- 1Department of Radiation Oncology, University Hospital Bonn, Bonn, Germany
- 2Department of Neurosurgery, University Hospital Bonn, Bonn, Germany
- 3Department of Neurology, Division of Neuro-Oncology, University Hospital Bonn, Bonn, Germany
Purpose: To simulate and analyze the dosimetric differences of intraoperative radiotherapy (IORT) or pre-operative single-fraction stereotactic radiosurgery (SRS) in addition to post-operative external beam radiotherapy (EBRT) in Glioblastoma (GB).
Methods: Imaging series of previously treated patients with adjuvant radiochemotherapy were analyzed. For SRS target definition, pre-operative MRIs were co-registered to planning CT scans and a pre-operative T1-weighted gross target volume (GTV) plus a 2-mm planning target volume (PTV) were created. For IORT, a modified (m)GTV was expanded from the pre-operative volume, in order to mimic a round cavity as during IORT. Dose prescription was 20 Gy, homogeneously planned for SRS and calculated at the surface for IORT, to cover 99% and 90% of the volumes, respectively. For tumors > 2cm in maximum diameter, a 15 Gy dose was prescribed. Plan assessment was performed after calculating the 2-Gy equivalent doses (EQD2) for both boost modalities and including them into the EBRT plan. Main points of interest encompass differences in target coverage, brain volume receiving 12 Gy or more (V12), and doses to various organs-at-risk (OARs).
Results: Seventeen pre-delivered treatment plans were included in the study. The mean GTV was 21.72 cm3 (SD ± 19.36) and mGTV 29.64 cm3 (SD ± 25.64). The mean EBRT and SRS PTV were 254.09 (SD ± 80.0) and 36.20 cm3 (SD ± 31.48), respectively. Eight SRS plans were calculated to 15 Gy according to larger tumor sizes, while all IORT plans to 20 Gy. The mean EBRT D95 was 97.13% (SD ± 3.48) the SRS D99 99.91% (SD ± 0.35) and IORT D90 83.59% (SD ± 3.55). Accounting for only-boost approaches, the brain V12 was 49.68 cm3 (SD ± 26.70) and 16.94 cm3 (SD ± 13.33) (p<0.001) for SRS and IORT, respectively. After adding EBRT results respectively to SRS and IORT doses, significant lower doses were found in the latter for mean Dmax of chiasma (p=0.01), left optic nerve (p=0.023), right (p=0.008) and left retina (p<0.001). No significant differences were obtained for brainstem and cochleae.
Conclusion: Dose escalation for Glioblastoma using IORT results in lower OAR exposure as conventional SRS.
Introduction
Since the standardization of adjuvant chemo-radiotherapy (CRT) for Glioblastoma (GB) over 15 years ago (1, 2), scarce progress has been achieved in order to improve the control and survival outcomes of these patients. Local recurrence, within the resection cavity or its close surroundings, remains to be the most frequent pattern of failure after combined treatment, including those patients in whom complete resection can be achieved, which promptly leads to detrimental clinical evolution and impaired quality of life (3, 4).
Under this rationale, different approaches have been proposed including new systemic agents and different RT modalities; however, they have repeatedly failed to improve the expected control and survival profiles (5, 6). Early strategies to improve such outcomes encompass dose-escalated RT. Different techniques have been assessed along the past four decades pre-dating the Temozolomide era, with mixed results. Increasing normofractionated doses in different levels was tested in the phase I RTOG 9803 study, meeting a safety profile up to 84 Gy and suggesting an improvement in survival outcomes at this dose level (7). Nevertheless, further studies have failed to confirm this hypothesis (8). Stereotactic radiotherapy, in either single (SRS) or multiple fractions (FSRT), has been investigated as well. Two major trials assessing both strategies (RTOG 9305 and RTOG 0023) in postoperative residual tumors could not demonstrate a significant survival benefit, although patients who achieved a complete resection allegedly profited from FSRT (9, 10). The main limitation of this strategy lies on toxicity, as increased rates of radionecrosis (RN) have been observed and are to be expected due to the usually large irradiation volumes.
Employing both low-dose (LDR) and high-dose rate (HDR) brachytherapy as an upscaling method has been additionally evaluated since the late 1980’s, with substantial differences between prescription doses, toxicity and control outcomes in reporting (11). Due to major concerns regarding radioprotection and an apparent increased rate of RN, probably related to a deeper dose prescription in healthy tissue, utilizing this approach has declined over time. Despite these issues, promising control rates have been published (12, 13). Similarly, earlier reports of electron-based intraoperative radiotherapy (IORT) suggested inspiring outcomes regarding local control, although no major impact on survival was noted.
Lately, based on these previous publications, the first clinical experiences of IORT with low-energy x-rays (kilovoltage) have described preliminary encouraging results in terms of both disease control and toxicity rates (14, 15). A most relevant feature of kilovoltage-IORT is a steep fall-off dose beyond the applicator, which allows preserving the surrounding unaffected tissue with an approximated 30% of the isodose reaching 1 cm (16).
This study provides a dosimetric comparison of pre-operative SRS against IORT as dose-escalation approaches in addition to normofractionated external-beam radiotherapy (EBRT) for patients diagnosed with glioblastoma.
