- 1Flora Conservation Department, Kadoorie Farm and Botanic Garden, Tai Po, Hong Kong SAR, China
- 2School of Biological Sciences, The University of Hong Kong, Pokfulam, Hong Kong SAR, China
- 3Missouri Botanical Garden, St. Louis, MO, United States
Anthropogenic disturbance has led to widespread clearance and degradation of tropical forests, and tree planting has been promoted as an effective solution for recovery. However, trees have been overwhelmingly planted in monocultures or low-diversity mixes and this is expected to have profound, lasting impacts on forest structure, diversity, and functioning. In this study, we tested the extent to which historical vegetation transition types (VTTs) constrain forest recovery in a secondary tropical landscape in Hong Kong, South China. To do so, we overlaid vegetation types (forest, shrubland, pine plantation, grassland) identified in aerial photographs taken in 1956 and 1963 of a 20-ha plot situated in Tai Po Kau Nature Reserve, allowing us to define six historic VTTs, namely: FF (forest to forest), GP (grassland to plantation), GS (grassland to shrubland), SS (shrubland to shrubland), SF (shrubland to forest), and SP (shrubland to plantation). We compared present-day forest structure and species diversity among these VTTs, as determined from a census conducted in 2015, using incidence- and abundance-based rarefaction and extrapolation, and we assessed species’ association within VTTs using a torus translation test. Our results reveal that stem density and species diversity in naturally regenerated forests were more similar to those of old-growth forest, whereas species diversity in areas occupied by pine plantations was significantly lower as compared with naturally regenerated areas. Despite 60 years of recovery, pine plantations were characterised by a significantly greater proportion of negatively associated species, and late-seral species were still predominantly confined to old-growth patches. Present-day species distribution is chiefly explained by the combined effects of topography and VTT (17.1%), with VTT alone explaining 4.4%. Our study demonstrates that VTT has a significant long-term impact on forest regeneration and community assembly and, importantly, that monocultural plantations (forest plantation) can greatly impede forest recovery. Remnant old-growth forest patches merit priority protection, and active restoration, including thinning and enhancement planting, is necessary to facilitate forest succession.
1. Introduction
The occurrence of tree species within forest communities is defined by an interplay of environmental variables and historic disturbance (Hermy and Verheyen, 2007). Key environmental variables that contribute to the establishment and survival of a tree within its habitat include topographic factors, light availability, moisture, humidity, and soil properties (Harms et al., 2001; Comita et al., 2007; Zuleta et al., 2020). A habitat-association relationship of this sort has been confirmed by a number of studies conducted in forest dynamic plots in the tropics (Gunatilleke et al., 2006; Lai et al., 2009; Bin et al., 2016; Guo et al., 2016). However, as most forest dynamic plots are established in primary forest, these studies overwhelmingly focus on quantifying the relative importance of various environmental factors and tend to disregard the role of anthropogenic disturbances (Uriarte et al., 2004; Thompson et al., 2007). Indeed, forest plots in primary forest do not generally account for a human disturbance dimension and may therefore not be suitable for testing the relative contribution of historic disturbance regimes in limiting species distributions (Davies et al., 2021).
However, more than half of all tropical moist forests globally are at various stages of secondary regrowth following human-induced impact and conversion (Wright, 2005; Brancalion et al., 2019). Such disturbance may not only lead to the loss of canopy cover and biodiversity, but also fundamentally alter environmental conditions and constrain the forest’s capacity to regenerate (Chazdon, 2003). Depending on the degree of degradation and specific land-use history, secondary forests (Brown and Lugo, 1990; Corlett, 1994; Dent and Wright, 2009) undergo contrasting successional pathways (Norden et al., 2011; Arroyo-Rodríguez et al., 2017). The particular pathway taken and the rate of succession will both be closely related to the initial composition during succession (Egler, 1954) and the same factors that govern the distribution of species in communities, that is, environmental variables and land-use history (Arroyo-Rodríguez et al., 2017). Both factors determine the remaining species pool (Karger et al., 2015), which comprises the species present in situ and/or those able to disperse out of remnant forest fragments nearby, as well as those represented in the soil seed bank (de Medeiros-Sarmento et al., 2021). Because the scale and intensity of disturbance dictates which species survive and remain locally able to reproduce, disperse and recruit, it also characterises the species pool available for recolonisation and succession (Brown and Boutin, 2009; Chase, 2003; Rozendaal et al., 2019).
Species pool is often greatly reduced after a clear-cut event and may only be able to partially recover (Xu et al., 2015). However, subsequent land-use type is also an important determining factor. In mountainous parts of South China, for example, where landscape-level forest clearance for agriculture dates back thousands of years, species survive in small, isolated, remnant forest fragments, or in secondary associations, forming small pockets of native biodiversity (Turner and Corlett, 1996). These pockets often conserve a significant proportion of the original species pool, some of which may be able to recolonise adjacent areas and thus contribute to the regeneration of continuous canopy cover given enough time (Turner and Corlett, 1996).
Whilst the remnant species pool determines which species are available to recolonise, the precise species composition of newly regenerated secondary communities at a given location is primarily defined by priority effect (Fukami, 2015; Fukami et al., 2016). This is an aggregate ecological mechanism that allows those species that remain present in the soil seed bank, or which arrive at a disturbed site sooner, to attain dominance at an early stage of succession, thus enabling them to replace themselves repeatedly from seed where no competing late-seral species are present to initiate species turn-over (Ashton et al., 2001; Paul et al., 2004; Goldsmith et al., 2011). This can lead to arrested succession (Goldsmith et al., 2011; Young and Peffer, 2010). Priority effects have a significant impact not only on the dynamics of forest ecosystems, but also on the properties of the active species pool (Fukami, 2015). As the recovery of tree species diversity and community composition is often a long-term process (Osazuwa-Peters et al., 2015), historic disturbance and subsequent priority effect can constrain succession for decades or centuries to come. It is thus hypothesised that the signature of different disturbance regimes can be detected in forest communities even over exceptionally long timespans (Johnstone et al., 2016).
Planting trees is considered an effective approach to overcome priority effects (Weidlich et al., 2021) and thereby facilitate natural succession of degraded tropical lands (Lugo, 1992; Lamb et al., 2005; Crouzeilles et al., 2017; Meli et al., 2017). It has generally been argued that biodiversity and ecosystem functioning are better restored via tree planting (i.e., active restoration) than by natural succession (i.e., passive restoration) alone (Crouzeilles et al., 2017; Atkinson and Bonser, 2020). However, whether this is true or not in all cases remains contentious (Reid et al., 2019). Monocultures and plantation forest comprising exceptionally low diversity (Food and Agriculture Organization of the United Nations, 2018) may hamper natural succession as the species pool will be limited and regeneration capacity thereby constrained through priority effects (Maestre and Cortina, 2004; Holl et al., 2013; Liu et al., 2018). Indeed, whether or not active restoration is more effective than natural regeneration will depend on the degree of disturbance and the remaining species pool, as well as the selection of species planted and method of restoration (Chazdon, 2003; Reid et al., 2019). An inappropriate restoration method–for example, the use of a limited species mix or of species not suited to the modified environment–often results in lower diversity and delayed succession (Reid et al., 2019). Exotic tree plantations often further exacerbate the degradation of ecological functioning, via accelerated soil erosion, the promotion of pest outbreaks, and suppression of recovery in other dependent taxa, such as birds (Maestre and Cortina, 2004). In this sense, tree planting could actually delay or stall recovery. Indeed, analyses in Hong Kong revealed that, at the landscape level, secondary succession of tropical forest can be much faster in naturally regenerated areas as compared to areas planted with monocultural stands of exotic Eucalyptus, Pinus, and Acacia species (Abbas et al., 2019).
In the present study, we sought to assess the impact of different disturbance regimes caused by historic land-use changes on the current distribution of tree species in South China’s secondary forest communities. To do so, we established a 20-ha forest dynamic plot following ForestGEO standards (Anderson-Teixeira et al., 2015; Davies et al., 2021) in Hong Kong. All trees inside the plot with a DBH (Diameter at Breast Height) ≥ 1 cm were mapped and identified, and their DBH was measured. To determine changes in forest cover over time and characterise specific vegetation transition types, we mapped historic vegetation cover as inferred from a time series of historic black and white aerial photographs. We hypothesise that the spatial distribution of species recovery will reflect historic disturbance regimes and that areas with the highest species diversity today will therefore be associated with small pockets of remnant old-growth forest. We test the correlation between present forest structure [standing tree stem density and above-ground biomass (AGB)], diversity, species composition, and species-vegetation associations with different historic disturbance regimes to address the following questions: (i) do species richness, diversity and the presence/absence of indicator species differ among present-day vegetation types characterised by different disturbance regimes, and (ii), what are the relative influences of disturbance regime and environmental factors on present-day species composition? We discuss our findings in the context of the regenerative potential of degraded tropical lands and the potential for active restoration to overcome inherent barriers to secondary succession.
2. Materials and methods
2.1. Study site
Hong Kong is located on the coast of South China and has a marginally tropical climate with an annual average rainfall of 2,325 mm and an annual mean temperature of 23°C (Abbas, 2017). The zonal vegetation is usually considered evergreen broad-leaved forest (Zhuang and Corlett, 1997). However, due to widespread anthropogenic activities over the past several centuries, no primary forest remains in the territory (Zhuang and Corlett, 1997; Corlett, 1999). Our study site is located in the Tai Po Kau Nature (henceforth, TPK) Reserve, which is situated in Hong Kong’s New Territories with an area of 460 ha and a steep, hilly topography. Despite the prevalence of plantations containing a mixture of native (Castanopsis fissa, Cinnamomum camphora, and Pinus massoniana) and exotic (Acacia confusa, Eucalyptus tereticornis, and Lophostemon confertus) species planted between 1956 and 1963, TPK’s numerous remote, narrow gullies have protected small fragments of old-growth forest that pre-date WWII. As such, the reserve is regarded as one of the most biologically important secondary lowland woodlands in the territory (Nicholson, 1996).
A 400 × 500 m 20-ha plot was established inside TPK (22.42°N, 114.17°E) in 2012 (Figure 1A). The elevation of the plot ranges from 214 to 344 m, and the incline of the slope varies from 0.9 to 33.6° (Figure 1B). A full tree census (Condit, 1998; Anderson-Teixeira et al., 2015) was undertaken in the plot in 2015. A total of 81,019 individual trees (117,203 stems, including branches) with DBH ≥ 1 cm were recorded, belonging to 172 species, 111 genera and 53 families. The most abundant species was the shrub Psychotria asiatica with 26,646 individuals, which accounted for 33% of all individuals (Supplementary Table 1). Data pertaining to dead trees (1,876 stems or branches) were removed, including one species that was represented by a single individual, leaving 171 species for further analysis.
2.2. Classification of historic vegetation types and change in vegetation transition type over time
We obtained three black-and-white aerial photographs of TPK from the Lands Department of the Hong Kong SAR Government, one from 1956 (Supplementary Figure 1A) representing the initial degraded state, one from 1963 (Supplementary Figure 1B) representing the early regeneration state, and one from 1983 (Supplementary Figure 1C) representing the closed canopy state. As the entire area was forested by 1983, the following assignment of vegetation to transition types was conducted for 1956 and 1963 only. The aerial photographs for these 2 years were firstly geo-referenced, co-registrated and resampled to the same 1 m resolution for comparison. Then, we used the “segment mean shift” algorithms (Comaniciu and Meer, 1999) in ArcGIS 10.7 (ESRI, 2019, Redlands, California, USA) to delineate vegetation parcels with similar texture and grey tone. Segmented vegetation parcels were then divided into three groups based on the K-means clustering of averaged grey tone values within each parcel (Yadav and Sharma, 2013). Finally, manual refinements were made by considering the spatial continuity of each vegetation type.
