- 1Institute of Marine Sciences, University of Portsmouth, Portsmouth, United Kingdom
- 2Centre for Blue Governance, University of Portsmouth, Portsmouth, United Kingdom
- 3Eden Project Learning, Green Build Hub, Eden Project, Bodelva, United Kingdom
- 4School of Biological and Marine Sciences, University of Plymouth, Plymouth, United Kingdom
- 5UK Centre for Ecology & Hydrology, Gifford, United Kingdom
- 6Centre for Aquatic Environments, University of Brighton, Brighton, United Kingdom
- 7Department of Landscape Management, Estonian University of Life Sciences, Tartu, Estonia
There has been limited research on the breakdown, recycling, and flux of carbon from large woody detritus (LWD) in mangrove forests. The breakdown of LWD is caused by guilds of terrestrial and marine biodegrading organisms that degrade wood at a range of rates and efficiencies. Spatial variations in environmental factors within mangroves affect the distribution and community of biodegrading organisms, which, in turn, impacts carbon flow and sequestration. We reveal the role of biodegrading organisms in LWD breakdown and the environmental factors that influence the distribution of biodegrading guilds within a mangrove forest in South East Sulawesi that supports a diversity of mangrove species typical of Indonesian mangrove forests, which constitute 20% of Global mangrove cover. Within the high intertidal regions, terrestrial biodegradation processes dominated upon LWD. After 12 months exposure on the forest floor, experimental wooden panels in these areas remained unchanged in mass and condition. In the low intertidal region, marine wood-boring animals belonging to the family Teredinidae were the dominant biodegraders of LWD, and their activity reduces LWD volume and speeds up the loss of LWD volume. More than 50% of the experimental wooden panels’ weight in these areas was lost after 12 months exposure on the forest floor. Although different biodegrading guilds occupy the same LWD niche, their distribution throughout the mangrove forest is influenced by inundation time. The change of biodegrading guilds within LWD between the terrestrial and the marine organisms was distinct, creating a biodegradation boundary in a distance as narrow as 1 m on the mangrove forest floor. These results are important, as rising sea levels have crucial implications for biodegrading guilds. A full understanding of factors affecting the biodegradation processes of LWD in mangrove forests is critical to accurately assess mangrove carbon stores and the fate of mangrove derived carbon.
Introduction
Mangrove forests form the characteristic vegetation of low wave energy tropical and subtropical shorelines. Due to their location they play an important role in land-to-sea transfer of organic carbon derived from the breakdown of vascular plant detritus (Cragg et al., 2020). Some of this detritus is brought by rivers, but much is derived from the high primary productivity of these forests (Duarte, 2017). Mangrove productivity is channeled into biomass compartments of leaves, branches, main stems and roots, with the proportion of woody tissue increasing as the tree grows, with over 70% of total biomass being composed of trunk wood in larger trees (Cintron and Schaeffer-Novelli, 1984; Ong et al., 2004). These biomass components eventually fall to the forest floor, where they are processed in situ or tidally exported. In situ processing drives the remarkably high capacity of mangrove forests to store carbon in their sediments (Donato et al., 2011; Breithaupt et al., 2012; Sanderman et al., 2018) where anoxic conditions in fine grained sediment tend to limit further breakdown (Jennerjahn, 2020; Kauffman et al., 2020). Crabs can play a major role in leaf breakdown (Thongtham et al., 2008). However, the fate of mangrove carbon derived from large woody detritus (LWD) is less understood.
Woody tissues can represent a high proportion of the above ground biomass of mangroves that increases as the tree grows (Rovai et al., 2021), but woody components are shed in increasing amounts throughout the life of the tree and eventually the tree falls to the forest floor where it will be partially submerged during the tidal cycle (Cragg et al., 2020). Measures of the woody component of litter fall at multiple sites in tropical Australia indicate that woody tissues represent 8–15% of total small litter fall (Duke et al., 1981). Litter traps are not designed to capture the rare events of falling trees or large branches, so the main input of wood onto the forest floor can only be measured by surveys of LWD, which show that quantities vary widely between sites (Allen et al., 2000; Donato et al., 2011). Kauffman et al. (2020) undertook a remarkable systematic study of organic carbon in mangrove forests at numerous sites around the world. They report that LWD (downed wood in their terminology) generally constitutes less than 10% of the above ground C store in live and dead tissue, with quantities ranging from 0 to 150 Mg C ha–1, with the upper values coming from forests where LWD quantities exceed standing biomass.
Large woody detritus enhances soil pedogenesis, provides habitat for both germinating seeds (Allen et al., 2000; Krauss et al., 2005) and animals (Hendy et al., 2013, 2014), and increases the mangrove forest nitrogen budget (Robertson and Daniel, 1989), thus contributing to ecosystem function and productivity. LWD also plays a crucial role in carbon flow and storage within mangrove ecosystems. Carbon fluxes originating from LWD within mangrove ecosystems are driven by fungi and woodborers, depending on the mangrove tidal zone (Kohlmeyer et al., 1995). These biodegrading organisms promote fragmention of LWD (Filho et al., 2008), facilitating incorporation into anoxic sediments, where the majority of mangrove carbon typically resides (Kauffman et al., 2020) and is generally slow to degrade due to mostly anoxic conditions (Jennerjahn, 2020). However, there are few studies on biodegradation of LWD in mangroves, and the processes of LWD biodegradation are complex and require the ability to unlock the enzyme-recalcitrant lignocellulose complex characteristic of woody plants (Cragg et al., 2020).
Rates of wood biodegradation vary between tidal zones within mangrove forests (Kohlmeyer et al., 1995). In the high intertidal region of a mangrove forest, decay of LWD is slow (Benner and Hodson, 1985) and caused mainly by white and brown rot fungi (basidiomycetes) (Kohlmeyer et al., 1995) and termites (Vane et al., 2013). In the mid to low intertidal regions, degradation of LWD is rapid (Middleton and McKee, 2001), driven mainly by teredinid bivalves commonly known as shipworms (Robertson and Daniel, 1989; Filho et al., 2008). Decay due to bacteria and ascomycete fungi also occurs in waterlogged wood, but at a much slower rate than that achieved by white and brown rot fungi (Singh et al., 2022).
Gradients of inundation and salinity may alter the distribution of biodegrading guilds as mangrove forests are habitats where terrestrial and marine influences interact. Teredinid wood-boring activity is particularly important in areas of mangrove forests with high levels of LWD (Robertson, 1990; Kohlmeyer et al., 1995). Teredinids convert fallen logs into fine fragments of fecal material (frass) that contribute to carbon cycling and reduce build-up of LWD in mangrove ecosystems (Filho et al., 2008). However, teredinids cannot tolerate the prolonged emersion that occurs in the high intertidal (Robertson, 1990). In the mid to low intertidal zones of a Rhizophora-dominated Australian mangrove forest where there is less organic content in the sediment, Robertson (1990) found that half of the original LWD was consumed by teredinids within 2 years, whereas in the high intertidal, where teredinids were absent, only 5% of the original mass of fallen logs was lost. Teredinids and isopod wood-borers are sensitive to salinity levels. In an extensive study of teredinids in LWD and wooden panels around Papua New Guinea, Rayner (1983) categorized the numerous teredinid species in these waters into stenohaline marine, euryhaline marine, euryhaline brackish water or stenohaline brackish water with the latter three categories occurring in mangrove ecosystems. Limnoriid crustaceans bore into wood in the intertidal zone, but are not tolerant of brackish conditions. They occur in the seaward regions of the forest investigated in this study (Cookson et al., 2012). Global-mean sea level (GMSL) has increased by approximately 1.5 mm yr–1 over the 20th century (Hay et al., 2015; Dangendorf et al., 2019) and is predicted to increase by 65 ± 12 cm by 2100 (Nerem et al., 2018). How climate change and sea level rise affect the distribution and activity of LWD biodegrading organisms, and by proxy, rates of carbon flow and carbon storage in mangrove ecosystems, is an open and urgent question. In the West Pacific region, geostrophic forcing causes seawater to accumulate along the western margin of the Pacific Basin, resulting in sea levels and rates of sea level rise in Southeast Asia that are higher than the global average (Weller et al., 2016). For example, the rate of sea-level rise in coastal Indonesia from 1992 to 2015 was approximately 7 mm per year (Surya et al., 2019), and climate change models predict a sea level rise of 74 cm by 2100 (Karondia et al., 2019) which would inundate large areas of coastal mangrove. Shifting tidal ranges will impact biodegradation of LWD through changes in the distribution of wood-boring guilds.
This study uses a mangrove ecosystem with a range of tree species and wood degrading organisms that are typical of the mesotidal mangrove forests of Indonesia (Cragg and Hendy, 2010), a country that contains 20% of the global mangrove forest area (Bunting et al., 2018). It aims to identify the organisms that initiate break down of woody detritus within four mangrove forests of Sulawesi, Indonesia, and to determine how tidal elevation, inundation and salinity affect their distribution and rates of biodegradation. Understanding the baseline structure of LWD biodegrading guilds and how they vary with tidal elevation, and how ecological conditions influence rates of biodegradation, is essential to understand carbon cycling and carbon sequestration in mangrove ecosystems.
Materials and Methods
Site Description
This study was undertaken in the mangroves situated in the north of Kaledupa Island located in the Wakatobi National Park, the third largest marine park in Indonesia. Average annual air and water temperatures vary between 26 and 28°C, and salinity in the mangroves varies between 1 and 40 psu. The mangroves in this area grow in shallow water with thin carbonate-rich sediments, patches of raised fossil coral occur in the mangroves and these impact the hydrodynamics and limit the development of mangrove roots. Dominant tree species in the seaward regions of these mangrove forests are Rhizophora stylosa, Sonneratia alba, and Bruguiera gymnorhiza, while the landward portions of the forest were dominated by Excoecaria agallocha, Xylocarpus spp., Rhizophora apiculata, and Heritiera littoralis (see Cragg and Hendy, 2010 for detailed site descriptions).
