent those of their affiliated organizations, or those of the publisher, the editors and the reviewers. Any product that may be evaluated in this article, or claim that may be made by its manufacturer, is not guaranteed or endorsed by the publisher.
References
Akoto, D. S., Partey, S. T., Abugre, S., Akoto, S., Denich, M., Borgemeister, C., et al. (2022). Comparative analysis of leaf litter decomposition and nutrient release patterns of bamboo and traditional species in agroforestry system in Ghana. Clean. Mater. 4:100068. doi: 10.1016/j.clema.2022.100068
Allison, S. D., LeBauer, D. S., Ofrecio, M. R., Reyes, R., Ta, A. M., and Tran, T. M. (2009). Low levels of nitrogen addition stimulate decomposition by boreal forest fungi. Soil Biol. Biochem. 41, 293–302. doi: 10.1016/j.soilbio.2008.10.032
Awasthi, P., Bargali, K., and Bargali, S. S. (2022a). Relative performance of woody vegetation in response to facilitation by Coriaria nepalensis in Central Himalaya, India. Russ. J. Ecol. 53, 191–203. doi: 10.1134/S1067413622030031
Awasthi, P., Bargali, K., Bargali, S. S., and Jhariya, M. K. (2022b). Structure and functioning of Coriaria nepalensis dominated shrublands in degraded hills of Kumaun Himalaya. I. Dry matter dynamics. Land Degrad. Dev. 33, 1474–1494. doi: 10.1002/ldr.4235
Awasthi, P., Bargali, K., Bargali, S. S., Khatri, K., and Jhariya, M. K. (2022c). Nutrient partitioning and dynamics in Coriaria nepalensis Wall dominated shrublands of degraded hills of Kumaun Himalaya. Front. For. Glob. Change 5:913127. doi: 10.3389/ffgc.2022.913127
Banerjee, P., and Prasad, B. (2020). Determination of concentration of total sodium and potassium in surface and ground water using a flame photometer. Appl. Water Sci. 10:113. doi: 10.1007/s13201-020-01188-1
Bargali, K., Maurya, N. R., and Bargali, S. S. (2015a). Effect of a nitrogen-fixing actinorhizal shrub on herbaceous vegetation in a mixed conifer forest of Central Himalaya. Curr. World Environ. 10, 957–966. doi: 10.12944/CWE.10.3.27
Bargali, K., Manral, V., and Bargali, S. S. (2015b). Weight loss pattern in decomposing litter of Coriaria nepalensis, an actinorhizal shrub from degraded land. Indian J. Agric. Sci. 85, 270–273.
Bargali, S. S., Shukla, K., Singh, L., and Ghosh, L. (2015). Leaf litter decomposition and nutrient dynamics in four tree species of dry deciduous forest. Trop. Ecol. 56, 57–66.
Bargali, S. S. (1996). Weight loss and nitrogen release in decomposing wood litter in an age series of eucalypt plantation. Soil Biol. Biochem. 28, 699–702.