Four vegetation types were identified in the aerial photographs: grassland, shrubland, pine plantation, and mixed natural forest. Whereas in 1956 the site comprised grassland, shrubland, and mixed natural forest (Supplementary Figure 1D), by 1963 the grassland had been substituted by pine plantation and shrubland (Supplementary Figure 1E). We overlaid the classified vegetation types in both images to derive six vegetation transition types (VTTs; Figure 2), namely, forest to forest (FF), grassland to pine plantation (GP), grassland to shrubland (GS), shrubland to shrubland (SS), shrubland to forest (SF), and shrubland to pine plantation (SP). Each VTT was regarded as reflecting a distinct vegetation change history. Because the FF VTT represented the occurrence of the oldest canopy cover in the plot, this vegetation was taken as reference old-growth forest. Whereas the GS, SS, and SF VTTs represented natural transitions were thus considered passive restoration, the GP VTT entailed management intervention was thus regarded as active restoration. The resulting vegetation transition map was converted to 20 m resolution (500 20 × 20 m subplots, Figure 2) based on the dominant rule (in which each grid cell was classified according to the VTT that accounted for the largest area within it) for further analyses. Since the area of SP (including six 20 × 20 m subplots, Figure 2) was <1 ha, this VTT was excluded from subsequent analysis.
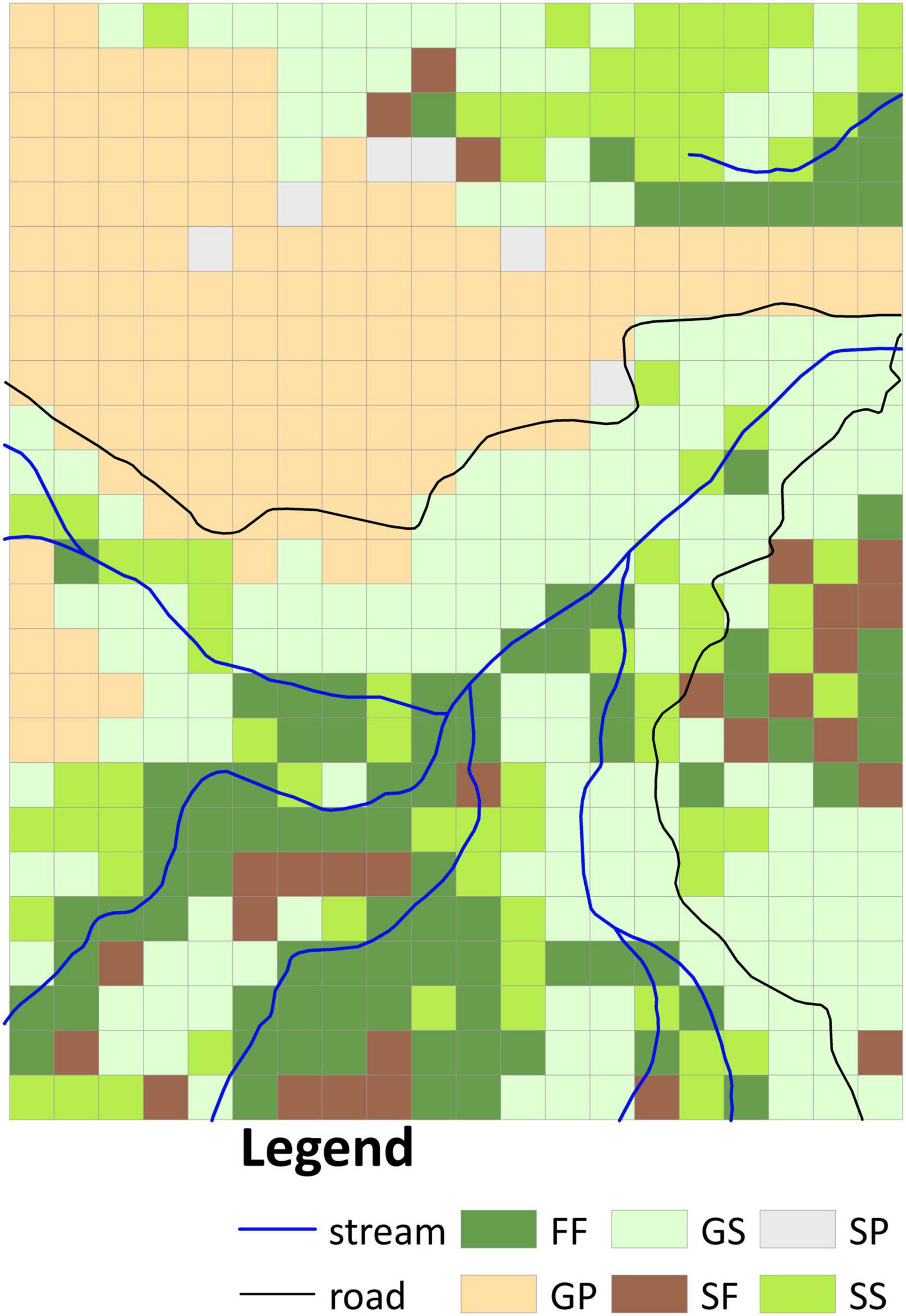
Figure 2. Classification of vegetation transition types (VTTs) from 1956 to 1963 at 20 m resolution. FF, forest to forest; GP, grassland to plantation; GS, grassland to shrubland; SS, shrubland to shrubland; SF, shrubland to forest; SP, shrubland to plantation.
2.3. Data analysis
2.3.1. Comparison of stem density and above-ground biomass among VTTs
We used stem density and AGB to compare present-day forest structure, as determined by the 2015 census, between VTTs. We calculated AGB in kg based on DBH for all the species occurring in each VTT following Chave et al. (2005), using the following equation:
Where ρ is wood density (g/cm3) and D is DBH (cm).
Wood densities were mainly compiled from studies conducted in Dinghushan (Zhang et al., 2018) and Heishiding (Yin et al., 2021), which represent the two closest ForestGEO plots to Hong Kong. Where data for certain species were not available from these sources, we obtained them from the R package BIOMASS (Réjou-Méchain et al., 2017). Where no wood density data were available, a mean value was derived from all other congeners, or from all species belonging to the same family, present in the plot.
We tested for significant differences in stem density and AGB between VTTs using resampling, which can reduce the effect of differences due to environmental factors and variable sample size (Chao and Jost, 2012; Chao et al., 2014). Twenty-five 20 × 20 m quadrats (total 1 ha) were randomly sampled for each VTT, and indices for both metrics were calculated in each quadrat. This procedure was repeated 1,000 times with replacement. 95% confidence intervals (CIs) were generated from the 2.5 and 97.5% quantiles, corresponding to significance at the 0.05 level (Goldsmith et al., 2006).
2.3.2. Comparison of diversity using rarefaction and extrapolation with Hill’s numbers
We used Hill’s numbers curves to determine the effect of VTT on biodiversity (Jost, 2007; Chao and Jost, 2012; Chao et al., 2014). The first three Hill’s numbers are: (1) species richness (q = 0), (2) the exponential of Shannon’s entropy index (q = 1), and (3) the inverse of Simpson’s concentration index (q = 2) (Hill, 1973). These values were calculated using both incidence-based and abundance-based data for each VTT using the “iNEXT” package in R (Hsieh et al., 2016). The equation for abundance-based Hill’s numbers is:
And the equation for incidence-based Hill’s numbers is:
Where S is the number of species; pi is the relative abundance of the ith species; and πi is the relative incidence of the ith species. Incidence was calculated based on occurrence in the 20 × 20 m quadrats.
2.3.3. Species turnover in different VTTs
In comparing Hill’s numbers among different vegetation types, we also tested if species turnover differs among VTTs (Wilson and Shmida, 1984; Koleff et al., 2003). Species turnover was visualised using an RGB colour map derived from non-metric multidimensional scaling (NMDS) (Thessler et al., 2005). To do so, we computed Bray–Curtis dissimilarities (Bray and Curtis, 1957) between all 20 × 20 m quadrats and constrained the results to three axes using the function “metaMDS” in the “vegan” package (Oksanen et al., 2022). The scores of the first three axes were converted to hexadecimal colour values for red, blue, and green, respectively. As the value of RGB colours must be positive integers, we added a constant (1.0) to the NMDS ordination axis scores to make them positive, multiplied them by 100 and then rounded the product. The red, green, and blue layers of each quadrat were overlaid and visualised to create an RGB colour map in ArcGIS (Thessler et al., 2005). Using this map, we were able to compare species composition chromatically (Thessler et al., 2005; Hogan et al., 2016), with greater similarity in colour being indicative of greater similarity in the range of species present.
2.3.4. Quantifying the relative importance of VTT and topography
We recorded elevation, slope incline, curvature and aspect in each 20 × 20 m quadrat. These variables have been widely used to define topography in other forest dynamic plots (Harms et al., 2001; Lai et al., 2009; Guo et al., 2017; Davies et al., 2021). All variables were standardised through z-score standardisation (see R code in Supplementary material). We then used variation partitioning to quantify the relative importance of VTT and topography in explaining species turnover (Legendre, 2008; Legendre and Legendre, 2012; Peres-Neto et al., 2006). This was carried out using the function “varpart” (Borcard et al., 1992; Legendre and Legendre, 2012) in vegan (Oksanen et al., 2022).
2.3.5. Species–VTT association using torus translation test
We used a torus translation test to evaluate the strength of association between species and VTT (Harms et al., 2001; Zuleta et al., 2020). This approach allowed us to compare the observed relative densities of a species with the expected densities generated from simulated habitat maps for each vegetation type (Harms et al., 2001; Comita et al., 2007; Lai et al., 2009). We shifted the observed vegetation map by 20 m increments in the four cardinal directions and calculated a relative density for each species. We then ran the torus translation procedure for three simulated maps based on the original map: mirror image, 180° rotation and 180° rotation of the mirror image. As the TPK plot has 500 (20 × 25) 20 m cells, we generated 1999 (500 × 4 − 1) simulated maps. If the relative density of a species determined from the original vegetation map deviated from 97.5% of the simulated relative densities (i.e., 0.05 significance for a two-tailed test), the species was judged to be statistically associated (either positively or negatively) with that VTT (Harms et al., 2001). Sixty-three species represented by <20 individuals were excluded for the purpose of this analysis, leaving 108 species (of a total of 171). Analyses were conducted with fgeo.habitat (Lepore, 2018) in R (see R code in Supplementary material).
3. Results
3.1. Change in vegetation type over time
The study area was found to have gradually transformed from a grassland-dominated landscape to a forest-dominated one as a result of natural succession and active restoration over the study period (Figure 3). Whereas 54.4% (108,770 m2) of the plot was grassland in 1956, with scattered patches of mixed natural forest occurring only in ravines along streams and accounting for just 15.9% of the total area (Figure 3), forests occupied 26.1% of the plot in 1963 and 100% in 1983. This transformation was mainly achieved through expansion of remnant natural forest from the streams. However, nearly half (GP: 50,814 m2) of the area occupied by grassland in 1956 had been replaced by pine plantation in 1963, with the remaining half (GS: 57,956 m2) becoming shrubland (Figure 4). More than half of the shrubland present in 1956 was still shrubland in 1963 (SS: 34,783 m2). Only a very small fraction (SP: 4,317 m2) of shrubland had been replaced by pine plantation in 1963.