Four mangrove forests were studied: Langira, which is situated in the low-mid intertidal zone; Sombano, which is located at the high intertidal zone and separated from Langira by a coral rock plateau; and Laulua and Galua, which both extend across the high and low intertidal zones within the mangroves (Figure 1).
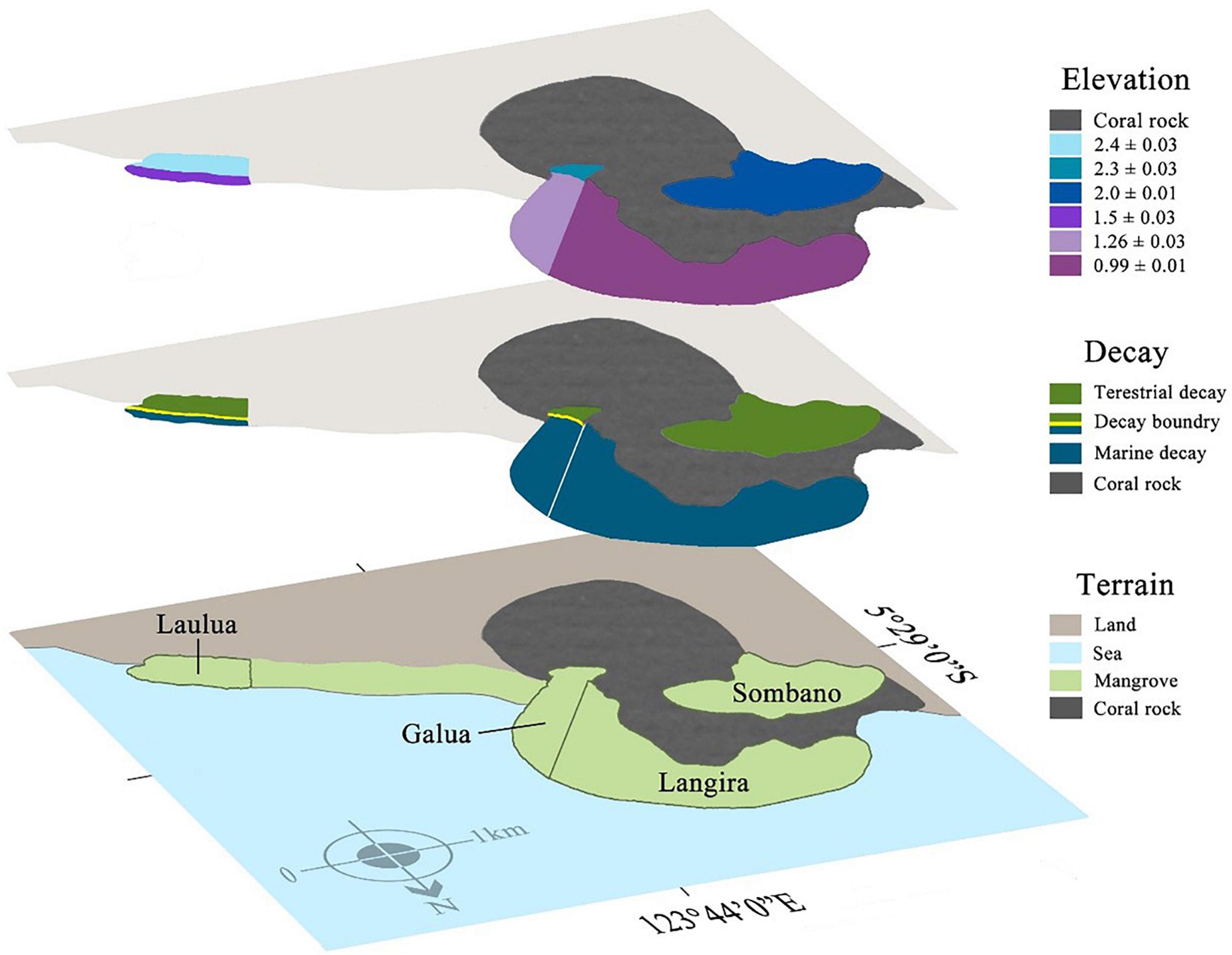
Figure 1. Biodegradation boundaries of large woody debris (LWD) within mangroves sites at Kaledupa Island, Indonesia. The three layers represent shore height elevation, the type of biodegradation process found on LWD and the type of terrain in each site. Each mangrove forest has been color-coded by the type of biodegradation process within that forest (terrestrial = green, marine = blue) and the height above mean sea level, elevation (meters mean ± SE) of each forest is also highlighted. The boundary between the terrestrial and marine biodegradation is indicated by the yellow line within the Galua and Laulua forests.
Large woody detritus in the Sombano site supports guilds of terrestrial wood degraders – basidiomycete fungi, termites and beetles which are absent in LWD in Langira site, where marine wood degraders, principally teredinids, dominate. These sites were used for comparison with the Laulua and Galua sites are home to both terrestrial and marine biodegraders of LWD, making identification of the environmental variables that determine differences between distribution of biodegrading guilds possible.
Wood Collection, Volume, and Wood Degradation Scale Classification
Five transects were established in the Sombano and Langira mangrove forests, extending from the low elevation to high elevation mangrove plant community fringes. Eight belt transects, each 20 m wide were used to characterize the biodegradation boundary in the Galua forest. Three belt transects, each 60 m wide were used for the Laulua forest. The belt transects began in areas dominated by terrestrial wood-degrading organisms, well above the high intertidal zones and ended in areas where marine wood-degrading organisms were the only organisms present at the seaward edges (Figure 1). Transect widths were determined by operational considerations regarding limitations imposed in some areas by the density of vegetation, particularly root systems, with sampling of some areas.
The volume of LWD was measured in 22 and 19 plots in Sombano and Langira respectively. The volume of LWD in the Galua and Laulua mangrove forests was measured in 24 and 9 plots respectively. All plots measured 20 × 20 m. All pieces of LWD, defined as being more than 2 cm in diameter, were measured to estimate their volume. Large woody items were collected at low tide from the transects. Within the Sombano and Langira forests values were given describing levels of degradation as defined in Table 1.
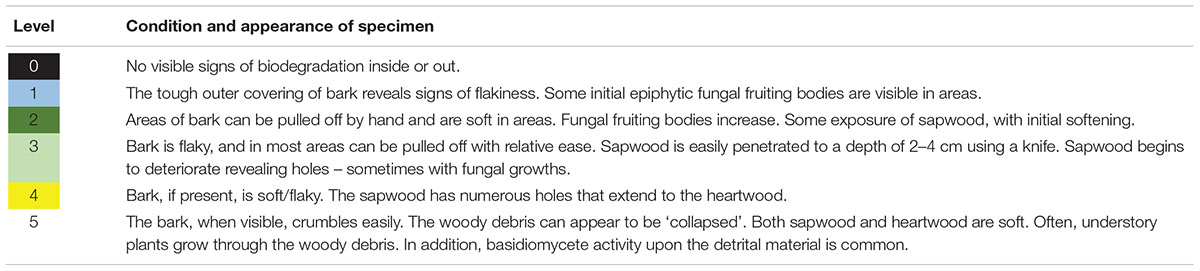
Table 1. Scale describing levels of fungal wood decay observed in large woody debris (LWD) in the Sombano and Langira mangrove forests. Note, level colors correspond to Figure 2A.
Rate of Wood-Loss in Panels
To assess the degree of teredinid activity, 12.5 cm × 11 cm × 2 cm panels of Bruguiera gymnorhiza were exposed in three of the mangrove forests. Twenty-five panels were deployed within both the Sombano and Langira mangrove forests, and sixty panels within the Galua forest. Fifteen panels were also located at the sub-tidal Kal 1 reef site adjacent to the Langira forest. Before field exposure, all panels were oven dried for 48 h at 60°C, weighed and then placed in the mangrove forests for 12 months, the weight loss of wood was determined using the oven-dry weight after exposure (48 h at 60°C). Panels were split and then imaged. The percentage of the sectional area of the panel represented by the teredinid tunnels was calculated using the digital analysis package ImageTool Version 3.00 (The University of Texas Health Science Centre at San Antonio). After exposure, panels were carefully split open to allow collection of calcareous pallets, the key taxonomic characters of teredinids. Pallets were counted and used to identify the teredinid species using the key in Turner (1971).
Panels were randomly placed at 0, 20, 100, 240, 380, and 500 m from the lowest elevation in areas of the Sombano mangrove forest. Then randomly placed at 20, 120, 140, 280, and 370 m within Langira. Within Sombano, panels were located at elevations >2 m relative to mean sea level. At Langira, panels were located at >2 m, 1.99–1.9 m, and <1.9 m relative to mean sea level, and in Galua, panels were located at >2.1 m, 2.1–2.0 m, 1.99–1.90 m and <1.90 m relative to mean sea level. Panels at the Kal 1 reef site were placed at 18 m depth. To determine the elevation above sea level along each mangrove transect the level of high tide was marked on mangrove trees using high visibility string. At low tide the distance from the substrate to the mark on the tree was measured and then subtracted from the height of high tide as given in the local tide tables (Figure 1).
Wood Degrading Guilds and Measurements of Environmental Variables
The Galua and Laulua mangrove forests were chosen to investigate the environmental variables that determine the boundary between terrestrial and marine wood-degrading guilds at sediment level (Figure 1) (terrestrial degraders are found in wood out of reach of the tides even at the seaward fringe). A total of 583 and 332 pieces of LWD were examined from the Galua and Laulua forests respectively. Volumes of LWD were calculated for every piece investigated. Emersion times for the LWD were estimated by relating their tidal height to data in local tide tables.
The Galua forest has a gradually inclining shoreline extending 900 m from the seaward edge to the strandline with an elevation range of 1.36 m from the high to low intertidal. The Laulua forest extends 220 m from the strandline out to the seaward edge. From 0 to 170 m the elevation above sea level ranged from 2.57 to 2.55 m elevation above sea level. From 170 to 220 m at the seaward edge the shore height elevation was recorded at 1.32 m above sea level – a drop in shore elevation of 1.23 m in 50 m.
