Weak phylogenetic and habitat effects on root trait variation of 218 Neotropical tree species
- 1Department of Biological Sciences, International Center for Tropical Botany, Florida International University, Miami, FL, United States
- 2AMAP (Botanique et Modélisation de l’Architecture des Plantes et des Végétations), Université de Montpellier, CIRAD, CNRS, INRAE, IRD, Montpellier, France
- 3Coordination of Environmental Dynamics, National Institute for Amazonian Research, Manaus, Brazil
- 4Laboratório de Ecologia e Evolução de Plantas da Amazônia (Labotam), Instituto Nacional de Pesquisas da Amazônia, Manaus, Brazil
- 5Facultad de Ciencias Forestales, Universidad Nacional de la Amazonía Peruana, Iquitos, Peru
- 6Programa de Pós-Graduação em Botânica, Instituto Nacional de Pesquisas da Amazônia, Manaus, Amazonas, Brazil
- 7Department of Biology of Organisms, Faculty of Sciences, Université Libre de Bruxelles, Brussels, Belgium
Introduction: Tropical forests harbor a large diversity of closely related tree species that can thrive across habitats. This biodiversity has been found to correspond to large functional diversity in aboveground traits, and likely also relates to belowground trait variation. Globally, root trait (co-)variation is driven by different belowground resource strategies of species, environmental variation, and phylogeny; however, these patterns mostly reflect observations from temperate biomes and remain unconfirmed in tropical trees. We examine phylogenetic and environmental effects on root trait (co-)variation of trees across habitats in an Amazonian rainforest.
Methods: Roots of 218 tree species from ten dominant families were sampled across three major habitats near Manaus, Brazil. We quantified five morphological and architectural root traits to (i) investigate how they reflected different resource strategies across species, (ii) compare them between families and superorders to test phylogenetic effects, and (iii) compare them between habitats to determine environmental effects on root trait expressions and variability.
Results: Root traits discriminated species along a tradeoff between root diameter and root branching and, secondly, due to variation in root tissue density. Our results further show weak phylogenetic effects on tropical tree root variation, for example, families from the same superorder showed large divergence in their root traits, while those from different superorders often overlapped in their root morphology and architecture. Root traits differed significantly between habitats but habitat type had only little effect on overall root trait variation.
Discussion: Our work suggests that the dimensions and drivers that underlie (co-)variation in tropical root traits may differ from global patterns defined by mostly temperate datasets. Due to (a)biotic environmental differences, different root trait dimensions may underlie the belowground functional diversity in (Neo)tropical forests, and we found little evidence for the strong phylogenetic conservatism observed in root traits in temperate biomes. We highlight important avenues for future research on tropical roots in order to determine the degree of, and shifts in functional diversity belowground as communities and environments change in tropical forests.
Introduction
Tropical rainforests in the Amazon basin harbor the highest tree diversity on earth (Ter Steege et al., 2020). Most of this diversity is concentrated in a relatively small number of plant families, including Chrysobalanaceae, Fabaceae, Lauraceae, Lecythidaceae, Malvaceae (sensu lato), Myrtaceae, Rubiaceae, and Sapotaceae, that can comprise > 60% of all canopy individuals (Gentry, 1988; ter Steege et al., 2013). These families usually include dozens of sympatric species thriving in close proximity and dominating communities with contrasting soil and hydric regime conditions (Kursar et al., 2009; Pitman et al., 2013; Allié et al., 2015). From a deterministic perspective, such ability to diversify in proximity suggests that closely related populations in these families can finely tune their trait expressions to better adapt to local differences in resource availabilities (Fine and Kembel, 2011). Part of such phenotypic adaptation occurs aboveground, where reproductive organs, leaves and wood properties vary among sympatric species, suggesting different functional strategies for resource acquisition and use, response to herbivore pressure, and pollination vectors, explaining genus diversification and forest community compositions in the Neotropics (Kursar et al., 2009; Fortunel et al., 2014; Dick and Pennington, 2019). Another, probably equally important but largely ignored part of functional diversity can be observed belowground where roots acquire water and nutrients essential to the functioning and performance of trees. Given the multiple belowground strategies that tree species can adopt to acquire different soil resources (Weemstra et al., 2016), it is likely that the high biodiversity of species in tropical forests is reflected by a multitude of root systems with different trait expressions and covariations. However, low root trait data availability in the tropics, especially at the species level (Cusack et al., 2021), has prevented us from characterizing tropical root trait diversity and its drivers, and thus from grasping a large component of the functional diversity of tropical forests.
Globally, interspecific variation in root traits can be captured in two main dimensions (Bergmann et al., 2020). First, a “collaboration axis” opposes species that produce thin roots with high specific root length (SRL, root length per unit root dry mass) to efficiently acquire soil resources (i.e., the “do-it-yourself” strategy), and species that construct thick, low-SRL roots with ample colonization space for (arbuscular) mycorrhizal fungi to which they outsource soil resource uptake (i.e., the “outsourcing” strategy). A second, independent “conservation axis,” in turn, separates species with mass-dense and presumably long-lived roots which permits long-term resource conservation, from species with high root nitrogen (N) concentrations indicating active root metabolism and fast root turnover. This “root economics space” (RES) framework has proved instrumental in explaining the different ways in which plants acquire belowground resources (Bergmann et al., 2020), how plants coordinate the functioning of their above- and belowground organs (Carmona et al., 2021; Weigelt et al., 2021), interspecific variation in tree growth (Weemstra et al., 2021b) and species distributions across climatic regions (Laughlin et al., 2021).
While the demonstrated RES spans species from different biomes, the vast majority of root data is collected in temperate and continental biomes (82% of the total number of species separated by biome while only 16% are tropical species) (Bergmann et al., 2020). As tropical areas encompass > 65% of all flowering plants (Wright, 2007), tropical species are vastly underrepresented in global databases, and the resultant RES framework therefore mostly reflects root trait patterns in the temperate zone. A separate analysis by Bergmann et al. (2020) among a subset of 81 tropical species in their dataset confirmed the existence of an RES featuring the collaboration and conservation axes and the four associated key traits described above. However, strong environmental differences between temperate and tropical forests suggest that different trait axes may describe belowground diversity in the (sub)tropics. For example, due to the overrepresentation of temperate species, the global RES may mostly reflect root trait expressions that favor the uptake of N, which is generally the limiting nutrient in temperate forests. In contrast, tropical trees on old, weathered soils mostly face phosphorus (P) limitations that may select for different root traits and tradeoffs. Firstly, arbuscular mycorrhizal (AM) fungi−that are mainly involved in P rather than N uptake−dominate mycorrhizal symbioses among (Neo)tropical trees, whereas ectomycorrhizal (EcM) symbiosis−that is involved in the acquisition of both P and organic and inorganic N−is more common in temperate regions (Brundrett, 2002; Soudzilovskaia et al., 2019). Because AM but not EcM fungal colonization is dependent on the size of the root cortex (Brundrett, 2002) and thus root diameter (Gu et al., 2014; Kong et al., 2014), having thick roots with ample space for AM fungi may be a more beneficial and prevalent strategy in tropical than temperate forests (Valverde-Barrantes et al., 2021). Secondly, tropical soils may select more strongly for highly branched roots than temperate systems. Owing to the high mobility of (inorganic) N and low mobility of P, depletion zones around the root are much larger for N than for P, leading to strong competition among densely clustered roots and making highly branched roots more beneficial for P than N uptake (Postma et al., 2014). This division in root architecture and function corroborates previous species separations along root branching patterns in subtropical trees (Kong et al., 2014), and the differences in phosphatase production of tropical trees with different root morphologies (Lugli et al., 2020). Given these biotic and abiotic environmental differences, different root trait dimensions may underlie a tropical RES.
Besides environmental factors, phylogeny is another major driver of root trait diversity that may have different effects in tropical and temperate forests. In data collected to date, root traits are phylogenetically conserved at the superorder level (Baylis, 1975; Kembel and Cahill, 2005; Chen et al., 2013; Comas et al., 2014; Gu et al., 2014; Kong et al., 2014; Valverde-Barrantes et al., 2015), owing in part to evolutionary changes in root morphology coinciding with shifts in mycorrhizal associations and dependencies (Comas et al., 2012; Ma et al., 2018). Associations with AM fungi are the ancestral state of the symbiosis, and the dependency of these symbionts on root cortical size and root diameter explains why more basal superorders, like Magnoliids, have thick roots with large cortices, and would thus typically adopt the outsourcing strategy (Baylis, 1975; Guo et al., 2008; Kubisch et al., 2015; Valverde-Barrantes et al., 2015, 2016). More recently derived clades (like Asterids and Rosids), in turn, started forming thin, highly branched roots that were more efficient for soil exploration and exploitation allowing them to reduce their dependency on mycorrhizal fungi (Comas et al., 2012)−i.e., the do-it-yourself strategy. However, this notion of root phylogenetic conservatism is largely based on compilations of temperate species. Although little is known about variation in tropical taxa, initial evidence suggests important departures from the observed temperate patterns. For example, roots of some species in rosid families with predominantly tropical distributions, like Euphorbiaceae, Moraceae, and Rutaceae, bear trait syndromes similar to those of the Magnoliids (Gu et al., 2014; Kong et al., 2014). Through niche segregation, such belowground functional hyperdiversity may allow different tropical species from the same families to perform well in the same or across environments, and thus weaken phylogenetic patterns across species in a tropical RES.
To test how environmental cues and phylogenetic backgrounds drive tropical root trait diversity, we created an unprecedented dataset collating root morphological and architectural information across 218 tree species in central western Amazonia growing in three distinct forest habitats [terra firme (TF), seasonally flooded (SF) and white-sand (WS) forests] representative of environmental gradients found in Amazonia. The main objectives of this study are to (i) characterize (co-)variation in five morphological and architectural root traits partially associated with the global RES across a large set of Neotropical tree species, and (ii) determine how and to what extent root trait variation is driven by ancestry and/or environmental factors. In line with these aims, we formulated three sets of hypotheses:
Hypothesis 1: (H1a) Across tropical species, root traits vary along a conservation axis (reflected by variation in root tissue density) but even more so along a collaboration axis. Along the collaboration axis we expect that (H1b) the do-it-yourself strategy consists of two components: a high SRL to expand the root surface area and thus root resource acquisition relative to biomass investments as predicted by the RES, and/or a high degree of root branching (not included in the global RES) to stimulate proliferation in nutrient-rich patches. Both root trait expressions reflect functions that can be outsourced to mycorrhizal fungi, and both may thus trade-off with the outsourcing strategy (i.e., reflected by high root diameter).
Hypothesis 2: (H2a) Within this tropical root traits space, magnoliid species have thick, low-SRL and poorly branched roots, rosid species have opposite trait values−i.e., thin, high-SRL and highly branched roots−whereas asterid species display intermediate trait expressions, as observed globally (Valverde-Barrantes et al., 2017). However, (H2b) within superorders, ancestry will play a minor role in the variation in root traits of tropical trees. Across species, root traits will therefore overlap across families from different superorders, and diverge across families from the same superorder, reflected by low phylogenetic conservatism in root traits at the superorder level, and stronger phylogenetic effects at lower taxonomic levels (e.g., family, genus, species).
Hypothesis 3: Between habitats, root traits differ in their expressions and variability at the community level (i.e., across all sampled trees). We expect that (H3a), trees growing on WS−sandy soils with the lowest soil water and nutrient availability−are expected to have the highest root tissue density to conserve resources; have thick, low-SRL roots reflecting a mycorrhizal outsourcing strategy because nutrient uptake is more efficient through mycorrhizal symbiosis; and have poorly branched roots, as soil resources are acquired predominantly through the mycorrhizal pathway, circumventing the need for proliferous root tips. Conversely, trees in SF forests−with highest soil fertility−would produce the least mass-dense roots; would have thin, high-SRL roots reflecting the do-it-yourself strategy because under higher nutrient levels, expensive carbon investments in mycorrhizal symbiosis are less necessary; and have highly branched roots to rapidly exploit nutrient (especially P) patches. Finally, trees on TF with intermediate soil fertility would have intermediate trait values compared to the other two habitats. We also hypothesize (H3b) that root trait variability will be smallest on WS and highest on SF, owing to stronger environmental filtering in low-resource environments.