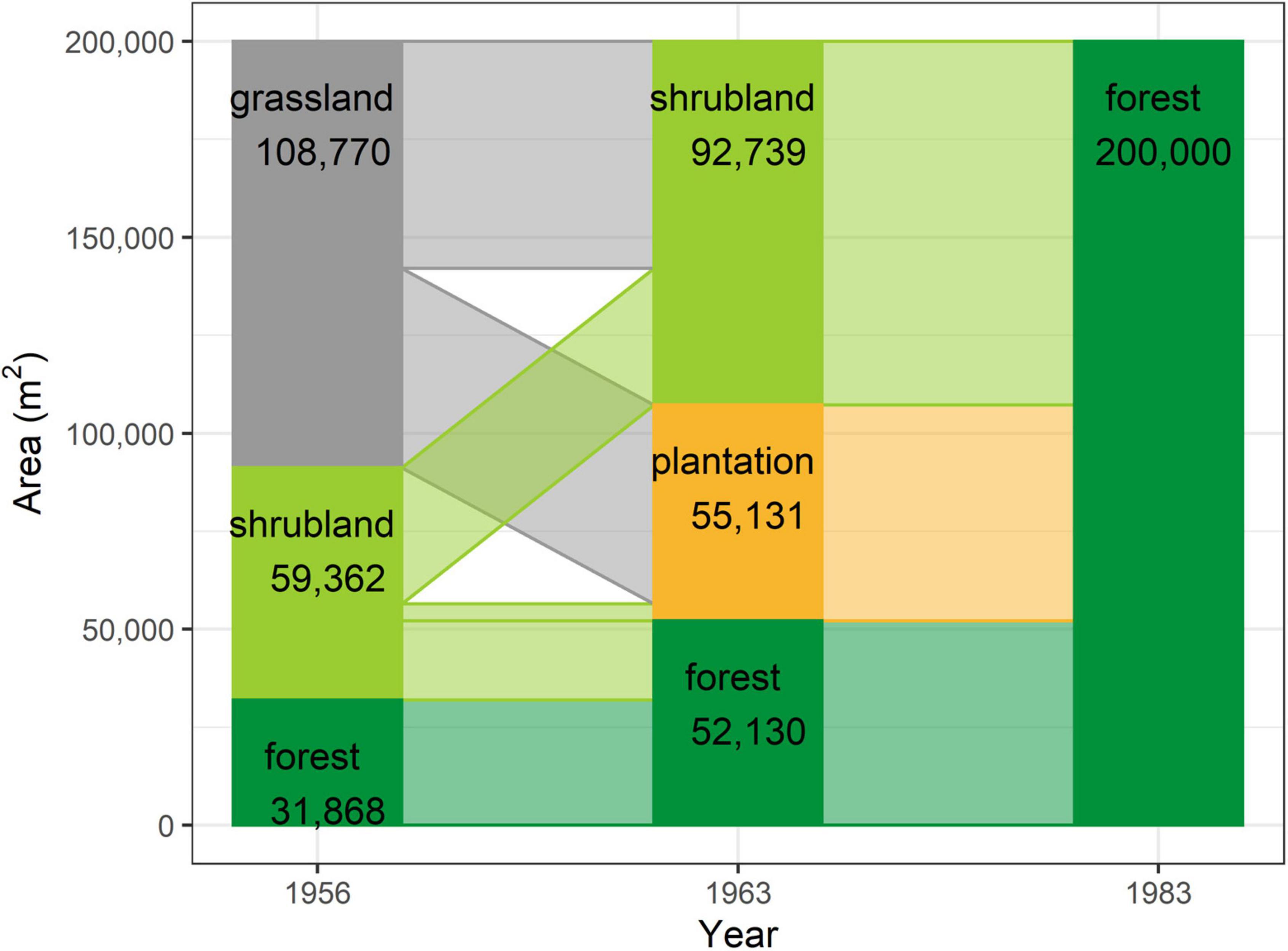
Figure 3. Schematic representation of the area accounted for by each vegetation type within the TPK plot at each time point.
3.2. Stem density and above-ground biomass among VTTs
Stem density was highest in SF and lowest in FF, and whilst there was no significant difference among GS, SS, and FF, it was significantly higher in GP than in SS and FF (Figure 5A). A similar pattern was observed in AGB, although the differences among VTTs were smaller, with that associated with GP being almost the same as for GS (Figure 5B). Differences in stem density were mainly caused by smaller trees (DBH 1–5 cm), while differences in AGB were mainly contributed by the trees with intermediate or large diameters (DBH ≥ 10 cm) (Supplementary Figure 2).
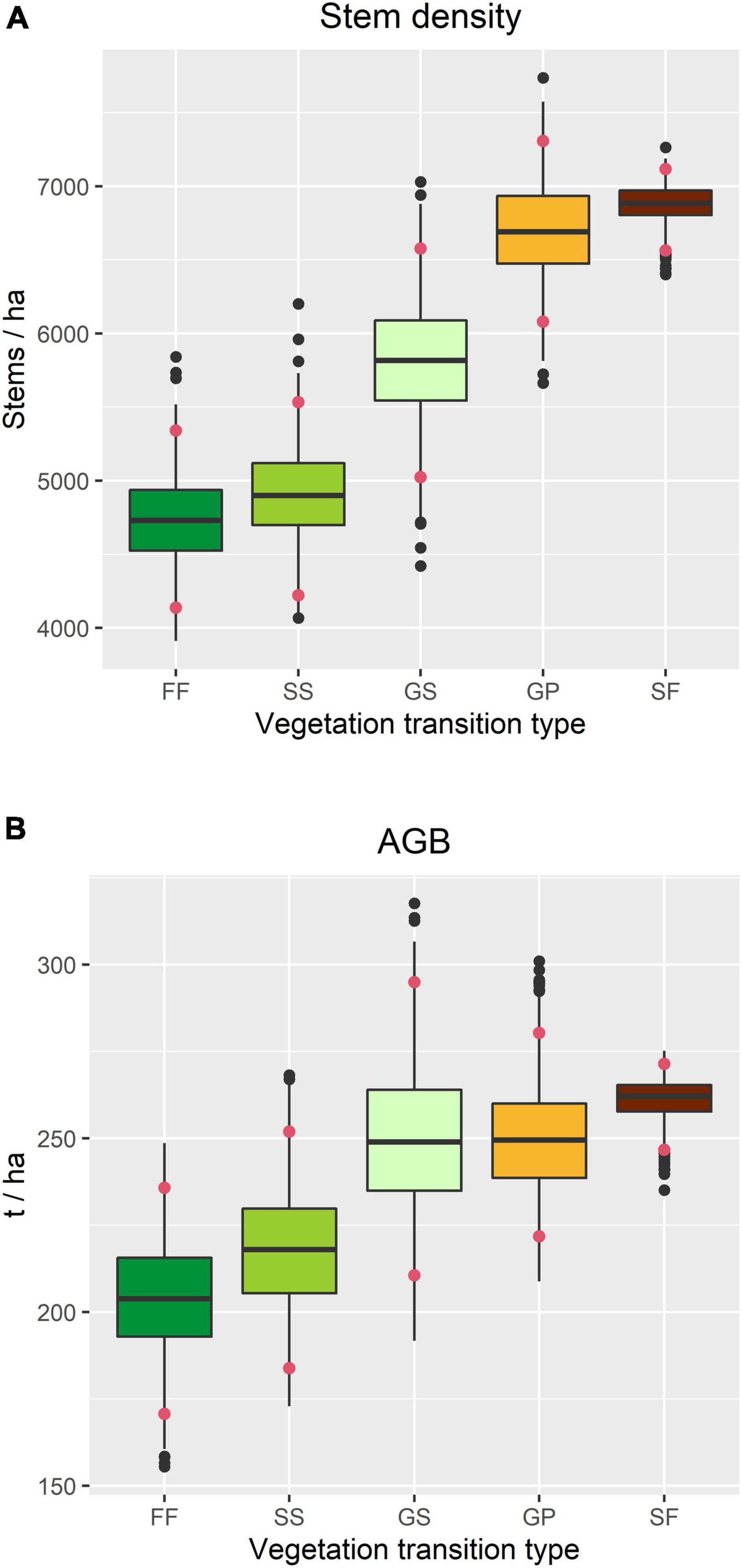
Figure 5. Structural attributes of the forest within the TPK plot in 2015, based on census data associated with each vegetation transition type. (A) Overall stem density. (B) Overall above-ground woody biomass (AGB). Confidence intervals (red dots) indicate the 2.5 and 97.5% quantiles.
3.3. Comparing Hill’s numbers among VTTs
Numbers of species and individuals associated with different VTTs are shown in Table 1. Psychotria asiatica was the dominant species in all vegetation types (Supplementary Table 2). Whereas FF was ascribed the highest values for all Hill’s numbers, diversity curves were the lowest for GP (Figures 6A–F). GS was significantly higher and closer to FF than it was to GP. For the abundance-based curves, Hill’s numbers for richness (q = 0) declined in the order FF > SS > GS > SF > GP. However, 95% CIs overlapped for GS, SS, and FF, indicating no significant difference. Hill’s numbers for the exponential of Shannon’s entropy index (q = 1) and for the inverse of Simpson’s concentration index (q = 2) declined in the order FF > SS > SF > GS > GP. In both cases, most curves were widely separated from one another, apart from GS and SF for q = 1 (Figures 6B,C). In the incidence-based curves, whereas the 95% CIs for GS, SS, and SF overlapped with one another and were close to those for FF, those for GP were much lower and clearly separated (Figures 6D–F). Coverage of sample size approximated to one for both abundance (Figure 6G) and incidence data (Figure 6H).

Table 1. Number of species and individuals in each vegetation transition type (see main text for definition of each acronym).
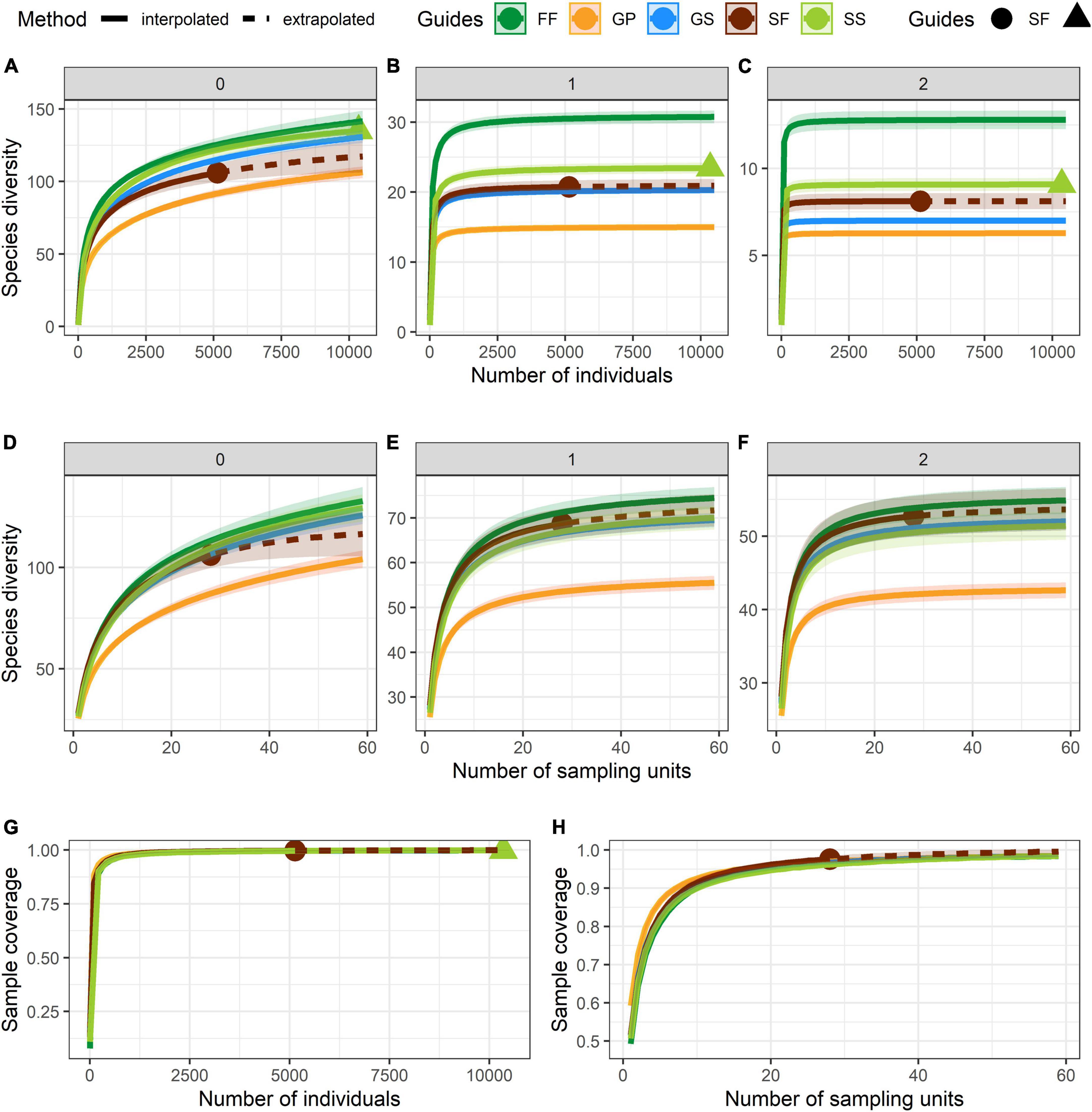
Figure 6. Curves of Hill’s numbers for each vegetation transition types (VTTs). (A–C) Abundance-based Hill’s numbers. (D–F) Incidence-based Hill’s numbers. In panels (A) and (D): q = 0 (species richness); in panels (B) and (E): q = 1 (exponential of Shannon’s entropy index); in panels (C) and (F): q = 2 (inverse of Simpson’s concentration index). (G) Sample completeness (sample-coverage) curve for abundance data. (H) Sample completeness (sample-coverage) curve for incidence data. 95% confidence intervals (CIs) were indicated by the shade areas above and below each curve and were obtained by bootstrapping 1,000 replicates. The sampling unit is 400 m2 (20 × 20 m quadrat) in the incidence-based analyses.