Methods
Patients and Procedures
Patients diagnosed with GB and treated according to the standard of care (2) were retrospectively screened, in order to retrieve their imaging series. Simulation-CTs and pre- and post-operative MRIs were identified and rigidly co-registered. Those patients having tumors closer than 1 cm to either chiasma, optical nerves or brainstem were excluded. Standard 60-Gy EBRT volumes were delineated including a T1- and FLAIR-weighted postoperative MRI on each patient’s set, accounting for any residual gross tumor (GTV) and the resection cavity, a 1.5-cm CTV modified to encompass any surrounding edema, and 0.3-cm PTV. To simulate an upfront pre-operative SRS boost, the preoperative GTV was reconstructed in all cases based on the MRI T1-weighted tumoral uptake. For defining the SRS planning target volume (PTV), a 2-mm isotropic expansion from the GTV was applied. For IORT, a modified (m)GTV was expanded from the pre-operative MRI-T1 GTV according to the outermost borders of the lesion, in order to mimic a round cavity as during IORT with a spherical applicator. Furthermore, mGTV contours were enlarged circumferentially and homogeneously to fit the immediate larger applicator. Both GTV and mGTV volumes were subtracted from normal brain tissue delineations. An exemplary case is displayed in Figures 1A, B.
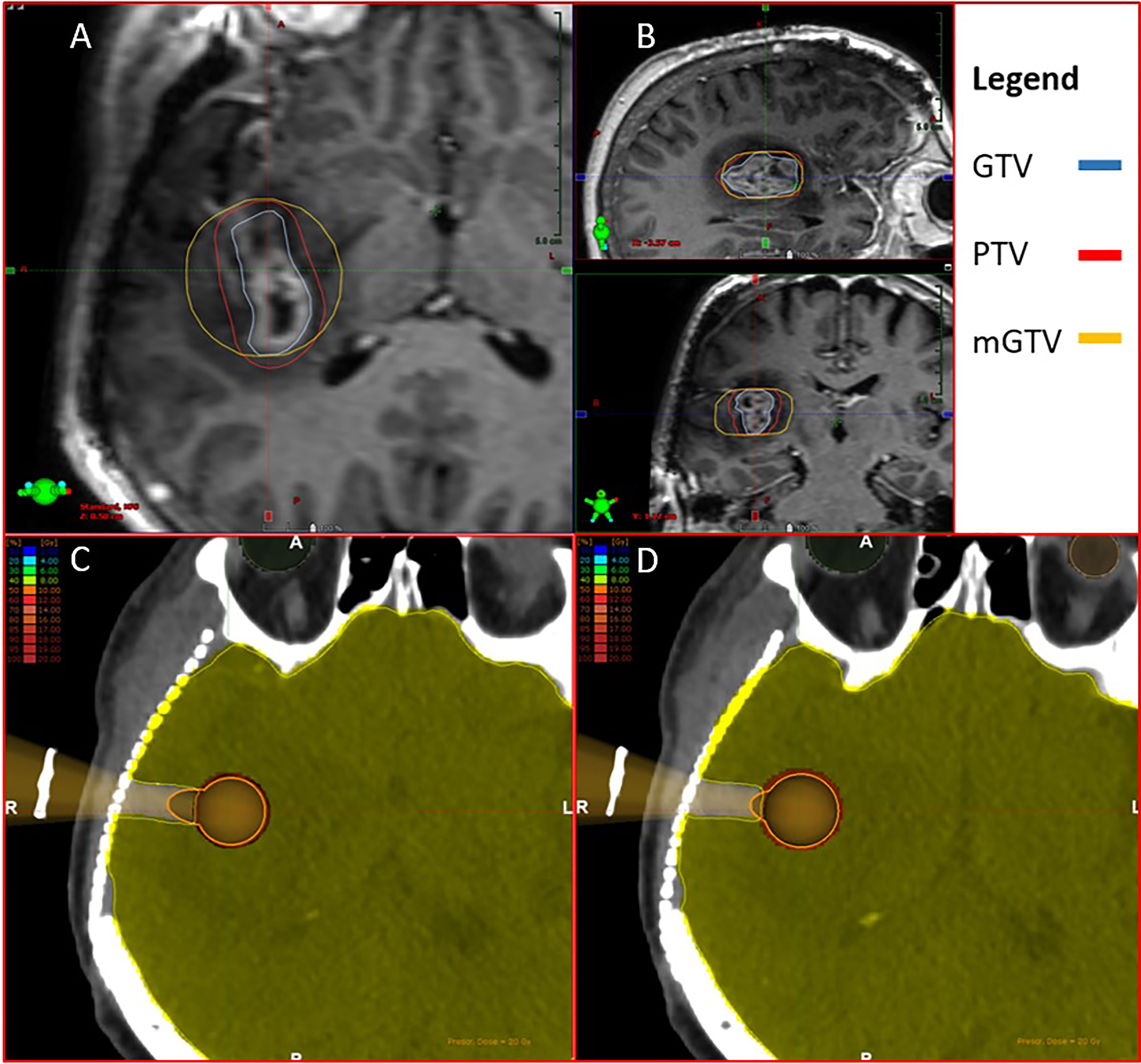
Figure 1 (A, B) Generated contours for intraoperative radiotherapy (IORT) and stereotactic radiosurgery (SRS) boosting. GTV: gross target volume, PTV: planning target volume, mGTV: modified gross target volume. mGTV was adjusted to fit the immediate larger apposite applicator. (C, D) Simulated path for the applicator placement. The healthy brain contour was removed from the hypothetical surgical trajectory.