Materials and methods
Study design
The study was carried out north of Manaus (3°6′6.98″S, 60°1′30″W) in the Amazonas State, Brazil (Supplementary Figure 1). The vegetation in the area is an old-growth lowland rainforest with a mean annual temperature range between 26°C (April) and 28°C (September) and a mean annual precipitation of around 2,400 mm (Araújo et al., 2002). The precipitation regime in the area includes a rainy season typically from January to May, and a drier period between July and September (Fontes et al., 2018). This study focused on the root traits of ten families (Table 1) that dominate in (Neo)tropical forests (Gentry, 1988) belonging to three major angiosperm superorders (Asterids, Magnoliids, and Rosids; Supplementary Figure 2) that have been reported to diverge on belowground syndromes at global scales (Valverde-Barrantes et al., 2015). These families are abundant on three common habitat types in lowland Amazonia forests that differ in their edaphic attributes (Baraloto et al., 2011) and in their taxonomic (Baraloto et al., 2021) and functional (Fortunel et al., 2014) compositions. The mycorrhizal type (EcM or AM) of tropical trees is not yet well documented, and while the vast majority of tree species in the tropics are AM, EcM associations have been observed within the Fabaceae, Moraceae and Myrtaceae. When scanning our roots, we observed no visual signs of EcM fungi (e.g., rhizomorphs or fungal mantles on root tips), so we assume that virtually all species in our dataset associate with AM fungi, but we cannot exclude the possibility of EcM associations.
Three forest sites were selected in the Manaus region: Reserva Ducke, RDS Uatumã, and Tarumã Mirim (see map: Supplementary Figure 1). At each site, we selected areas that represented the three major rain forest habitat types with contrasting soil environments that are among the most common habitats in lowland Amazonia, and each have a distinct flora (Fine and Kembel, 2011; Baraloto et al., 2021). First, upland TF forests on clay-rich soils include relatively nutrient rich and clay-dominated Ferrasols (Araújo et al., 2002). Second, SF forests include stands where the water table is always within 60 cm depth and remains at the soil surface for at least two consecutive months each year (Baraloto et al., 2011). The third habitat are WS forests, characterized by soils with extremely low cation and P content in the parent material, high proportions of sand, and little organic material below the surface horizons, usually classified as Podzols (Quesada et al., 2009). To describe these habitats more specifically for our study sites, we collected and analyzed soil samples as further explained below.
For each habitat per site, we established two to six plots with at least 500 m between plots giving a total of 27 plots. These plots consisted of a 200 m transect along which ten lateral 50 m long, and 2-m wide sub-transects were established at 20 m distance from each other. Within each plot, we selected between 5 and 10 individuals of each lineage for root sampling (average of 60 ± 5 individuals per plot), with a range of 361–429 trees per habitat, per site. Individual tree sampling focused on the most abundant taxonomic group present across all plots. In the case of families like Burseraceae, Chrysobalanaceae, Fabaceae, Lecythidaceae, and Sapotaceae, we sampled species within a single genus: Protium, Licania (sensu lato), Inga, Eschweilera, and Micropholis, respectively, since these genera were present in nearly all plots. For the Lauraceae, Malvaceae, Moraceae, Myrtaceae, and Rubiaceae, we sampled individuals within the family irrespective of the genus. Individuals were juvenile trees ranging from 1 to 4 meters in tree height. We focused on juveniles because large canopy trees often have expansive root systems that are difficult to trace and guarantee species’ identification. How juvenile root traits resemble those of larger adults in this old-growth forest remains an open question since ontogenetic effects on tropical root traits are rarely studied. Nevertheless, our sampling design compared species and sites for a controlled ontogenetic stage.
In total, our dataset comprised 1,222 trees from 218 species representing 52 genera. Per family, between 81 (Sapotaceae) and 153 (Myrtaceae) trees were sampled, ranging in their taxonomic representation from 5 (Malvaceae) to 36 (Lauraceae) species (Table 1 and Supplementary Figure 2). Between habitats, the number of trees varied between 361 (on TF) and 432 (on white-sands), and the number of species between 95 (in white-sand habitats) and 124 (on TF) (Supplementary Table 1). Individual vouchers of each morpho-species in each plot were collected for posterior identification. All vouchers were deposited in the herbarium of the Instituto Nacional de Pesquisas da Amazonia (INPA).
Root sampling and trait measurements
In this study, our definition of “fine roots” follows an order-based, morphometric classification (Fitter, 1987; Pregitzer et al., 2002; Freschet et al., 2021a) which includes only the first three root orders that, for trees, play the largest roles in soil resource uptake (Pregitzer et al., 2002; McCormack et al., 2015). These root orders are in turn determined based on their position along the root system: first-order roots, also “root tips,” are the most distal ends of a root system (Fitter, 1987). Higher order roots, in turn, are the parents of the lower order roots: the parent roots from a first-order root are second-order roots, and where two second-order roots meet, the third-order root begins, etc (Freschet et al., 2021a). Roots become increasingly thick with root order as they progressively transition from resource acquisition to resource transport and plant anchorage (McCormack et al., 2015). Roots above the third-order are therefore less relevant in the context of a RES which conceptualizes resource uptake strategies, and are excluded from this study.
Roots were sampled for each focal tree, using standard protocols described in great detail by Freschet et al. (2021a). In short, we partially exposed a coarse woody root at the base of a target individual identified to species. These coarse roots were traced by gently excavating them until reaching the most distal parts of the root system to ensure sampled roots belonged to the target individual and species. For each individual, we excavated 3–5 roots each consisting of 5–10 long (higher-order) roots to which fine roots (i.e., first- to third-order roots) were attached. These roots were sampled from the top 10 cm of the soil where they are most abundant (and because deeper roots are more difficult to trace). The fine roots were then sorted, sealed in a plastic bag, and kept on ice until further preparation of samples. In the laboratory, we selected and washed all fine roots by removing any adhering soil aggregates and any dead root fragments identified based on their toughness and flexibility (Brassard et al., 2013). Individual clusters of fine roots were scanned using a high-resolution flatbed scanner (Epson Scanner Perfection V700 Photo, USA, 600 DPI resolution, 256-level gray-scale).
Scans of first- to third-order roots were analyzed using WinRhizo software (2007 Pro version, Regent Instruments Inc., Québec, Canada) providing measurements on total root length, average root diameter, total root volume and the number of root tips (i.e., first-order roots). Then, root samples were oven-dried for 48 h at 65°C and weighed. From these data, we obtained average diameter (mm) directly from WinRhizo, and calculated SRL (total root length divided by total root dry weight, m g–1), root tissue density (total root dry weight divided by total root volume, g cm–3), specific root tip abundance (SRTA; number of root tips divided by the total dry root mass, root tips g–1), and root branching intensity (number of root tips divided by the total root length, root tips cm–1).
Soil properties across habitats
To confirm soil differences between the three studied habitats at our three study sites, we collected and analyzed samples from surface soils (0–10 cm soil depth) from which roots were sampled. Soil samples were collected at each intersection between the ten sub-transects and the main transect, and the ten soil samples then pooled per plot (n = 27). Soil analyses were carried out at INPA following standardized protocols. More specifically, soil texture, i.e., sand, silt and clay concentrations, was determined by quantifying their settling rates and changes in suspension densities in an aqueous solution using a hydrometer. Soil samples were further analyzed following standardized methods for pH (in KCl), total N (Kjeldahl analysis), available P (Mehlich-1 method), exchangeable potassium (K) (Mehlich-1 method), exchangeable calcium (Ca) and magnesium (Mg) (1M KCl replacement), and organic carbon (C) and organic matter (Walkley-Black method).
Statistical analyses
All statistical analyses were carried out with R statistical software (R Core Team, 2022). Firstly, the phylogenetic relationships among our ten studied families and their superorders were qualitatively visualized (Supplementary Figure 2) using FigTree software (Version 1.4.3).1 The phylogeny is based on a phylogenetic tree constructed by Baraloto et al. (2012) using molecular sequences of matK and rbcL chloroplast genes from approximately 650 tree species of Amazonian forests. To broadly describe our root trait dataset, we determined trait means and standard errors at the species and family level, calculated coefficients of trait variation (standard deviation/mean) across all individuals (n = 1,222) and across species means (n = 218), and tested bivariate Pearson correlations among root traits at the species level. Prior to further data analyses, soil and root trait data were normalized using a Box-Cox transformation and standardized [using the decostand function in the “vegan” package; Oksanen et al., 2019]. To examine differences in soil properties across habitats for the 27 plots, we ran ANCOVA models with individual soil properties as dependent variables, “habitat” as main effect, and “site” as blocking variable. When ANCOVA results showed a significant habitat effect (α = 0.05), a Tukey’s test (Tukey, 1949) was carried out with the TukeyHSD function (“stats” package; R Core Team, 2022) to compare soil properties between pairs of habitats.
To test H1 (the existence of a RES and its trait dimensions), we determined multivariate root trait covariations using a principal component analysis (PCA) across mean traits of all 218 species with the prcomp function in the “stats” package (R Core Team, 2022). Parallel analysis (Horn, 1965; using the paran function in the “paran” package; Dinno, 2018) showed that the first two principal components (PCs) were significant, and we extracted these for further analyses. Per family, we calculated the absolute difference between the lower and upper end of the 95% confidence intervals for species’ scores on the two significant PCs to determine each family’s range along these axes. We then tested whether these ranges differed between PCs across all families with a Wilcoxon rank sum test (wilcox.test in “stats” package). To determine if families that were more flexible (i.e., having larger ranges) along PC1 were also more flexible along PC2, we calculated the ratio between these two ranges (range along PC1/range along PC2). The null hypothesis is that root traits are equally variable along both axes, so we used a one-sample Wilcoxon rank sum to test whether range ratios were overall different from 1.
We then tested whether superorders and families differed in their root traits, and compared their positions in the root traits space (H2). We conducted permutational multivariate analyses of variance (PERMANOVA; Anderson, 2017) to compare their centroids (i.e., their multivariate root trait means) using the adonis function in the “vegan” package (Oksanen et al., 2019). Centroids and their 95% confidence intervals were extracted using the ordiellipse function in “vegan” (Oksanen et al., 2019). When PERMANOVA tests were significant (α = 0.05), we carried out a post hoc comparison among pairs of superorders or families with the pairwise.adonis function in the “pairwiseAdonis” package (Martinez Arbizu, 2017).
To determine how root trait expressions relate to habitat conditions (H3), differences in root traits among habitats were tested at the individual tree level with an ANCOVA (n = 1,222 trees); because the PCA was carried out at the species level across habitats, we did not compare PC scores between habitats. Root trait data across individuals were compared between habitats, families and their interactions as main effects, as well as within families, using “site” as a blocking variable with an ANCOVA, followed by Tukey’s test (Tukey, 1949; in “stats” package) when ANCOVA results showed a significant main effect (α = 0.05). Overall root trait variances were compared between habitats with “site” as blocking variable with an ANCOVA across trait residuals (i.e., the absolute differences between individual trait values and trait medians per habitat), followed by Tukey’s test for pairwise comparisons between habitats when F values were significant (α = 0.05).
Finally, following H2 and H3, we compared the relative contributions of habitat and different taxonomic levels (superorder, family, genus and species) to the variability in the root traits. To this end, we applied a linear mixed model [with the lmer function in the “lme4” package (Bates et al., 2015)] across all 1,222 trees with a given root trait as dependent variable and only a nested random intercept (“species” nested in “genus” nested in “family” nested in “superorder” nested in “habitat”). The overall variation was partitioned by calculating the percentage of variance explained by each random factor relative to the total variance explained by the model.