Berg, B., and McClaugherty, C. (2014). Plant Litter: Decomposition, Humus Formation, Carbon Sequestration. Berlin: Springer-Verlag, doi: 10.1007/978-3-662-05349-2
Bohara, M., Yadav, R. K. P., Dong, W., Cao, J., and Hu, C. (2019). Nutrient and isotopic dynamics of litter decomposition from different land uses in naturally restoring Taihang Mountain, North China. Sustainability 11:1752. doi: 10.3390/su11061752
Bradford, M. A., Berg, B., Maynard, D. S., Wieder, W. R., and Wood, S. A. (2016). Understanding the dominant controls on litter decomposition. J. Ecol. 104, 229–238. doi: 10.1111/1365-2745.12507
Bradford, M. A., Veen, G. F., Bonis, A., Bradford, E. M., Classen, A. T., Cornelissen, J. H. C., et al. (2017). A test of the hierarchical model of litter decomposition. Nat. Ecol. Evol. 1, 1836–1845. doi: 10.1038/s41559-017-0367-4
Caliman, J. P., Godinho, T. O., Caldeira, M. V. W., Rocha, J. H. T., Drury, M. L., and Castro, K. C. (2020). Seasonal pattern of nutrient cycling in the Atlantic Forest across a topographic gradient. Sci. For. 48:e3200. doi: 10.18671/scifor.v48n125.23
Farooq, T. H., Li, Z., Yan, W., Shakoor, A., Kumar, U., Shabbir, R., et al. (2022). Variations in litter fall dynamics, C:N:P stoichiometry and associated nutrient return in pure and mixed stands of camphor tree and masson pine forests. Front. Environ. Sci. 10:903039. doi: 10.3389/fenvs.2022.903039
Gaisie, E., Saddick, A., Agyeman, K., Adjei-Gyapong, T., and Quansah, G. (2016). Leaf decomposition and the nutrients release from multipurpose tree for crop production. Int. J. Sci. Res. Sci. Eng. Technol. 2, 345–352.
Galloway, J. N., Townsend, A. R., Erisman, J. W., Bekunda, M., Cai, Z., Freney, J. R., et al. (2008). Transformation of the nitrogen cycle: Recent trends, questions, and potential solutions. Science 320, 889–892. doi: 10.1126/science.1136674
Guo, C., Cornelissen, J. H. C., Zhang, Q. Q., and Yan, E. R. (2019). Functional evenness of N-to-P ratios of evergreen-deciduous mixtures predicts positive non-additive effect on leaf litter decomposition. Plant Soil 436, 299–309. doi: 10.1007/s11104-018-03925-7
Hobbie, S. E., Eddy, W. C., Buyarski, C. R., Adair, E. C., Ogdahl, M. L., and Weisenhorn, P. (2012). Response of decomposing litter and its microbial community to multiple forms of nitrogen enrichment. Ecol. Monogr. 82, 389–405. doi: 10.1890/11-1600.1
Huang, Y., Michel, K., An, S. Z., and echmeister-Boltenstern, S. (2011). Changes in microbial-community structure with depth and time in a chronosequence of restored grassland soils on the Loess Plateau in northwest China. J. Plant Nutr. Soil Sci. 174, 765–774. doi: 10.1002/jpln.201000397
Joshi, R. K., and Garkoti, S. C. (2020). Litter dynamics, leaf area index and forest floor respiration as indicators for understanding the role of Nepalese alder in white oak forests in central Himalaya, India. Ecol. Indic. 111:106065. doi: 10.1016/j.ecolind.2020.106065
Karki, H., Bargali, K., and Bargali, S. S. (2022). Dynamics of fine root and soil nitrogen in Mangifera indica based agroforestry systems in Central Himalaya, India. Land Degrad. Dev. 1–16. doi: 10.1002/ldr.4406
Krishna, M. P., and Mohan, M. (2017). Litter decomposition in forest ecosystems: A review. Energy Ecol. Environ. 2, 236–249. doi: 10.1007/s40974-017-0064-9
Kumar, M., Kumar, M., Pandey, R., Zhi-Guo, Y., and Cabral-Pinto, M. (2021). Forest soil nutrient stocks along altitudinal range of Uttarakhand Himalayas: An aid to nature based climate solutions. Catena 207:105667. doi: 10.1016/j.catena.2021.105667
Kumar, M., Kumar, A., Thakur, T. K., Sahoo, U. K., Kumar, R., Konsam, B., et al. (2022). Soil organic carbon estimation along an altitudinal gradient of chir-pine forests of Garhwal Himalaya, India: A field inventory to remote sensing approach. Land Degrad. Dev 1–14. doi: 10.1002/ldr.4393
Lanuza, O., Casanoves, F., Delgado, D., and Van den Meersche, K. (2019). Leaf litter stoichiometry affects decomposition rates and nutrient dynamics in tropical forests under restoration in Costa Rica. Restor. Ecol. 27, 549–558. doi: 10.1111/rec.12893
Li, H. T., Yu, G. R., Li, J. Y., Liang, T., and Chen, Y. R. (2007). Dynamics of litter decomposition and phosphorus and potassium release in Jinggang Mountain region of Jiangxi Province, China. Ying Yong Sheng Tai Xue Bao 18, 233–240.