The 60-Gy plans were set to cover 95% of the volume with 100% of the intended dose. Dose prescription was 20 Gy, homogeneously planned for SRS and prescribed to the surface of the IORT applicator, to cover 99% and 90% of the volumes, respectively. For SRS plans with GTVs larger than 2 cm in maximum diameter, a 15 Gy dose was prescribed. All contours, EBRT and SRS calculations were performed on Eclipse 13.6 for TrueBeam STx (Varian Medical Systems, Palo Alto, CA, USA), equipped with a High Definition Multileaf Collimator (HD 120MLC). Intraoperative RT contours were exported to and planned with Radiance (GMV SA, Madrid, Spain), employing a Monte Carlo simulation algorithm for low-energy x-rays delivered with Intrabeam (Carl Zeiss Meditec AG, Jena, Germany).
Delivered SRS and IORT doses to OARs were converted to 2-Gy equivalents (EQD2) considering a 2-Gy α/β factor (17) if exceeding a 2-Gy exposure in this single application. These dose results were added to the 60-Gy EBRT plans for final assessment.
Constraints were adopted from the INTRAGO II protocol (NCT02685605) for both boost modalities with a maximum 8-Gy tolerance dose to OARs, encompassing the brain volume (cm3) receiving 12 Gy (V12) to be lower than 10 cm3. In addition, the combined 60-Gy EBRT and boost doses were set to a total EQD2 Dmax 66 Gy for brainstem, Dmax 55 Gy for chiasma and optical nerves, cochleae Dmean 35 Gy and retinae Dmean 45 Gy.
Endpoints
Main points of interest include differences in target coverage (V99 = 99% for SRS and V90 = 100% for IORT), brain V12 between only SRS and IORT plans, constraint compliance, and maximum (Dmax = 0.03 cm3) and mean dose (Dmean) exposure to brainstem, chiasma, optic nerves, retinae and cochleae, correspondingly.
Statistical Analysis
Comparative mean and median measurements and their corresponding standard deviation (SD) or ranges are described accordingly. The t-test was employed to determine the statistical significance between differences in continuous variables, assuming a p ≤ 0.05.
Ethics
This investigation was released from Institutional Review Board (IRB) approval due to its retrospective comparative planning nature. All data sets were anonymized prior to the analysis and no personal information is consigned in this manuscript, in concordance with the principles of the Declaration of Helsinki
Results
Patients and Planning Features
After patient selection, seventeen pre-delivered treatment plans were included in the study. The mean preoperative GTV volume was 21.72 cm3 (SD ± 19.36) and mGTV 29.64 cm3 (SD ± 25.64). The mean EBRT and SRS PTVs were 254.09 (SD ± 80.0) and 36.20 cm3 (SD ± 31.48), respectively. Eight SRS plans were calculated to 15 Gy according to larger tumor sizes, whereas all IORT plans to 20 Gy. The median applicator size was 35 mm (15 – 50). These features are summarized in Table 1.
The mean EBRT D95 was 97.13% (SD ± 3.48), the SRS D99 99.91% (SD ± 0.35) and IORT D90 83.59% (SD ± 3.55). The mean SRS conformity index (CI) was 1.26 (SD ± 0.35). Accounting for only-boost approaches, the brain V12 was 49.68 cm3 (SD ± 26.70) and 16.94 cm3 (SD ± 13.33) (p<0.001) for SRS and IORT, respectively. No SRS patients in contrast to eight IORT patients could fulfill the V12 tolerance criterion. All IORT plans with mGTVs under 30 cm3 or applicators smaller than 3.5 cm and a plan with a 44.8 cm3 lesion and a 45 mm applicator achieved V12 exposures under 10 cm3. Two additional IORT patients showed V12 exposures of 13.16 and 12.84 cm3, with smaller target volumes as the abovementioned. Regarding the other OAR constraints, four SRS patients reached doses over the preset tolerance of brainstem, chiasma, left and right optic nerves, in comparison to three IORT patients with overdosing on the three latter structures.
After SRS and IORT EQD2 calculations and adding EBRT results, mean brainstem Dmax was 44.06 Gy (SD ± 17.75) and 42.91 (SD ± 16.84; p=0.228), respectively. Combined SRS and IORT exposure to chiasma was Dmax 28.96 Gy (SD ± 19.09) and 27.72 Gy (SD ±18.50; p=0.01), right optic nerve Dmax 19.51 Gy (SD ± 18.69) and 19.48 (SD ± 19.48; p=0.977), and left optic nerve Dmax 15.71 Gy (SD ± 16.23) and 14.60 Gy (SD ± 14.66; p=0.023). For mean Dmean exposures, right retina received 7.28 Gy (SD ± 6.37) and 6.50 Gy (SD ±5.71; p=0.008), left retina 6.24 Gy (SD ± 5.61) and 5.66 Gy (SD ± 5.57, p<0.001), right cochlea 10.74 Gy (SD ± 10.74) and 11.85 Gy (SD ± 15.35; p=0.295), and left cochlea 9.43 Gy (SD ± 10.75) and 9.72 Gy (SD ± 10.96; p=0.645). These results are shown in Table 2.
Graphic examples of tissue exposure and dose distribution can be observed in Figures 2 and 3. The detailed resulting doses for the entire cohort are displayed in Appendix 1.