Results
Overall tropical root trait variation and correlations
Across all 1,222 trees, root diameter ranged from 0.17 to 1.33 mm (mean = 0.53 mm); root tissue density varied between 0.11 and 0.90 g cm–3 (mean = 0.40 g cm–3); SRL varied between 1.2 and 135.1 m g–1 (mean = 16.8 m g–1); SRTA ranged between 0.1 and 41.1 tips g–1 (mean = 3.7 tips g–1); and branching intensity ranged from 0.4 to 6.5 tips cm–1 (mean = 1.9 tips cm–1). At the species level, root diameter ranged from 0.20 to 1.23 mm (mean = 0.52 mm); root tissue density varied between 0.12 and 0.75 g cm–3 (mean = 0.40 g cm–3); SRL varied between 3.0 and 73.7 m g–1 (mean = 16.7 m g–1); SRTA ranged between 0.2 and 21.2 tips g–1 (mean = 3.4 tips g–1); and branching intensity ranged from 0.5 to 4.4 tips cm–1 (mean = 1.8 tips cm–1) (Supplementary Table 2). These values fall into the range of values reported for tropical forests globally (Freschet et al., 2017) and in the region (Lugli et al., 2020; Valverde-Barrantes et al., 2021). Across families, Myrtaceae species had on average the thinnest roots, and Lauraceae the thickest (Figure 1). Mean root tissue density was lowest for Malvaceae and highest for Chrysobalanaceae. Myrtaceae had the highest mean SRTA and branching intensity. Mean SRL was lowest for Lecythidaceae species and highest for Rubiaceae. Species from the Lauraceae family had on average the fewest root tips per root mass and length.
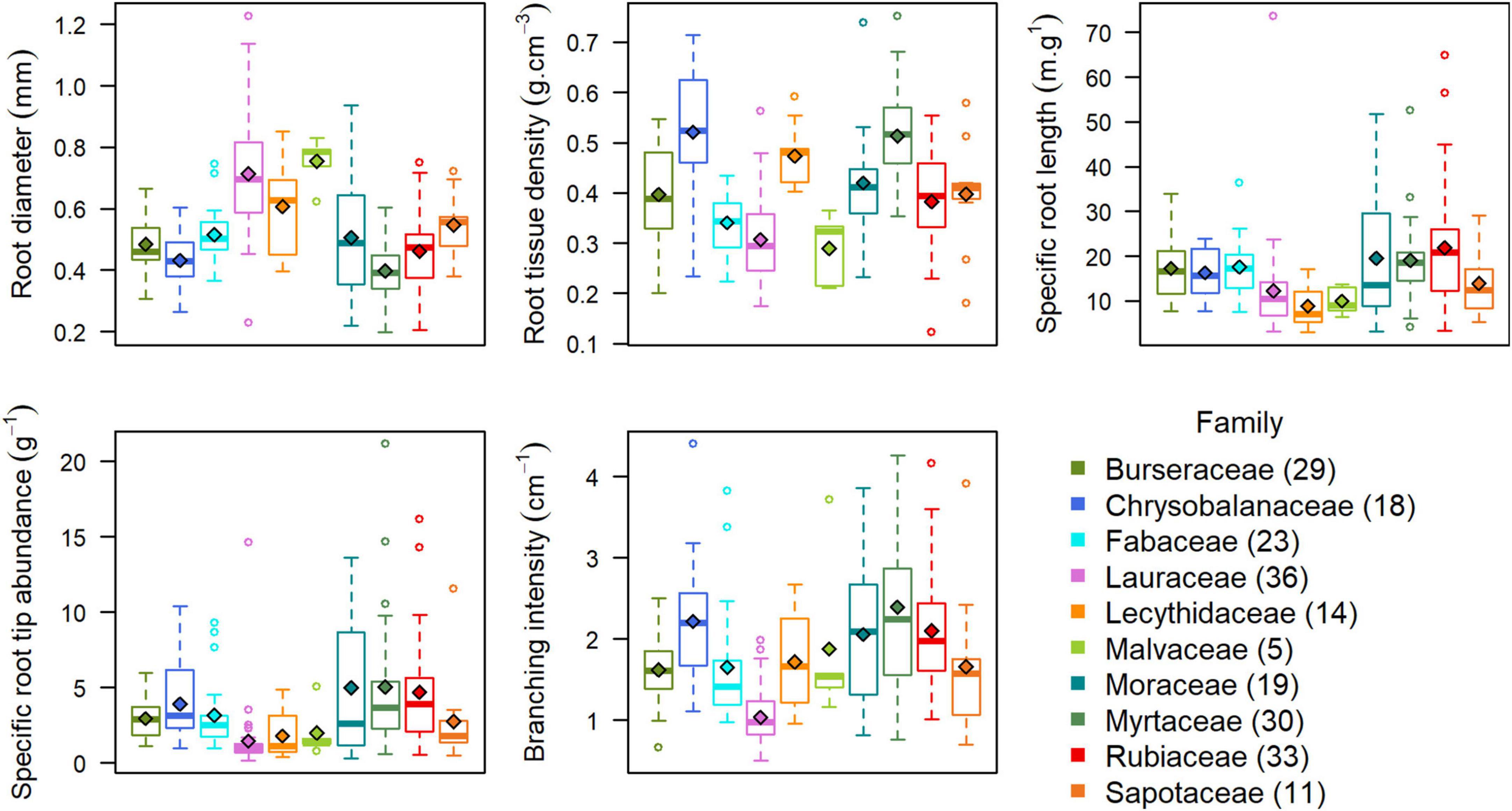
Figure 1. Trait data distributions per family. Trait data were first averaged per species across the three habitats, so that each species is equally represented at the family level. Horizontal bars in each box represent the family median, diamonds represent the family mean. The legend shows which boxplots corresponds to which family and the number of species per family between parentheses.
Across both the individual trees (n = 1,222 trees) and species’ means (n = 218 species), coefficients of variation were highest for SRTA and lowest for root tissue density (Supplementary Table 3). Pairs of root traits were strongly correlated across the 218 species−only the relationship between SRTA and root tissue density was not significant (Supplementary Table 4). Specifically, root diameter was negatively correlated with root tissue density, SRL, SRTA and branching intensity. Root tissue density correlated negatively with SRL but positively with branching intensity. Specific root length, SRTA and branching intensity were all positively correlated.
Multivariate relationships across species’ mean root traits
Multivariate analyses across the 218 tropical species identified two significant dimensions along which the five root traits covaried (parallel analyses: n iterations = 150; adjusted eigenvalues: PC1: 3.07; PC2: 1.20). The first principal component (PC1) explained 65% of the total variation among the five root traits and was mostly associated with root diameter and SRTA loading negatively and positively, respectively (Figure 2 and Supplementary Table 4). Specific root length and root branching intensity were also positively related to PC1, but less strongly than SRTA. In other words, species with relatively high scores on PC1 had more root tips per unit root length but especially per unit root mass, higher SRL, and thinner roots. PC2 explained an additional 26% of the total root trait variance and was predominantly related to interspecific variation in root tissue density, and, to a lesser extent SRL, so that species with higher scores on root PC2 have lower root tissue density and moderately higher SRL (Figure 2 and Supplementary Table 4).
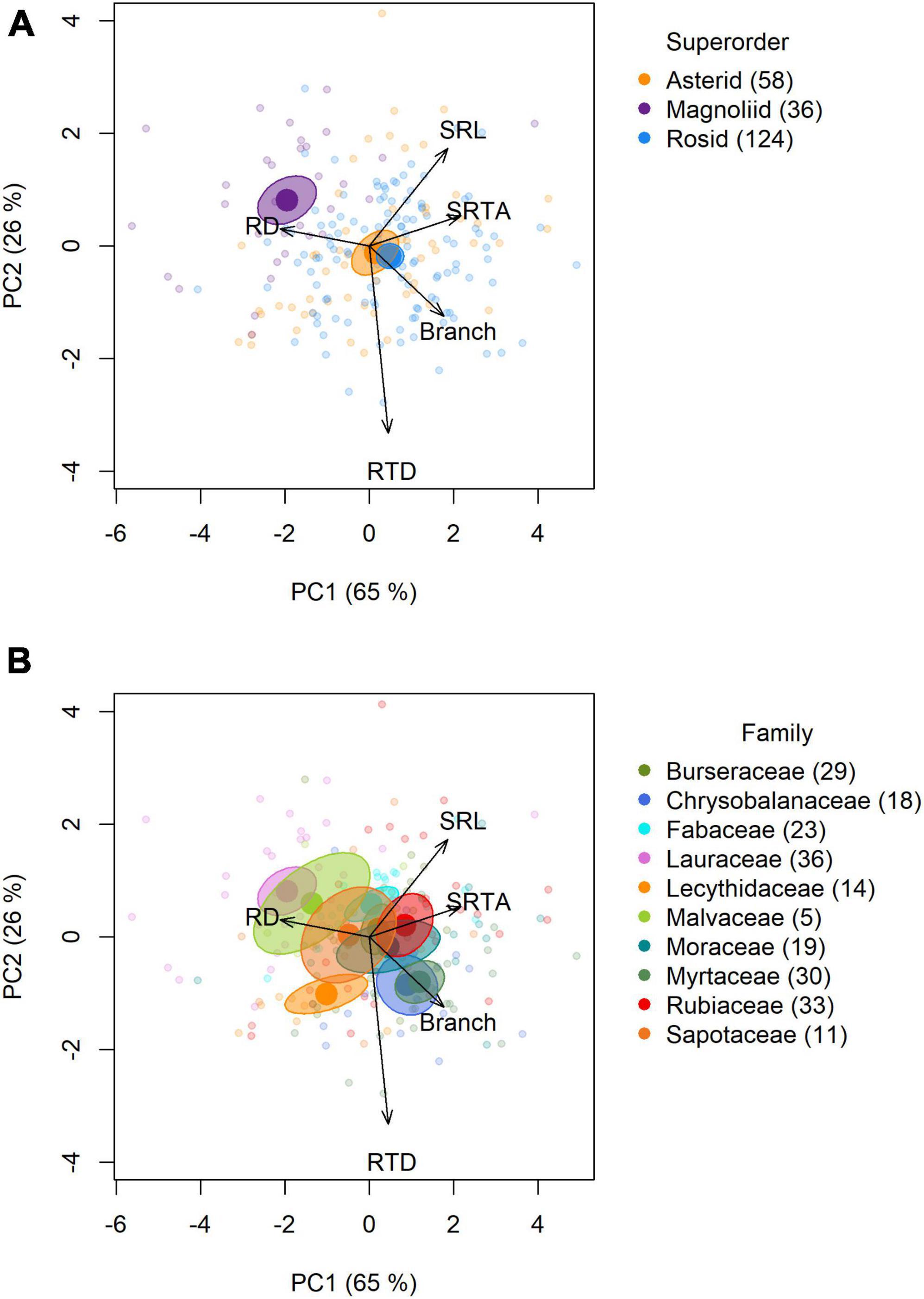
Figure 2. Species’ scores on PC1 and PC2 and associated root trait loadings (vectors). Each data point (small, filled circles) represents a single species (total n = 218 species). Large, filled circles represent the centroid (i.e., the multivariate mean of the five root traits) of each (A) superorder or (B) family, and ellipsoids represent corresponding 95% confidence intervals. SRL, specific root length (root length per unit root dry mass); SRTA, specific root tip abundance (n of root tips per unit root dry mass); Branch, root branching intensity (n root tips per unit root length); RTD, root tissue density (root dry mass per root volume); RD, root diameter.
Ranges along PC1 (i.e., the difference between lower and upper ends of the 95% confidence intervals for PC1 across all species within each family) were lowest for Burseraceae and highest for Malvaceae (Table 2). Ranges along PC2 were lowest for Fabaceae and highest for Malvaceae. Overall, the average range along root PC1 was significantly larger than the average range along PC2 (Wilcoxon rank sum test: V = 54, n = 10, p = 0.015). Family-level differences were not associated with superorders: mean ranges along PC1 or PC2 between the Asterids and Rosids did not differ significantly (Student’s t-test; PC1 ranges: t = 0.20, n = 9 families, p = 0.85; PC2 ranges: t = 0.25, n = 9 families, p = 0.82; Table 2). These families were not compared with Magnoliids as we only sampled one magnoliid family.