In areas where LWD was surveyed, ground water salinities at low tide were measured using a Bellingham and Stanley E-Line Aquatic hand-held Refractometer and expressed in Practical Salinity Units (psu). In areas without water, a small hole was dug to access the ground water. All wood-degrading organisms from the LWD samples were extracted and identified.
Identification of Marine and Terrestrial Wood Degraders
Beetle (coleopteran) larvae, teredinid pallets and worker and soldier termites, were stored in absolute ethanol for later identification. Using an Olympus E-510 SLR with a 50mm Macro-lens, digital images of all bracket fungi and termites, focusing on morphology and color characteristics were used for identification.
Data Analysis
Differences in LWD volumes between mangrove forests at different tidal elevations were tested using a General Linear Model with site and tidal elevation as fixed factors. A one-way ANOVA was used to test for differences between the dry weights of panels and distance from the strandline. Regression analysis was used to determine relationships between the percentage of the cross-sectional area represented by teredinid tunnels and the level of fragmentation to each panel. Chi-Square tests were used to test for differences in teredinid settlement at different tidal elevations. A Kruskal–Wallis test was used to test for differences of wood-decay between mangrove sites. All percentage data were normalized using arcsin transformations, and count data were square root transformed. The suitability of transformations was scrutinized by examining residuals. Post hoc Tukey’s pairwise comparison tests separated values into statistically distinct subsets in all ANOVAs using MINITAB (MINITAB Inc, version 13.20, Pennsylvania, United States).
The univariate and non-parametric multivariate techniques of the distance-based linear modeling package (DISTLM) contained in PRIMER 6.1 (PrimerE Ltd, Albany, Auckland: Plymouth Routines in Multivariate Ecological Research) was used to explore relationships between wood-degrading guilds and the environmental variables emersion time and salinity. The relationships between distributions of wood-degrading guilds and environmental variables in different forests were examined using PERMANOVA, based on Bray-Curtis matrices. The DISTLM model produces a sequential test, which assess the variation each environmental variable has on its own, and a conditional test, assessing the variation of all the variables (McArdle and Anderson, 2001). The most parsimonious model for predicting the distribution of biodegrading organisms affected by emersion time and salinity was identified using the Akaike information criterion (AIC) and R2. AIC ranks models from all possible combinations of the environmental variables. The DISTLM was based on presence/absence data with 9999 permutations and a Sorensons transformation. Environmental variables were log transformed.
Results
Wood Degradation Within the Sombano and Langira Mangrove Forests
Levels of fungal and bacterial decay, as indicated by softening of 168 LWD samples and other features summarized in Table 1, were greatest within the Sombano forest (Figure 2A). Woody debris beyond 400–500 m was harder. With greater distances from the landward edge, the levels of fungal wood degradation decreased (Kruskal–Wallis test, degradation rating vs. distance from land, P ≤ 0.001).
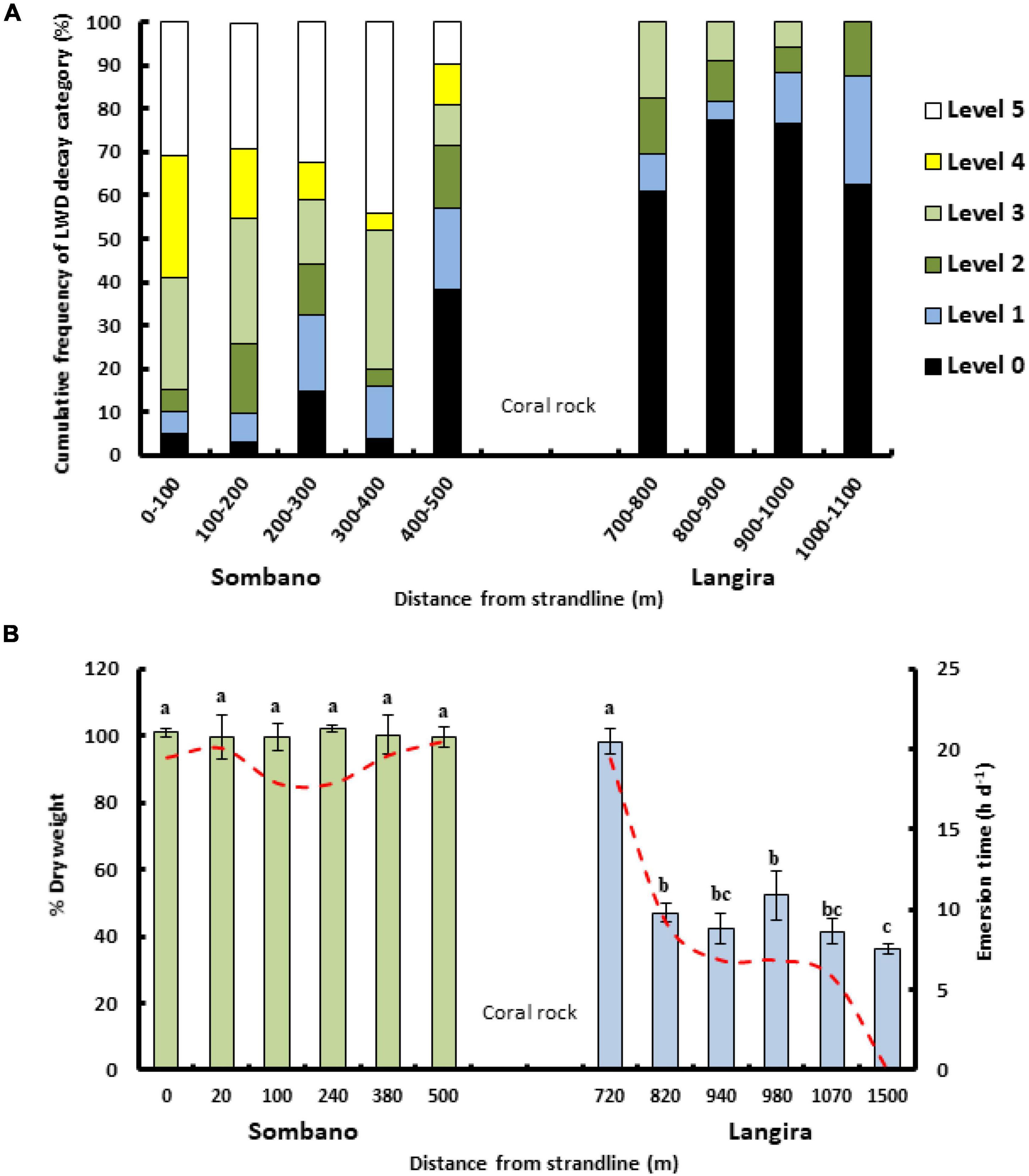
Figure 2. Wood decay levels correspond to emersion time and increasing distance from the land. (A) The frequency (%) of fungal decay levels assigned to LWD (n = 168) within Sombano and Langira. Decay levels correspond to the scale outlined in Table 1, with level 0 being the least decayed and level 5 the most decayed. (B) The percentage dry-weight of experimental wooden panels (mean ± SE) exposed for 12 months within different areas of the Sombano and Langira forests. Emersion times are represented by dashed line. Wood loss is from teredinid activity. Letters above the bars = post hoc Tukey’s pairwise test groupings.
Sombano and Langira Panel Analysis – Wood Loss
The percentage dry-weight loss of wood from panels was greatest in the Langira forest (Figure 2B). When emersion was more than 10 h per day, percent weight loss was low. When emersion was 10 h per day or less, weight loss was high (one-way ANOVA, distance from land vs. percentage dry weight; F11,48 = 178.75, p ≤ 0.001). Wood consumption rates increased with decreasing emersion time [Figure 2B, one-way ANOVA emersion time (h) vs. wood loss (g d–1) per panel, F11,48 = 178.75, p ≤ 0.001]. With increasing teredinid attack an increasing percentage of the whole panels broke up [Regression analysis, (y = 0.46x –7.29) R2 = 0.416, F1,58 = 41.3, p ≤ 0.001].
Wood-Biodegrading Organisms on Large Woody Detritus
Bracket Fungi
Bracket fungi were found only in the Sombano and Galua forests (Table 2). Both forests had mutually exclusive mycoflora except for Ganoderma sp. and Pycnoporus sanguineus, which were found in both forests (Figure 3A). The mycoflora consisted of 13 species belonging to eight families. Almost all bracket fungi within the Sombano and Galua forests were found on fallen tree species that were specific hosts for particular fungal species. For example, Schizophyllum commune was found only in the landward regions in the Galua forest on dead Xylocarpus granatum trees. Trametes elegans was found in the Sombano forest on Excoecaria agallocha and Bruguiera gymnorhiza wood.
