The fetal pain paradox
- University of Mary, Bismarck, ND, United States
Controversy exists as to when conscious pain perception in the fetus may begin. According to the hypothesis of cortical necessity, thalamocortical connections, which do not form until after 24–28 weeks gestation, are necessary for conscious pain perception. However, anesthesiologists and neonatologists treat age-matched neonates as both conscious and pain-capable due to observable and measurable behavioral, hormonal, and physiologic indicators of pain. In preterm infants, these multimodal indicators of pain are uncontroversial, and their presence, despite occurring prior to functional thalamocortical connections, has guided the use of analgesics in neonatology and fetal surgery for decades. However, some medical groups state that below 24 weeks gestation, there is no pain capacity. Thus, a paradox exists in the disparate acknowledgment of pain capability in overlapping patient populations. Brain networks vary by age. During the first and second trimesters, the cortical subplate, a unique structure that is present only during fetal and early neonatal development, forms the first cortical network. In the third trimester, the cortical plate assumes this function. According to the subplate modulation hypothesis, a network of connections to the subplate and subcortical structures is sufficient to facilitate conscious pain perception in the fetus and the preterm neonate prior to 24 weeks gestation. Therefore, similar to other fetal and neonatal systems that have a transitional phase (i.e., circulatory system), there is now strong evidence for transitional developmental phases of fetal and neonatal pain circuitry.
Introduction
Controversy exists as to when conscious pain perception in the fetus may begin. Currently, two hypotheses prevail that are distinguished by a demarcating line at 24 weeks gestation. First, according to the hypothesis of cortical necessity, functional thalamocortical projections to the somatosensory cortex that develop after 24–28 weeks gestation are required before conscious pain perception is possible (1, 2). Second, the subplate modulation hypothesis holds that functional activity in the cortical subplate and/or subcortical structures is sufficient to mediate pain perception in the fetus before 24 weeks gestation (3), and possibly as early as 12 weeks gestation (4). The subplate, a transient layer located beneath the cortical plate in the developing cortex, forms the predominant cortical circuitry from the first through third trimesters (5).
Determining the onset of pain perception is important, as invasive procedures affect both the fetus and the preterm neonate before 24 weeks, prompting consideration of analgesia (pain relief) and anesthesia (loss of physical sensation with or without loss of consciousness). In clinical practice, observable and measurable behavioral, hormonal, and physiologic indicators of pain, prior to 24 weeks gestation, have guided the use of analgesics in neonatology, fetal surgery, and fetal anesthesiology for decades. Pain is acknowledged and treated in the earliest preterm neonates <24–28 weeks using validated pain assessment tools (6). Fetal surgeons administer direct fetal analgesia and anesthesia as early as 15–16 weeks gestation (7, 8). Fetal anesthesiologists recommend the use of fetal anesthesia from the second trimester onward (>14 weeks gestation) (9), or in all invasive maternal-fetal procedures regardless of gestational age, in order to “inhibit the humoral stress response, decrease fetal movement, and blunt any perception of pain [(10), p. 1167].”
Conversely, the American College of Obstetricians and Gynecologists (ACOG), the Society for Maternal Fetal Medicine (SMFM), and the Royal College of Obstetricians and Gynaecologists (RCOG) state that (1) pain perception requires a comprehensive network of neural connections to the cerebral cortex that is not possible until at least 24–25 weeks and unlikely until after 28 weeks gestation; (2) accepted behavioral and physiologic indicators of pain in the extremely preterm infant (<28 weeks) are reflexive or spontaneous and not indicative of a pain experience; and (3) the use of anesthesia or analgesia in neonatal and prenatal surgery serves purposes unrelated to pain, such as preventing long-term consequences of stress responses and decreasing fetal movement (1, 2, 11). Thus, a paradox exists in the disparate acknowledgment and treatment of pain perception by different medical groups in neonates <24–28 weeks gestation and fetuses of similar age (Figure 1).