Furthermore, there was a significant positive relationship between the ranges along PC1 and PC2 (Pearson r = 0.81, n = 10, p = 0.004). All families but Burseraceae had a range ratio > 1, indicating that within families, interspecific variability was larger along PC1 than PC2. Across the ten families, the mean range ratio (i.e., the range along PC1 relative to the range along PC2) was significantly larger than 1 (Wilcoxon rank sum test: V = 54, n = 10, p = 0.004), i.e., species were overall more spread along PC1 than PC2.
Phylogenetic differences in tropical root trait variation
Overall, species of the three different superorders occupied distinct positions within the multivariate root traits space (Figure 2A and Supplementary Table 5). Pairwise comparisons showed that magnoliid centroids differed significantly from rosid and asterid centroids, with magnoliid species being associated with high root diameter, low SRL, low branching and low root tissue density. Asterid and rosid centroids did not differ significantly from each other, and were both located near the origin.
Root trait centroids also differed significantly across families (Supplementary Table 5). We used pairwise comparisons to test the centroids between all 45 pairs of families. Of these 45 pairs, 18 included families from the same superorder, and 27 pairs included families from different superorders; and 30 pairs showed significant, non-overlapping centroids (Supplementary Table 5). Seven pairs of families from the same superorder had overlapping centroids: e.g., Fabaceae and Burseraceae centroids (both Rosids) did not differ significantly and were both positioned close to the origin (Figure 2B). The other 11 pairs of families from the same superorder had significantly different centroids: e.g., both belonging to the Asterids, Rubiaceae roots were characterized by high SRL, high SRTA and low root diameter, while Lecythidaceae roots were comparatively thick, dense roots with few root tips and low SRL. Comparing families from different superorders, 19 out of 27 pairs differed significantly in their root trait centroids: e.g., roots of Chrysobalanaceae (a rosid) species were thin and highly branched, in contrast to Lauraceae (magnoliid) roots that were thick and poorly branched. In turn, eight pairs of families from different superorders did not differ in their centroids: e.g., Lauraceae (a magnoliid family) and Malvaceae (a rosid family) both had similarly thick, poorly branched roots.
Root trait variation across habitats and families
Between habitats, all soil properties that we measured differed significantly (Table 3 and Supplementary Table 6). White-sands were significantly higher in sand, and lower in silt and clay concentrations and lower in total N concentrations, organic C, organic matter and K and Mg concentrations than both the TF and SF habitats that did not differ from each other in these attributes. In turn, SF soils had significantly higher concentrations of available P than the WS and SF habitats. White-sand soils were lower in soil pH than TF, while SF habitats had intermediate soil pH that did not differ significantly from the other two habitats. Seasonally flooded habitats had lower soil C/N ratios, and higher Ca and Mg concentrations than the WS habitats, and TF habitats had intermediate values.

Table 3. Soil properties averaged across plot per habitat across the three study sites (SEM in parentheses).
Across all 1,222 trees, root traits were significantly different between families and their interactions with habitats (this interaction effect was near-significant for SRL: P = 0.06; Figure 3 and Supplementary Table 7)−in other words, the relationships between habitats and root trait expressions differed among families. Overall, root diameter was lowest on SF and significantly higher on both TF and WS. Root tissue density was highest on TF, and lowest on WS and SF. Specific root tip abundance was overall higher on SF than on both TF and WS. Specific root length differed across all three habitats and was highest on SF and lowest on TF. Branching intensity was on average higher on SF than on TF and WS and did not differ significantly between the latter two habitats.
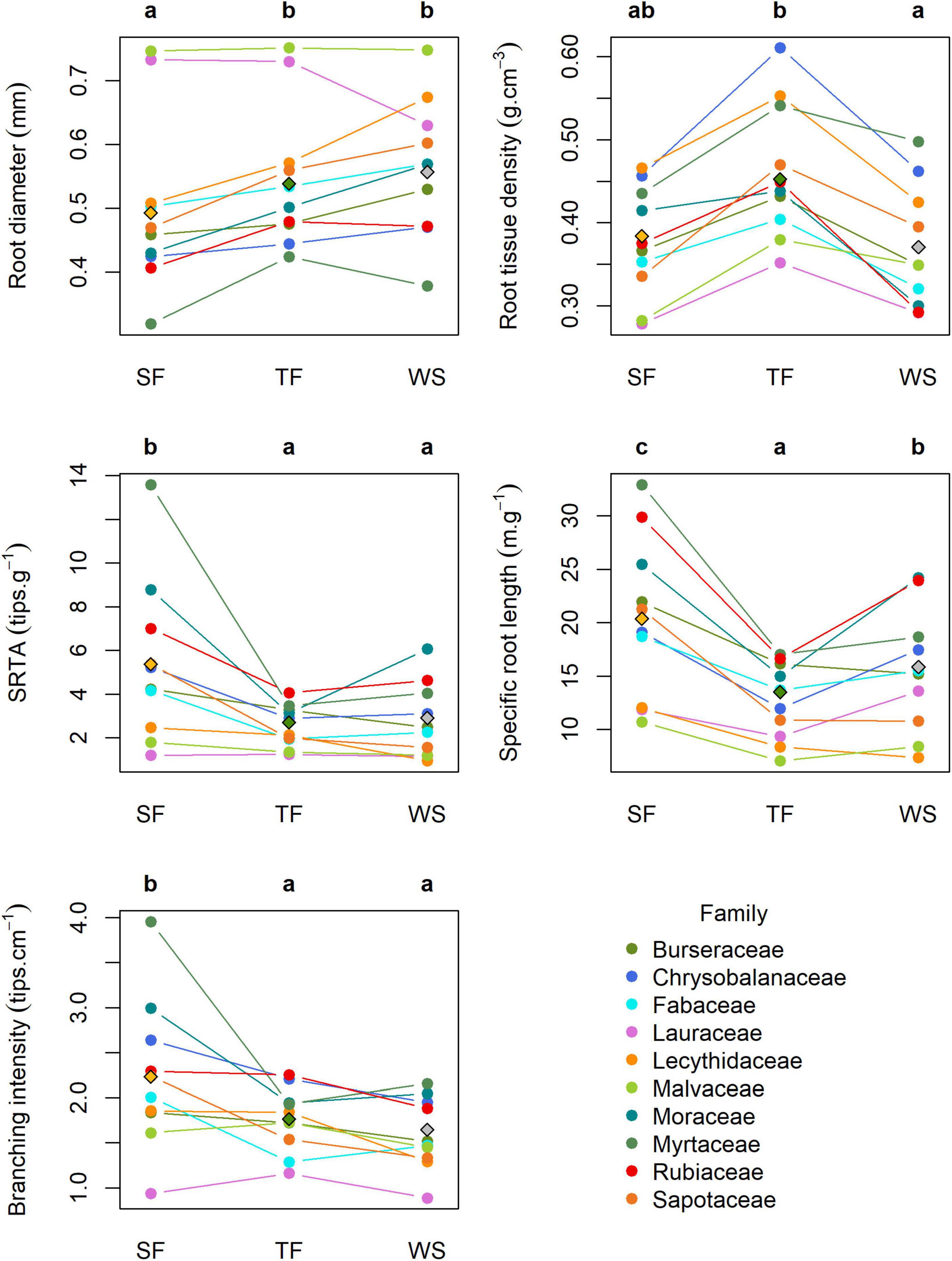
Figure 3. Root trait means across habitats. Diamonds represent the overall means across all 1,222 trees per habitat. Colored circles represent family means per habitat across all trees of a family, with colored lines connecting data points from the same family, and not necessarily indicating significant trait differences between habitats within families. Different letters indicate significant differences between overall habitat means (i.e., diamonds; α = 0.05). Corresponding ANCOVA statistics are presented in Supplementary Tables 7, 8. SRTA, specific root tip abundance. Family trait means and SEM by habitat are presented in Supplementary Table 8.
Within families, root trait patterns across habitats were largely, but not always, consistent across families (Figure 3 and Supplementary Table 8). Specifically, trees of all ten families had the highest root tissue density on TF habitats and the lowest tissue density on WS and/or SF, with the exception of Malvaceae, Myrtaceae and Sapotaceae that produce similarly dense roots on TF and WS. Branching intensity and SRTA differed significantly between habitats within eight families, and was generally highest on SF and lowest on WS and or TF. Exceptions included Lauraceae trees that had higher branching intensity on TF than on SF and WS but similar SRTA between habitats; Rubiaceae trees that had lowest branching intensity on WS but this difference was not significant from the other habitats; and Malvaceae trees that showed no significant difference in either branching intensity or SRTA. Eight families (all but Lauraceae and Malvaceae) showed significant differences in SRL with highest and lowest SRL on SF and TF, respectively. Finally, root diameter was highest on WS and lowest on SF for six families, and did not differ between habitats for Chrysobalanaceae, Fabaceae, Lauraceae and Malvaceae.
Variances in SRTA, SRL, and branching intensity were significantly higher on SF habitats than on both TF and WS (Table 4 and Supplementary Table 9). Variances in root diameter and tissue density did not differ significantly between the three habitats.
Relative contributions of habitat and phylogeny to overall root trait variation
Phylogenetic levels and habitat contributed to different extents to root trait variation across all trees (Figure 4). Across all traits, 38–60% of the variance remained unexplained. Besides that, overall variability in root diameter and tissue density was most explained by trait differences between families (23–28%). For SRTA and SRL, genus was the main explanatory taxonomic level (16–19%). Variance in branching intensity was best explained by variation between species (28%). Both habitat and superorder played no, or only a marginal role in the overall variance in root traits (0–11%).
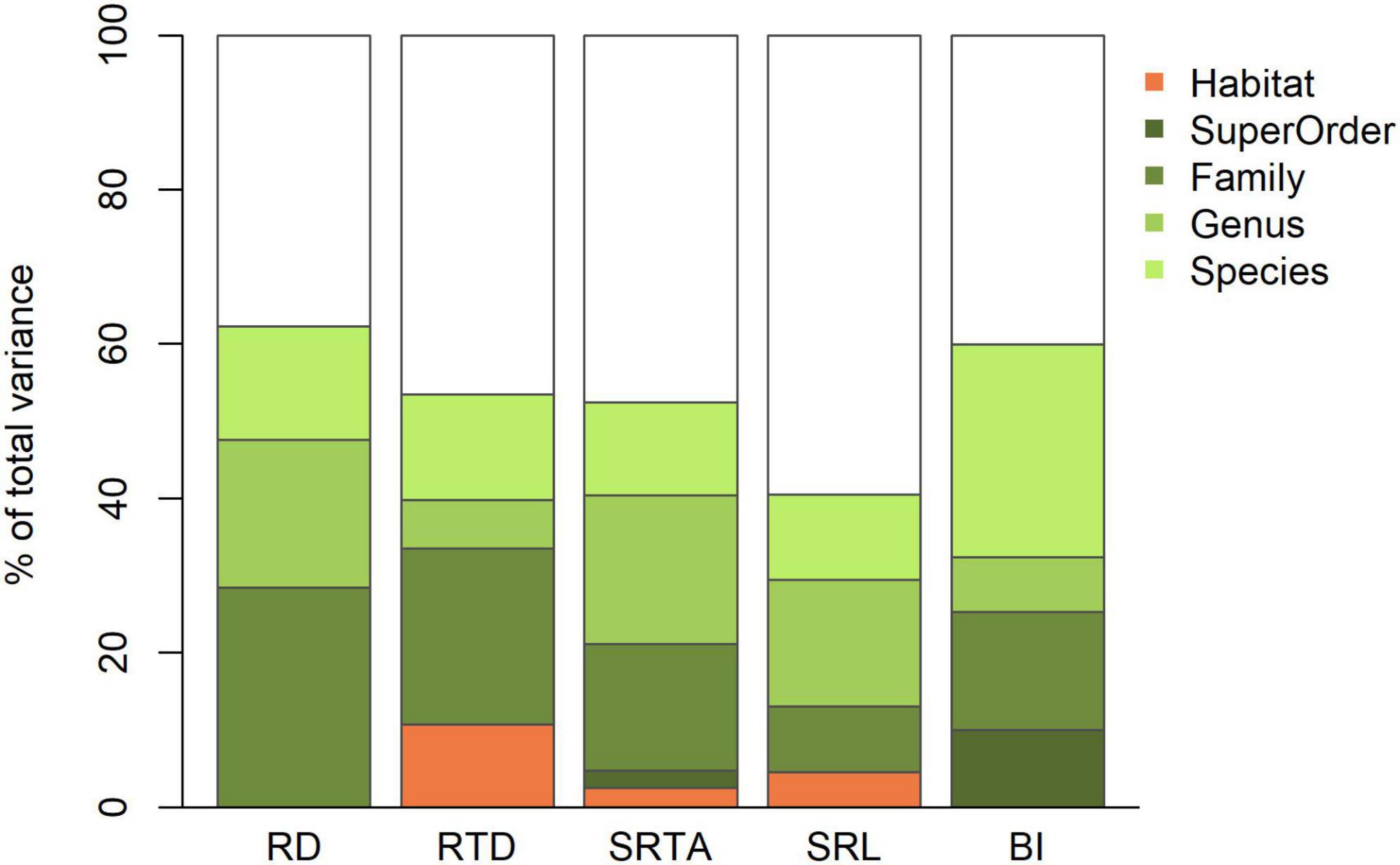
Figure 4. Percentage of total root trait variance across all 1,222 trees explained by habitat and taxonomic levels. RD, root diameter; RTD, root tissue density; SRL, specific root length; SRTA, specific root tip abundance; BI, branching intensity.