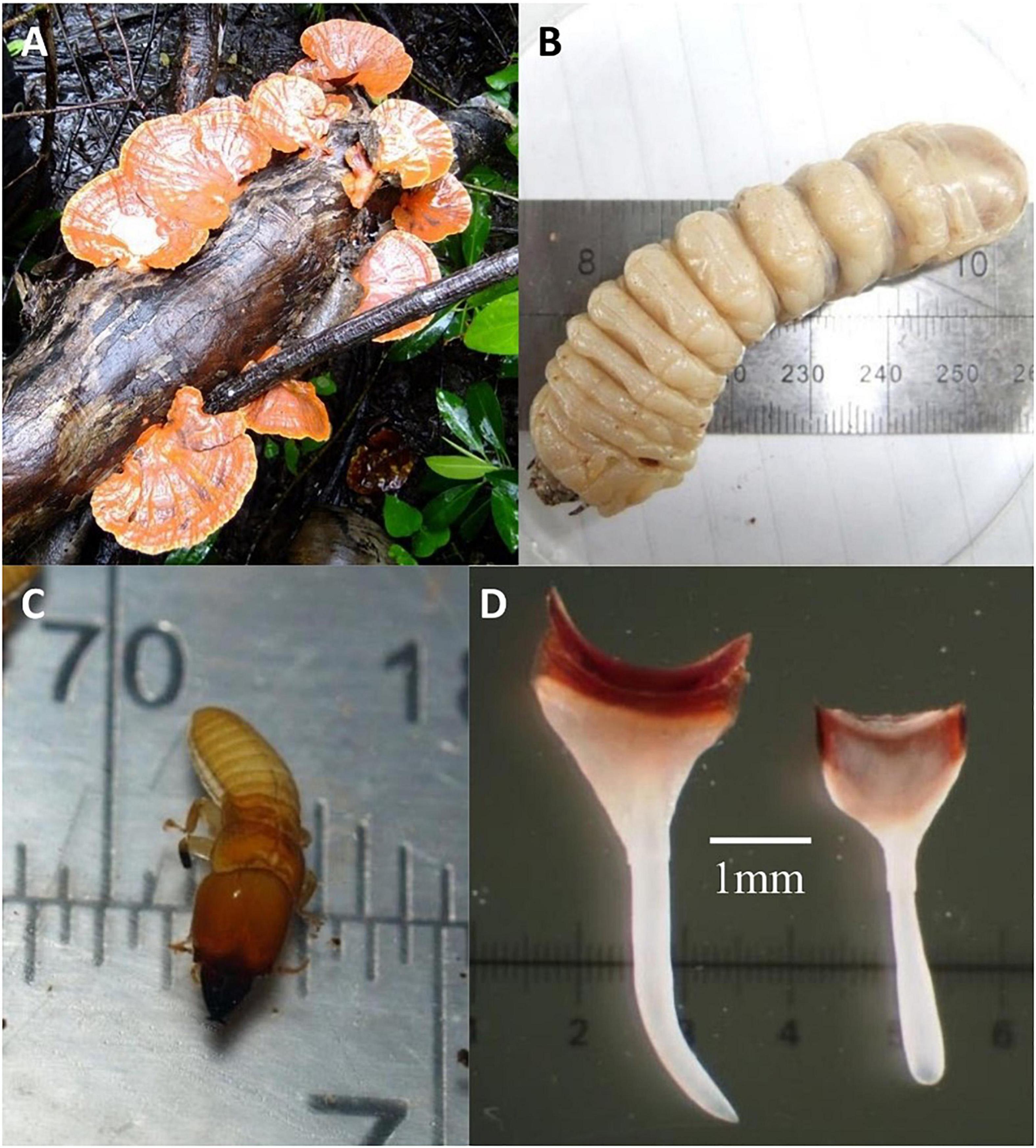
Figure 3. Wood degrading organisms found in mangrove forests of Kaledupa Island, Indonesia. (A) The bracket fungi, Pycnoporus sanguineus on a fallen Xylocarpus sp. tree in the Galua forest; (B) a beetle larva of the family Cerambycidae from the Galua forest; (C) frequently found in decaying mangrove wood, the termite Cryptotermes from the Galua and Laulua forests; (D) teredinid pallets belonging to Lyrodus massa removed from experimental wooden panels in the Langira mangrove forest.
Beetle (Coleopteran) Larvae
Larvae from four beetle families were found in the mid to high intertidal areas of the forests: the Cerambycidae, Elateridae, Oedemeridae, and Tenebrionidae (Figure 3B and Table 2), were found in the Sombano and Galua forests. The Oedemeridae were found in all sites except the Sombano forest. We found two items of LWD in the low intertidal containing oedemerid beetle larvae.
Termites
Five genera of termites from three families were found in the mid to high intertidal areas of the Sombano, Galua, and Laulua mangrove forests (Figure 3C and Table 2). Of the five genera, Cryptotermes, Coptotermes, Microcerotermes, and Nasutitermes were found in fallen wood in Galua and Laulua. Prorhinotermes were found only in the Galua forest. Prorhinotermes, Coptotermes, and Nasutitermes were found in arboreal nests above spring high tide.
Teredinids
Teredinids were recorded from all sites except Sombano (Table 2). Seven teredinid species were identified (Figure 3D): Bactronophorus thoracites, Dicyathifer mannii, Teredo furcifera, T. fulleri, T. mindanensis, Lyrodus massa, and Spathoteredo obtusa. Teredo furcifera were the most abundant teredinids found in the experimental panels and S. obtusa were the least abundant.
The Distribution of Wood-Degrading Guilds
Fallen wood in the Sombano forest was degraded by terrestrial organisms. Conversely, the Langira forest predominantly had marine wood-degraders with a few terrestrial wood degrading organisms found in the high intertidal areas. However, the Galua and Laulua mangrove forests contained both terrestrial and marine wood degrading organisms.
We found more than one type of wood-degrading organism processing wood in twenty-five items of LWD in the high intertidal region of Galua, with thirteen couplings of: termites and beetle larvae, eight items of LWD with termites and bracket fungi, and four items of LWD with beetle larvae and bracket fungi. In Laulua only one item of LWD in the high intertidal region contained termites and beetle larvae. More than 97% of LWD items in the Galua and Laulua forests contained only a single type of biodegrading organism.
The Volume of Large Woody Detritus in Mangrove Forests
The Sombano mangrove forest, in the high intertidal (>2 m above mean sea level) had the greatest volume of LWD per unit area (Figure 4, one-way ANOVA, LWD volume vs. site, F3,66 = 18.31, p ≤ 0.001). The volume of LWD from Sombano was 102.9 ± 19.5 m3 (mean ± SE) and the largest log recorded was 9.6 m3. The volume of LWD varied at different tidal elevations (ANOVA, LWD volume vs. tidal elevation, F3,66 = 9.58, p ≤ 0.001). In the high intertidal areas of the Langira, Galua, and Laulua mangrove forests, the volumes of LWD were significantly greater compared to those found toward to the seaward edge. The largest logs located in the Galua and Laulua mangrove forests at >2.0 m above sea level were 1.83 m3 and 0.5 m3 respectively, while the largest logs found in the low intertidal from Galua and Laulua measured 0.32 m3 and 0.05m3 respectively.
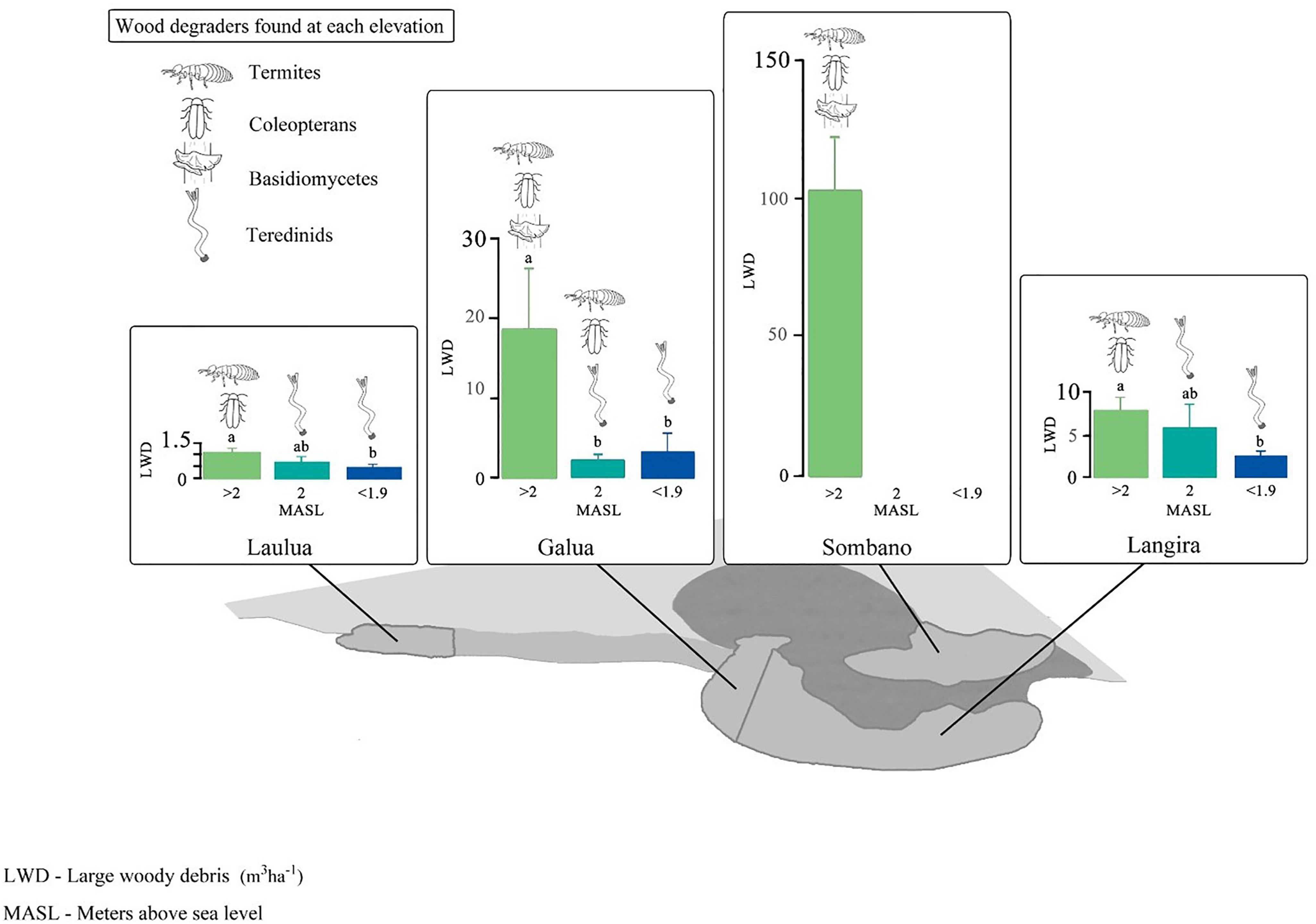
Figure 4. Guilds of wood-degrading organisms across four mangrove forests in Kaledupa Island, Indonesia. Letters above the bars represent differences according to post hoc Tukey’s pairwise tests. Note, the scales on the y-axes differ.