Li, R., Yang, Q., Guan, X., Chen, L., Wang, Q., Wang, S., et al. (2022). High quality litters with faster initial decomposition produce more stable residue remaining in a subtropical forest ecosystem. Catena 213:106134. doi: 10.1016/j.catena.2022.106134
Liu, S., Yang, R., Peng, X., Hou, C., Ma, J., and Guo, J. (2022). Contributions of plant litter decomposition to soil nutrients in ecological tea gardens. Agriculture 12:957. doi: 10.3390/agriculture12070957
Lv, Y., Wang, C., Wang, F., Zhao, G., Pu, G., Ma, X., et al. (2013). Effects of nitrogen addition on litter decomposition, soil microbial biomass, and enzyme activities between leguminous and non-leguminous forests. Ecol. Res. 28, 793–800. doi: 10.1007/s11284-013-1060-y
Majila, B. S., Joshi, G. C., and Kala, C. P. (2005). Patterns in litter fall and litter decomposition along an altitudinal gradient in the Binsar Wildlife Sanctuary, Central Himalaya. Int. J. Sustain. Dev. World Ecol. 12, 205–212. doi: 10.1080/13504500509469631
Makkonen, M., Berg, M. P., Handa, I. T., Hattenschwiler, S., van Ruijven, J., van Bodegom, P. M., et al. (2012). Highly consistent effects of plant litter identity and functional traits on decomposition across a latitudinal gradient. Ecol. Lett. 15, 1033–1041. doi: 10.1111/j.1461-0248.2012.01826.x
Manral, V., Bargali, K., Bargali, S. S., Jhariya, M. K., and Padalia, K. (2022). Relationships between soil and microbial biomass properties and annual flux of nutrients in Central Himalayan forests, India. Land Degrad. Dev. 33, 2014–2025. doi: 10.1002/ldr.4283
Manral, V., Bargali, K., Bargali, S. S., and Shahi, C. (2020). Changes in soil biochemical properties following replacement of Banj oak forest with Chir pine in Central Himalaya, India. Ecol. Process. 9:30. doi: 10.1186/s13717-020-00235-8
Marchante, E., Marchante, H., Freitas, H., Kjoller, A., and Struwe, S. (2019). Decomposition of an N-fixing invasive plant compared with a native species: Consequences for ecosystem. Appl. Soil Ecol. 138, 19–31. doi: 10.1016/j.apsoil.2019.02.016
Monroy, S., Larranaga, A., Martinez, A., Perez, J., Molinero, J., Basaguren, A., et al. (2022). Temperature sensitivity of microbial litter decomposition in freshwaters: Role of leaf litter quality and environmental characteristics. Microb. Ecol. [Epub ahead of print]. doi: 10.1007/s00248-022-02041-5
Mourya, N. R., Bargali, K., and Bargali, S. S. (2019). Impacts of Coriaria nepalensis colonization on vegetation structure and regeneration dynamics in a mixed conifer forest of Indian Central Himalaya. J. For. Res. 30, 305–317. doi: 10.1007/s11676-018-0613-x
Ndagurwa, H. G., Dube, J. S., and Mlambo, D. (2015). Decomposition and nutrient release patterns of mistletoe litters in a semi-arid savanna, southwest Z imbabwe. Aust. Ecol. 40, 178–185. doi: 10.1111/aec.12191
Olsen, S. R., Cole, C. V., Watanabe, F. S., and Dean, L. A. (1954). Estimation Of Available Phaosphorus In Soils By Extraction With Sodium Bicarbonate. Washington, DC: Department of Agriculture Circular, US, 939.