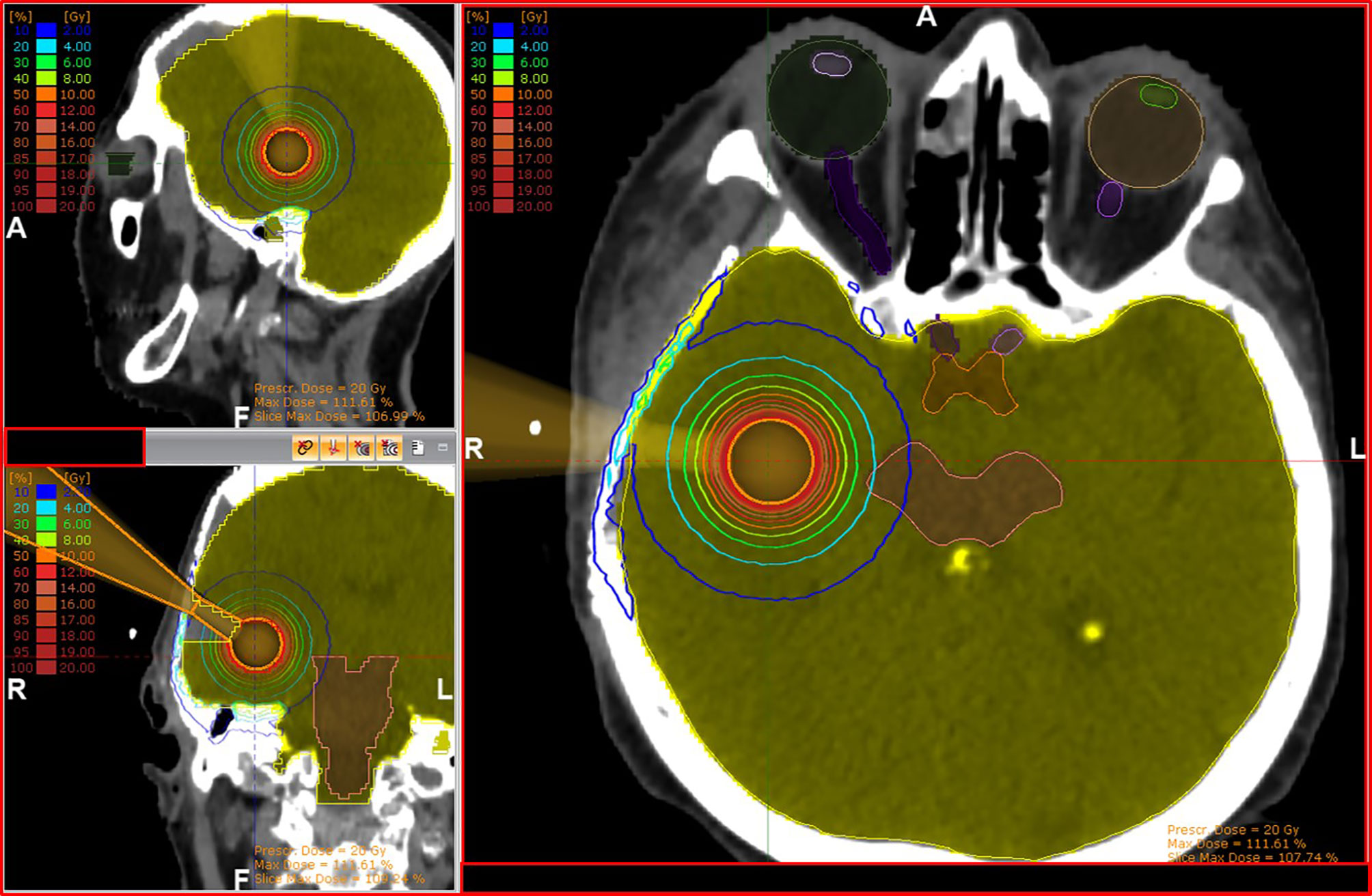
Figure 2 Exemplary case with three-dimensional views of isodose line distribution for a 2.5-cm IORT applicator. Doses reaching the reconstruction plaque should be disregarded to resemble a real-world surgical situation.
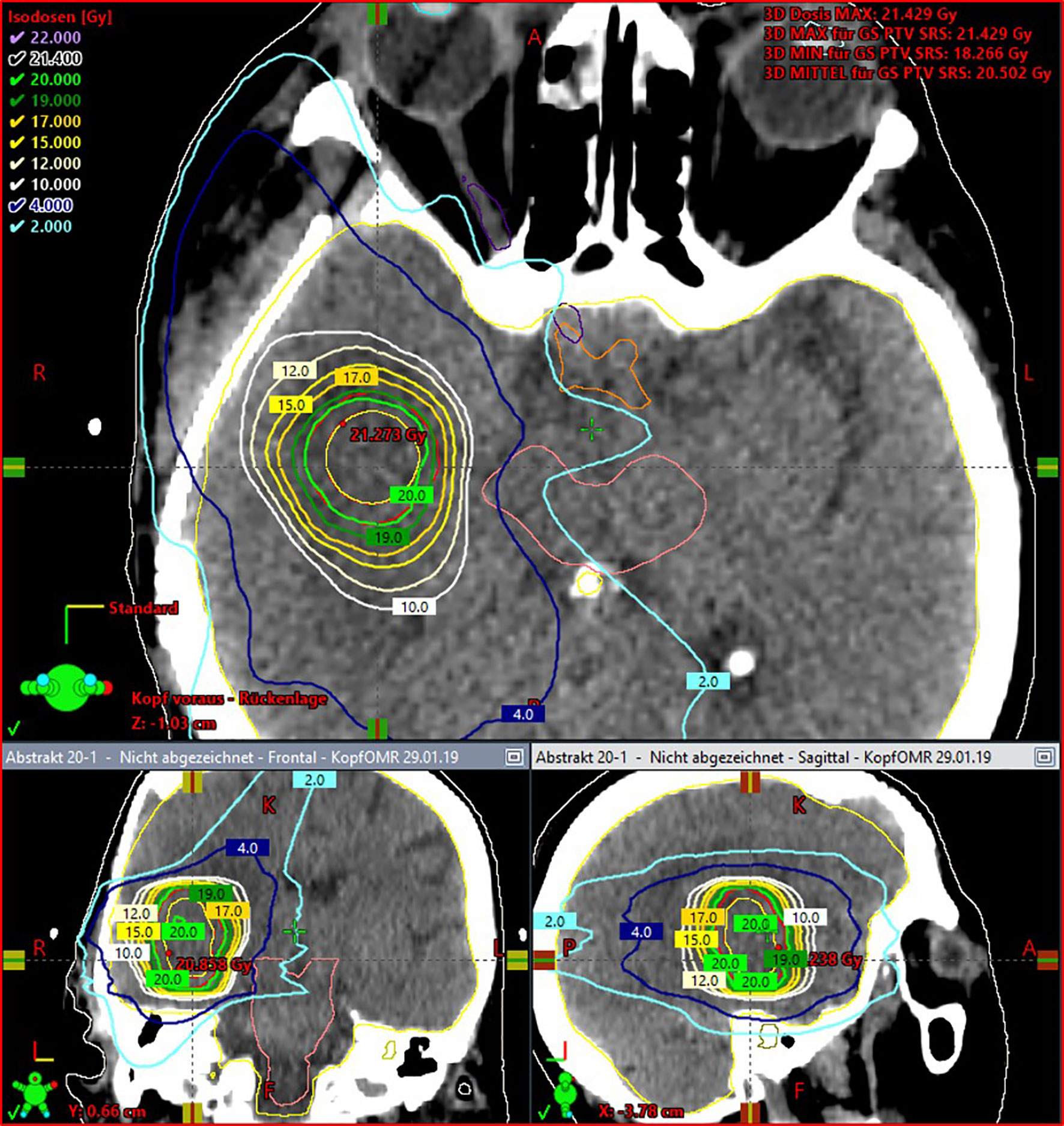
Figure 3 Exemplary case with three-dimensional views of isodose line distribution for upfront SRS boosting, homogeneously delivered to a 2-mm PTV.
Discussion
This is the first dosimetric comparison of pre-operative SRS and kilovoltage IORT as dose-escalation approaches for patients with GB. The rationale for comparing these modalities lies on both the ongoing INTRAGO II trial and historical results from randomized trials, which failed to demonstrate clinical benefit of post-operative SRS (10). Furthermore, an inherent feature of post-surgical irradiation is a significant shape variability of resection cavities (18), which usually leads to rather large boost volumes and increased rates of toxicity. Despite some minor shortcomings, reconstructing the pre-operative GTVs yields a more reliable and homogeneous comparison mean between upfront SRS and IORT deliveries. Nevertheless, employing dose-escalated RT is nowadays a controversial strategy to improve GB treatment outcomes and must be explored in a prospective manner. Previous experiences from the RTOG 9305 and 0023 failed to demonstrate any survival benefit when targeting residual disease (9, 10). On the contrary, although not powered for this endpoint, the phase I RTOG 9803 trial suggested a survival benefit for these patients in comparison to the standard of care (7). This hypothesis has been further pondered according to mathematical models suggesting enhanced results after dose-escalation, based on both clinical and in-vitro data (19, 20). From the clinical perspective, a relevant feature inherent to the previously mentioned studies was assessing patients with residual tumors and targeting these lesions, which does not allow a comprehensive management of the entire surgical cavity. This should be taken into consideration for dose-escalation strategies, as the prognostic value of residual disease is widely known to be impairing in terms of survival. The ongoing INTRAGO II trial (NCT02685605) will help elucidate this matter.
Target volume coverage was assessed according to institutional and international EBRT (D95 = 100%), SRS (D99 = 99%) and brachytherapy (D90 = 100%) planning standards (21–23). A major discrepancy was observed between both boost techniques in terms of target coverage. The mGTV portion lying behind the “neck” of the applicator does not receive a significant dose, implying a poor distribution at this level. However, in a real-life setting, this area represents the surgical entry path and as such, it would not be accounted for calculations. In addition, the contours generated on this platform cannot be modified to allow hollow structures. Therefore, these dosimetric differences, although reported, must be disregarded due to practical reasons. A direct or statistical comparison would not be feasible according to the abovementioned and it is suggested to assess each single modality by separate. A graphical example can be seen in Figures 1C, D.