Effect of Environmental Variables Upon the Distribution of Wood-Degrading Guilds
In Galua, bracket fungi were found only at the strandline, where the greatest emersion time was recorded (one-way ANOVA, emersion time vs. biodegradation process; F3,442 = 318.52, p ≤ 0.001). Woody debris with mutually exclusive communities of either termites or beetle larvae were also found in these areas. There was no significant difference in emersion time of wood with termites or with beetle larvae. Similar results were found in the Laulua forest, with LWD containing mutually exclusive communities of either termites or beetle larvae. Wood containing teredinids had significantly lower emersion times than wood containing terrestrial wood-degrading organisms (one-way ANOVA, emersion time vs. biodegradation process; F2,103 = 211.77, p ≤ 0.001).
The elevation in the intertidal areas of each mangrove forest where terrestrial wood-degradation occurred was significantly higher than in areas of the forest exposed to marine biodegradation only (Figure 1, one-way ANOVA, elevation above sea level vs. intertidal zone, F5,644 = 236.84, p ≤ 0.001).
The Change Between Terrestrial and Marine Wood-Degrading Organisms
While the distance from the strandline of members of the wood degrading guilds varied between Galua and Laulua, a biodegradation-boundary marked by the sharp change between terrestrial and marine wood-degrading organisms in LWD was recorded within an elevation of 0.04 m of each other in the Galua and Laulua forests. The change between the terrestrial and marine consortia in the Galua and Laulua forests was remarkably similar, which occurred at 1.82 ± 0.01 (mean ± SE) m elevation above sea level and 1.78 ± 0.02 (mean ± SE) m elevation above sea level respectively (Figure 5, one-way ANOVA, sea level boundary change vs. site, F1,27 = 3, p ≥ 0.05).
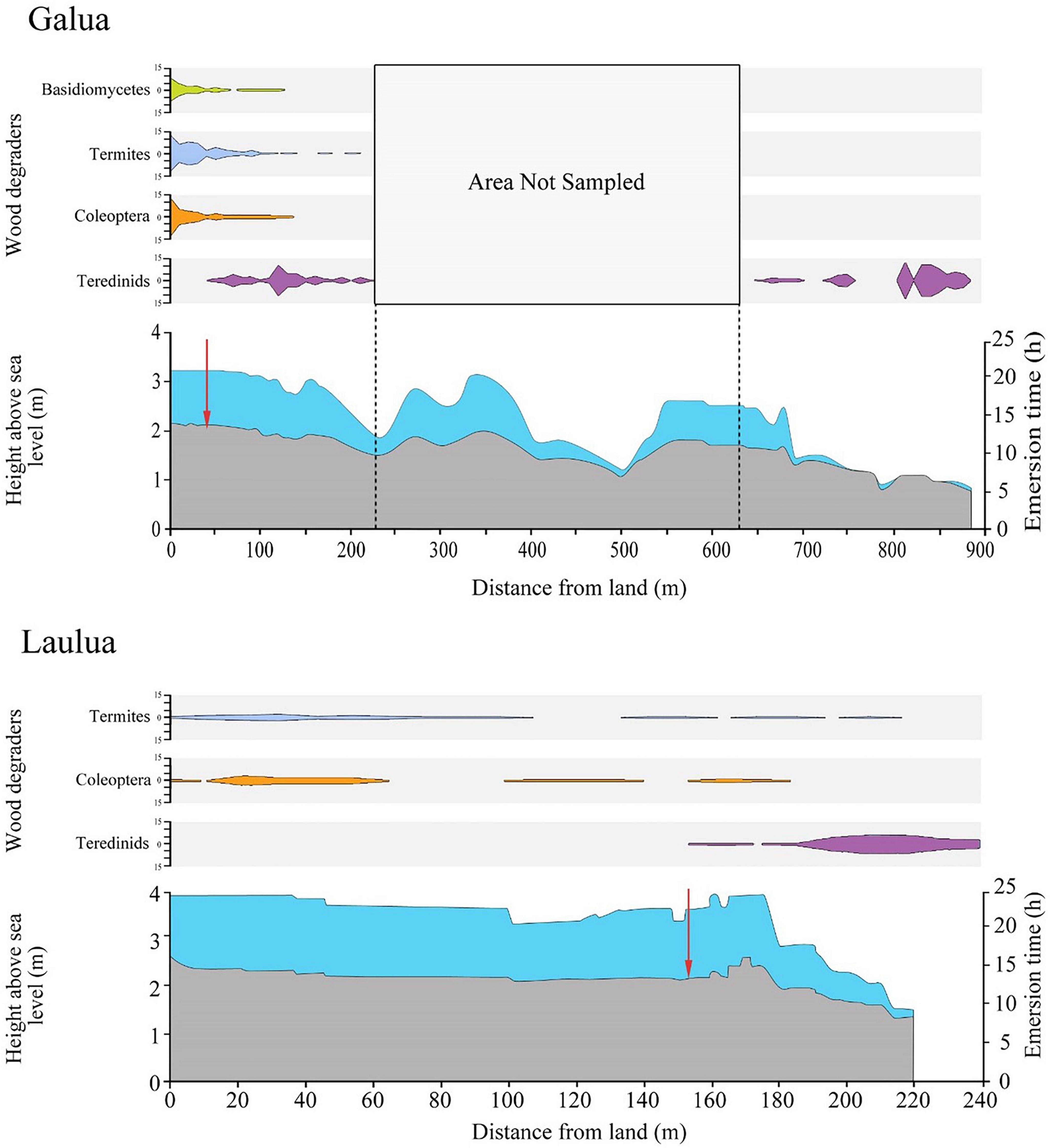
Figure 5. Elevation and wood-degrading guilds within Galua and Laulua mangrove forests. Scale bars for wood degrading guilds represent total abundance of LWD items found containing that guild. Red arrows represent biodegradation boundary between terrestrial and marine wood-degraders. LWD items with no visible degradation were not plotted, and the area between 220 and 600 m in Galua was not sampled. Strandline on x-axis = 0 m. Note, x-axes differ.
Comparisons of the Distribution of Wood Biodegrading Guilds Between Localities
Spatial distributions of wood biodegrading guilds between the Laulua and Galua mangrove forests varied significantly within each forest (PERMANOVA Pseudo-F1,550 = 56.9, p ≤ 0.001). In the Laulua forest, the most parsimonious model (AIC = 785.9, R2 = 0.49) for the wood-degrading guilds explained 49% of the variation, with emersion explaining 42% and salinity explaining 7% (DistLM sequential test, Pseudo-F ranging from 13.7 to 76; in all cases P ≤ 0.001). Emersion explained 98.9% of the total variation, followed by salinity with 1.1%.
In the Galua forest, the most parsimonious model (AIC = 3393.5, R2 = 0.36) for the variation in wood-degrading consortia explained 36% of that variation, with emersion explaining 35% followed salinity explaining 1% (DistLM sequential test, Pseudo-F ranging from 8.1 to 236; in all cases P ≤ 0.001). Emersion explained 98% of the total variation, followed by salinity with 2%.
Teredinid Settlement in Panels
No differences were found in teredinid recruitment to panels placed at intertidal regions in the Galua mangrove forest (Figure 6, Chi-Squared, P ≥ 0.05). However, a greater proportion of teredinid activity was recorded in panels in areas below the mid intertidal. The degraded panels in the high intertidal had minimal teredinid activity and they each contained one teredinid tunnel measuring between 0.02 and 0.2 cm2. Teredinid tunnels in panels in the mid to low intertidal regions occupied between 0.1 and 3.4 cm2 of the surface area of the panels.
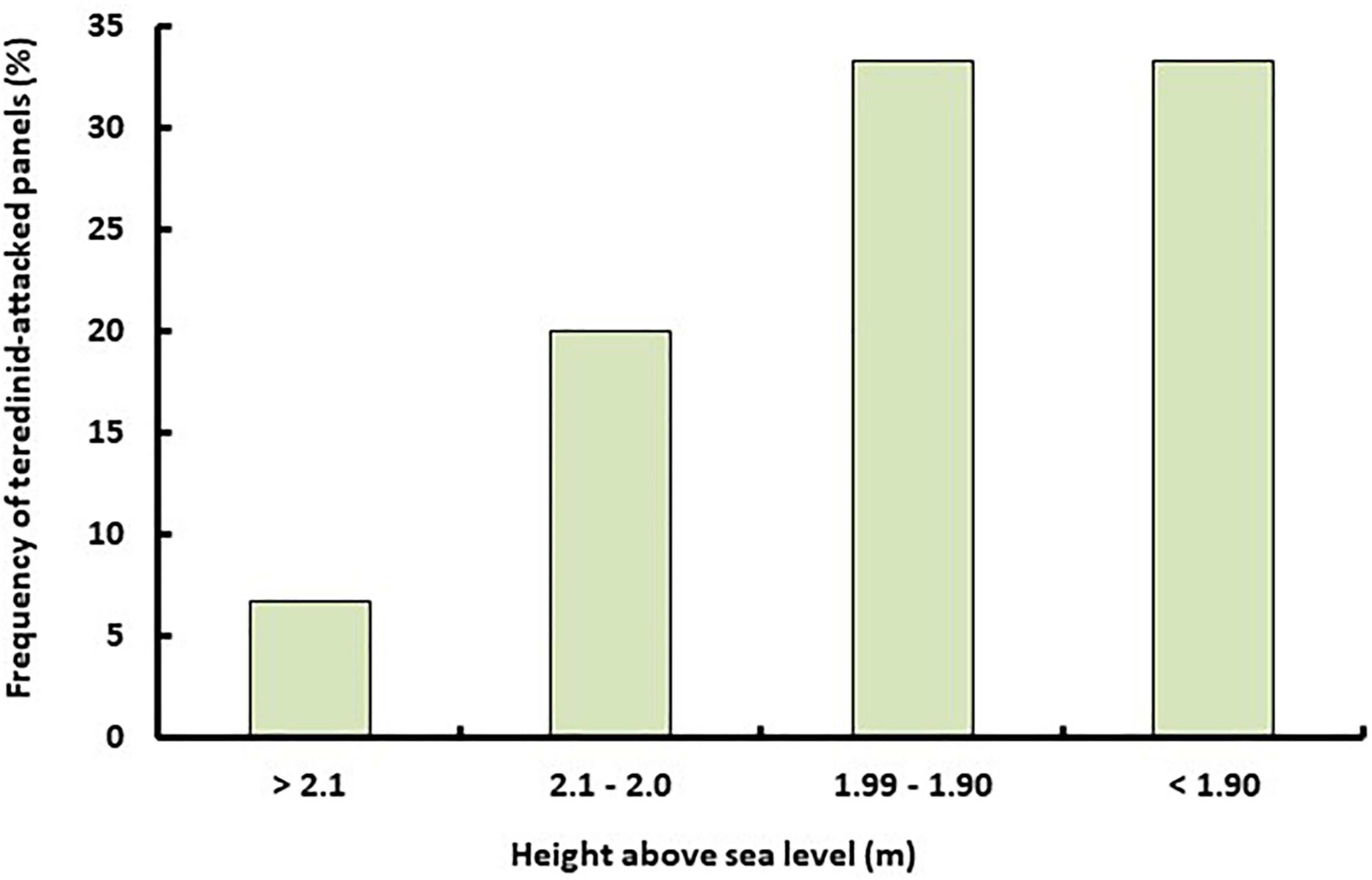
Figure 6. Teredinid biodegrading activity increases as emersion time decreases. The frequency of teredinid-attacked experimental wooden panels (n = 15) placed in areas of different elevations in Galua forest, Kaledupa Island, Indonesia.