Discussion
Root trait dimensions in a tropical forest
The root economics space (RES) organizes species from around the globe (although predominantly temperate regions) along a belowground collaboration axis (reflecting a tradeoff between species with thick, highly mycorrhized roots, and species with high-SRL roots that are less reliant on mycorrhizal fungi for resource uptake), and an independent conservation axis (associated with a tradeoff between root tissue density prolonging root lifespan, and root N concentration, assumingly related to nutrient acquisition) (Bergmann et al., 2020). In line with our first hypothesis (H1a), two axes described root trait variation across 218 tropical species from the Brazilian western-central Amazon. Here, root PC1 reflects a negative relationship between root diameter and SRL, resembling the collaboration axis in the global RES, while a second, independent root PC2 separates species based on their root tissue density. While the root PC2 that we observed resembles the belowground conservation axis due to its close association with root tissue density (although we did not measure root N concentrations), further work is needed to clarify the belowground traits that reflect root resource conservation, as argued below.
While the positive loading of SRL (and negative loading of root diameter) on PC1 would support the existence of a tropical collaboration axis, SRL loaded almost equally strong on PC2, reflecting a negative relationship with root tissue density (as also observed in pairwise correlations here and by Bergmann et al. (2020), but not in the multivariate global RES). This negative relationship can be explained mathematically, as a high root tissue density implies more root mass per unit root volume and generally decreasing the root mass per unit root length. It seems also partly driven by the presence of the Malvaceae and especially Lauraceae species (together accounting for nearly 20% of the total number of species) that have comparatively thick, low-SRL and low-density roots. Because AM (but not EcM) fungi select for a large cortex size for root colonization, and cortex tissue is less mass-dense than stele tissue (Kong et al., 2014), both the thick roots and low root tissue density of the Lauraceae and Malvaceae species may reflect their AM dependency. Since AM fungi predominantly take up soil P and have limited or no capacities to access soil N (Brundrett, 2002), this specific combination of thick roots with low tissue densities may prevail in tropical species that are most commonly AM (Soudzilovskaia et al., 2019), and generally limited by soil P rather than N (Vitousek, 2004).
Having such highly mycorrhized roots with low tissue density may seem disadvantageous from a cost−benefit perspective, because the symbiosis requires C from the host [although whether this constitutes a real cost to the tree is contested (Corrêa et al., 2012; Weemstra et al., 2022)] and low-density roots are assumed to be short lived across growth forms (Bergmann et al., 2020) and as demonstrated across grass species (Ryser, 1996). However, across tree species, evidence suggests that root lifespan is generally more closely related to root diameter (Gu et al., 2011; McCormack et al., 2012; Adams et al., 2013; Hansson et al., 2013; Weemstra et al., 2016) than to root tissue density (Withington et al., 2006; McCormack et al., 2012), indicating that variation in root tissue density alone might not tell the full story on how trees conserve root resources. Nutrient returns on C investments in the symbiosis may still be favorable for thick-rooted species, and thick roots promoting both arbuscular mycorrhizal colonization and long lifespans may simultaneously contribute to resource uptake and conservation (Kong et al., 2016, 2017; Weemstra et al., 2021b). The relationships between root traits and resource acquisition and conservation strategies−and therefore the RES dimensions−may thus differ between tropical and temperate forests: tropical forests are dominated by AM symbiosis that rely on thick roots with a large cortex and are equipped for P uptake, while temperate forests have more EcM associations that do not require thick roots for colonization and allow both P and (mineral and organic) N uptake (Soudzilovskaia et al., 2019). Our results further suggest that deducing belowground resource uptake strategies (e.g., conservation) from root traits (e.g., tissue density) in a RES requires more physiological information (on e.g., root lifespan) about the mechanistic links between tree root form and function.
Consistent with H1b, root branching traits were associated with root PC1, and negatively related to root diameter. The degree of root branching reflects a species’ capacity to proliferate in resource-rich patches (Hodge, 2004), and can thus be interpreted as an important, different element of the do-it-yourself strategy (additional to a high SRL, and alternative to thick roots with high AM fungal dependency that have their own mechanisms to acquire soil resources) in any biome (e.g., temperate forests; Liese et al., 2017). It may be particularly relevant in old, weathered tropical soil that are generally limited by soil P: due to its low mobility, P depletion zones around the roots are much smaller than for more mobile nitrate, so that highly branched roots would compete less for P than nitrate (Postma et al., 2014), and would be especially beneficial for tropical trees. In order to formally compare the importance of root branching globally, more data on root architecture are, however, required−the global RES (Bergmann et al., 2020) currently does not include information on root branching due to limited data availability across biomes, but our work identifies branching properties as important candidate traits to test and include in a tropical, P-limited RES.
Tropical families are more flexible in their belowground collaboration than conservation
Also consistent with H1a, the tropical families studied here were overall more flexible along root PC1, which may reflect the belowground collaboration axis, than along root PC2, associated with root tissue density. For example, within the Fabaceae, Lecythidaceae, and Moraceae families, interspecific variability along the collaboration axis was more than twice as large as along the conservation axis. Possibly, biophysical properties are a greater constraint to variation in root tissue density than in root diameter. While root tissue density may not be the sole predictor of tree root lifespan, it may play a role in root protection. Low root tissue density may thus provide insufficient structural and/or chemical protection (Xia et al., 2021) against soilborne pathogens and dense soils (Bengough et al., 2006), whereas dense root tissue may incur high costs of biosynthesis and low rates of physiological activity (Makita et al., 2012). By comparison, biophysical constraints to root diameter may be weaker, leading to larger adaptive ranges in root diameter and SRL than in tissue density. On the one end, thin, high-SRL roots are efficient in soil exploration and exploitation (Freschet et al., 2021b), and can still be highly loaded with secondary compounds for chemical protection (Xia et al., 2021). On the other end, thick roots with a large cortex can be very acquisitive when highly colonized by AM fungi, and may still be cost-efficient as they may be long-lived (although root lifespan remains poorly quantified in tropical trees).
Flexibility along the two root PCs seemed to differ between families, although it should be noted that we could not formally test this, and that not all families were equally taxonomically represented. Nonetheless, based on visual inspection, Burseraceae, Fabaceae, and Myrtaceae showed less within-family variation along the two root trait dimensions than families like Malvaceae, Sapotaceae, and Moraceae. These differences across families were not associated with superorders, i.e., mean ranges along PC1 or PC2 between the Asterids and Rosids did not differ significantly. Range differences between families may be related to the uneven taxonomic representation across families within our dataset, as some families were only represented by a single, dominant genus on our study sites (e.g., Inga in the Fabaceae family). Because genus identity was an important driver of root diameter, SRTA and SRL (Figure 4), this may explain why variability along especially root PC1 was constrained within these families, and demonstrates that more species and genera need to be measured to capture the full diversity of root strategies in tropical forests. At the same time, genus representation within families is not the only explanation for the extent to which root traits diverge. For example, the Malvaceae included only five species from three genera, but displayed the greatest trait variability along PC1 and PC2 of all families. Together with the observation that families that were more variable along one PC were also more variable along the other, these results suggest different levels of selection on root trait variability that may be−at least partly−phylogenetically determined at the family, but not superorder, level.
Unlike in temperate forests with abiotic constraints to root activity and lifespan (e.g., low temperatures), the drivers of belowground functional diversity in the tropics may lie in the soil biotic realm. Soilborne pathogen loads are for example far higher in tropical than temperate soils (Delgado-Baquerizo et al., 2020), non-mycorrhizal species relatively few (Soudzilovskaia et al., 2019), and N-fixing trees associating with Rhizobia are abundant (Houlton et al., 2008). An important research need pertains to the observation that biotic defense functions mostly established for leaf traits (e.g., Coley and Barone, 1996) cannot be extrapolated to roots because of their simultaneous symbiotic and antagonistic interactions with soil biota: high secondary compounds with antifungal properties in roots not only protect against plant pathogens but also inhibit mycorrhization (Xia et al., 2021). As this relationship between root protection and (mycorrhizal) resource uptake links the collaboration and conservation axes, it deserves greater attention in future (tropical and global) RES studies.
Root trait (co-)variation across tropical families do not follow temperate phylogenetic patterns
Across the 218 tropical species studied here, superorders differed in their root traits. As hypothesized (H2a), magnoliid species had thick, poorly branched roots of low mass-density compared to rosid and asterid species, but unlike expected, Asterids and Rosids showed large overlap in their root trait expressions. Similarly, Valverde-Barrantes et al. (2015) found distinct root morphologies for magnoliid species, and overlapping traits for Rosids and Asterids across 34 temperate tree species. Pierick et al. (2022) also found overall higher root diameter for magnoliid than rosid and asterid species in the tropics, but this difference was mainly driven by the thick roots of Lauraceae and Myristicaceae species, while other magnoliid families in their study (Annonaceae, Chloranthaceae, Piperaceae) did not have markedly thicker roots. It should be noted that our study included only one magnoliid family (Lauraceae), so collecting additional root data on other important magnoliid families in the tropics (e.g., Annonaceae, Myristicaceae, and Piperaceae) is necessary to verify phylogenetic patterns in tropical root trait (co)variation.
Our findings are consistent with the idea that more basal superorders, like Magnoliids, primarily rely on AM symbiosis for resource uptake (Baylis, 1975), facilitated by having thick roots with a large cortex to promote AM colonization (Brundrett, 2002). It is hypothesized that as more recently derived angiosperms spread to higher latitudes during the Cretaceous, they altered their root traits to adapt to colder and drier climates (Baylis, 1975; Comas et al., 2012; Ma et al., 2018): lower water and nutrient availability would select against thick, expensive AM roots with mostly access to soil P, and favor more explorative and exploitative root systems with thin, high-SRL roots, and promote the formation of EcM associations to access P and N bound in organic matter (Comas et al., 2012). In the tropics, where environmental variables do not pose such strong constraints to root diameter, multiple root morphologies can coexist: roots are on average thicker, but, in line with our results, thin roots with high soil exploration and exploitation capacities are also common (Gu et al., 2014; Freschet et al., 2017; Ma et al., 2018). Our work, however, also highlights the large belowground trait variability across families within superorders and substantial root trait overlap across superorders, supporting hypothesis 2b. For example, within the Asterids, Rubiaceae species had on average significantly thinner, more branched roots and higher SRL than the Lecythidaceae and Sapotaceae species; Myrtaceae and Chrysobalanaceae roots were denser, thinner and more branched than Malvaceae and Fabaceae (all Rosids); and Moraceae species showed a large range of morphological adaptations ranging from “magnoliid”-like roots to very thin root systems. In other words, phylogenetic conservatism may act on root trait variation across superorders (but not between Asterids and Rosids) but not across families within superorders, so that species’ root trait expressions from hyperdiverse tropical families cannot be inferred from shared ancestry.