3.4. Species turnover in different VTTs
Species composition was closely correlated with topography and varied across VTTs (Figure 7). Meanwhile, compositional differences were captured along the elevational gradient in all VTTs except for the area of pine plantation represented by GP (Figure 7).
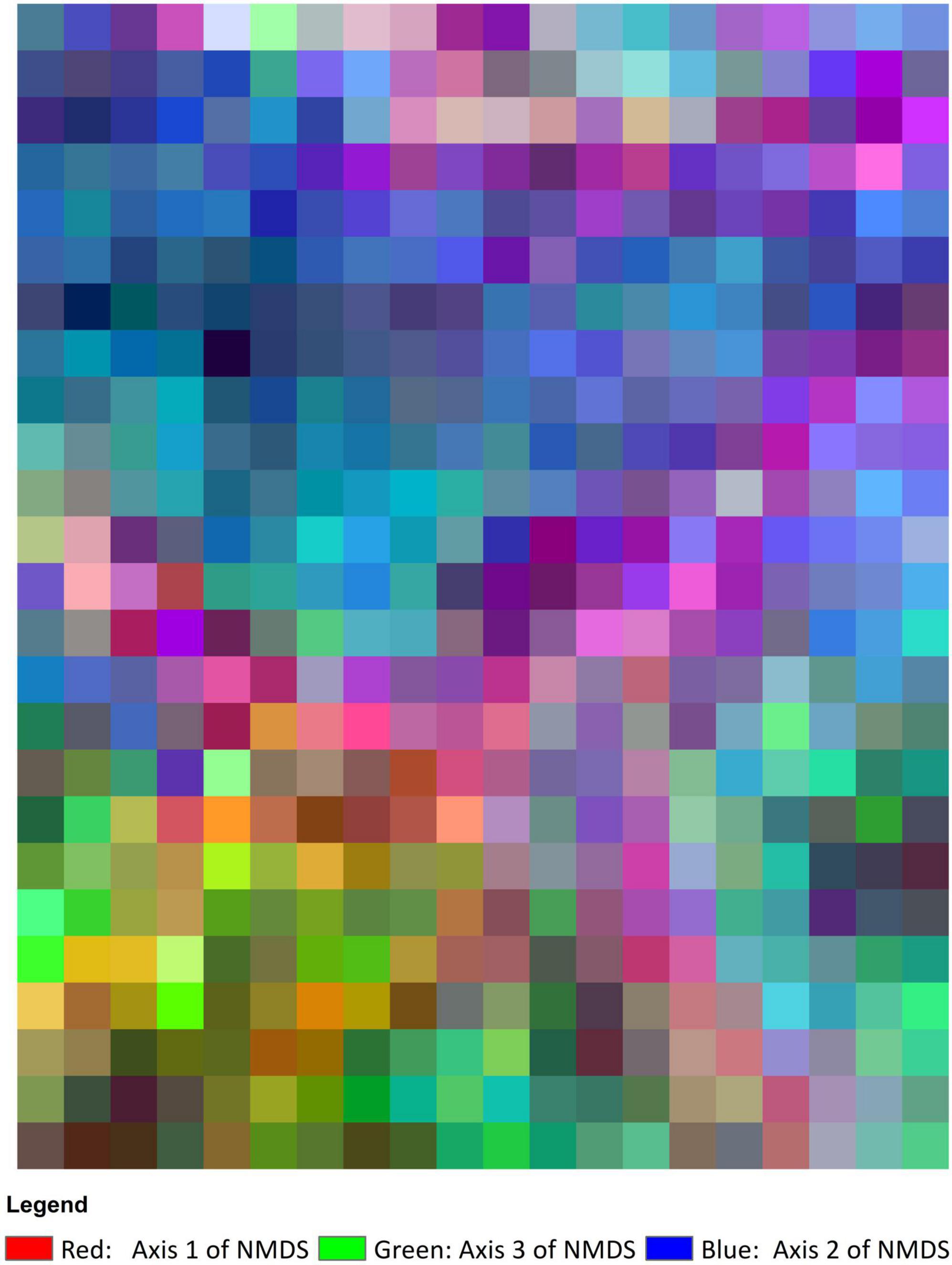
Figure 7. Species composition map, showing the colours derived from the three axes of the NMDS ordination analysis. The more similar the colour, the more similar the species composition. Cell size is 20 × 20 m.
3.5. The relative importance of VTT and topography
Variation partitioning showed that VTT and topography accounted for 17.1% of total variation in species composition. Whereas VTT alone explained 4.4% of total variance and topography alone explained 9.7%, both factors together explained 2.9% of variance (Figure 8).
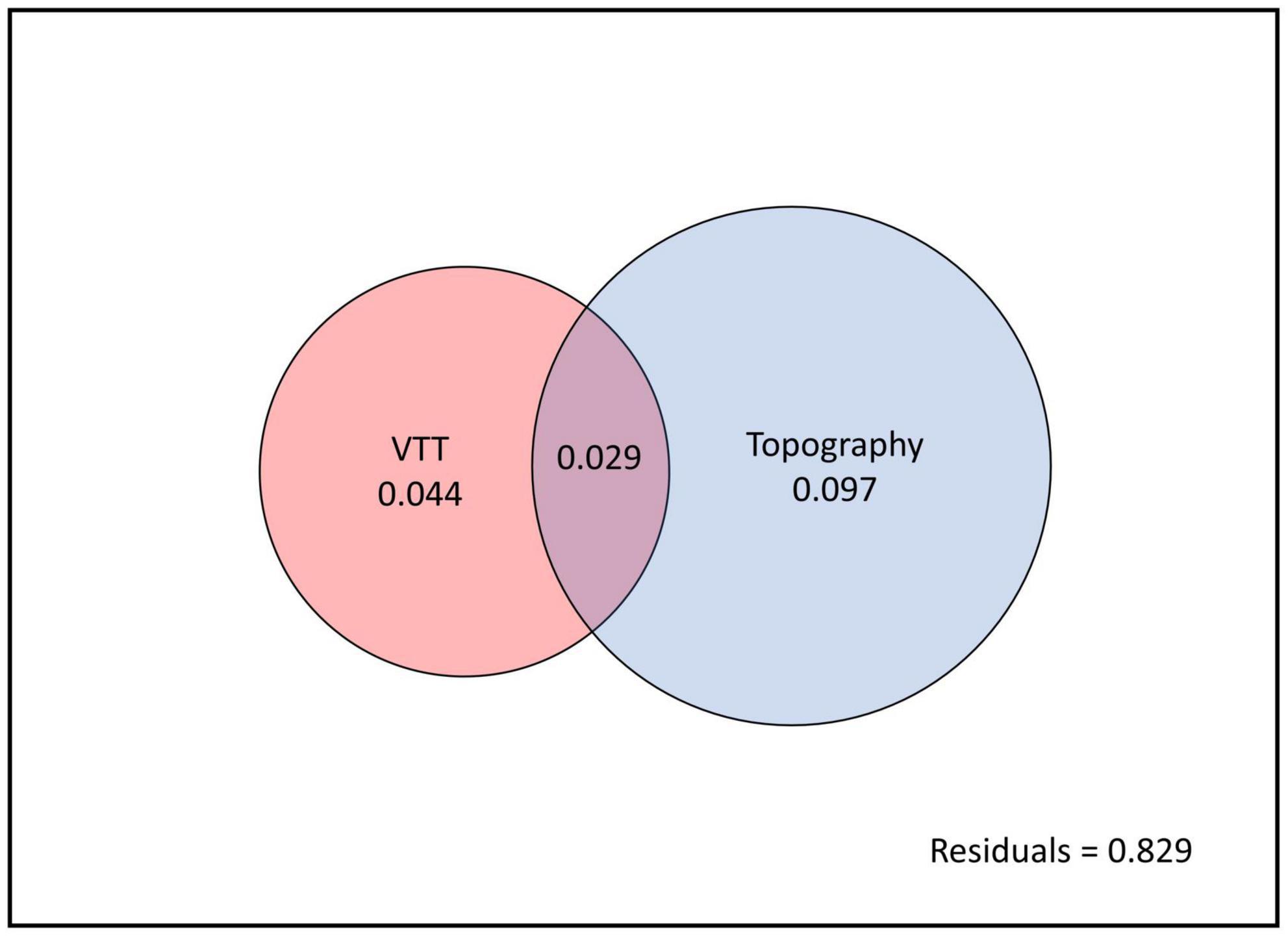
Figure 8. Variation partitioning for vegetation transition types (VTTs) and topography. The entire panel (black outline) indicates total variation partitioning in species composition between explained (coloured circles) and unexplained (white background, the residual) components. Non-overlapping parts of the two circles represent the variation accounted for each factor alone and overlapping parts represent the variation accounted for by both factors together.
3.6. Species–VTT association using torus translation test
Of the 108 species tested in the torus translation test, 70 (64.8%) were significantly associated with at least one VTT (Supplementary Table 2). Twenty-seven species (25.0%) were negatively associated with GP (Table 2), which was the highest number of species among all VTTs, and 17 species (15.7%) were negatively associated with SF. Equally, different VTTs were significantly associated with certain species. FF had the highest number of positively associated species (31) (Table 3), which was significantly greater than that for all other VTTs, whereas FF, GS, and SS had relatively few negatively associated species (Table 2).
4. Discussion
In this study, we explored the impact of historical vegetation type changes, as characterised by a series of empirically defined VTTs, on stem density, AGB, species diversity, and species composition in a secondary lowland forest in South China. We quantified the relative contribution of VTT and topographic variables on the fine-scale distribution of tree species. We report that, at the landscape scale, the distribution of trees today is still heavily constrained by historical VTTs, even after 60 years of combined passive and active recovery. Our findings clearly demonstrate that, although stem density and AGB may recover in a few decades, species diversity and composition do not recover that rapidly.
4.1. Generalists dominate the entire plot
Species colonising during early succession are usually regarded as generalists or “winner” species (McKinney and Lockwood, 1999; Zhao et al., 2015) owing to their ability to tolerate, and attain dominance under harsh conditions. This appears to be the case at our study site. The common understorey shrub Psychotria asiatica alone accounted for 33% of all stems, and the fast-growing trees Aporosa dioica, Diospyros morrisiana, and Machilus chekiangensis were among the most abundant species throughout the entire plot (Supplementary Table 1). These species exhibit several ecological traits characteristic of generalists, including fruits that are attractive to common wildlife and which are consequently widely dispersed, and high drought-tolerance during the early growth phase, allowing them to compete with grasses and so colonise grassland (Tabarelli et al., 2012; Zhao et al., 2015). The seed source of these winner species may have been nearby patches of old-growth forest or shrubland during the initial degraded phase, or they could have dispersed into the plot from further afield.
Classical models of forest succession predict that once the canopy has closed, mid- or late-seral species arrive and establish, thereby facilitating community enrichment (Ashton et al., 2001; Poorter et al., 2021). Our results show that this may be unlikely to happen rapidly, given that late-seral species are often rare and overwhelmingly restricted to small patches of old-growth forest (FF), and that seed dispersers are locally extinct, hence limiting opportunities for recovery.
4.2. Forest structure and diversity of different VTTs
Whilst initial vegetation type was found to have a significant impact on present-day abundance-based diversity indices, impact in terms of stem density, AGB and incidence-based diversity indices, which relate to spatial distribution, was limited. In terms of forest age, the different VVTs identified in the TPK plot decline in the order FF > (SF, SS) > (GP, GS). In old-growth forest, individual tree girth is usually greater and stem density is lower as compared with younger secondary forest (but see Augusto et al., 2000). Indeed, our results confirm that, at TPK, younger forest (i.e., SF, SS, GP, and GS) tends to have a higher stem density than does older forest (FF). This difference appears to be primarily caused by the presence of very large numbers of small saplings and shrubs (1 cm < DBH ≤ 5 cm) in shrubland and young forest.