Prescription doses, selected in two levels, resemble of those according to the RTOG 9305 protocol for the larger lesions and the INTRAGO II protocol for the smaller ones. The latter allows a therapeutic range between 30 to 20 Gy, according to the applicator’s proximity to OARs (< 1.5 cm) or exposure over tolerance (Dmax 8 Gy). In this cohort, all IORT plans were calculated to 20 Gy delivered at the surface, easing the comparison between both techniques and mimicking real-life conditions, mostly for SRS plans. Due to these handicaps and difficulties in achieving the predefined constraints, eight SRS plans had to be performed with a 15-Gy prescription, in contrast to no IORT plans. Despite these adjustments and after adding EBRT doses, four SRS plans could not meet the non-V12 constraints in comparison to three IORT plans. Nevertheless, it is noteworthy that, although significant differences between some mean OAR doses were observed, these outcomes might not be clinically significant given the marginal numeric variability. This is naturally variable amongst patients according to the irradiated area or volume, although clear advantage of IORT over SRS can be anticipated for smaller irradiated targets, thus increased healthy tissue sparing. Doses reaching the unaffected organ can be restricted due to its steep fall-off profile and no need of any security margin around the mGTV (resection cavity). This is the most relevant factor for significantly diminishing brain V12 with this approach. It is worth to mention that the SRS PTV volumes herein obtained were considerably larger than those of mGTV for IORT and this might be highly variable according to local standards. However, even when considering applying smaller or no PTV expansions, the dose-distribution profile of kilovoltage still results superior in comparison to megavoltage. The 2-mm PTV expansion used in this study represents our local standard for linear accelerator-based SRS, which might vary according to local standards or available technology, such as multi-source cobalt-based SRS. Regarding the planning technique, all SRS plans were calculated homogeneously, as described under Methods. This was decided according to our local standards, which in our experience yields similar surrounding tissue exposure, as different other features influence the fall-off dose profile, such as number of rotations, arcs, amongst others. In the setting of a pre-operative SRS, as proposed in this study, intratumoral dose-escalation would not play a relevant role, when the cavity border is of higher importance.
It is worth highlighting the potential advantage of lower V12 results as a RN prediction tool. With almost all previously reported experiences converging on a similar point, it is of considerable relevance to maintain this parameter within acceptable ranges (17, 24). In our cohort, patients who had mGTV smaller than 30 cm3 or were planned with ≤ 3.5 cm applicators yielded improved V12 results. On the contrary, no SRS plan would be acceptable for clinical practice, even after limiting the prescription doses to 15 Gy. It should be considered as well, that no specific constraints for healthy brain irradiation have been defined when combining IORT/SRS to EBRT. Therefore, increased attention should be given to exposed unaffected tissue. Based on published clinical experiences, an estimated 15 – 20% grade 3 RN is to be expected after combining EBRT and IORT; however, no variables were individually assessed to determine which of them could mostly contribute to these events (14, 15). These outcomes are closely bound to earlier dosimetric data, which described a direct relationship between larger applicator sizes and increased doses in depth (16). According to this information, patient selection must be carefully performed in order to avoid undesired adverse events.
Limitations to this investigation include both its retrospective nature and definition of intraoperative target volumes. Although this method might be the most accurate to retrospectively simulate a surgical bed, intrinsic anatomical variations inherent to the surgical procedure and tissue re-accommodation, which currently cannot be accounted for, are still to be present. In addition, due to the differences in dose levels, a plan sum for both EBRT and SRS/IORT modalities seems impractical when assessing the healthy brain exposure. Implications of this drawback might include an inability to calculate accurately the actual clinical risks of dose escalation. Parameters such as V12 have been studied largely for single fraction applications, with no available data on combined normo- and ablative fractionations. Results of ongoing clinical trials will help to overcome this shortcoming, based on their already available preliminary results (14, 15). Furthermore, the relative biological effectiveness (RBE) factor of kilovoltage therapy has not been considered amongst the resulting calculations of IORT (25). The large doses applied and low reliability of the linear-quadratic model at such levels, in addition to a lacking clinical validation, led us to omit its application. The linear-quadratic model itself carries certain flaws when assessing large doses per fraction; however, it has been widely adopted to allow comparisons between treatment schemes and pool data, even in settings like this (26). We suggest deeming all available factors when considering IORT-boosting. Taken together, this investigation provides relevant information regarding the exposed healthy brain tissue and OARs for both dose-escalation strategies. Intraoperative imaging with the applicator in situ is required to accurately reproduce treatment delivery.
Conclusion
Dose escalation with IORT yields significant lower healthy brain V12 exposure in comparison to pre-operative SRS for Glioblastoma patients, while allowing a higher dose delivery to the surgical bed. Larger IORT applicator sizes might relate to increased V12 results. Careful patient selection must be performed in order to diminish RN rates.
Data Availability Statement
The original contributions presented in the study are included in the article/Supplementary Material. Further inquiries can be directed to the corresponding author.
Ethics Statement
This investigation was released from Institutional Review Board (IRB) approval due to its retrospective comparative planning nature. All data sets were anonymized prior to the analysis and no personal information is consigned in this manuscript, in concordance with the principles of the Declaration of Helsinki.
Author Contributions
GS: study conceptualization and design, data production, collection and statistical analysis, manuscript drafting and editing. ZS: data production and collection. TM: study design, data production and analysis. JH: data production. DS: manuscript review and editing. DK: manuscript review and editing. SG: data review. MS: manuscript review. MH: manuscript review. HV: manuscript review. UH: manuscript review. FG: study design, manuscript review and editing. LS: study conceptualization and design, manuscript review and editing. All authors contributed to the article and approved the submitted version.
Conflict of Interest
GS: personal fees from Carl Zeiss Meditec AG and personal fees from Roche Pharma AG, not related to this work. FG: research grants and travel expenses from ELEKTA AB; grants, stocks, travel expenses and honoraria from NOXXON Pharma AG; research grants, travel expenses and honoraria from Carl Zeiss Meditec AG; travel expenses and honoraria from Bristol-Myers Squibb, Roche Pharma AG, MSD Sharp and Dohme GmbH and AstraZeneca GmbH; non-financial support from Oncare GmbH and Opasca GmbH, not related to this work.