Olson, J. S. (1963). Energy storage and balance of producers and decomposers in ecological systems. Ecology 14, 322–331.
Petersen, R. C., and Cummins, K. W. (1974). Leaf processing in a woodland stream. Freshw. Biol. 4, 343–368.
Rai, I. D. (2012). Ecological Attributes Of Timberline Vegetation With Special Reference To Climatic Variability In Kedarnath Wildlife Sanctuary, Ph.D. thesis, Nainital: Kumaun University.
Rawat, N., Nautiyal, B. P., and Nautiyal, M. C. (2010). Litter decomposition rate and nutrient release from different litter forms in a Himalayan alpine ecosystem. Environmentalist 30, 279–288. doi: 10.1007/s10669-010-9275-8
Robertson, G. P., and Paul, E. A. (1999). “Decomposition and soil organic matter dynamics,” in Methods Of Ecosystem Science, eds O. E. Sala, R. B. Jackson, H. A. Mooney, and R. W. Howarth (Berlin: Springer Verlag), 104–116.
Sanderman, J., and Amundson, R. (2005). “Biogeochemistry of decomposition and detrital processing,” in Treatise on Geochemistry, eds H. D. Holland, W. H. Schlesinger, and K. K. Turekian (Amsterdam: Elsevier), 217–272. doi: 10.1016/B978-0-08-095975-7.00807-X
Santi, C., Bogusz, D., and Franche, C. (2013). Biological nitrogen fixation in non-legume plants. Ann. Bot. 111, 743–767. doi: 10.1093/aob/mct048
Santonja, M., Fernandez, C., Gauquelin, T., and Baldy, V. (2015). Climate change effects on litter decomposition: Intensive drought leads to a strong decrease of litter mixture interactions. Plant Soil 393, 69–82. doi: 10.1007/s11104-015-2471-z
Silveira, M. L., Reddy, K. R., and Comerford, N. B. (2011). Litter decomposition and soluble, carbon, nitrogen, and phosphorus release in a forest ecosystem. Open J. Soil Sci. 1, 86–96.
Singh, J. S., and Singh, S. P. (1992). Forests of Himalaya: Structure, Functioning and Impact of Man. Nainital: Gyanodaya Prakashan.
Siqueira, D. P., de Carvalho, G. C. M. W., de Souza Silva, J. G., Caldeira, M. V. W., and Barroso, D. G. (2022). Litter decomposition and nutrient release for two tropical N-fixing species in Rio de Janeiro, Brazil. J. For. Res. 33, 487–496. doi: 10.1007/s11676-021-01383-z
Smeti, E., von Schiller, D., Karaouzas, I., Laschou, S., Vardakas, L., Sabater, S., et al. (2019). Multiple stressor effects on biodiversity and ecosystem functioning in a Mediterranean temporary river. Sci. Total Environ. 647, 1179–1187. doi: 10.1016/j.scitotenv.2018.08.105
Steffen, W., Richardson, K., Rockstrom, J., Cornell, S. E., Fetzer, I., Bennett, E. M., et al. (2015). Planetary boundaries: Guiding human development on a changing planet. Science 347:1259855. doi: 10.1126/science.1259855
Steidinger, B. S., Crowther, T. W., Liang, J., Van Nuland, M. E., Werner, G. D., Reich, P. B., et al. (2019). Climatic controls of decomposition drive the global biogeography of forest-tree symbioses. Nature 569, 404–408. doi: 10.1038/s41586-019-1128-0
Su, Y., Ma, X., Gong, Y., Ahmed, Z., Han, W., Li, K., et al. (2022). Global patterns and drivers of litter decomposition under nitrogen enrichment: A meta-analysis. Front. For. Glob. Change 5:895774. doi: 10.3389/ffgc.2022.895774
Tamura, M., Suseela, V., Simpson, M., Powell, B., and Tharayil, N. (2017). Plant litter chemistry alters the content and composition of organic carbon associated with soil mineral and aggregate fractions in invaded ecosystems. Glob. Change Biol. 23, 4002–4018. doi: 10.1111/gcb.13751