Discussion
Spatial Variation in Large Woody Detritus Biodegrading Guilds
This study found significantly different volumes of LWD dependent upon the location within the mangrove forest (Figures 1, 2). Greater quantities of LWD were found in the high intertidal regions of the mangrove forests where the primary biodegradation process was microbial, and therefore slow. Further down the shore to the mid and low intertidal areas, LWD volume decreased due to rapid degradation by guilds of animals and to a lesser extent fungi and then, after fragmentation, removal by ebbing tides.
The Sombano mangrove forest does not extend below the high intertidal and maintains wood-degrading organisms that are found in terrestrial forests, including bracket fungi, beetles, and termites. In the adjacent Langira mangrove forest, the types of wood-degrading organisms were different and the elevation was lower, extending down to mean sea-level. Although both mangrove forests are adjacent to one another, salinity and immersion time differ considerably. These environmental differences facilitated the contrast of wood-degrading guilds between both localities.
In the Sombano mangrove forest and the high intertidal region in the Galua mangrove forest, basidiomycetes were found on almost all LWD. Basidiomycetes provide habitat for many other organisms and enable the regeneration of forests and soil formation (Ananda and Sridhar, 2004; Lonsdale et al., 2008). In these areas of the forests the environmental conditions for basidiomycetes were favorable due to lower salinities and longer shorter inundation periods. In the mid to low intertidal areas were absent and the level of wood decay was decreased. In areas of the mid intertidal and below, higher salinity within the LWD and longer inundation periods may prevent establishment of basidiomycete fungi (Castillo and Demoulin, 1997; Ejechi, 2002), as found in this present study. At the sites examined in this study, there was a critical inundation level below which basidiomycete activity is negligible. Ascomycetes are not limited in the same way, they cause much less rapid decay. This type of decay cannot be readily assessed in the field.
No basidiomycete fruiting bodies were observed in the Laulua mangrove forest. At this locality, in the high to mid intertidal elevations of the mangrove forest the area was dry. Large areas had been harvested, leaving open areas without dense canopy, reducing the humidity. These conditions were not favorable for basidiomycetes (Ananda and Sridhar, 2004). Temperature effects are likely to be patchy and affected by canopy gaps, and stochastic by nature. Many LWD items in this area were broken down by beetle larvae and termites. It is likely that beetle larvae and termites are unable to tolerate long periods of inundation. Beetle larvae and termites are therefore important for the structure and function of mangrove forests in those areas that are not environmentally suitable to basidiomycetes. Beetle larvae are responsible for canopy-gap formation, increased litter-fall due to localized tissue death, and nutrient enrichment due to their mechanical boring within trees (Feller, 2002). Mangrove longhorn beetles, Aeolesthes holosericeus, were found in many standing and fallen Excoecaria agallocha trees in the high intertidal regions of the Sombano forest. Further toward the mid intertidal regions in the Langira, Galua, and Laulua mangrove forests false blister beetle larvae from the family Oedemeridae were abundant in LWD. These larvae are commonly found world-wide in marine driftwood and pilings (Arnett, 1951). Oedermerids can tolerate tidal immersion (Arnett, 1951), which explains their occurrence in LWD in this study.
Also common were the termite genera, Cryptotermes and Microcerotermes, found in LWD in the high intertidal areas of the mangrove forests. Coptotermes, Prorhinotermes, and Nasutitermes were found in arboreal nests in the forest canopy of the Sombano, Galua, and Laulua forests. Arboreal termites live in well-defined nests that are separated from their foraging grounds (Korb, 2008). Tree-dwelling termites are long distance foragers (Roisin et al., 2006) that can travel in relative safety because their runways are covered (Merritt and Starr, 2010). Wood-dwelling mangrove termites, however, adopt a different strategy; they live in LWD within the galleries they create (Adams et al., 2007). Wood-dwelling termites, Cryptotermes were found frequently within decaying wood in the high intertidal regions of the Galua and Laulua forests. Their galleries extended above and below the high tide mark. Cryptotermes colonies remain within a single item of decaying wood where it serves as both food and shelter (Korb and Lenz, 2004). Cryptotermes can detect substrate-borne vibrations (Adams et al., 2007; Evans et al., 2007) that may help these termites avoid potential inundation by retreating from areas within their galleries that will be submerged. The well-developed nests also provide environmental buffering from unfavorable outside temperatures (Korb, 2008). Evaporative cooling takes place within LWD, protecting animals within the wood from increasing temperatures outside (Hendy et al., 2013, 2014). A large proportion of the LWD items in the high intertidal areas of the mangrove forests studied were consumed by termites, with estimates of LWD containing up to 70% by volume termite galleries (IWH personal observations).
Other wood-degrading organisms were found in LWD in the mid to low intertidal areas of the mangrove forests, such as limnoriid isopods and teredinids. Limnoriids and teredinids are predominantly aquatic and these animals are only able to tolerate limited tidal emersion in the mangrove forests. However, limnoriids do not tolerate low salinities, though they were found, albeit infrequently, in the Langira site (Cookson et al., 2012). Limnoriids are more common within Caribbean mangrove forests where salinities remain at suitable levels (Cragg, 2007).
In the mid to low intertidal regions in the Langira, Galua, and Laulua mangrove forests (Figure 5), the breakdown of LWD was dominated by teredinids. Adult teredinids are sessile and disperse by means of planktonic larvae. The availability of LWD is highly variable in mangrove forests (Duke et al., 1981; Kauffman et al., 2020), which influences recruitment of different teredinid species. For example, the teredinid genera dominant in the LWD in this study, Lyrodus and Teredo, both brood larvae, have reduced free-swimming larval periods and hence more limited dispersal potential, whereas the much less abundant broadcast spawning genus Spathoteredo has a greater dispersal potential due to an extended free-swimming larval period. Although Spathoteredo has a broad equatorial distribution (Turner, 1966), Lyrodus and Teredo may have a competitive edge in areas with large volumes and frequent occurrences of LWD. Species with a short free-swimming larval phase tend to be retained in the natal environment (MacIntosh et al., 2012).
Within the low intertidal zones of the forests, LWD was smaller and more fragmented than the LWD within the high intertidal. Teredinid tunneling activity and wood consumption rates increased with decreasing emersion time. Increased teredinid activity along with higher wave energy in the lower intertidal, will increase fragmentation and breakdown of wood.
The rate of deposition and export of particulate and dissolved matter from LWD breakdown will vary with elevation in the mangrove forests. This can be seen in the differences in substrate between the high intertidal regions and the low to mid intertidal regions. The high intertidal regions have less wave energy with greater leaf litter cover and volume of LWD contributing toward a deeper mud substratum. In contrast, the mid and low intertidal regions have calcareous-sand substrate where wave energy and tidal flow is stronger. Charles et al. (2020) found similar results with a 256% increase in the total below-ground carbon biomass in areas of the mangrove higher up the intertidal regions compared to the areas in mid to low intertidal regions. This has also been noted for temperate and Arctic coastal wetlands, where organic matter content has been found to be higher in the higher elevation areas than lower, particularly where accretion rates have historically exceeded sea level rise (Ward et al., 2014; Ward, 2020).
Shifting Biodegradation Boundaries in Relation to Sea Level Rise
Wood breakdown and wood-degradation within mangrove forests may be affected by sea level rise, depending on the geomorphology of the mangrove. In Indo-Pacific, some mangrove forests may be able to keep pace with rising sea levels, however, mangroves with a low tidal range may become submerged (Lovelock et al., 2015). Moreover, Saintilan et al. (2020) report that the rate of global warming is increasing. Accelerating rising sea levels may exceed the rate of landward mangrove accretion within 30 years (Saintilan et al., 2020).
This study undertakes the first baseline survey of LWD biodegradation in a mangrove ecosystem, providing a framework for future predictions of biodegradation boundary changes in relation to climate change and sea level rise. In this study, the transition from terrestrial and marine wood-degrading organisms occurs over a distance of 1–2 m on the forest floor, therefore a rise in sea level would shift the distribution of wood degraders from terrestrial to marine guilds where teredinids would become dominant. Further, increasing access of LWD to teredinids will increase rates of LWD biodegradation, resulting in a faster turnover of carbon within mangrove forests. The shift in activity of wood degrading organisms will have significant consequences for mangrove forests where landward accretion is not possible, e.g., due to coral-rock plateaus, as found in the Sombano and Langira sites from this study, or where topographical or infrastructure barriers occur (Ward and Lacerda, 2021). This alteration in the spatial distribution of carbon in mangroves is likely to be regionally variable as a result of species differences in LWD degraders (Cragg, 2007), as well as linked to variations in the impacts of sea level rise globally and linked to tidal variation (Ward et al., 2016; Lacerda et al., 2022). Additionally, as LWD biodegrading guilds play a key role in the structure and function of mangrove forests and adjacent ecosystems, the altered proportion of wood degrading organisms will result in different LWD biodegradation processes, which will affect the sediment deposition and export of POM and DOM from mangrove forests (Robertson and Daniel, 1989).