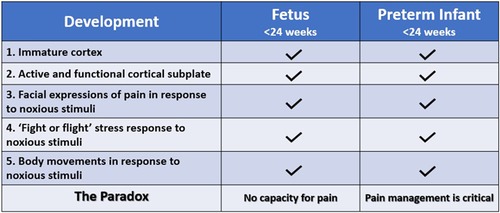
Figure 1. The fetal pain paradox. Both the fetus and preterm infant <24 weeks gestation have an immature cerebral cortex (12) and an active, functional cortical sublate (13, 14). Both mount hormonal and hemodynamic stress responses (6, 15) and demonstrate pain-related facial expressions (6, 16) and body movements (6, 15) following noxious stimuli. The standard of care for preterm infants, according to the American Academy of Pediatrics, is pain management utilizing validated pain assessment tools. However, the American College of Obstetricians and Gynecologists (11), the Society for Maternal Fetal Medicine (1), and the Royal College of Obstetricians and Gynaecologists (2) state that pain perception is not possible until after 24–28 weeks gestation.
Comparison between the preterm infant and the age-matched fetus is supported by similarities between these two populations. The fetus and the preterm infant share a predominantly fetal physiology with an immature cortex and an active cortical subplate. Both exhibit pain-related responses to noxious stimuli including body movements, facial expressions, and hormonal and physiologic responses (Figure 1). Both may also exhibit “freeze and dive” behavioral and physiologic responses to painful stimuli, in which the fetus and preterm infant become immobilized, and observable responses to noxious stimuli are muted or absent due to lack of energy reserves or underlying physiologic stress (17–20). Differences between the extrauterine and intrauterine environments also affect such comparisons. First, very low gestational age infants are generally in a critical state of health requiring intensive care, while the age-matched fetus in utero is generally in a state of homeostasis. Second, different sensory experiences in the extrauterine environment, particularly medically-indicated noxious procedures (ie. heel lances), may affect neurodevelopment. Studies show that premature infants with at least 40 days in the neonatal intensive care unit (NICU) have increased neuronal responses to noxious stimuli compared to healthy neonates born at the same corrected age (21). While comparison between preterm neonates and fetuses has limitations, much can be learned from their shared anatomy and physiology, as compared to adult or animal studies.
Differences in early development
Nociceptive pathways in the fetus and preterm infant differ from an older infant and adult in several ways, including (1) the presence of the subplate in the first through third trimesters (22); (2) the lack of descending inhibitory pathways (Descending Pain Modulatory System, DPMS) to mitigate pain until post term development, resulting in a fetus that is “extremely sensitive to painful stimuli [(23), p. 1031, (24)];” (3) large receptive fields, resulting in low pain threshold and poor pain localization (25, 26), such that noxious stimuli to the foot, for example, may be perceived as affecting the entire leg; and (4) increased vulnerability of the developing nervous system to painful procedures experienced early in life, resulting in an increased risk of harmful long-term sequelae such as altered pain sensitivity and neurocognitive development, including impairment in cognition, learning disorders, attentional disorders, behavioral problems, and motor abnormalities (6, 10, 20, 27, 28).
There is noted caution in attributing conscious pain perception to nonverbal populations. Pain is a subjective experience that, without verbal report, can only be inferred from behavioral, physiological, and neural markers (29). However, it is generally acknowledged in terminology and clinical practice, that pain in extremely preterm infants is underassessed and undertreated, with an urgent need to improve pain assessment and management in the youngest premature infants (19, 27, 29–31). A paradox exists not only in the disparate acknowledgment of pain perception by different medical and scientific groups, but also in the disparate use of pain terminology attributed to the nonverbal preterm infant, but not to the age-matched fetus, in whom references to nociception predominate.
An evolving understanding of pain
The field of fetal pain research is complex and multidisciplinary, with unique perspectives offered from a variety of domains (Figure 2). Scientific understanding and evidence-based medicine change over time, particularly regarding the ability of the infant and the fetus to experience pain (Figure 3).