Higher stem densities in these younger forest communities could indicate that competition among individuals is intense (Picard, 2019) and that natural stem-thinning is therefore likely to ensue (Field et al., 2020; Wu et al., 2020). However, stem density peaks in SF, despite this representing a comparatively advanced state of secondary succession in the sequence observed at TPK, suggesting that self-thinning has failed along this successional pathway. We note that an over-dominance of generalist shrubs (e.g., Psychotria asiatica) and faster growing, early seral trees (e.g., Machilus checkiangensis) in the SF VTT is linked to lower species diversity, higher stem density, and crucially, an absence of late-seral species, as compared with old-growth FF. This appears to limit tree girth increment and prevent species turnover, two features consistent with arrested succession (Kennard, 2002; Whitfeld et al., 2014). The markedly different species composition observed between the SF and FF VTTs reinforces this inference and suggests that the successional pathway taken by post-disturbance secondary vegetation at TPK does not lead to reinstatement of authentic late-seral communities.
Poorter et al. (2016) found that AGB can recover by up to 90% of old-growth values after more than 60 years of succession, and that the impact of land-use history on present-day forest composition and structure may be limited in the neotropics. In contrast, our findings demonstrate that land-use history as reflected by historical VTTs can have a significant impact on the recovery of AGB, even after six decades of post-disturbance recovery, which is more in line with predictions made by Jakovac et al. (2021). Previous studies have shown that AGB is mainly accounted for by large-diameter stems (Stephenson et al., 2014; Lutz et al., 2018) and that large trees are expected to occur more in old-growth forest than in younger associations (Brown et al., 1997). Our results show that in tropical Asia, where old-growth forest has been extensively converted into agricultural lands, this may not be entirely correct. We find that comparatively young secondary communities, represented by GS, GP, and SF in our study, have higher AGB than FF, the oldest forest community in our analysis. This appears to be because the number of small stems is significantly greater in the younger communities and the number of larger trees is low in FF. Indeed, tree size in South China’s remnant old-growth patches are limited owing to several factors. Firstly, although the remnant patches may contain late-seral species, they too have usually been subject to intensive disturbance histories, including fire (Chau and Corlett, 1994), firewood collection (Corlett, 1999), and selective logging (Zhuang and Corlett, 1997) over a period of centuries. Secondly, because these patches are generally restricted to steep ravines, tree size may have been limited by rocky conditions and a lack of top soil due to seasonal flooding, which can lead to stunted tree growth (Lopez and Kursar, 2003). Thirdly, once the canopy of immediately adjacent secondary forest has been able to close through regeneration, the environment along the streams could be too dark and therefore less suitable for remaining late-seral species to recruit and spread (Lebrija-Trejos et al., 2011).
In comparison, GS, GP, and SF appear to have higher AGB because winner, generalist species (McKinney and Lockwood, 1999; Tabarelli et al., 2012; Filgueiras et al., 2021) can successfully establish and grow to a larger size or attain a higher stem density on nearby slopes (Zhao et al., 2015). Indeed, significant increases in AGB are mainly generated by the presence of larger stems (DBH > 20 cm) throughout all VTTs, but because high stem density is associated with higher AGB, it does not necessarily reflect a recovery in species composition (Rozendaal et al., 2019). The exceptionally high number of stems in the young secondary forest communities studied here is primarily made up of generalist and winner species, indicative of arrested succession (Goldsmith et al., 2011).
4.3. Factors affecting species accumulation and seedling establishment
In analysing forest succession, it is common to substitute space for time to represent a chronosequence (Abbas et al., 2016, 2019; Poorter et al., 2021). In the present study, however, we used historic aerial photographs to identify past vegetation types and reconstruct successional pathways, allowing us to estimate the impact of historic land use more accurately. Although species composition was not documented in each subplot at the time the photographs were taken, we were able to retrospectively determine vegetation type using a statistical approach. By overlaying vegetation type distributions as inferred from the resulting time sequence, the specific disturbance history of each subplot could be inferred. This approach reduces ambiguity and highlights subtle but important differences between diverging successional pathways (Norden et al., 2011; Arroyo-Rodríguez et al., 2017; Jakovac et al., 2021).
Vegetation transition types alone was found to explain 4.4% of variation in present-day species distribution, as compared to 9.7% contributed by topography. A further 2.9% of variation was explained by both factors combined. It is not surprising that the effects of VTT are confounded with those caused by topography, given that both factors tend to act synergistically in constraining ecological tolerance (Guo et al., 2017; Schmitt et al., 2021). For example, it is conceivable that in ravines that are too steep or too rocky for conversion to agriculture, pre-existing forest cover was deliberately retained to maintain water supply (Luke et al., 2017).
Previous studies have shown that, although species richness can recover within a few decades in the tropics (Abbas et al., 2019), species composition may only partially recover (Xu et al., 2015). Similarly, our data reveal that, whereas species richness in the various VTTs was able to recover within six decades, species composition has not converged with that observed in old-growth patches (FF) over the same period, indicating that passive forest succession in these VTTs is slow. Potential underlying reasons for this include dispersal limitation (Palma et al., 2021), reduced species pool (Zobel et al., 1998), priority effects (Fukami, 2015; Fukami et al., 2016), competition (Connell and Slatyer, 1977; Chen et al., 2019), and environmental filtering (Jakovac et al., 2016, 2021), all of which are known to affect both tree seedlings and saplings.
4.4. Why are late-seral species restricted to small old-growth patches?
Multiple studies have found that differences in species composition can be explained by dispersal limitation (Condit et al., 2002; Shen et al., 2009; Palma et al., 2021), environmental gradients (Graco-Roza et al., 2022), and stochasticity (Asefa et al., 2020). However, the term “dispersal limitation” may be too general, encompassing numerous inter-related factors. In fact, there may be several inter-dependent reasons for the failure of establishment of late-successional species (Uhl, 1987), such as those which were found to be restricted to FF in the present study.
Firstly, the number of seeds that allow these species to establish in neighbouring areas may be far below the minimum threshold required for successful expansion (Palma et al., 2020) due, for example, to high rates of seed predation (Chung and Corlett, 2006) or because mother trees are confined to less than optimal conditions and are thus suppressed by over-dominant surrounding trees (Ding and Zang, 2021).
Secondly, a species’ ability to germinate and persist will be affected by seed quality, which is itself influenced by genetic factors (Leimu et al., 2010). Trees occurring in old-growth patches within secondary forest are often isolated from other conspecific populations, and hence subject to reduced gene flow (Zeng et al., 2012). Consequently, these species typically exhibit the effects of inbreeding (Leimu et al., 2010; Young et al., 1996) and so exhibit reduced seed viability and depressed seed-set (Kuussaari et al., 2009; Dullinger et al., 2012). Since this is often indicative of extinction debt (Kuussaari et al., 2009), conservation attention is important.
Thirdly, even if the species were able to produce a sufficient number of viable seeds, an absence of suitable dispersal agents may prevent them from recolonising surrounding vegetation types (Corlett, 2017; Silva et al., 2020). Brancalion et al. (2018) have shown that late-seral species tend to have larger fruits or seeds that are dispersed by animals or birds. In our study, a number of species within the FF VTT have larger fruits or seeds that are attractive to animals, for example, Lithocarpus elizabethae, Sloanea sinensis, Artocarpus styracifolius, and Elaeocarpus nitentifolius (Corlett, 2011). Successful dispersal of these species into adjacent areas is dependent on a range of dispersal agents, including hornbills, large squirrels, gibbons and other birds and mammals which are no longer present in Hong Kong (Corlett, 2011). One solution for overcoming this ecological barrier and associated extinction debt would be to reintroduce these locally extinct animal species back into Hong Kong’s secondary forests (Svenning and Faurby, 2017; Carver et al., 2021). However, it is questionable whether today’s secondary vegetation could support the survival of these large dispersal agents: Hong Kong’s secondary forests are mostly too homogenised to provide year-round food supply (van Schaik et al., 1993; Lichstein et al., 2004; Keith et al., 2009; Corlett, 2011).
4.5. Low-diversity forest plantations can impede natural recovery
Pine trees have been widely used for afforestation in Hong Kong (Corlett, 1999). However, the pine trees previously planted in TPK (P. massoniana) were mostly eradicated by wilt disease during the 1970s, resulting in reversion to grassland and shrubland (Zhuang, 1997). The impact of this can be seen in our results: species diversity was significantly greater in GS than in GP, and it approximated more closely to that found in FF, indicating that natural regeneration was more effective than low diversity active restoration in terms of overall ecological recovery. Indeed, species composition in areas previously under pine plantation differed markedly from that in other VTTs, even after decades of recovery and natural succession. The number of negatively associated species within the pine plantation area was much greater than that in other VTTs, particularly with respect to species indicative of old-growth primary forest, such as Turpinia arguta, Mallotus hookerianus, and Ficus pyriformis. Previous research suggests that Pinus species impose an intense priority effect on natural regeneration (Tomimura et al., 2012) through niche pre-emption and modification (Fukami, 2015). In addition, monocultural plantations are known to negatively affect soil properties, including cation exchange capacity and availability of phosphorous and potassium (Caravaca et al., 2002; Maestre and Cortina, 2004), plus they can adversely affect the composition and diversity of the seedling layer (Comita et al., 2010). It thus seems important to recognise that any short- to medium-term benefits associated with monocultural plantations in terms of soil stabilisation and reduced fire risk (Corlett, 1999) could be counteracted by these kinds of long-term detrimental impacts, which can in turn lead to arrested succession (Goldsmith et al., 2011). Indeed, planting low diversity species mixes can be risky (Holl et al., 2022), strongly influencing the successional pathway and available species pool in a way that can mean complete canopy coverage is not attained.
4.6. Management and conservation implications
Although biotic homogenisation is often prevalent in secondary forests (McKinney and Lockwood, 1999; Holl et al., 2022), secondary forests themselves are not necessarily homogeneous. In particular, patches of old-growth forest that harbour a diverse set of late-seral species may be scattered within a secondary forest matrix in a non-random manner (Liu et al., 2019; Liu and Slik, 2014). Such patches are likely to retain higher species diversity and thus be of greater conservation value (Cardoso et al., 2022), and our results underscore the importance of ensuring that they serve as reservoirs of regenerative potential by identifying and removing key barriers to spread.
Indeed, we reveal that the effects of historical VTTs can persist for 60 years or longer, demonstrating that past disturbance regimes should be taken into account in studies of community assembly and forest succession in degraded tropical landscapes. Our data indicate that many species are unable to colonise monocultural plantations, reinforcing their artificially low diversity, and secondarily making them more vulnerable to pests and diseases, thereby retarding succession (Siemann and Rogers, 2006; Liu et al., 2018; Wu et al., 2021). Conversely, multispecies forest plantations are likely to offer preferable outcomes in terms of ecosystem services (Feng et al., 2022).
Conservation intervention is clearly necessary to secure the long-term persistence of rare, late-seral species, and overcome the bottlenecks discussed here, by, for instance, selectively thinning the understorey to improve fine-scale conditions that support dispersal, establishment and turnover (Deng et al., 2020; Ding and Zang, 2021). Enhancement planting should also be considered to increase overall biodiversity after thinning (Corlett, 2011; Abbas et al., 2020), and such interventions may not only be critical for trees and shrubs, but also herbaceous elements, too.
Data availability statement
Publicly available datasets were analyzed in this study. This data can be found here: https://forestgeo.si.edu/sites/asia/hong-kong.
Author contributions
HZ: data curation, formal analysis, investigation, methodology, visualization, writing original draft, and review and editing. JZ: conceptualization, data curation, methodology, software, validation, writing original draft, and review and editing. MLC: formal analysis, methodology, visualization, and review and editing. BCHH: investigation, methodology, project administration, and review and editing. GAF: conceptualization, investigation, methodology, project administration, and review and editing. SWG: conceptualization, investigation, project administration, and review and editing. All authors contributed to the article and approved the submitted version.