Conclusion
Tidal height has a large influence on the rate of wood degradation due to the environmental boundary set by inundation frequency and duration, between marine and terrestrial wood degrading consortia, which have markedly different degradation rates. The high intertidal areas of the forests contained large volumes of soft LWD, slowly broken down by fungi. In the mid to low intertidal areas of the mangrove forests, the volumes of LWD were lower and the wood was less decayed, but was more rapidly broken down due to tunneling by teredinids. This leads to a rapid turnover of LWD in the mid to low intertidal regions, maintaining the important flux of carbon from within intertidal mangrove forests to adjacent ecosystems (Abrantes et al., 2015). Further studies from estuarine sites would enable the effect of salinity to be separated from the effect of inundation regime.
This baseline survey of LWD biodegradation in a tropical mangrove ecosystem, provides a framework for future predictions of biodegradation boundary changes in relation to climate change and sea level rise. The movement of the terrestrial/marine biodegradation boundary due to increasing sea levels will result in a shift in the wood degrading guilds, and in turn affect their carbon processing ability. These changes will impact the function of the mangrove ecosystem and carbon cycles. A full understanding of the factors affecting the biodegradation processes in mangrove forests is critical to accurately assess mangrove carbon stores and the fate of mangrove derived carbon.
Data Availability Statement
The original contributions presented in the study are included in the article/supplementary material, further inquiries can be directed to the corresponding author.
Author Contributions
IH: conceptualization, methodology, formal analysis, investigation, writing – original draft, writing – review and editing, visualization, and project administration. JRS: writing – formal analysis, review and editing, and project administration. MT: formal analysis, review and editing, and project administration. AE and RW: formal analysis, review and editing. SC: conceptualization, methodology, formal analysis, investigation, writing – original draft, writing – review and editing, visualization, and supervision. All authors: contributed to the article and approved the submitted version.
Funding
Operation Wallacea provided research funding for IH. The funder was not involved in the study design, collection, analysis, interpretation of data, the writing of this article or the decision to submit it for publication.
Conflict of Interest
The authors declare that the research was conducted in the absence of any commercial or financial relationships that could be construed as a potential conflict of interest.
Publisher’s Note
All claims expressed in this article are solely those of the authors and do not necessarily represent those of their affiliated organizations, or those of the publisher, the editors and the reviewers. Any product that may be evaluated in this article, or claim that may be made by its manufacturer, is not guaranteed or endorsed by the publisher.
Acknowledgments
We thank D. Smith and P. Mansell for support during field activities. We also thank R. Nembhard and Kungdan for their hard work and help recording volumes of dead wood and Jason Williams for help with creation of GIS maps. All data were collected under the permit: LIPI 04/TKPIPA/FRP/SM/IV/2011.
References
Abrantes, K. G., Johnston, R., Connolly, R. M., and Sheaves, M. (2015). Importance of mangrove carbon for aquatic food webs in wet–dry tropical estuaries. Estuaries Coasts 38, 383–399. doi: 10.1007/s12237-014-9817-2
Adams, E. S., Atkinson, L., and Bulmer, M. S. (2007). Relatedness, recognition errors, and colony fusion in the termite Nasutitermes corniger. Behav. Ecol. Sociobiol. 61, 1195–1201. doi: 10.1007/s00265-007-0349-7
Allen, J. A., Ewel, K. C., Keeland, B. D., Tara, T., and Smith, T. J. III (2000). Downed wood in micronesian mangrove forests. Wetlands 20, 169–176. doi: 10.1672/0277-5212(2000)020[0169:dwimmf]2.0.co;2
Ananda, K., and Sridhar, K. R. (2004). Diversity of filamentous fungi on decomposing leaf and woody litter of mangrove forests in the southeast coast of India. Curr. Sci. India 87, 1431–1437.
Arnett, R. H. (1951). A revision of the neartic oedemeridae. Am. Midl. Nat. 45, 257–391. doi: 10.2307/2421732
Benner, R., and Hodson, R. E. (1985). Microbial degradation of the leachable and lignocellulosic components of leaves and wood from Rhizophora mangle in a tropical mangrove swamp. Mar. Ecol. Prog. Ser. 23, 221–230. doi: 10.3354/meps023221
Breithaupt, J. L., Smoak, J. M., Smith, T. J. III, Sanders, C. J., and Hoare, A. (2012). Organic carbon burial rates in mangrove sediments: strengthening the global budget. Global Biogeochem. Cycles 26, 1–11.
Bunting, P., Rosenqvist, A., Lucas, R. M., Rebelo, L. M., Hilarides, L., and Thomas, N. et al;. (2018). The global mangrove watch-a new 2010 global baseline of mangrove extent. Remote Sens. 10, 1669.
Castillo, G., and Demoulin, V. (1997). NaCl salinity and temperature effects on growth of three wood-rotting basidiomycetes from a Papua New Guinea coastal forest. Mycol. Res. 101, 341–344. doi: 10.1017/s0953756296002717
Charles, S. P., Kominoski, J. S., Armitage, A. R., Guo, H., Weaver, C. A., and Pennings, S. C. (2020). Quantifying how changing mangrove cover affects ecosystem carbon storage in coastal wetlands. Ecology 101:e02916. doi: 10.1002/ecy.2916
Cintron, G., and Schaeffer-Novelli, Y. (1984). “Methods for studying mangrove structure,” in The Mangrove Ecosystem: Research Methods, eds S. C. Snedaker and J. G. Snedaker (Paris: UNESCO), 91–113. doi: 10.1017/cbo9781139173650.003
Cookson, L. J., Cragg, S. M., and Hendy, I. W. (2012). Wood-boring limnoriids (Crustacea, Isopoda) including a new species from mangrove forests of the Tukang Besi Archipelago, Indonesia. Zootaxa 3248, 25–34. doi: 10.11646/zootaxa.3248.1.2
Cragg, S. M. (2007). “Marine wood boring invertebrates of new guinea and is surrounding waters,” in The Ecology of Papua, eds B. M. Behler and A. J. Marshall (Singapore: Periplus), 539–563.
Cragg, S. M., Friess, D. A., Gillis, L. G., Trevathan-Tackett, T. M., Terrett, O. M., Watts, J. E. M., et al. (2020). Vascular plants are globally significant contributors to marine carbon fluxes and sinks. Annu. Rev. Mar. Sci. 12, 469–497. doi: 10.1146/annurev-marine-010318-095333
Cragg, S. M., and Hendy, I. W. (2010). “Mangrove forests of the Wakatobi National Park,” in Marine Research and Conservation in the Coral Triangle, eds J. Clifton, R. K. F. Unsworth, and D. J. Smith (Hauppauge, NY: Nova Science Publishers).
Dangendorf, S., Hay, C., Calafat, F. M., Marcos, M., Piecuch, C. G., Berk, K., et al. (2019). Persistent acceleration in global sea-level rise since the 1960s. Nat. Clim. Change 9, 705–710. doi: 10.1038/s41558-019-0531-8
Donato, D. C., Kauffman, J. B., Murdiyarso, D., Kurnianto, S., Stidham, M., and Kanninen, M. (2011). Mangroves among the most carbon-rich forests in the tropics. Nat. Geosci. 4, 293–297. doi: 10.1038/ngeo1123
Duarte, C. M. (2017). Reviews and syntheses: hidden forests, the role of vegetated coastal habitats in the ocean carbon budget. Biogeosciences 14, 301–310. doi: 10.5194/bg-14-301-2017
Duke, N. C., Bunt, S. J., and Williams, T. W. (1981). Mangrove litter fall in north-eastern Australia. I. Annual totals by component in selected species. Aust. J. Bot. 29, 547–553. doi: 10.1071/bt9810547
Ejechi, B. O. (2002). Microbial deterioration of partially submerged service timbers in a tropical inter-tidal zone. Int. Biodeter. Biodegr. 51, 115–118. doi: 10.1016/s0964-8305(02)00093-8
Evans, T. A., Inta, R., and Lenz, M. (2007). Foraging vibration signals attract foragers and identify food size in the drywood termite, Cryptotermes secundus. Insect. Soc. 54, 374–382. doi: 10.1007/s00040-007-0958-1
Feller, I. C. (2002). The role of herbivory by wood-boring insects in mangrove ecosystems in Belize. Oikos 97, 167–176. doi: 10.1034/j.1600-0706.2002.970202.x
Filho, C. S., Tagliaro, C. H., and Beasley, C. R. (2008). Seasonal abundance of the shipworm Neoteredo reynei (Bivalvia, Teredinidae) in mangrove driftwood from a northern Brazilian beach. Iheringia Ser. Zool. 98, 17–23. doi: 10.1590/s0073-47212008000100002
Hay, C. C., Morrow, E., Kopp, R. E., and Mitrovica, J. X. (2015). Probabilistic reanalysis of twentieth-century sea-level rise. Nature 517, 481–484. doi: 10.1038/nature14093
Hendy, I. W., Eme, J., Dabruzzi, T. F., Nembhard, R. V., Cragg, S. M., and Bennett, W. A. (2013). Dartfish use teredinid tunnels in fallen mangrove wood as a low-tide refuge. MEPS 486, 237–245. doi: 10.3354/meps10370
Hendy, I. W., Michie, M., and Taylor, B. W. (2014). Habitat creation and biodiversity maintenance in mangrove forests: teredinid bivalves as ecosystem engineers. PeerJ 2, e591. doi: 10.7717/peerj.591
Jennerjahn, T. C. (2020). Relevance and magnitude of ‘Blue Carbon’ storage in mangrove sediments: Carbon accumulation rates vs. stocks, sources vs. sinks. Estuar. Coastal Shelf Sci. 247:107027. doi: 10.1016/j.ecss.2020.107027
Karondia, L. A., Handoko, E. Y., and Hapsari, H. (2019). 3D modelling of sea-level rise impact in Semarang, Indonesia. IOP Conf. Ser. Earth Environ. Sci. 389:012005. doi: 10.1088/1755-1315/389/1/012005
Kauffman, J. B., Adame, M. F., Arifanti, V. B., Schile-Beers, L. M., Bernadino, A. F., Bhomia, R. K., et al. (2020). Total ecosystem carbon stocks of mangroves across broad global environmental and physical gradients. Ecol. Monog. 90:e01405.