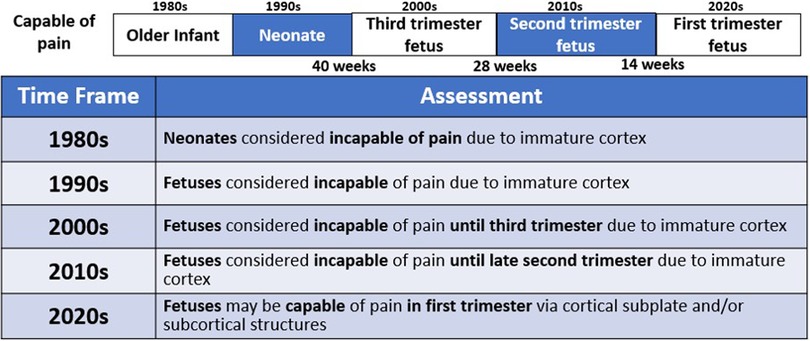
Figure 3. An evolving understanding of pain. Scientific understanding and recognition of pain capacity in the neonate and fetus have evolved over time. In the 1980s and 1990s, medical consensus held that neonates (32, 38) and fetuses (15) lacked the capacity to perceive pain. In the 200s (12), 2010s (34), and 2020s (4, 35, 36), the understanding of fetal pain capacity has shifted.
Until the 1980s, early studies of neurologic development concluded that neonates and young infants lacked the brain structures and connections necessary for pain perception (32, 37, 38). Surgery on infants was frequently performed with paralysis, but without pain management, with catastrophic outcomes (33). During bedside invasive procedures, clinicians suspended consideration of observable pain indicators in view of neurological studies that had concluded that neonatal pain was impossible (37, 38).
In the 1990s, fetuses of all gestations were considered incapable of pain and invasive fetal procedures were conducted without analgesia or anesthesia, until studies demonstrated fetal cardiovascular and hormonal stress responses to invasive procedures (15, 39–41). The field of fetal anesthesiology arguably began in 2001 after research demonstrated that fetal responses to noxious stimuli were attenuated by analgesics (42, 43). Researchers demonstrated that fetal stress responses (cortisol and β-endorphin) do not occur with needling of the non-innervated umbilical cord. However, a significant increase in these stress hormones does occur with needling through the innervated fetal trunk accompanied by vigorous body and breathing movements(15). These physiologic and behavioral responses are nullified by the use of analgesics (42), resulting in a fetus that is “still and appears quiescent and calm [(4), p. 6].” These fetal studies mirrored neonatal studies from the 1980s which (1) showed that analgesics blunt hormonal stress responses to surgery (44); and (2) began the era of neonatal pain management (33, 38).
In the 2000s, studies concluded that fetal pain did not develop until the third trimester (>28 weeks) due to a lack of cortical function (12). In the 2010s, researchers determined that the physiologic capacity to perceive pain developed during the second trimester (14–28 weeks), ranging from before (23, 45) and after thalamocortical connectivity at 24 weeks (34). In the 2020s, researchers suggest that the necessity of the cortex in pain perception may have been overestimated (4, 16, 35, 46). Recent evidence indicates that thalamic projections to the subplate at 12 weeks gestation may be functionally equivalent to thalamocortical connections that develop at 24 weeks gestation (4), signifying that fetal pain mediated by the subplate and subcortical structures may be possible as early as the first trimester (<14 weeks) (Figure 4) (4, 35, 36, 47). Fetal responses to noxious stimuli during clinically-indicated procedures are listed in Table 1. Notably, published prospective studies of fetal responses to noxious stimuli have only been conducted in Europe (15, 39, 40, 42, 55) and South America (16, 57, 58), despite the exponential increase in fetal surgeries, particularly in North America.
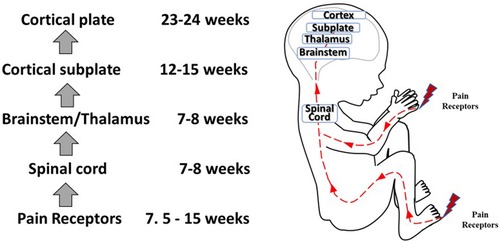
Figure 4. Development of nociceptive pathways. Peripheral pain receptors develop in most areas of the fetus between 7.5–15 weeks gestation (48). Afferents reach the spinal cord (49), the brainstem, and thalamus by 7–8 weeks (50, 51). Thalamic projections to the cortical subplate emerge at 12–15 weeks (14, 34, 52) and to the cortical plate after 23–24 weeks gestation (53).