Acknowledgments
We acknowledge the constructive comments received from mentors present at the 2019 ForestGEO Annual Analytical Workshop funded by the Smithsonian Institution, especially Stuart J. Davies and Sabrina E. Russo for their suggestions on data analysis. We thank Chengjin Chu’s lab for providing wood density data for several species. We also thank Prof. Anne Chao for providing comments and suggestions on rarefaction and extrapolation with Hill’s numbers. We greatly thank the people who conducted the field survey and data entry for the Tai Po Kau Plot. The survey would not have been possible without financial support from HSBC.
Conflict of interest
The authors declare that the research was conducted in the absence of any commercial or financial relationships that could be construed as a potential conflict of interest.
Publisher’s note
All claims expressed in this article are solely those of the authors and do not necessarily represent those of their affiliated organizations, or those of the publisher, the editors and the reviewers. Any product that may be evaluated in this article, or claim that may be made by its manufacturer, is not guaranteed or endorsed by the publisher.
Supplementary material
The Supplementary Material for this article can be found online at: https://www.frontiersin.org/articles/10.3389/ffgc.2023.1098666/full#supplementary-material
References
Abbas, S. (2017). Remote sensing of forest succession in Hong Kong’s country parks. Available online at: https://theses.lib.polyu.edu.hk/handle/200/8886 (accessed July 13, 2022).
Abbas, S., Nichol, J. E., and Fischer, G. A. (2016). A 70-year perspective on tropical forest regeneration. Sci. Total Environ. 544, 544–552. doi: 10.1016/j.scitotenv.2015.11.171
Abbas, S., Nichol, J. E., Fischer, G. A., Wong, M. S., and Irteza, S. M. (2020). Impact assessment of a super-typhoon on Hong Kong’s secondary vegetation and recommendations for restoration of resilience in the forest succession. Agric. For. Meteorol. 280:107784. doi: 10.1016/j.agrformet.2019.107784
Abbas, S., Nichol, J. E., Zhang, J., and Fischer, G. A. (2019). The accumulation of species and recovery of species composition along a 70 year succession in a tropical secondary forest. Ecol. Indic. 106:105524. doi: 10.1016/j.ecolind.2019.105524
Anderson-Teixeira, K. J., Davies, S. J., Bennett, A. C., Gonzalez-Akre, E. B., Muller-Landau, H. C., Joseph Wright, S., et al. (2015). CTFS-ForestGEO: A worldwide network monitoring forests in an era of global change. Glob. Change Biol. 21, 528–549. doi: 10.1111/gcb.12712
Arroyo-Rodríguez, V., Melo, F. P. L., Martínez-Ramos, M., Bongers, F., Chazdon, R. L., Meave, J. A., et al. (2017). Multiple successional pathways in human-modified tropical landscapes: New insights from forest succession, forest fragmentation and landscape ecology research: Multiple successional pathways. Biol. Rev. 92, 326–340. doi: 10.1111/brv.12231
Asefa, M., Wen, H., Brown, C., Cao, M., Xu, K., and Hu, Y. (2020). Ecological drivers of tree assemblage in tropical, subtropical and subalpine forests. J. Veg. Sci. 31, 107–117. doi: 10.1111/jvs.12819
Ashton, M. S., Gunatilleke, C. V. S., Singhakumara, B. M. P., and Gunatilleke, I. A. U. N. (2001). Restoration pathways for rain forest in southwest Sri Lanka: A review of concepts and models. For. Ecol. Manag. 154, 409–430. doi: 10.1016/S0378-1127(01)00512-6
Atkinson, J., and Bonser, S. P. (2020). “Active” and “passive” ecological restoration strategies in meta-analysis. Restor. Ecol. 28, 1032–1035. doi: 10.1111/rec.13229
Augusto, A., Tabanez, J., and Viana, V. M. (2000). Patch structure within Brazilian Atlantic forest fragments and implications for conservation. Biotropica 32, 925–933. doi: 10.1111/j.1744-7429.2000.tb00630.x
Bin, Y., Spence, J., Wu, L., Li, B., Hao, Z., Ye, W., et al. (2016). Species-habitat associations and demographic rates of forest trees. Ecography 39, 9–16. doi: 10.1111/ecog.00787
Borcard, D., Legendre, P., and Drapeau, P. (1992). Partialling out the spatial component of ecological variation. Ecology 73, 1045–1055. doi: 10.2307/1940179
Brancalion, P. H. S., Bello, C., Chazdon, R. L., Galetti, M., Jordano, P., Lima, R. A. F., et al. (2018). Maximizing biodiversity conservation and carbon stocking in restored tropical forests. Conserv. Lett. 11:e12454. doi: 10.1111/conl.12454
Brancalion, P. H. S., Niamir, A., Broadbent, E., Crouzeilles, R., Barros, F. S. M., Almeyda Zambrano, A. M., et al. (2019). Global restoration opportunities in tropical rainforest landscapes. Sci. Adv. 5:eaav3223. doi: 10.1126/sciadv.aav3223
Bray, J. R., and Curtis, J. T. (1957). An ordination of the upland forest communities of Southern Wisconsin. Ecol. Monogr. 27, 325–349. doi: 10.2307/1942268
Brown, C. D., and Boutin, C. (2009). Linking past land use, recent disturbance, and dispersal mechanism to forest composition. Biol. Conserv. 142, 1647–1656. doi: 10.1016/j.biocon.2009.02.035
Brown, S., and Lugo, A. E. (1990). Tropical secondary forests. J. Trop. Ecol. 6, 1–32. doi: 10.1017/S0266467400003989
Brown, S., Schroeder, P., and Birdsey, R. (1997). Aboveground biomass distribution of US eastern hardwood forests and the use of large trees as an indicator of forest development. For. Ecol. Manag. 96, 37–47. doi: 10.1016/S0378-1127(97)00044-3
Caravaca, F., Garcia, C., Hernández, M. T., and Roldán, A. (2002). Aggregate stability changes after organic amendment and mycorrhizal inoculation in the afforestation of a semiarid site with Pinus halepensis. Appl. Soil Ecol. 19, 199–208. doi: 10.1016/S0929-1393(01)00189-5
Cardoso, F. C. G., Capellesso, E. S., de Britez, R. M., Inague, G., and Marques, M. C. M. (2022). Landscape conservation as a strategy for recovering biodiversity: Lessons from a long-term program of pasture restoration in the southern Atlantic Forest. J. Appl. Ecol. 59, 2309–2321. doi: 10.1111/1365-2664.14240
Carver, S., Convery, I., Hawkins, S., Beyers, R., Eagle, A., Kun, Z., et al. (2021). Guiding principles for rewilding. Conserv. Biol. 35, 1882–1893. doi: 10.1111/cobi.13730
Chao, A., and Jost, L. (2012). Coverage-based rarefaction and extrapolation: Standardizing samples by completeness rather than size. Ecology 93, 2533–2547. doi: 10.1890/11-1952.1
Chao, A., Gotelli, N. J., Hsieh, T. C., Sander, E. L., Ma, K. H., Colwell, R. K., et al. (2014). Rarefaction and extrapolation with Hill numbers: A framework for sampling and estimation in species diversity studies. Ecol. Monogr. 84, 45–67. doi: 10.1890/13-0133.1
Chase, J. M. (2003). Community assembly: When should history matter? Oecologia 136, 489–498. doi: 10.1007/s00442-003-1311-7
Chau, L. K. C., and Corlett, R. T. (1994). “Fire and weather in Hong Kong,” in Proceedings of the 12th conference on fire and forest meteorology, Jekyll Island, GA, 442–452.
Chave, J., Andalo, C., Brown, S., Cairns, M. A., Chambers, J. Q., Eamus, D., et al. (2005). Tree allometry and improved estimation of carbon stocks and balance in tropical forests. Oecologia 145, 87–99. doi: 10.1007/s00442-005-0100-x
Chazdon, R. L. (2003). Tropical forest recovery: Legacies of human impact and natural disturbances. Perspect. Plant Ecol. Evol. Syst. 6, 51–71. doi: 10.1078/1433-8319-00042
Chen, Y., Uriarte, M., Wright, S. J., and Yu, S. (2019). Effects of neighborhood trait composition on tree survival differ between drought and postdrought periods. Ecology 100:e02766. doi: 10.1002/ecy.2766
Chung, K. P. S., and Corlett, R. T. (2006). Rodent diversity in a highly degraded tropical landscape: Hong Kong, South China. Biodivers. Conserv. 15, 4521–4532. doi: 10.1007/s10531-005-5102-9
Comaniciu, D., and Meer, P. (1999). “Mean shift analysis and applications,” in Proceedings of the 7th IEEE international conference on computer vision, (Kerkyra: IEEE), 1197–1203. doi: 10.1109/ICCV.1999.790416
Comita, L. S., Condit, R., and Hubbell, S. P. (2007). Developmental changes in habitat associations of tropical trees. J. Ecol. 95, 482–492.
Comita, L. S., Thompson, J., Uriarte, M., Jonckheere, I., Canham, C. D., and Zimmerman, J. K. (2010). Interactive effects of land use history and natural disturbance on seedling dynamics in a subtropical forest. Ecol. Appl. 20, 1270–1284. doi: 10.1890/09-1350.1
Condit, R., Pitman, N., Leigh, E. G., Chave, J., Terborgh, J., Foster, R. B., et al. (2002). Beta-diversity in tropical forest trees. Science 295, 666–669. doi: 10.1126/science.1066854
Connell, J. H., and Slatyer, R. O. (1977). Mechanisms of succession in natural communities and their role in community stability and organization. Am. Nat. 111, 1119–1144. doi: 10.1086/283241
Corlett, R. T. (1994). What is secondary forest? J. Trop. Ecol. 10, 445–447. doi: 10.1017/S0266467400008129
Corlett, R. T. (1999). Environmental forestry in Hong Kong: 1871–1997. For. Ecol. Manag. 116, 93–105. doi: 10.1016/S0378-1127(98)00443-5
Corlett, R. T. (2011). Seed dispersal in Hong Kong, China: Past, present and possible futures. Integr. Zool. 6, 97–109. doi: 10.1111/j.1749-4877.2011.00235.x
Corlett, R. T. (2017). Frugivory and seed dispersal by vertebrates in tropical and subtropical Asia: An update. Glob. Ecol. Conserv. 11, 1–22. doi: 10.1016/j.gecco.2017.04.007
Crouzeilles, R., Ferreira, M. S., Chazdon, R. L., Lindenmayer, D. B., Sansevero, J. B. B., Monteiro, L., et al. (2017). Ecological restoration success is higher for natural regeneration than for active restoration in tropical forests. Sci. Adv. 3:e1701345. doi: 10.1126/sciadv.1701345
Davies, S. J., Abiem, I., Abu Salim, K., Aguilar, S., Allen, D., Alonso, A., et al. (2021). ForestGEO: Understanding forest diversity and dynamics through a global observatory network. Biol. Conserv. 253:108907. doi: 10.1016/j.biocon.2020.108907
de Medeiros-Sarmento, P. S., Ferreira, L. V., and Gastauer, M. (2021). Natural regeneration triggers compositional and functional shifts in soil seed banks. Sci. Total Environ. 753:141934. doi: 10.1016/j.scitotenv.2020.141934
Deng, C., Zhang, S., Lu, Y., Froese, R. E., Xu, X., Zeng, J., et al. (2020). Thinning effects on forest evolution in Masson pine (Pinus massoniana Lamb.) conversion from pure plantations into mixed forests. For. Ecol. Manag. 477:118503. doi: 10.1016/j.foreco.2020.118503
Dent, D. H., and Wright, J. S. (2009). The future of tropical species in secondary forests: A quantitative review. Biol. Conserv. 142, 2833–2843. doi: 10.1016/j.biocon.2009.05.035
Ding, Y., and Zang, R. (2021). Effects of thinning on the demography and functional community structure of a secondary tropical lowland rain forest. J. Environ. Manage. 279:111805. doi: 10.1016/j.jenvman.2020.111805
Dullinger, S., Gattringer, A., Thuiller, W., Moser, D., Zimmermann, N. E., Guisan, A., et al. (2012). Extinction debt of high-mountain plants under twenty-first-century climate change. Nat. Clim. Change 2, 619–622. doi: 10.1038/nclimate1514
Egler, F. E. (1954). Vegetation science concepts I. Initial floristic composition, a factor in old-field vegetation development with 2 figs. Veg. Acta Geobot. 4, 412–417. doi: 10.1007/BF00275587
Feng, Y., Schmid, B., Loreau, M., Forrester, D. I., Fei, S., Zhu, J., et al. (2022). Multispecies forest plantations outyield monocultures across a broad range of conditions. Science 376, 865–868. doi: 10.1126/science.abm6363
Field, J. P., Breshears, D. D., Bradford, J. B., Law, D. J., Feng, X., and Allen, C. D. (2020). Forest management under megadrought: Urgent needs at finer scale and higher intensity. Front. For. Glob. Change 3:502669. doi: 10.3389/ffgc.2020.502669
Filgueiras, B. K. C., Peres, C. A., Melo, F. P. L., Leal, I. R., and Tabarelli, M. (2021). Winner–loser species replacements in human-modified landscapes. Trends Ecol. Evol. 36, 545–555. doi: 10.1016/j.tree.2021.02.006
Food and Agriculture Organization of the United Nations (2018). Global forest resources assessment FRA 2020, terms and definitions, i8661en. Rome: Food and Agriculture Organization of the United Nations.