Tan, B., Yin, R., Zhang, J., Xu, Z., Liu, Y., He, S., et al. (2021). Temperature and moisture modulate the contribution of soil fauna to litter decomposition via different pathways. Ecosystems 24, 1142–1156. doi: 10.1007/s10021-020-00573-w
Tu, L. H., Hu, H. L., Chen, G., Peng, Y., Xiao, Y. L., Hu, T. X., et al. (2014). Nitrogen addition significantly affects forest litter decomposition under high levels of ambient nitrogen deposition. PLoS One 9:e88752. doi: 10.1371/journal.pone.0088752
Turmel, M. S., Speratti, A., Baudron, F., Verhulst, N., and Govaerts, B. (2015). Crop residue management and soil health: A systems analysis. Agric. Syst. 134, 6–16. doi: 10.1016/j.agsy.2014.05.009
Wang, Y., Chang, S. X., Fang, S., and Tian, Y. (2014). Contrasting decomposition rates and nutrient release patterns in mixed vs singular species litter in agroforestry systems. J. Soils Sediments 14, 1071–1081. doi: 10.1007/s11368-014-0853-0
Wieczorek, D., Zyszka-Haberecht, B., Kafka, A., and Lipok, J. (2022). Determination of phosphorus compounds in plant tissues: From colourimetry to advanced instrumental analytical chemistry. Plant Methods 18:22. doi: 10.1186/s13007-022-00854-6
Yang, K., Zhu, J., Zhang, W., Zhang, Q., Lu, D., Zhang, Y., et al. (2022). Litter decomposition and nutrient release from monospecific and mixed litters: Comparisons of litter quality, fauna and decomposition site effects. J. Ecol. 110, 1673–1686. doi: 10.1111/1365-2745.13902
Yang, R., Dong, J., Li, C., Wang, L., Quan, Q., and Liu, J. (2021). The decomposition process and nutrient release of invasive plant litter regulated by nutrient enrichment and water level change. PLoS One 16:e0250880. doi: 10.1371/journal.pone.0250880
Zhang, J., Li, H., Zhang, H., Zhang, H., and Tang, Z. (2021). Responses of litter decomposition and nutrient dynamics to nitrogen addition in temperate shrublands of North China. Front. Plant Sci. 11:618675. doi: 10.3389/fpls.2020.618675
Keywords: decomposition, decay coefficient, degraded lands, litter bags, litter types, nutrient dynamics
Citation: Awasthi P, Bargali K, Bargali SS and Khatri K (2022) Nutrient return through decomposing Coriaria nepalensis litter in degraded hills of Kumaun Himalaya, India. Front. For. Glob. Change 5:1008939. doi: 10.3389/ffgc.2022.1008939
Received: 01 August 2022; Accepted: 24 August 2022;
Published: 13 September 2022.
Edited by:
Munesh Kumar, Hemwati Nandan Bahuguna Garhwal University, IndiaReviewed by:
Satish Garkoti, Jawaharlal Nehru University, IndiaAmit Kumar, Nanjing University of Information Science and Technology, China
Copyright © 2022 Awasthi, Bargali, Bargali and Khatri. This is an open-access article distributed under the terms of the Creative Commons Attribution License (CC BY). The use, distribution or reproduction in other forums is permitted, provided the original author(s) and the copyright owner(s) are credited and that the original publication in this journal is cited, in accordance with accepted academic practice. No use, distribution or reproduction is permitted which does not comply with these terms.
*Correspondence: Surendra Singh Bargali, c3VyZW5kcmFraXJhbkByZWRpZmZtYWlsLmNvbQ==
†ORCID: Pankaj Awasthi, orcid.org/0000-0002-0213-231X; Kiran Bargali, orcid.org/0000-0002-8554-8803; Surendra Singh Bargali, orcid.org/0000-0001-6341-0945; Kavita Khatri, orcid.org/0000-0002-8035-9632