Pain and nociception: definitions and development
The definition of pain established by the International Association for the Study of Pain (IASP) in 1979 states that pain is an “unpleasant sensory and emotional experience associated with actual or potential tissue damage, or described in terms of such damage (59).” In 2020, revisions to the IASP definition described pain as an “unpleasant sensory and emotional experience associated with, or resembling that associated with, actual or potential tissue damage,” noting that “verbal description is only one of several behaviors to express pain; inability to communicate does not negate the possibility that a human or a nonhuman animal experiences pain [(60), p. 1977].”
As noted by the IASP, in the preverbal population, several behaviors express pain and its unpleasantness. In the extremely preterm neonate (<28 weeks gestation), validated pain assessment tools utilize behavioral indicators of pain, including pain-related facial expressions, body and limb movements, and changes in breathing patterns, as well as physiologic indicators of pain (6). These indicators are likewise present in the fetus in response to noxious stimuli and occur in both the fetus and extremely preterm infant prior to thalamocortical connectivity (Figure 1). The use of age-dependent multimodal pain assessment tools, rather than univariate analysis, increases the sensitivity and specificity of detecting pain in preverbal populations, avoids underestimation of pain, and allows discrimination between responses to noxious and innocuous stimuli (19, 20, 30, 31, 61).
The IASP defines nociception as “the neural process of encoding noxious stimuli,” while the consequences of nociception may include autonomic and behavioral responses, as well as pain perception (62). Nociception, the neural transmission of noxious signals, does not always result in pain sensation, as in cases of general anesthesia or spinal cord transection in which transmission of signals to the brain is blocked or prevented (12, 63). Anesthesiologists note that pain perception typically occurs concomitantly with stress responses, such that pain is unlikely if hormonal stress responses are absent (64). In the fetus, hormonal stress responses are absent to minimal when analgesia is used during invasive procedures, leading researchers to consider the possibility of pain perception in the fetus once noxious-evoked stress responses are evident (Table 1) (42, 55, 65, 66).
Questions remain as to (1) when nociception in the developing fetus triggers a conscious perception of pain; and (2) whether the cortex after 24 weeks or the subplate/subcortical structures prior to 24 weeks are sufficient for the pain experience. Nociceptive pathways, extending from peripheral receptors to the brain emerge during early fetal development, reaching the brainstem, thalamus, and cortical subplate by 12 weeks gestation (Figure 4) and the cortical plate after 24 weeks gestation. Notably, the thalamus relays all afferent sensorimotor information (excluding olfaction) first to the subplate and later to the cortical plate. Peripheral sensory receptors develop in most areas of the fetus between 7.5–14 weeks gestation (48). Figure 5 demonstrates the developing sensory innervation in the human hand from 7 to 11 weeks gestation (67). Peripheral afferents reach the spinal cord (49), brainstem, and thalamus by 7–8 weeks gestation (50, 51). The first thalamic projections to the subplate arrive at 12–15 weeks gestation (34, 52, 68), earlier than the 20–22 weeks cited in older studies (69, 70). After 23–24 weeks gestation, thalamocortical fibers project to the cortical plate, particularly to layer 4 (L4) of the developing somatosensory cortex (12, 53).
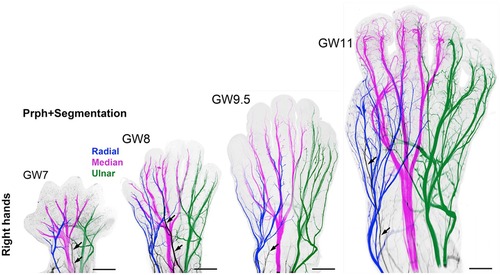
Figure 5. 3D analysis of the sensory innervation of the developing human hand (67). Time series illustrating the developing innervation of sensory nerves of the right hand from GW7–GW11, labeled for the neuron-specific intermediate filament protein peripherin (Prph). Individual segmentation of the radial (blue), median (magenta), and ulnar (green) nerves are shown. The musculocutaneous nerve (arrows) transiently extends into the hand. Prph, neuron-specific intermediate filament protein peripherin; 3D, three-dimensional; GW, gestational week.