Whilst our analyses do not allow a direct comparison between a tropical and global RES (as we included different traits), globally, tree families have been found to be more diverse in their root strategies in the tropics than in temperate or boreal systems (Ma et al., 2018). Part of this diversity is explained by the evolutionary trajectory of species: tropical forests host both basal and more-derived angiosperm species with−as we showed here−distinct root morphology and branching leading to greater root trait diversity, whereas higher latitudes are relatively sparsely covered by species from more ancient superorders reducing belowground trait variability (Ma et al., 2018). This study, however, suggests that this belowground diversity in tropical forests may not only be higher due to a larger collection of coexisting species from different superorders, but also result from large trait diversification within tropical families. Unlike the general phylogenetic hypothesis that predicts that root traits are strongly structured at the superorder level, our results indicate that this diversification may be especially strong at lower taxonomic levels (genus, species; Figure 4).
Consistent tropical root trait patterns across habitats
Environmental−especially edaphic−variables further drive belowground trait variation across trees (e.g., Freschet et al., 2013; Weemstra et al., 2017; Lugli et al., 2021; Pierick et al., 2022). To test these root trait−environment relationships, we selected three habitat types [WS, SF, and TF habitats] with different soil properties (Baraloto et al., 2011) that have known effects on root traits. Prior to comparing root trait expressions and variability between these habitats, we verified habitat descriptions from previous studies with our own soil data. We found that across study sites, the WS habitats were characterized by adverse soil conditions for tree growth (e.g., sandy, acidic soils with low nutrient availability) compared to TF and SF habitats. The latter two habitats differed mostly in soil available P, which was 3.5 times higher on SF than on TF and WS.
Consistent with our hypothesis (H3a), trees on WS had thick, low-SRL and poorly branched roots, but−unlike expected−also the least dense roots. Together, these traits may reflect a relatively large cortex size [which is associated with both high root diameter and low tissue density (Kong et al., 2014)], allowing higher AM colonization rates (Brundrett, 2002), thus circumventing the need for highly branched, proliferous roots to exploit local nutrient hotspots. In contrast, roots in SF habitats were thinner, had relatively low tissue density, a higher SRL and were more branched, as expected: on these more fertile soils, tree roots themselves may be preferred over investing C in fungal symbionts for soil resource acquisition−although the true C costs of mycorrhizal symbiosis to the host tree are yet to be established (Corrêa et al., 2012; Weemstra et al., 2022).
Between TF and SF habitats, all root traits differed significantly. Their soil properties were largely similar, except for soil P, which was more than three times lower on TF than on SF, suggesting that this is a major driver of root trait variation in Neotropical forests (Lugli et al., 2020, 2021). In both SF and WS, with low soil P, roots were thicker and less branched, and may thus rely more heavily on AM fungi that promote P uptake, as argued above. However, root tissue density was higher on TF than on WS (and SF)−both overall and within most families−contrasting our argument above that AM colonization requires low-density roots, and we can only speculate on potential explanations. Firstly, soilborne pathogens may be more abundant on TF due to relatively high nutrient concentrations and lack of seasonal flooding, so that trees allocate more secondary compounds to their root cortex for chemical protections, leading to denser root tissue (Xia et al., 2021). This strategy may come at the expense of AM associations due the antimicrobial properties of these secondary metabolites (Xia et al., 2021). These interactions between root traits, soil symbionts and antagonists therefore warrant further study, especially in the tropics with high soilborne pathogen loads and AM dependence. Secondly, trees can cope with soil P limitations through several belowground trait strategies beyond root morphology and architecture (Lugli et al., 2020; Dallstream et al., 2022), potentially leading to distinct root properties between habitats with similar P concentrations. Another possibility is that unmeasured abiotic properties interfere with root trait expressions, e.g., higher clay contents (Table 3) or larger water deficits in TF may select for denser roots (Bengough et al., 2006). Root trait variations are the result of the complex interplay between multiple biotic and abiotic variables. Unraveling the mechanisms between roots and their environment in order to explain functional and species diversity from a belowground perspective therefore requires in-depth studies into these different factors and potentially a new paradigm for tropical forest functioning.
Earlier work showed that environmental filtering of plant species is stronger in adverse environments: harsh habitats permit only a restricted and thus more similar set of plant strategies, while favorable environments allow a larger diversity of trait expressions (Weiher and Keddy, 1995; Cornwell et al., 2006; Swenson et al., 2012). This mechanism has been identified as a major driver of community assembly across vegetation systems including (Neo)tropical forests (Kraft and Ackerly, 2010; Ding et al., 2019). Such habitat filtering processes may explain why variability in root branching traits and SRL (expressed as trait coefficients of variation) was higher on the more fertile SF habitats than on low-nutrient WS soils. Because soil properties related to soil fertility strongly overlapped between SF and TF, we would further expect similar degrees of environmental filtering (i.e., similar root trait variability) on these two habitats; however, in TF habitats, the variation in SRTA, root branching intensity and SRL was significantly lower than on SF habitats and similar to that on WS. Since soil available P was the only soil variable that was significantly lower on TF than on SF habitats, it may not only be a main constraint to root trait expressions (as previously discussed), but also to root trait variability, consistent with previous work on TF soils in the same region (Lugli et al., 2020, 2021).
Stronger root trait convergence in TF and WS habitats may reflect both intraspecific trait variation (habitat conditions causing conspecific trees to adjust their traits in similar directions), and species turnover (habitat conditions filtering species based on their traits). We lacked sufficient replicate trees across the three habitats (only two out of 218 species had more than five individuals on each of the three habitats) to quantify the contribution of intraspecific root trait variation, but we did observe larger species overlap between TF and WS habitats (28 species) than between TF and SF habitats (14 species). Our results point at soil P being a main driver of species distributions in these central-western Amazonian forests, potentially due to habitat filtering of taxa that depend on AM symbiosis and that have the root traits that facilitate this. At the same time, we acknowledge that there are many different belowground traits (e.g., related to root exudation rates) through which soil P in its many forms can be acquired (Lugli et al., 2021; Dallstream et al., 2022). Since P is a potentially key determinant of root and by extension tree functioning, these additional traits require more attention in future belowground studies in the tropics.
Finally, root trait differences between habitats observed across families were largely consistent with those within families. While the relationships between root traits and habitat differed overall between families, these differences were more quantitative (i.e., traits differing to different degrees) than qualitative (i.e., traits shifting in different directions across habitats). Among the ten families, Lauraceae and Malvaceae had the least variable root traits: their SRTA, SRL, root diameter (Lauraceae), and branching intensity (Malvaceae) did not differ significantly between habitats. This low variation may be attributed to the lower plasticity of thick roots (Liu et al., 2015), potentially because proliferation in nutrient patches is outsourced to mycorrhizal fungi (Eissenstat et al., 2015). The largely consistent patterns in root traits across habitats contrast recent work that shows highly idiosyncratic root trait responses to edaphic variation (Weemstra et al., 2021a; Spitzer et al., 2022). These trait differences were, however, observed at the intraspecific level, whereas in our study belowground differences between habitats included both inter- and intraspecific differences in root traits. Our study design did not permit determining the extent to which intraspecific belowground variation drives community root trait patterns, but it may explain the small contribution of habitat type to root trait diversity, and the large share of unexplained variability in root traits (35–60% depending on the trait). Quantifying intraspecific variation in root traits would therefore be a next step into unraveling the drivers belowground diversity in tropical systems, into the diverse ways through which species respond belowground to their environment, or species are being replaced along edaphic gradients.
Outlook
Together, our results suggest that globally observed patterns in, and drivers of root trait (co-)variation−where tropical species are largely underrepresented−may not necessarily extend to tropical forests. Firstly, root traits have been found to be strongly phylogenetically conserved across temperate lineages, but shared ancestry may be a weaker driver in the tropics. We confirmed earlier work demonstrating thicker and less branched roots for more basal clades, that would be more dependent on mycorrhizal symbiosis for resources, compared to more recently derived superorders. However, the large divergence and overlap of root traits across families of the same and of different superorders, respectively, suggest that tropical tree lineages strongly diversified belowground at taxonomic scales below the superorder level. Such large belowground functional diversity may explain why a large number of species of relatively few families can dominate a wide variety of habitats, as well as the tremendous biodiversity of tropical forests.
Secondly, root trait variation may be determined by different (a)biotic constraints across biomes. Our tropical study species were largely organized along two root trait axes similar to the collaboration and conservation axes identified in the global RES (Bergmann et al., 2020). However, different traits may load differently on these axes due to the importance of root branching for the uptake of P which is typically more limiting in tropical than temperate systems; because AM symbiosis that dominates in tropical forests may select for different (combinations of) root traits; and because tropical trees may have to balance complicated tradeoffs between symbiotic and antagonist soil biota as they generally face higher soilborne pathogen pressures and are more dependent on AM fungi. The importance of accounting for these different environmental drivers of belowground diversity is further supported by our habitat comparisons that highlight the importance of soil P availability in driving root trait expressions and variability, and possibly filtering species based on their belowground traits. Furthermore, we speculate that the relationships between especially root tissue density and resource conservation proposed by the global RES is not as straightforward for trees; this calls for more mechanistic work to determine relationships between root form and function, and careful interpretation of the RES axes.
At the same time, our work did not compare a global or temperate and tropical RES, because we prioritized measuring different traits, and this would be a focus area for future research. To identify and compare the dimensions of belowground functional diversity between biomes, plant ecological research likely needs to include different belowground plant traits (e.g., related to the root microbiome including both symbionts and pathogens; root lifespan), functions (e.g., defense and conservation) and environmental properties (e.g., reflecting nutrient limitations but also biotic soil properties, like soilborne pathogen loads) than those commonly measured based on their assumed importance (Freschet et al., 2021b) in temperate systems. Quantifying and conceptualizing belowground trait variation across species and habitats in tropical forests and testing it against the global RES deserves further attention in ecology, given the importance of belowground traits for important forest [e.g., forest productivity, niche differentiation, species assembly, and resilience against (a)biotic stress] and ecosystem (e.g., C, water and nutrient cycling) processes.
Data availability statement
The original contributions presented in this study are included in the article/Supplementary material, further inquiries can be directed to the corresponding author.
Author contributions
MW analyzed data and wrote and revised the first draft of this manuscript. OV-B, CF, and CB designed this study and collected data. EO, EP, MV, AV, and JV collected data. All authors provided substantial comments to the manuscript, provided approval for publication of the content, and agreed to be accountable for all aspects of this work.
Funding
This research was supported by grants from the US National Science Foundation (DEB-0918240, DEB-0918878, and DEB-1549964), the US Department of Energy (DE-SC0004335), the Natural Sciences and Engineering Research Council of Canada (NSERC RGPIN-2018-04620), and the Agence Nationale de la Recherche (CEBA, ref. ANR-10- LABX25-01, and NEBEDIV, ref. ANR-13-BSV7-009). Funding was also provided by FAPEAM through the proposal “Collaborative training for the study of beta-diversity in tropical forests – TREEBEDIVFAPEAM 014/2013 – AIRD/GUYAMAZ II”. Permission to work in Brazil for the NEBEDIV project: “Authorization to work in Brazil, AEX-CNPq 01300.000800/2016-84”.
Conflict of interest
The authors declare that the research was conducted in the absence of any commercial or financial relationships that could be construed as a potential conflict of interest.