Fukami, T. (2015). Historical contingency in community assembly: Integrating niches, species pools, and priority effects. Annu. Rev. Ecol. Evol. Syst. 46, 1–23. doi: 10.1146/annurev-ecolsys-110411-160340
Fukami, T., Mordecai, E. A., and Ostling, A. (2016). A framework for priority effects. J. Veg. Sci. 27, 655–657. doi: 10.1111/jvs.12434
Goldsmith, G. R., Comita, L. S., and Chua, S. C. (2011). Evidence for arrested succession within a tropical forest fragment in Singapore. J. Trop. Ecol. 27, 323–326. doi: 10.1017/S0266467411000010
Goldsmith, G. R., Comita, L. S., Morefield, L. L., Condit, R., and Hubbell, S. P. (2006). Long-term research impacts on seedling community structure and composition in a permanent forest plot. For. Ecol. Manag. 234, 34–39. doi: 10.1016/j.foreco.2006.06.011
Graco-Roza, C., Aarnio, S., Abrego, N., Acosta, A. T. R., Alahuhta, J., Altman, J., et al. (2022). Distance decay 2.0–A global synthesis of taxonomic and functional turnover in ecological communities. Glob. Ecol. Biogeogr. 31, 1399–1421. doi: 10.1111/geb.13513
Gunatilleke, C. V. S., Gunatilleke, I. A. U. N., Esufali, S., Harms, K. E., Ashton, P. M. S., Burslem, D. F. R. P., et al. (2006). Species–habitat associations in a Sri Lankan dipterocarp forest. J. Trop. Ecol. 22, 371–384. doi: 10.1017/S0266467406003282
Guo, Y., Wang, B., Li, D., Mallik, A. U., Xiang, W., Ding, T., et al. (2017). Effects of topography and spatial processes on structuring tree species composition in a diverse heterogeneous tropical karst seasonal rainforest. Flora 231, 21–28. doi: 10.1016/j.flora.2017.04.002
Guo, Y., Wang, B., Mallik, A. U., Huang, F., Xiang, W., Ding, T., et al. (2016). Topographic species–habitat associations of tree species in a heterogeneous tropical karst seasonal rain forest, China. J. Plant Ecol. 10, 450–460. doi: 10.1093/jpe/rtw057
Harms, K. E., Condit, R., Hubbell, S. P., and Foster, R. B. (2001). Habitat associations of trees and shrubs in a 50-ha neotropical forest plot: Habitat associations of trees and shrubs. J. Ecol. 89, 947–959. doi: 10.1111/j.1365-2745.2001.00615.x
Hermy, M., and Verheyen, K. (2007). Legacies of the past in the present-day forest biodiversity: A review of past land-use effects on forest plant species composition and diversity. Ecol. Res. 22, 361–371. doi: 10.1007/s11284-007-0354-3
Hill, M. O. (1973). Diversity and evenness: A unifying notation and its consequences. Ecology 54, 427–432. doi: 10.2307/1934352
Hogan, J. A., Zimmerman, J. K., Uriarte, M., Turner, B. L., and Thompson, J. (2016). Land-use history augments environment–plant community relationship strength in a Puerto Rican wet forest. J. Ecol. 104, 1466–1477. doi: 10.1111/1365-2745.12608
Holl, K. D., Luong, J. C., and Brancalion, P. H. S. (2022). Overcoming biotic homogenization in ecological restoration. Trends Ecol. Evol. 37, 777–788. doi: 10.1016/j.tree.2022.05.002
Holl, K. D., Stout, V. M., Reid, J. L., and Zahawi, R. A. (2013). Testing heterogeneity–diversity relationships in tropical forest restoration. Oecologia 173, 569–578. doi: 10.1007/s00442-013-2632-9
Hsieh, T. C., Ma, K. H., and Chao, A. (2016). iNEXT: An R package for rarefaction and extrapolation of species diversity (Hill numbers). Methods Ecol. Evol. 7, 1451–1456. doi: 10.1111/2041-210X.12613
Jakovac, C. C., Bongers, F., Kuyper, T. W., Mesquita, R. C. G., and Peña-Claros, M. (2016). Land use as a filter for species composition in Amazonian secondary forests. J. Veg. Sci. 27, 1104–1116. doi: 10.1111/jvs.12457
Jakovac, C. C., Junqueira, A. B., Crouzeilles, R., Peña-Claros, M., Mesquita, R. C. G., and Bongers, F. (2021). The role of land-use history in driving successional pathways and its implications for the restoration of tropical forests. Biol. Rev. 96, 1114–1134. doi: 10.1111/brv.12694
Johnstone, J. F., Allen, C. D., Franklin, J. F., Frelich, L. E., Harvey, B. J., Higuera, P. E., et al. (2016). Changing disturbance regimes, ecological memory, and forest resilience. Front. Ecol. Environ. 14:369–378. doi: 10.1002/fee.1311
Jost, L. (2007). Partitioning diversity into independent alpha and beta components. Ecology 88, 2427–2439. doi: 10.1890/06-1736.1
Karger, D. N., Tuomisto, H., Amoroso, V. B., Darnaedi, D., Hidayat, A., Abrahamczyk, S., et al. (2015). The importance of species pool size for community composition. Ecography 38, 1243–1253. doi: 10.1111/ecog.01322
Keith, S. A., Newton, A. C., Morecroft, M. D., Bealey, C. E., and Bullock, J. M. (2009). Taxonomic homogenization of woodland plant communities over 70 years. Proc. R. Soc. B Biol. Sci. 276, 3539–3544. doi: 10.1098/rspb.2009.0938
Kennard, D. K. (2002). Secondary forest succession in a tropical dry forest: Patterns of development across a 50-year chronosequence in lowland Bolivia. J. Trop. Ecol. 18, 53–66. doi: 10.1017/S0266467402002031
Koleff, P., Gaston, K. J., and Lennon, J. J. (2003). Measuring beta diversity for presence-absence data. J. Anim. Ecol. 72, 367–382. doi: 10.1046/j.1365-2656.2003.00710.x
Kuussaari, M., Bommarco, R., Heikkinen, R. K., Helm, A., Krauss, J., Lindborg, R., et al. (2009). Extinction debt: A challenge for biodiversity conservation. Trends Ecol. Evol. 24, 564–571. doi: 10.1016/j.tree.2009.04.011
Lai, J., Mi, X., Ren, H., and Ma, K. (2009). Species-habitat associations change in a subtropical forest of China. J. Veg. Sci. 20, 415–423. doi: 10.1111/j.1654-1103.2009.01065.x
Lamb, D., Erskine, P. D., and Parrotta, J. A. (2005). Restoration of degraded tropical forest landscapes. Science 310, 1628–1632. doi: 10.1126/science.1111773
Lebrija-Trejos, E., Pérez-García, E. A., Meave, J. A., Poorter, L., and Bongers, F. (2011). Environmental changes during secondary succession in a tropical dry forest in Mexico. J. Trop. Ecol. 27, 477–489. doi: 10.1017/S0266467411000253
Legendre, P. (2008). Studying beta diversity: Ecological variation partitioning by multiple regression and canonical analysis. J. Plant Ecol. 1, 3–8. doi: 10.1093/jpe/rtm001
Leimu, R., Vergeer, P., Angeloni, F., and Ouborg, N. J. (2010). Habitat fragmentation, climate change, and inbreeding in plants: Habitat fragmentation, climate change and inbreeding in plants. Ann. N. Y. Acad. Sci. 1195, 84–98. doi: 10.1111/j.1749-6632.2010.05450.x
Lepore, M. (2018). fgeo.habitat: Analize soils and tree-habitat data. Available online at: at: https://github.com/helixcn/fgeo.habitat (accessed June 20, 2022).
Lichstein, J. W., Grau, H. R., and Aragón, R. (2004). Recruitment limitation in secondary forests dominated by an exotic tree. J. Veg. Sci. 15, 721–728. doi: 10.1111/j.1654-1103.2004.tb02314.x
Liu, C. L. C., Kuchma, O., and Krutovsky, K. V. (2018). Mixed-species versus monocultures in plantation forestry: Development, benefits, ecosystem services and perspectives for the future. Glob. Ecol. Conserv. 15:e00419. doi: 10.1016/j.gecco.2018.e00419
Liu, J.-J., and Slik, J. W. F. (2014). Forest fragment spatial distribution matters for tropical tree conservation. Biol. Conserv. 171, 99–106. doi: 10.1016/j.biocon.2014.01.004
Liu, J.-J., Coomes, D. A., Gibson, L., Hu, G., Liu, J., Luo, Y., et al. (2019). Forest fragmentation in China and its effect on biodiversity. Biol. Rev. 94, 1636–1657. doi: 10.1111/brv.12519
Lopez, O. R., and Kursar, T. A. (2003). Does flood tolerance explain tree species distribution in tropical seasonally flooded habitats? Oecologia 136, 193–204. doi: 10.1007/s00442-003-1259-7
Lugo, A. E. (1992). “Tree plantations for rehabilitating damaged forest lands in the tropics,” in Ecosystem rehabilitation: Ecosystem analysis and synthesis, ed. K. Mohan (Hague: SPB Academic Publishing), 247–255.
Luke, S. H., Barclay, H., Bidin, K., Chey, V. K., Ewers, R. M., Foster, W. A., et al. (2017). The effects of catchment and riparian forest quality on stream environmental conditions across a tropical rainforest and oil palm landscape in Malaysian Borneo. Ecohydrology 10, e1827. doi: 10.1002/eco.1827
Lutz, J. A., Furniss, T. J., Johnson, D. J., Davies, S. J., Allen, D., Alonso, A., et al. (2018). Global importance of large-diameter trees. Glob. Ecol. Biogeogr. 27, 849–864. doi: 10.1111/geb.12747
Maestre, F. T., and Cortina, J. (2004). Are Pinus halepensis plantations useful as a restoration tool in semiarid Mediterranean areas? For. Ecol. Manag. 198, 303–317. doi: 10.1016/j.foreco.2004.05.040
McKinney, M. L., and Lockwood, J. L. (1999). Biotic homogenization: A few winners replacing many losers in the next mass extinction. Trends Ecol. Evol. 14, 450–453. doi: 10.1016/S0169-5347(99)01679-1
Meli, P., Holl, K. D., Rey Benayas, J. M., Jones, H. P., Jones, P. C., Montoya, D., et al. (2017). A global review of past land use, climate, and active vs. passive restoration effects on forest recovery. PLoS One 12:e0171368. doi: 10.1371/journal.pone.0171368
Nicholson, B. (1996). Tai Po Kau nature reserve, new territories, Hong Kong: A reafforestation history. Asian J. Environ. Manag. 4, 103–120.