The subplate, discovered in 1974, is a transient layer located beneath the cortical plate in the developing cerebral cortex, expanding to four times the thickness of the cortical plate by mid-gestation (71). MRI of the fetal subplate at 19 weeks gestation is shown in Figure 6 (black arrowhead) (72). Neurons from the subplate then migrate to their mature position in the cortex, and the subplate largely disintegrates between 3 months preterm and 3 months post-term (22), while the cortical plate forms layers 2–6 of the developing cortex (73).
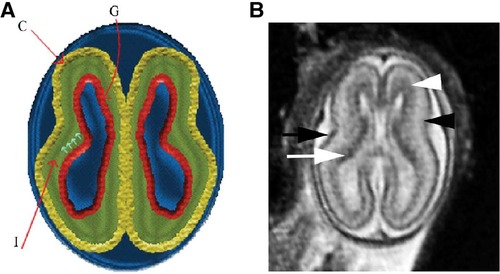
Figure 6. Normal multilayered magnetic resonance imaging (MRI) appearance of fetal brain early in gestation (72). (A) A diagram representing the fetal brain at 19 weeks of gestation shows smooth surface and multilayered appearance of the parenchyma with an inner germinal matrix (G), intermediate layer (I), and a developing cortex (C). The small arrows point to the direction of the migrating neurons from germinal matrix to the developing cortex. (B) Axial balanced fast field echo MR image of a normal brain at 19 weeks of gestation shows a smooth surface and multilayered parenchyma with an inner hypointense germinal matrix (white arrow), an intermediate layer, and an outer hypointense developing cortex (black arrow). Two additional sublayers can be identified: subventricular zone (white arrowhead) and subplate (black arrowhead). Subventricular zone is thick in the frontal region and shows slightly hypointense signal as it contains germinal matrix with increased cell production. The subplate zone appears slightly hyperintense as it has high water content because of extracellular matrix.
Two behavioral responses to noxious stimuli are discussed in more detail below: pain-related facial expressions and limb movements/withdrawal in response to noxious stimulation.
Noxious-Evoked facial expressions and limb movements
Facial expressions are a cornerstone of neonatal pain assessment and are recognized as sensitive and specific predictors of the presence and severity of pain, despite the brainstem origins of these markers (74), particularly when multiple facial movements are assessed. Per the American Academy of Pediatrics, only five neonatal pain scales have been rigorously tested in extremely preterm infants <24–28 weeks gestation; all utilize facial expressions to assess pain (6).
Facial expression-based pain scales, such as the Neonatal Facial Coding System, score 9 distinct facial movements in order to discriminate pain from non-pain states in extremely preterm infants (<28 weeks). Other validated neonatal pain scales use fewer facial expressions but in combination with physiologic, behavioral, and contextual indices. At early gestational ages, multimodal pain assessment tools are critical in differentiating signs and symptoms of pain from those attributable to other causes (6). For example, the Premature Infant Pain Profile-Revised (PIPP-R), a composite behavioral and physiologic pain scale for preterm neonates from 25 weeks gestation, consists of 7 items: 3 measures of facial expressions of pain (brow bulge, nasolabial furrow, and eye squeeze), 2 physiological indices (heart rate and oxygen saturation) and 2 contextual parameters (gestational age and behavioral state), scored at three levels. The range of scores indicates minimal to no pain (<7), moderate pain (7–12), or severe pain (>12) (75). Ranges with cut-off values such as these have proven effective in discriminating pain from non-pain states at early gestational ages (76, 77).
In 2019, Green and colleagues utilized a partial PIPP-R pain scale, (using 3 measures of facial expressions, but not physiologic or contextual parameters) in scoring neonatal responses to a noxious heel lance vs. a control heel lance which did not pierce the skin. The study concluded that facial expressions did not reliably distinguish noxious from non-noxious stimuli in the earliest gestational ages studied (28 weeks) compared to late preterm infants (>33 weeks) (28). A subsequent study in 2022 disputed these findings, noting that the use of multidimensional pain assessment tools (facial expression, brain activity, heart rate, and limb withdrawal) discriminated noxious from non-noxious procedures with an accuracy of 78%–79% in 28–31 week preterm infants. This underscores the need for a multimodal approach to acute pain assessment, including physiologic, behavioral, and contextual parameters, particularly at earlier gestational ages (6).