Kohlmeyer, J., Bebout, B., and Volkmann-Kohlmeyer, B. (1995). Decomposition of mangrove wood by marine fungi and teredinids in Belize. Mar. Ecol. 16, 27–39. doi: 10.1111/j.1439-0485.1995.tb00392.x
Korb, J. (2008). “The ecology of social evolution in termites,” in Ecology of Social Evolution, eds J. Korb and J. Heinze (Heidelberg: Springer Press), 151–174. doi: 10.1007/978-3-540-75957-7_7
Korb, J., and Lenz, M. (2004). Reproductive decision-making in the termite, Cryptotermes secundus (Kalotermitidae), under variable food conditions. Behav. Ecol. 15, 390–395. doi: 10.1093/beheco/arh033
Krauss, K. W., Doyle, T. W., Twilley, R. R., Smith, T. T., Whelan, K. R. T., and Sullivan, J. K. (2005). Woody debris in the mangrove forests of south Florida. Biotropica 37, 9–15. doi: 10.1111/j.1744-7429.2005.03058.x
Lacerda, L., Ward, R. D., Borges, R., and Cesar Ferreira, A. (2022). Mangrove trace-metal biogeochemistry response to global climate change. Front. For. Global Change 5:817992. doi: 10.3389/ffgc.2022.817992
Lonsdale, D., Pautasso, M., and Holdenrieder, O. (2008). Wood-decaying fungi in the forest – conservation needs and management options. Eur. J. For. Res. 127, 1–22. doi: 10.1007/s10342-007-0182-6
Lovelock, C. E., Cahoon, D. R., Friess, D. A., Guntenspergen, G. R., Krauss, K. W., Reef, R., et al. (2015). The vulnerability of Indo-Pacific mangrove forests to sea-level rise. Nature 526, 559–563. doi: 10.1038/nature15538
MacIntosh, H., de Nys, R., and Whalan, S. (2012). Shipworms as a model for competition and coexistence in specialized habitats. Mar. Ecol. Prog. Ser. 461, 95–105. doi: 10.3354/meps09823
McArdle, B. H., and Anderson, M. J. (2001). Fitting multivariate models to community data: a comment on distance-based redundancy analysis. Ecology 82, 290–297. doi: 10.1890/0012-9658(2001)082[0290:fmmtcd]2.0.co;2
Merritt, N. R. C., and Starr, C. K. (2010). Comparative nesting habits and colony composition of three arboreal termites (Isoptera: Termitidae) in Trinidad and Tobago, West Indies. Sociobiology 56, 611–622.
Middleton, A. B., and McKee, L. K. (2001). Degradation of mangrove tissues and implications for peat formation in Belizean island forests. J. Ecol. 89, 818–828. doi: 10.1046/j.0022-0477.2001.00602.x
Nerem, R. S., Beckley, B. D., Fasullo, J. T., Hamlington, B. D., Masters, D., and Mitchum, G. T. (2018). Climate-change–driven accelerated sea-level rise detected in the altimeter era. Proc. Natl. Acad. U.S.A. 115, 2022–2025. doi: 10.1073/pnas.1717312115
Ong, J. E., Gong, W. K., and Wong, C. H. (2004). Allometry and partitioning of the mangrove Rhizophora apiculate. For. Ecol. Manag. 188, 395–408. doi: 10.1016/j.foreco.2003.08.002
Rayner, S. M. (1983). Distribution of teredinids (Mollusca: Teredinidae) in Papua New Guinea. Rec. Aust. Mus. 35, 61–67. doi: 10.3853/j.0067-1975.35.1983.302
Robertson, A. I. (1990). Plant-animal interactions and the structure and function of mangrove forest ecosystems. Aust. J. Ecol. 16, 433–443. doi: 10.1111/j.1442-9993.1991.tb01073.x
Robertson, A. I., and Daniel, P. A. (1989). Decomposition and the annual flux of detritus from fallen timber in tropical mangrove forests. Limnol. Oceanogr. 34, 640–646. doi: 10.4319/lo.1989.34.3.0640
Roisin, Y., Dejean, A., Corbara, B., Orivel, J., Samaniego, M., and Leponce, M. (2006). Vertical stratification of the termite assemblage in a neotropical rainforest. Oecologia 149, 301–311. doi: 10.1007/s00442-006-0449-5
Rovai, A. S., Coelho-Jr, C., de Almeida, R., Cunha-Lignon, M., Menghini, R. P., Twilley, R. R., et al. (2021). Ecosystem-level carbon stocks and sequestration rates in mangroves in the Cananéia-Iguape lagoon estuarine system, southeastern Brazil. For. Ecol. Manag. 479:118553. doi: 10.1016/j.foreco.2020.118553
Saintilan, N., Khan, N. S., Ashe, E., Kelleway, J. J., Rogers, K., Woodroffe, C. D., et al. (2020). Thresholds of mangrove survival under rapid sea level rise. Science 368, 1118–1121. doi: 10.1126/science.aba2656
Sanderman, J., Hengl, T., Fiske, G., Solvik, K., Adame, M. F., Benson, L., et al. (2018). A global map of mangrove forest soil carbon at 30 m spatial resolution. Environ. Res. Lett. 13:055002. doi: 10.1088/1748-9326/aabe1c
Singh, A. P., Kim, Y. S., and Chavan, R. R. (2022). Advances in understanding microbial deterioration of buried and waterlogged archaeological woods: a review. Forests 13:394. doi: 10.3390/f13030394
Surya, M. Y., Zhiguo, H., Xia, Y., and Li, L. (2019). Impacts of sea level rise and river discharge on the hydrodynamics characteristics of Jakarta Bay (Indonesia). Water 11, 1384–1398. doi: 10.3390/w11071384
Thongtham, N., Kristensen, E., and Puangprasan, S. Y. (2008). Leaf removal by sesarmid crabs in Bangrong mangrove forest, Phuket, Thailand; with emphasis on the feeding ecology of Neoepisesarma versicolor. Estuar. Coastal Shelf Sci. 80, 573–580. doi: 10.1016/j.ecss.2008.09.017
Turner, R. D. (1966). A Survey and Illustrated Catalogue of the Teredinidae (Mollusca: Bivalvia). Cambridge, MA: Harvard, The Museum of Comparative Zoology, 265.
Turner, R. D. (1971). Marine Borers, Fungi, and Fouling Organisms of Wood. Paris: Organisation for Economic Co-operation and Development.
Vane, C. H., Kim, A. W., Moss-Hayes, V., Snape, C. E., Diaz, M. C., Khan, N. S., et al. (2013). Degradation of mangrove tissues by arboreal termites (Nasutitermes acajutlae) and their role in the mangrove C cycle (Puerto Rico): chemical characterization and organic matter provenance using bulk δ13C, C/N, alkaline CuO oxidation-GC/MS, and solid-state 13CNMR. Geochem. Geophys. Geosyst. 14, 3176–3191. doi: 10.1002/ggge.20194
Ward, R. (2020). Carbon sequestration and storage in norwegian arctic coastal wetlands: impacts of climate change. Sci. Total Environ. 748:141343. doi: 10.1016/j.scitotenv.2020.141343
Ward, R., Burnside, N., Joyce, C., Sepp, K., and Teasdale, P. A. (2014). Recent rates of sedimentation on irregularly flooded Boreal Baltic coastal wetlands: responses to recent changes in sea level. Geomorphology 217, 61–72. doi: 10.1016/j.geomorph.2014.03.045
Ward, R., Friess, D., Day, R., and Mackenzie, R. (2016). Impacts of climate change on global mangrove ecosystems: a regional comparison. Ecosyst. Health Sustain. 2, 1–25.
Ward, R., and Lacerda, L. D. (2021). “Responses of mangrove ecosystems to sea level change,” in Dynamic Sedimentary Environment of Mangrove Coasts, eds D. Friess and F. Sidik (Amsterdam: Elsevier).
Keywords: coastal wetlands, biodegradation, blue carbon, wood decay, fungi, shipworms, carbon cycling, sea level rise (SLR)
Citation: Hendy IW, Shipway JR, Tupper M, Etxabe AG, Ward RD and Cragg SM (2022) Biodegraders of Large Woody Debris Across a Tidal Gradient in an Indonesian Mangrove Ecosystem. Front. For. Glob. Change 5:852217. doi: 10.3389/ffgc.2022.852217
Received: 10 January 2022; Accepted: 02 May 2022;
Published: 25 May 2022.
Edited by:
Daniel Friess, National University of Singapore, SingaporeReviewed by:
Raghab Ray, The University of Tokyo, JapanBeth A. MIddleton, United States Geological Survey (USGS), United States
Copyright © 2022 Hendy, Shipway, Tupper, Etxabe, Ward and Cragg. This is an open-access article distributed under the terms of the Creative Commons Attribution License (CC BY). The use, distribution or reproduction in other forums is permitted, provided the original author(s) and the copyright owner(s) are credited and that the original publication in this journal is cited, in accordance with accepted academic practice. No use, distribution or reproduction is permitted which does not comply with these terms.
*Correspondence: Ian W. Hendy, aWFuLmhlbmR5QHBvcnQuYWMudWs=