The remaining authors declare that the research was conducted in the absence of any commercial or financial relationships that could be construed as a potential conflict of interest.
Publisher’s Note
All claims expressed in this article are solely those of the authors and do not necessarily represent those of their affiliated organizations, or those of the publisher, the editors and the reviewers. Any product that may be evaluated in this article, or claim that may be made by its manufacturer, is not guaranteed or endorsed by the publisher.
Supplementary Material
The Supplementary Material for this article can be found online at: https://www.frontiersin.org/articles/10.3389/fonc.2021.759873/full#supplementary-material
References
1. Stupp R, Hegi ME, Mason WP, van den Bent MJ, Taphoorn MJB, Janzer RC, et al. Effects of Radiotherapy With Concomitant and Adjuvant Temozolomide Versus Radiotherapy Alone on Survival in Glioblastoma in a Randomised Phase III Study: 5-Year Analysis of the EORTC-NCIC Trial. Lancet Oncol (2009) 10(5):459–66. doi: 10.1016/s1470-2045(09)70025-7
2. Stupp R, Mason WP, van den Bent MJ, Weller M, Fisher B, Taphoorn MJ, et al. Radiotherapy Plus Concomitant and Adjuvant Temozolomide for Glioblastoma. N Engl J Med (2005) 352(10):987–96. doi: 10.1056/NEJMoa043330
3. Petrecca K, Guiot MC, Panet-Raymond V, Souhami L. Failure Pattern Following Complete Resection Plus Radiotherapy and Temozolomide is at the Resection Margin in Patients With Glioblastoma. J Neurooncol (2013) 111(1):19–23. doi: 10.1007/s11060-012-0983-4
4. Gaspar LE, Fisher BJ, Macdonald DR, Leber DV, Halperin EC, Clifford SS, et al. Supratentorial Malignant Glioma: Patterns of Recurrence and Implications for External Beam Local Treatment. Int J Radiat Oncol Biol Phys (1992) 24(1):55–7. doi: 10.1016/0360-3016(92)91021-e
5. Gilbert MR, Dignam JJ, Armstrong TS, Wefel JS, Blumenthal DT, Vogelbaum MA, et al. A Randomized Trial of Bevacizumab for Newly Diagnosed Glioblastoma. N Engl J Med (2014) 370(8):699–708. doi: 10.1056/NEJMoa1308573
6. Stupp R, Hegi ME, Gorlia T, Erridge SC, Perry J, Hong Y-K, et al. Cilengitide Combined With Standard Treatment for Patients With Newly Diagnosed Glioblastoma With Methylated MGMT Promoter (CENTRIC EORTC 26071-22072 Study): A Multicentre, Randomised, Open-Label, Phase 3 Trial. Lancet Oncol (2014) 15(10):1100–8. doi: 10.1016/s1470-2045(14)70379-1
7. Tsien C, Moughan J, Michalski JM, Gilbert MR, Purdy J, Simpson J, et al. Phase I Three-Dimensional Conformal Radiation Dose Escalation Study in Newly Diagnosed Glioblastoma: Radiation Therapy Oncology Group Trial 98-03. Int J Radiat Oncol Biol Phys (2009) 73(3):699–708. doi: 10.1016/j.ijrobp.2008.05.034
8. Badiyan SN, Markovina S, Simpson JR, Robinson CG, DeWees T, Tran DD, et al. Radiation Therapy Dose Escalation for Glioblastoma Multiforme in the Era of Temozolomide. Int J Radiat Oncol Biol Phys (2014) 90(4):877–85. doi: 10.1016/j.ijrobp.2014.07.014
9. Cardinale R, Won M, Choucair A, Gillin M, Chakravarti A, Schultz C, et al. A Phase II Trial of Accelerated Radiotherapy Using Weekly Stereotactic Conformal Boost for Supratentorial Glioblastoma Multiforme: RTOG 0023. Int J Radiat Oncol Biol Phys (2006) 65(5):1422–8. doi: 10.1016/j.ijrobp.2006.02.042
10. Souhami L, Seiferheld W, Brachman D, Podgorsak EB, Werner-Wasik M, Lustig R, et al. Randomized Comparison of Stereotactic Radiosurgery Followed by Conventional Radiotherapy With Carmustine to Conventional Radiotherapy With Carmustine for Patients With Glioblastoma Multiforme: Report of Radiation Therapy Oncology Group 93-05 Protocol. Int J Radiat Oncol Biol Phys (2004) 60(3):853–60. doi: 10.1016/j.ijrobp.2004.04.011
11. Gutin PH, Prados MD, Phillips TL, Wara WM, Larson DA, Leibel SA, et al. External Irradiation Followed by an Interstitial High Activity Iodine-125 Implant “Boost” in the Initial Treatment of Malignant Gliomas: NCOG Study 6G-82-2 Gliomas: NCOG Study 6H-82-2. Int J Radiat Oncol Biol Phys (1991) 21(3):601–6. doi: 10.1016/0360-3016(91)90676-u
12. Adkison JB, Thomadsen B, Howard SP. Systemic Iodine 125 Activity After GliaSite Brachytherapy: Safety Considerations. Brachytherapy (2008) 7(1):43–6. doi: 10.1016/j.brachy.2007.11.001
13. Barbarite E, Sick JT, Berchmans E, Bregy A, Shah AH, Elsayyad N, et al. The Role of Brachytherapy in the Treatment of Glioblastoma Multiforme. Neurosurg Rev (2017) 40(2):195–211. doi: 10.1007/s10143-016-0727-6