In 2021 and 2022, Bernardes and colleagues utilized another pediatric pain scale, the Neonatal Facial Coding System, to score 7 different fetal facial expressions in response to anesthetic puncture of the fetal thigh during intrauterine surgery. Blinded investigators analyzed 4D-US images before and after the anesthetic puncture. These researchers concluded that both second and third trimester fetuses respond to noxious stimuli with pain-related facial expressions and that a cutoff value of 5 out of 7 facial expressions effectively discriminated pain from nonpainful auditory stimuli (16, 58). Figures 7, 8 show noxious-evoked facial expressions at 31 weeks and 23 weeks, respectively. Figure 7 highlights the need for modified pain scales at earlier gestational ages. Paradoxically, the Society for Maternal Fetal Medicine and the Royal College of Obstetricians and Gynaecologists state that these fetal facial expressions are reflexes and “do not reflect any experience of pain or suffering (1,B4; 2),” even though they are accepted indicators of pain in age-matched neonates (6).
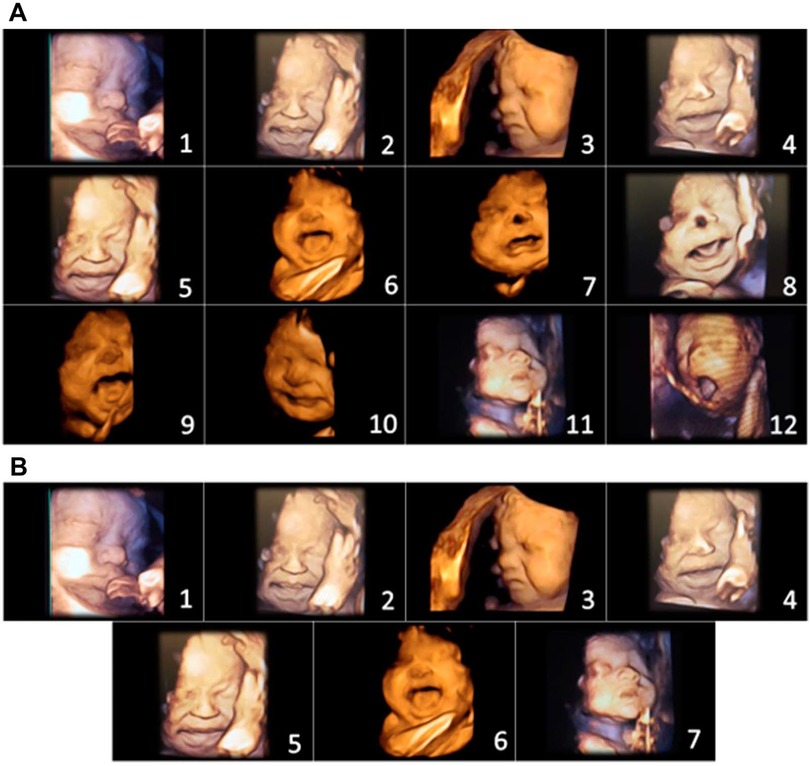
Figure 7. Pain assessment tool for third trimester fetuses during anesthetic injection into the thigh during fetal surgery (58). (A) Initial items from neonatal facial coding system and 2 supplementary items. 1. Brow lowering. 2. Eyes squeezed shut. 3. Deepening of the nasolabial furrow. 4. Open lips. 5. Horizontal mouth stretch. 6. Vertical mouth stretch. 7. Lip purse. 8. Taut tongue. 9. Tongue protrusion. 10. Chin quiver. 11. Neck deflection 12. Yawning. (B) Final items from the Fetal-5 Scale. 1. Brow lowering. 2. Eyes squeezed shut. 3. Deepening of the nasolabial furrow. 4. Open lips. 5. Horizontal mouth stretch. 6. Vertical mouth stretch. 7. Neck deflection.