Publisher’s note
All claims expressed in this article are solely those of the authors and do not necessarily represent those of their affiliated organizations, or those of the publisher, the editors and the reviewers. Any product that may be evaluated in this article, or claim that may be made by its manufacturer, is not guaranteed or endorsed by the publisher.
Supplementary material
The Supplementary Material for this article can be found online at: https://www.frontiersin.org/articles/10.3389/ffgc.2023.1187127/full#supplementary-material
Footnotes
References
Adams, T. S., McCormack, M. L., and Eissenstat, D. M. (2013). Foraging strategies in trees of different root morphology: The role of root lifespan. Tree Physiol. 33, 940–948. doi: 10.1093/treephys/tpt067
Allié, E., Pélissier, R., Engel, J., Petronelli, P., Freycon, V., Deblauwe, V., et al. (2015). Pervasive local-scale tree-soil habitat association in a tropical forest community. PLoS One 10:e0141488. doi: 10.1371/journal.pone.0141488
Anderson, M. (ed.) (2017). “Permutational multivariate analysis of variance (PERMANOVA),” in Wiley statsref: Statistics reference online, (New York, NY: John Wiley & Sons), 1–15. doi: 10.1002/9781118445112.stat07841
Araújo, A. C., Nobre, A. D., Kruijt, B., Elbers, J. A., Dallarosa, R., Stefani, P., et al. (2002). Comparative measurements of carbon dioxide fluxes from two nearby towers in a central Amazonian rainforest: The manaus LBA site. J. Geophys. Res. Atmos. 107, 58–20. doi: 10.1029/2001JD000676
Baraloto, C., Jason, V., Julien, E., Pascal, P., Nállarett, D., Marcos, R., et al. (2021). Biogeographic history and habitat specialization shape floristic and phylogenetic composition across Amazonian forests. Ecol. Monogr. 91:e01473. doi: 10.1002/ecm.1473
Baraloto, C., Olivier, J., Timothy, C. E., Paine, K., Dexter, C., Cruaud, L., et al. (2012). Using functional traits and phylogenetic trees to examine the assembly of tropical tree communities: Assembly of tropical tree communities. J. Ecol. 100, 690–701. doi: 10.1111/j.1365-2745.2012.01966.x
Baraloto, C., Suzanne, R., Quentin, M., Lilian, B., Claire, F., Bruno, H., et al. (2011). Disentangling stand and environmental correlates of aboveground biomass in amazonian forests: Aboveground biomass in Amazonian forests. Glob. Change Biol. 17, 2677–2688. doi: 10.1111/j.1365-2486.2011.02432.x
Bates, D., Mächler, M., Bolker, B., and Walker, S. (2015). Fitting linear mixed-effects models using Lme4. J. Stat. Softw. 67, 1–48. doi: 10.18637/jss.v067.i01
Baylis, G. (1975). “The magnolioid mycorrhiza and mycotrophy in root systems derived from It,” in Endomycorrhizas, eds F. Sanders, B. Mosse, and P. Tinker (New York, NY: Academic Press), 373–389.
Bengough, A., Bransby, M., Hans, J., McKenna, S., Roberts, T., and Valentine, T. (2006). Root responses to soil physical conditions; growth dynamics from field to cell. J. Exp. Bot. 57, 437–447. doi: 10.1093/jxb/erj003
Bergmann, J., Weigelt, A., van der Plas, F., Laughlin, D., Kuyper, T., Guerrero-Ramirez, N., et al. (2020). The fungal collaboration gradient dominates the root economics space in plants. Sci. Adv. 6:eaba3756. doi: 10.1126/sciadv.aba3756
Brassard, B., Chen, H., Cavard, X., Laganière, J., Reich, P., Bergeron, Y., et al. (2013). Tree Species diversity increases fine root productivity through increased soil volume filling. J. Ecol. 101, 210–219. doi: 10.1111/1365-2745.12023
Brundrett, M. (2002). Coevolution of roots and mycorrhizas of land plants. New Phytol. 154, 275–304. doi: 10.1046/j.1469-8137.2002.00397.x
Carmona, C., Bueno, C., Toussaint, A., Träger, S., Díaz, S., Moora, M., et al. (2021). Fine-root traits in the global spectrum of plant form and function. Nature 597, 683–687. doi: 10.1038/s41586-021-03871-y
Chen, W., Hui, Z., David, M., and Dali, G. (2013). Variation of first-order root traits across climatic gradients and evolutionary trends in geological time: Root trait variation and evolution. Glob. Ecol. Biogeogr. 22, 846–856. doi: 10.1111/geb.12048
Coley, P. D., and Barone, J. A. (1996). Herbivory and plant defenses in tropical forests. Annu. Rev. Ecol. Syst. 27, 305–335. doi: 10.1146/annurev.ecolsys.27.1.305
Comas, L. H., Mueller, K. E., Taylor, L. L., Midford, P. E., Callahan, H. S., and Beerling, D. J. (2012). Evolutionary patterns and biogeochemical significance of angiosperm root traits. Int. J. Plant Sci. 173, 584–595. doi: 10.1086/665823
Comas, L., Callahan, H., and Midford, P. (2014). Patterns in root traits of woody species hosting arbuscular and ectomycorrhizas: Implications for the evolution of belowground strategies. Ecol. Evol. 4, 2979–2990. doi: 10.1002/ece3.1147
Cornwell, W., Schwilk, L., and Ackerly, D. (2006). A trait-based test for habitat filtering: Convex hull volume. Ecology 87, 1465–1471. doi: 10.1890/0012-9658(2006)87[1465:attfhf]2.0.co;2
Corrêa, A., Gurevitch, J., Martins-Loução, M. A., and Cruz, C. (2012). C allocation to the fungus is not a cost to the plant in ectomycorrhizae. Oikos 121, 449–463. doi: 10.1111/j.1600-0706.2011.19406.x
Cusack, D., Addo-Danso Shalom, D., Agee Elizabeth, A., Andersen Kelly, M., Marie, A., Batterman Sarah, A., et al. (2021). Tradeoffs and synergies in tropical forest root traits and dynamics for nutrient and water acquisition: Field and modeling advances. Front. For. Glob. Change 4:704469. doi: 10.3389/ffgc.2021.704469
Dallstream, C., Monique, W., and Fiona, M. (2022). A framework for fine-root trait syndromes: Syndrome coexistence may support phosphorus partitioning in tropical forests. Oikos 2022:e08908. doi: 10.1111/oik.08908
Delgado-Baquerizo, M., Guerra, C. A., Cano-Díaz, C., Egidi, E., Jun-Tao, W., Nico, E., et al. (2020). The proportion of soil-borne pathogens increases with warming at the global scale. Nat. Clim. Change 10, 550–554. doi: 10.1038/s41558-020-0759-3
Dick, C., and Pennington, R. T. (2019). History and geography of neotropical tree diversity. Annu. Rev. Ecol. Evol. Syst. 50, 279–301. doi: 10.1146/annurev-ecolsys-110617-062314
Ding, Y., Runguo, Z., Xinghui, L., Jihong, H., and Yue, X. (2019). The effect of environmental filtering on variation in functional diversity along a tropical elevational gradient. J. Veg. Sci. 30, 973–983. doi: 10.1111/jvs.12786
Dinno, A. (2018). Paran: Horn’s test of principal components/factors. Available online at: https://CRAN.R-project.org/package=paran (accessed October 11, 2022).
Eissenstat, D., Kucharski, J., Zadworny, M., Adams, T., and Koide, R. (2015). Linking root traits to nutrient foraging in arbuscular mycorrhizal trees in a temperate forest. New Phytol. 208, 114–124. doi: 10.1111/nph.13451
Fine, P. V. A., and Kembel, S. W. (2011). Phylogenetic community structure and phylogenetic turnover across space and edaphic gradients in western amazonian tree communities. Ecography 34, 552–65. doi: 10.1111/j.1600-0587.2010.06548.x
Fitter, A. H. (1987). An architectural approach to the comparative ecology of plant root systems. New Phytol. 106, 61–77.
Fontes, C., Dawson, T., Jardine, K., McDowell, N., Gimenez, B., Anderegg, L., et al. (2018). Dry and hot: The hydraulic consequences of a climate change-type drought for Amazonian trees. Philos. Trans. R. Soc. Lond. B Biol. Sci. 373:20180209. doi: 10.1098/rstb.2018.0209
Fortunel, C., Timothy Paine, C. E., Fine, P. V. A., Kraft, N. J. B., and Baraloto, C. (2014). Environmental factors predict community functional composition in Amazonian forests. J. Ecol. 102, 145–155. doi: 10.1111/1365-2745.12160
Freschet, G., Bellingham, P., Lyver, P., Bonner, K., and Wardle, D. (2013). Plasticity in above- and belowground resource acquisition traits in response to single and multiple environmental factors in three tree species. Ecol. Evol. 3, 1065–1078. doi: 10.1002/ece3.520
Freschet, G., Pagès, L., Iversen, C., Comas, L., Rewald, B., Roumet, C., et al. (2021a). A starting guide to root ecology: Strengthening ecological concepts and standardising root classification, sampling, processing and trait measurements. New Phytol. 232, 973–1122. doi: 10.1111/nph.17572
Freschet, G., Roumet, C., Comas, L., Weemstra, M., Bengough, A., Rewald, B., et al. (2021b). Root traits as drivers of plant and ecosystem functioning: Current understanding, pitfalls and future research needs. New Phytol. 232, 1123–1158. doi: 10.1111/nph.17072
Freschet, G., Valverde-Barrantes, O. J., Caroline, M., Joseph, M., McCormack, M. L., Cyrille, V., et al. (2017). Climate, soil and plant functional types as drivers of global fine-root trait variation. J. Ecol. 105, 1182–1196. doi: 10.1111/1365-2745.12769
Gentry, A. (1988). Changes in plant community diversity and floristic composition on environmental and geographical gradients. Ann. Mo. Bot. Gard. 75, 1–34. doi: 10.2307/2399464
Gu, J., Shuiqiang, Y., Yue, S., Zhengquan, W., and Dali, G. (2011). Influence of root structure on root survivorship: An analysis of 18 tree species using a minirhizotron method. Ecol. Res. 26, 755–762. doi: 10.1007/s11284-011-0833-4
Gu, J., Xu, Y., Dong, X., Wang, H., and Wang, Z. (2014). Root diameter variations explained by anatomy and phylogeny of 50 tropical and temperate tree species. Tree Physiol. 34, 415–425. doi: 10.1093/treephys/tpu019
Guo, D., Xia, M., Wei, X., Chang, W., Liu, Y., and Wang, Z. (2008). Anatomical traits associated with absorption and mycorrhizal colonization are linked to root branch order in twenty-three Chinese temperate tree species. New Phytol. 180, 673–683. doi: 10.1111/j.1469-8137.2008.02573.x
Hansson, K., Heljä-Sisko, H., Shambhu, P., and Holger, L. (2013). Fine root production and turnover of tree and understorey vegetation in scots pine, silver birch and Norway spruce stands in SW Sweden. For. Ecol. Manag. 309, 58–65. doi: 10.1016/j.foreco.2013.01.022
Hodge, A. (2004). The plastic plant: Root responses to heterogeneous supplies of nutrients. New Phytol. 162, 9–24. doi: 10.1111/j.1469-8137.2004.01015.x
Horn, J. (1965). A rationale and test for the number of factors in factor analysis. Psychometrika 30, 179–185. doi: 10.1007/BF02289447
Houlton, B., Wang, Y., Vitousek, P., and Field, C. (2008). A unifying framework for dinitrogen fixation in the terrestrial biosphere. Nature 454, 327–330. doi: 10.1038/nature07028
Kembel, S., and Cahill, J. (2005). Plant phenotypic plasticity belowground: A phylogenetic perspective on root foraging trade-offs. Am. Nat. 166, 216–230. doi: 10.1086/431287