14. Sarria GR, Sperk E, Han X, Sarria GJ, Wenz F, Brehmer S, et al. Intraoperative Radiotherapy for Glioblastoma: An International Pooled Analysis. Radiother Oncol (2020) 142:162–7. doi: 10.1016/j.radonc.2019.09.023
15. Giordano FA, Brehmer S, Murle B, Welzel G, Sperk E, Keller A, et al. Intraoperative Radiotherapy in Newly Diagnosed Glioblastoma (INTRAGO): An Open-Label, Dose-Escalation Phase I/II Trial. Neurosurgery (2019) 84(1):41–9. doi: 10.1093/neuros/nyy018
16. Sethi A, Emami B, Small W, Thomas TO. Intraoperative Radiotherapy With INTRABEAM: Technical and Dosimetric Considerations. Front Oncol (2018) 8:74(74). doi: 10.3389/fonc.2018.00074
17. Milano MT, Grimm J, Niemierko A, Soltys SG, Moiseenko V, Redmond KK, et al. Single- and Multifraction Stereotactic Radiosurgery Dose/Volume Tolerances of the Brain. Int J Radiat Oncol Biol Phys (2021) 110(1):68–86. doi: 10.1016/j.ijrobp.2020.08.013
18. Rogers CM, Jones PS, Weinberg JS. Intraoperative MRI for Brain Tumors. J Neurooncol (2021) 151(3):479–90. doi: 10.1007/s11060-020-03667-6
19. Burnet NG, Jena R, Jefferies SJ, Stenning SP, Kirkby NF. Mathematical Modelling of Survival of Glioblastoma Patients Suggests a Role for Radiotherapy Dose Escalation and Predicts Poorer Outcome After Delay to Start Treatment. Clin Oncol (R Coll Radiol) (2006) 18(2):93–103. doi: 10.1016/j.clon.2005.08.017
20. Dionysiou DD, Stamatakos GS, Gintides D, Uzunoglu N, Kyriaki K. Critical Parameters Determining Standard Radiotherapy Treatment Outcome for Glioblastoma Multiforme: A Computer Simulation. Open BioMed Eng J (2008) 2:43–51. doi: 10.2174/1874120700802010043
21. Hodapp N. [The ICRU Report 83: Prescribing, Recording and Reporting Photon-Beam Intensity-Modulated Radiation Therapy (IMRT)]. Strahlenther Oncol (2012) 188(1):97–9. doi: 10.1007/s00066-011-0015-x
22. Kirisits C, Rivard MJ, Baltas D, Ballester F, De Brabandere M, van der Laarse R, et al. Review of Clinical Brachytherapy Uncertainties: Analysis Guidelines of GEC-ESTRO and the AAPM. Radiother Oncol (2014) 110(1):199–212. doi: 10.1016/j.radonc.2013.11.002
23. Wilke L, Andratschke N, Blanck O, Brunner TB, Combs SE, Grosu AL, et al. ICRU Report 91 on Prescribing, Recording, and Reporting of Stereotactic Treatments With Small Photon Beams : Statement From the DEGRO/DGMP Working Group Stereotactic Radiotherapy and Radiosurgery. Strahlenther Onkol (2019) 195(3):193–8. doi: 10.1007/s00066-018-1416-x
24. Minniti G, Clarke E, Lanzetta G, Osti MF, Trasimeni G, Bozzao A, et al. Stereotactic Radiosurgery for Brain Metastases: Analysis of Outcome and Risk of Brain Radionecrosis. Radiat Oncol (2011) 6:48. doi: 10.1186/1748-717X-6-48
25. Liu Q, Schneider F, Ma L, Wenz F, Herskind C. Relative Biologic Effectiveness (RBE) of 50 kV X-Rays Measured in a Phantom for Intraoperative Tumor-Bed Irradiation. Int J Radiat Oncol Biol Phys (2013) 85(4):1127–33. doi: 10.1016/j.ijrobp.2012.08.005
Keywords: dose escalation, SRS, IORT, kilovoltage, glioblastoma
Citation: Sarria GR, Smalec Z, Muedder T, Holz JA, Scafa D, Koch D, Garbe S, Schneider M, Hamed M, Vatter H, Herrlinger U, Giordano FA and Schmeel LC (2021) Dosimetric Comparison of Upfront Boosting With Stereotactic Radiosurgery Versus Intraoperative Radiotherapy for Glioblastoma. Front. Oncol. 11:759873. doi: 10.3389/fonc.2021.759873
Received: 17 August 2021; Accepted: 13 October 2021;
Published: 28 October 2021.
Edited by:
William Small, Jr., Loyola University Chicago, United StatesReviewed by:
Raphael Pfeffer, Assuta Medical Center, IsraelJoshua Silverman, New York University, United States
Luis Schiappacasse, Centre Hospitalier Universitaire Vaudois (CHUV), Switzerland
Copyright © 2021 Sarria, Smalec, Muedder, Holz, Scafa, Koch, Garbe, Schneider, Hamed, Vatter, Herrlinger, Giordano and Schmeel. This is an open-access article distributed under the terms of the Creative Commons Attribution License (CC BY). The use, distribution or reproduction in other forums is permitted, provided the original author(s) and the copyright owner(s) are credited and that the original publication in this journal is cited, in accordance with accepted academic practice. No use, distribution or reproduction is permitted which does not comply with these terms.
*Correspondence: Gustavo R. Sarria, Z3VzdGF2by5zYXJyaWFAdWtib25uLmRl