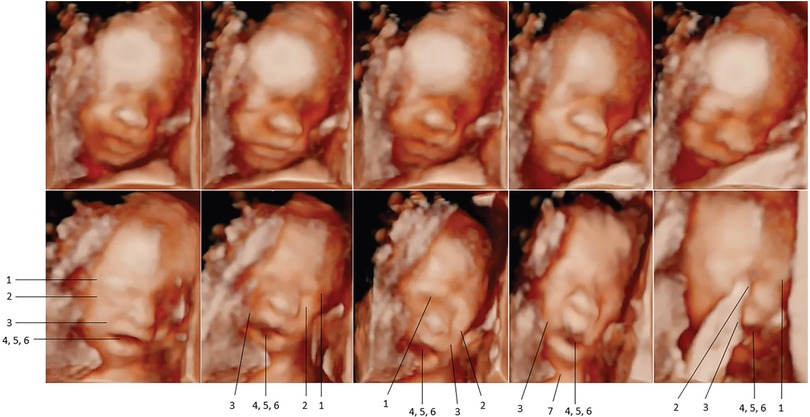
Figure 8. Pain-related facial expressions in 23-week fetus in response to intramuscular injection of fetal thigh, analyzed by blinded investigators (16). Four-dimenstional ultrasound images of fetal facial expressions analyzed before (upper row) and after (lower row) anesthetic puncture, demonstrating the lack of pain-related facial response before and the presence of pain-related facial expressions after the painful stimulus. Seven criteria were considered to be indicative of fetal pain response: 1, brow lowering; 2, eyes tightly shut; 3, deepening of the nasolabial furrow; 4, open lips; 5, vertical mouth stretch; 6, horizontal mouth stretch; and 7, neck extension.
Several neonatal pain scales use limb movements in response to noxious stimuli as a pain indicator. Fetal responses to noxious stimuli also include withdrawal of the limbs (Table 1).
Reflex withdrawal is often dismissed by proponents of cortical necessity as a spinal cord-mediated response, not indicative of supraspinal processing. Recent research regarding reflex limb withdrawal notes that in term infants (with cortical plate connectivity) withdrawal from noxious stimuli strongly correlates with nociceptive brain activity in the cortical plate, indicating that noxious limb withdrawal occurs concomitantly with transmission of pain signals to the brain (78). Further evidence of correlation in preterm infants (who have subplate, but not cortical plate, connectivity) is needed. No known studies have similarly investigated the relationship between noxious-evoked reflex withdrawal in preterm infants and activation of the subplate and subcortical circuitry. Additionally, some researchers discount reflex limb withdrawal as a nondiscriminative pain marker due to lack of differentiation between tactile and painful stimulation in preterm infants (79). However, research by Gursul and colleagues in 2019 found that the magnitude of limb withdrawal is discriminative, with a greater response occurring with noxious stimulation compared to tactile stimulation, as measured by EMG (20). Likewise, more intense noxious stimuli, such as intramuscular injections which are quantified as causing severe pain, trigger higher behavioral reactivity scores and discriminative facial expressions compared to noxious procedures of lower pain intensity such as heel lances (80).
Fetal responses to noxious stimuli
Fetal pain research began in the 1990s, shortly after recognition of neonatal pain, in fetuses as early as 16 weeks gestation. Fetal therapeutic interventions, generally involving puncture of the fetal trunk, provided the occasion to evaluate noxious-evoked responses in the fetus during invasive procedures (Table 1). Fetal responses to noxious stimuli include sizeable biochemical and circulatory hormonal and hemodynamic stress responses by 16–20 weeks gestation, which are blunted by analgesics (42). These responses include significant increases in stress hormones (β-endorphin 590% and cortisol 183%), vigorous body and breathing movements (15), significant elevation of noradrenaline (41), and significant decreases in middle cerebral artery pulsatility index consistent with a brain-sparing response (39, 40). Administration of direct opioid fetal analgesia prevented these responses (42). Fetal stress responses occur independently of maternal responses (55). Two studies documented responses to inadvertent noxious stimulation: (1) contact of the amniocentesis needle with fetal limbs, leading to brisk limb withdrawal (54); and (2) intrauterine surgery inadvertently initiated without fetal anesthesia resulting in bradycardia, which resolved after administration of epinephrine and fetal anesthesia (56). The fetus may mount a similar hormonal stress response under conditions of hypoxemia, such as when the umbilical cord or placenta is strangulated or ablated. During hypoxemic or noxious events, the fetus also may exhibit a freeze and dive response, characterized by inhibition of movements, bradycardia, and redistribution of blood flow to vital organs (18).