Kong, D. L., Wang, J. J., Kardol, P., Wu, H. F., Zeng, H., Deng, X. B., et al. (2016). Economic strategies of plant absorptive roots vary with root diameter. Biogeosciences 13, 415–424. doi: 10.5194/bg-13-415-2016
Kong, D., Ma, C., Zhang, Q., Li, L., Chen, X., Zeng, H., et al. (2014). Leading dimensions in absorptive root trait variation across 96 subtropical forest species. New Phytol. 203, 863–872. doi: 10.1111/nph.12842
Kong, D., Wang, J., Zeng, H., Liu, M., Miao, Y., Wu, H., et al. (2017). The nutrient absorption-transportation hypothesis: Optimizing structural traits in absorptive roots. New Phytol. 213, 1569–1572 doi: 10.1111/nph.14344
Kraft, N., and Ackerly, D. D. (2010). Functional trait and phylogenetic tests of community assembly across spatial scales in an Amazonian forest. Ecol. Monogr. 80, 401–422. doi: 10.1890/09-1672.1
Kubisch, P., Hertel, D., and Leuschner, C. (2015). Do ectomycorrhizal and arbuscular mycorrhizal temperate tree species systematically differ in root order-related fine root morphology and biomass? Front. Plant Sci. 6:64. doi: 10.3389/fpls.2015.00064
Kursar, T., Bettina, M. J. E., Amy, B., Melvin, T. T., Bouchra, E. O., and Juan, P. (2009). Tolerance to low leaf water status of tropical tree seedlings is related to drought performance and distribution. Funct. Ecol. 23, 93–102. doi: 10.1111/j.1365-2435.2008.01483.x
Laughlin, D., Mommer, L., Sabatini, F., Bruelheide, H., Kuyper, T., McCormack, M., et al. (2021). Root traits explain plant species distributions along climatic gradients yet challenge the nature of ecological trade-offs. Nat. Ecol. Evol. 5, 1123–1134. doi: 10.1038/s41559-021-01471-7
Liese, R., Alings, K., and Meier, I. (2017). Root branching is a leading root trait of the plant economics spectrum in temperate trees. Front. Plant Sci. 8:315. doi: 10.3389/fpls.2017.00315
Liu, B., Li, H., Zhu, B., Koide, R., Eissenstat, D., and Guo, D. (2015). Complementarity in nutrient foraging strategies of absorptive fine roots and arbuscular mycorrhizal fungi across 14 coexisting subtropical tree species. New Phytol. 208, 125–136. doi: 10.1111/nph.13434
Lugli, L., Kelly, M., Luiz, E. O. C. A., Amanda, L., Hellen, F., Lucia, F., et al. (2020). Multiple phosphorus acquisition strategies adopted by fine roots in low-fertility soils in Central Amazonia. Plant Soil 450, 49–63. doi: 10.1007/s11104-019-03963-9
Lugli, L., Rosa, J., Andersen, K., Di Ponzio, R., Almeida, R., Pires, M., et al. (2021). Rapid responses of root traits and productivity to phosphorus and cation additions in a tropical lowland forest in Amazonia. New Phytol. 230, 116–128. doi: 10.1111/nph.17154
McCormack, M. L., Adams, T., Smithwick, E., and Eissenstat, D. (2012). Predicting fine root lifespan from plant functional traits in temperate trees. New Phytol. 195, 823–831. doi: 10.1111/j.1469-8137.2012.04198.x
Ma, Z., Guo, D., Xu, X., Lu, M., Bardgett, R., Eissenstat, D., et al. (2018). Evolutionary history resolves global organization of root functional traits. Nature 555, 94–97. doi: 10.1038/nature25783
Makita, N., Kosugi, Y., Dannoura, M., Takanashi, S., Niiyama, K., Kassim, A., et al. (2012). Patterns of root respiration rates and morphological traits in 13 tree species in a tropical forest. Tree Physiol. 32, 303–312. doi: 10.1093/treephys/tps008
McCormack, M., Dickie, I., Eissenstat, D., Fahey, T., Fernandez, C., Guo, D., et al. (2015). Redefining fine roots improves understanding of below-ground contributions to terrestrial biosphere processes. New Phytol. 207, 505–518. doi: 10.1111/nph.13363
Oksanen, J., Blanchet, F. G., Michael, F., Roeland, K., Pierre, L., Dan, M., et al. (2019). Vegan: Community ecology package. Available online at: https://CRAN.R-project.org/package=vegan (accessed October 5, 2022).
Pierick, K., Roman, M., Christoph, L., and Jürgen, H. (2022). Elevational trends of tree fine root traits in species-rich tropical Andean forests. Oikos 2022:e08975. doi: 10.1111/oik.08975
Pitman, N., Miles, R., and John, W. (2013). Oligarchies in Amazonian tree communities: A ten-year review. Ecography 36, 114–123. doi: 10.1111/j.1600-0587.2012.00083.x
Postma, J., Dathe, A., and Lynch, J. (2014). The optimal lateral root branching density for maize depends on nitrogen and phosphorus availability. Plant Physiol. 166, 590–602. doi: 10.1104/pp.113.233916
Pregitzer, K. S., DeForest, J. L., Burton, A. J., Allen, M. F., Ruess, R. W., and Hendrick, R. L. (2002). Fine root architecture of nine North American trees. Ecological Monographs 72, 293–309. doi: 10.2307/3100029
Quesada, C. A., Lloyd, J., Schwarz, M., Patiño, S., Baker, T. R., Czimczik, C., et al. (2009). Chemical and physical properties of amazon forest soils in relation to their genesis. Biosciences 6, 3923–3992. doi: 10.5194/bgd-6-3923-2009
R Core Team (2022). R: A language and environment for statistical computing. Vienna: R Foundation for Statistical Computing.
Ryser, P. (1996). The importance of tissue density for growth and life span of leaves and roots: A comparison of five ecologically contrasting grasses. Funct. Ecol. 10:717. doi: 10.2307/2390506
Soudzilovskaia, N., van Bodegom, P., Terrer, C., Zelfde, M., McCallum, I., Luke, M. M., et al. (2019). Global mycorrhizal plant distribution linked to terrestrial carbon stocks. Nat. Commun. 10:5077. doi: 10.1038/s41467-019-13019-2
Spitzer, C., Maja, K., David, A., Michael, J., and Paul, K. (2022). Root trait variation along a sub-arctic tundra elevational gradient. Oikos 2023:e08903. doi: 10.1111/oik.08903
Swenson, N., Brian, J., Jason, P., Andrew, J., Brad, B., Michael, D., et al. (2012). The biogeography and filtering of woody plant functional diversity in North and South America: Functional trait biogeography. Glob. Ecol. Biogeogr. 21, 798–808. doi: 10.1111/j.1466-8238.2011.00727.x
ter Steege, H., Pitman, N., Sabatier, D., Baraloto, C., Salomão, R., Guevara, J., et al. (2013). Hyperdominance in the Amazonian tree flora. Science 342:1243092. doi: 10.1126/science.1243092
Ter Steege, H., Prado, P., Lima, R., Pos, E., de Souza Coelho, L., de Andrade Lima Filho, D., et al. (2020). Biased-corrected richness estimates for the Amazonian tree flora. Sci. Rep. 10:10130. doi: 10.1038/s41598-020-66686-3
Valverde-Barrantes, O. J., Kurt, A., and Christopher, B. (2015). Fine root morphology is phylogenetically structured, but nitrogen is related to the plant economics spectrum in temperate trees. Funct. Ecol. 29, 796–807. doi: 10.1111/1365-2435.12384
Valverde-Barrantes, O., Amber, L., Kurt, A., and Christopher, B. (2016). Phylogenetically structured traits in root systems influence arbuscular mycorrhizal colonization in woody angiosperms. Plant Soil 404, 1–12. doi: 10.1007/s11104-016-2820-6
Valverde-Barrantes, O., Authier, L., Schimann, H., and Baraloto, C. (2021). Root anatomy helps to reconcile observed root trait syndromes in tropical tree species. Am. J. Bot. 108, 744–755. doi: 10.1002/ajb2.1659
Valverde-Barrantes, O., Freschet, G., Roumet, C., and Blackwood, C. (2017). A worldview of root traits: The influence of ancestry, growth form, climate and mycorrhizal association on the functional trait variation of fine-root tissues in seed plants. New Phytol. 215, 1562–1573. doi: 10.1111/nph.14571
Vitousek, P. (2004). Nutrient cycling and limitation: Hawai’i as a model system. Princeton, NJ: Princeton University Press.
Weemstra, M., Frank, J. S., Eric, J. W. V., Thomas, W. K., Leo, G., and Liesje, M. (2017). Fine-root trait plasticity of beech (Fagus Sylvatica) and Spruce (Picea Abies) forests on two contrasting soils. Plant Soil 415, 175–188. doi: 10.1007/s11104-016-3148-y
Weemstra, M., Jenny, Z., David, A., and María, N. U. (2021b). Tree growth increases through opposing above-ground and below-ground resource strategies. J. Ecol. 103, 3502–3512. doi: 10.1111/1365-2745.13729
Weemstra, M., Grégoire, T. F., Alexia, S., and Catherine, R. (2021a). Patterns in intraspecific variation in root traits are species-specific along an elevation gradient. Funct. Ecol. 35, 342–356. doi: 10.1111/1365-2435.13723
Weemstra, M., Mommer, L., Visser, E., van Ruijven, J., Kuyper, T., Mohren, G., et al. (2016). Towards a multidimensional root trait framework: A tree root review. New Phytol. 211, 1159–1169. doi: 10.1111/nph.14003
Weemstra, M., Thomas, W. K., Frank, J. S., and María, N. (2022). Incorporating belowground traits: Avenues towards a whole-tree perspective on performance. Oikos 2023:e08827. doi: 10.1111/oik.08827
Weigelt, A., Mommer, L., Andraczek, K., Iversen, C., Bergmann, J., Bruelheide, H., et al. (2021). An integrated framework of plant form and function: The belowground perspective. New Phytol. 232, 42–59. doi: 10.1111/nph.17590
Weiher, E., and Keddy, P. A. (1995). Assembly rules, null models, and trait dispersion: New questions from old patterns. Oikos 74:159. doi: 10.2307/3545686
Withington, J., Peter, B. R., Jacek, O., and David, M. E. (2006). Comparisons of Structure and life span in roots and leaves among temperate trees. Ecol. Monogr. 76, 381–397.
Wright, S. J. (2007). “Plant diversity in tropical forests,” in Functional plant ecology, eds P. Francisco and V. Fernando, 2nd Edn, Vol. 18, (Boca Raton, FL: CRC Press).
Keywords: Amazon forest, functional diversity, phylogeny, belowground traits, root economics space, tropical forests
Citation: Weemstra M, Valverde-Barrantes OJ, Fortunel C, Oblitas Mendoza EM, Prata EMB, Vásquez Pilco M, Vicentini A, Vleminckx J and Baraloto C (2023) Weak phylogenetic and habitat effects on root trait variation of 218 Neotropical tree species. Front. For. Glob. Change 6:1187127. doi: 10.3389/ffgc.2023.1187127
Received: 15 March 2023; Accepted: 27 April 2023;
Published: 26 May 2023.
Edited by:
Camille Emilie Defrenne, Michigan Technological University, United StatesReviewed by:
Hongbo Li, Chinese Academy of Agricultural Sciences, ChinaMengguang Han, Peking University, China
Coline Boonman, Aarhus University, Denmark
Copyright © 2023 Weemstra, Valverde-Barrantes, Fortunel, Oblitas Mendoza, Prata, Vásquez Pilco, Vicentini, Vleminckx and Baraloto. This is an open-access article distributed under the terms of the Creative Commons Attribution License (CC BY). The use, distribution or reproduction in other forums is permitted, provided the original author(s) and the copyright owner(s) are credited and that the original publication in this journal is cited, in accordance with accepted academic practice. No use, distribution or reproduction is permitted which does not comply with these terms.
*Correspondence: Monique Weemstra, moniqueweemstra@hotmail.com