Norden, N., Mesquita, R. C. G., Bentos, T. V., Chazdon, R. L., and Williamson, G. B. (2011). Contrasting community compensatory trends in alternative successional pathways in central Amazonia. Oikos 120, 143–151. doi: 10.1111/j.1600-0706.2010.18335.x
Oksanen, J., Simpson, G. L., Blanchet, F. G., Kindt, R., Legendre, P., Minchin, P. R., et al. (2022). vegan: Community ecology package Version 2.6-4. Available online at: https://CRAN.R-project.org/package=vegan (accessed October 12, 2022).
Osazuwa-Peters, O. L., Jiménez, I., Oberle, B., Chapman, C. A., and Zanne, A. E. (2015). Selective logging: Do rates of forest turnover in stems, species composition and functional traits decrease with time since disturbance?–A 45year perspective. For. Ecol. Manag. 357, 10–21. doi: 10.1016/j.foreco.2015.08.002
Palma, A. C., Goosem, M., Fensham, R. J., Goosem, S., Preece, N. D., Stevenson, P. R., et al. (2021). Dispersal and recruitment limitations in secondary forests. J. Veg. Sci. 32:e12975. doi: 10.1111/jvs.12975
Palma, A. C., Goosem, M., Stevenson, P. R., and Laurance, S. G. W. (2020). Enhancing plant diversity in secondary forests. Front. For. Glob. Change 3:571352. doi: 10.3389/ffgc.2020.571352
Paul, J. R., Randle, A. M., Chapman, C. A., and Chapman, L. J. (2004). Arrested succession in logging gaps: Is tree seedling growth and survival limiting? Afr. J. Ecol. 42, 245–251. doi: 10.1111/j.1365-2028.2004.00435.x
Peres-Neto, P. R., Legendre, P., Dray, S., and Borcard, D. (2006). Variation partitioning of species data matrices: Estimation and comparison of fractions. Ecology 87, 2614–2625. doi: 10.1890/0012-9658(2006)87[2614:vposdm]2.0.co;2
Picard, N. (2019). Asymmetric competition can shape the size distribution of trees in a natural tropical forest. For. Sci. 65, 562–569. doi: 10.1093/forsci/fxz018
Poorter, L., Bongers, F., Aide, T. M., Almeyda Zambrano, A. M., Balvanera, P., Becknell, J. M., et al. (2016). Biomass resilience of neotropical secondary forests. Nature 530, 211–214. doi: 10.1038/nature16512
Poorter, L., Craven, D., Jakovac, C. C., van der Sande, M. T., Amissah, L., Bongers, F., et al. (2021). Multidimensional tropical forest recovery. Science 374, 1370–1376. doi: 10.1126/science.abh3629
Reid, J. L., Fagan, M. E., Lucas, J., Slaughter, J., and Zahawi, R. A. (2019). The ephemerality of secondary forests in southern Costa Rica. Conserv. Lett. 12:e12607. doi: 10.1111/conl.12607
Réjou-Méchain, M., Tanguy, A., Piponiot, C., Chave, J., and Hérault, B. (2017). biomass: An r package for estimating above-ground biomass and its uncertainty in tropical forests. Methods Ecol. Evol. 8, 1163–1167. doi: 10.1111/2041-210X.12753
Rozendaal, D. M. A., Bongers, F., Aide, T. M., Alvarez-Dávila, E., Ascarrunz, N., Balvanera, P., et al. (2019). Biodiversity recovery of Neotropical secondary forests. Sci. Adv. 5:eaau3114. doi: 10.1126/sciadv.aau3114
Schmitt, S., Tysklind, N., Derroire, G., Heuertz, M., and Hérault, B. (2021). Topography shapes the local coexistence of tree species within species complexes of Neotropical forests. Oecologia 196, 389–398. doi: 10.1007/s00442-021-04939-2
Shen, G., Yu, M., Hu, X.-S., Mi, X., Ren, H., Sun, I.-F., et al. (2009). Species–area relationships explained by the joint effects of dispersal limitation and habitat heterogeneity. Ecology 90, 3033–3041. doi: 10.1890/08-1646.1
Siemann, E., and Rogers, W. E. (2006). Recruitment limitation, seedling performance and persistence of exotic tree monocultures. Biol. Invasions 8, 979–991.
Silva, W. R., Zaniratto, C. P., Ferreira, J. O. V., Rigacci, E. D. B., Oliveira, J. F., Morandi, M. E. F., et al. (2020). Inducing seed dispersal by generalist frugivores: A new technique to overcome dispersal limitation in restoration. J. Appl. Ecol. 57, 2340–2348. doi: 10.1111/1365-2664.13731
Stephenson, N. L., Das, A. J., Condit, R., Russo, S. E., Baker, P. J., Beckman, N. G., et al. (2014). Rate of tree carbon accumulation increases continuously with tree size. Nature 507, 90–93. doi: 10.1038/nature12914
Svenning, J.-C., and Faurby, S. (2017). Prehistoric and historic baselines for trophic rewilding in the Neotropics. Perspect. Ecol. Conserv. 15, 282–291. doi: 10.1016/j.pecon.2017.09.006
Tabarelli, M., Peres, C. A., and Melo, F. P. L. (2012). The ‘few winners and many losers’ paradigm revisited: Emerging prospects for tropical forest biodiversity. Biol. Conserv. 155, 136–140. doi: 10.1016/j.biocon.2012.06.020
Thessler, S., Ruokolainen, K., Tuomisto, H., and Tomppo, E. (2005). Mapping gradual landscape-scale floristic changes in Amazonian primary rain forests by combining ordination and remote sensing: Mapping floristic changes in Amazonian rain forests. Glob. Ecol. Biogeogr. 14, 315–325. doi: 10.1111/j.1466-822X.2005.00158.x
Thompson, J., Lugo, A. E., and Thomlinson, J. (2007). Land use history, hurricane disturbance, and the fate of introduced species in a subtropical wet forest in Puerto Rico. Plant Ecol. 192, 289–301. doi: 10.1007/s11258-007-9318-5
Tomimura, C., Singhakumara, B. M. P., and Ashton, P. M. S. (2012). Pattern and Composition of secondary succession beneath Caribbean pine plantations of Southwest Sri Lanka. J. Sustain. For. 31, 818–834. doi: 10.1080/10549811.2011.640076
Turner, I. M., and Corlett, R. T. (1996). The conservation value of small, isolated fragments of lowland tropical rain forest. Trends Ecol. Evol. 11, 330–333. doi: 10.1016/0169-5347(96)10046-X
Uhl, C. (1987). Factors controlling succession following slash-and-burn agriculture in Amazonia. J. Ecol. 75:377. doi: 10.2307/2260425
Uriarte, M., Canham, C. D., Thompson, J., and Zimmerman, J. K. (2004). a neighborhood analysis of tree growth and survival in a hurricane-driven tropical forest. Ecol. Monogr. 74, 591–614. doi: 10.1890/03-4031
van Schaik, C. P., Terborgh, J. W., and Wright, S. J. (1993). The phenology of tropical forests: Adaptive significance and consequences for primary consumers. Annu. Rev. Ecol. Syst. 24, 353–377. doi: 10.1146/annurev.es.24.110193.002033
Weidlich, E. W. A., Nelson, C. R., Maron, J. L., Callaway, R. M., Delory, B. M., and Temperton, V. M. (2021). Priority effects and ecological restoration. Restor. Ecol. 29:e13317. doi: 10.1111/rec.13317
Whitfeld, T. J. S., Lasky, J. R., Damas, K., Sosanika, G., Molem, K., and Montgomery, R. A. (2014). Species richness, forest structure, and functional diversity during succession in the New Guinea lowlands. Biotropica 46, 538–548. doi: 10.1111/btp.12136
Wilson, M. V., and Shmida, A. (1984). Measuring beta diversity with presence-absence data. J. Ecol. 72:1055. doi: 10.2307/2259551
Wright, S. J. (2005). Tropical forests in a changing environment. Trends Ecol. Evol. 20, 553–560. doi: 10.1016/j.tree.2005.07.009
Wu, C., Jiang, B., Yuan, W., Shen, A., Yang, S., Yao, S., et al. (2020). On the management of large-diameter trees in China’s forests. Forests 11:111. doi: 10.3390/f11010111
Wu, W., Kuang, L., Li, Y., He, L., Mou, Z., Wang, F., et al. (2021). Faster recovery of soil biodiversity in native species mixture than in Eucalyptus monoculture after 60 years afforestation in tropical degraded coastal terraces. Glob. Change Biol. 27, 5329–5340. doi: 10.1111/gcb.15774
Xu, H., Li, Y., Liu, S., Zang, R., He, F., and Spence, J. R. (2015). Partial recovery of a tropical rain forest a half-century after clear-cut and selective logging. J. Appl. Ecol. 52, 1044–1052. doi: 10.1111/1365-2664.12448
Yadav, J., and Sharma, M. (2013). A review of k-mean algorithm. Int. J. Eng. Trends Technol. 4, 2972–2976.
Yin, D., Liu, Y., Ye, Q., Cadotte, M. W., and He, F. (2021). Trait hierarchies are stronger than trait dissimilarities in structuring spatial co-occurrence patterns of common tree species in a subtropical forest. Ecol. Evol. 11, 7366–7377. doi: 10.1002/ece3.7567
Young, A., Boyle, T., and Brown, T. (1996). The population genetic consequences of habitat fragmentation for plants. Trends Ecol. Evol. 11, 413–418. doi: 10.1016/0169-5347(96)10045-8
Young, T. P., and Peffer, E. (2010). “Recalcitrant understory layers” revisited: Arrested succession and the long life-spans of clonal mid-successional species. Can. J. For. Res. 40, 1184–1188. doi: 10.1139/X10-066
Zeng, X., Michalski, S. G., Fischer, M., and Durka, W. (2012). Species diversity and population density affect genetic structure and gene dispersal in a subtropical understory shrub. J. Plant Ecol. 5, 270–278. doi: 10.1093/jpe/rtr029
Zhang, H., Chen, H. Y. H., Lian, J., John, R., Li Ronghua Liu, H., et al. (2018). Using functional trait diversity patterns to disentangle the scale-dependent ecological processes in a subtropical forest. Funct. Ecol. 32, 1379–1389. doi: 10.1111/1365-2435.13079
Zhao, M., Pan, B., Tan, Y., and Corlett, R. T. (2015). Winners and losers among tree species in Xishuangbanna: Which traits are most important? Sci. Bull. 60, 916–924. doi: 10.1007/s11434-015-0799-7
Zhuang, X.-Y. (1997). Rehabilitation and development of forest on degraded hills of Hong Kong. For. Ecol. Manag. 99, 197–201. doi: 10.1016/S0378-1127(97)00205-3
Zhuang, X.-Y., and Corlett, R. T. (1997). Forest and forest succession in Hong Kong. China. J. Trop. Ecol. 13, 857–866. doi: 10.1017/S0266467400011032
Zobel, M., van der Maarel, E., and Dupré, C. (1998). Species pool: The concept, its determination and significance for community restoration. Appl. Veg. Sci. 1, 55–66. doi: 10.2307/1479085
Keywords: subtropical forest, land-use history, biodiversity, aerial photograph, torus translation test, succession, forest restoration, vegetation transition types
Citation: Zhu H, Zhang J, Cheuk ML, Hau BCH, Fischer GA and Gale SW (2023) Monoculture plantations impede forest recovery: Evidence from the regeneration of lowland subtropical forest in Hong Kong. Front. For. Glob. Change 6:1098666. doi: 10.3389/ffgc.2023.1098666
Received: 15 November 2022; Accepted: 05 January 2023;
Published: 01 February 2023.
Edited by:
Nophea Sasaki, Asian Institute of Technology, ThailandReviewed by:
Bhoj Raj Ghimire, Nepal Open University, NepalYan Gao, Universidad Nacional Autónoma de México, Mexico
Copyright © 2023 Zhu, Zhang, Cheuk, Hau, Fischer and Gale. This is an open-access article distributed under the terms of the Creative Commons Attribution License (CC BY). The use, distribution or reproduction in other forums is permitted, provided the original author(s) and the copyright owner(s) are credited and that the original publication in this journal is cited, in accordance with accepted academic practice. No use, distribution or reproduction is permitted which does not comply with these terms.
*Correspondence: Jinlong Zhang, amx6aGFuZ0BrZmJnLm9yZw==; Stephan W. Gale,
c3RlcGhhbmdhbGVAa2ZiZy5vcmc=