Primary studies of fetal responses to noxious stimulation utilizing 4D-US are novel. In 2018, an experimental model to assess and quantify acute pain responses during intrauterine surgery was described for the first time (57). As previously mentioned, Bernardes and colleagues utilized a modified neonatal pain assessment scale, the Neonatal Facial Coding System, to analyze 7 pain-related facial expressions in the fetus during anesthetic puncture of the fetal thigh. Blinded investigators rated fetal facial expressions pre and post-injection in fetuses from 23 weeks gestation. The study concluded that fetuses demonstrate discriminative facial expressions of acute pain following intramuscular injection (Figures 7, 8) (16, 58). Researchers emphasized the need for continuous monitoring of fetal activity and responses to invasive fetal procedures to better assess and treat procedural and post-procedural pain (3).
Though utilization of analgesia and anesthesia during fetal surgery began in the early 1980s and an anesthesiology and fetal therapy consensus statement in 2021 recommends administration of fetal anesthesia in all invasive maternal-fetal procedures (10), optimal anesthetic techniques and dosages continue to evolve. In 2022, a systematic review of anesthesia for fetal operative procedures concluded that several anesthesia approaches are utilized with no standardized protocols or dosage regimens based on the type of fetal procedure. A lack of standardized intraoperative fetal monitoring was also noted (81).
Anesthetic management during invasive fetal procedures employs a range of modalities with varying degrees of placental transfer and safety profiles (10). The use of maternal general anesthesia, particularly during open fetal surgery, allows for the transfer of anesthetics to the placental circulation, however, the need for higher doses of volatile anesthetic agents can have a substantial adverse impact on fetal hemodynamics and prolonged use raises concern of fetal neurotoxicity. Supplemental maternal intravenous anesthesia to reduce the dosage of volatile agents used during general anesthesia may lower this risk and allow adequate transplacental transfer (82). However, maternal anesthesia via local anesthetic infiltration or neuraxial blockade (ie. epidural anesthesia) may be preferred during fetal interventions. Though some transplacental transfer may occur, direct fetal anesthesia via intramuscular or intravenous administration is recommended in order to reliably blunt the fetal stress response to invasive procedures and to facilitate fetal pain relief and appropriate fetal positioning (10, 83, 84). Direct fetal anesthesia generally includes a cocktail of an opioid analgesic (i.e.. fentanyl), a nondepolarizing muscle relaxant (i.e., rocuronium) to achieve fetal immobility, and an anticholinergic agent (i.e., atropine) to minimize the risk of fetal bradycardia and is recommended for all surgeries on innervated tissue (85).
Noxious-evoked brain activity
The use of neural markers in the preterm neonate has the potential to link functional neuroimaging to clinically observable pain-related measures to increase the sensitivity and specificity of pain assessment tools and to help discriminate pain from non-pain states. Modalities such as electroencephalography (EEG), functional magnetic resonance imaging (fMRI), magnetoencephalography (MEG), and near-infrared spectroscopy (NIRS) have been studied to determine brain activity at rest and during noxious and non-noxious stimulation in early neurologic development (19, 20, 86–88).
Neurological pain signatures (NPS) of noxious-evoked brain activity hold promise for the future, though numerous technical, ethical, and design issues currently preclude the diagnostic utility of such neural measures of pain (1, 89, 90). The use of noxious-specific brain activity, as a surrogate measure of pain, is particularly challenging for several reasons: (1) brain networks vary by age, with a subplate-dominant network in the first to third trimesters and a cortical-dominant network post-term (22), leading to differing patterns of neural activity by developmental stage; (2) inferring pain perception based on functional activity or inactivity within brain regions is difficult (27); (3) pain is a dynamic process, with neural responses affected by a variety of factors, including physiologic stress and gestational age (20, 89). Researchers conclude it is highly unlikely that a neural marker will capture the dynamic nature of pain in its entirety (19, 27, 89). Instead, clinicians and neuroscientists recognize the necessity of composite, multimodal pain assessment tools to increase predictive value, potentially including developmentally-specific neural measures, in preventing the underdiagnosis and undertreatment of pain (29) and in discriminating between pain and non-pain states (20, 31, 61, 91).
The emergence of consciousness
Pain perception depends not only on transmission of nociceptive signals to the brain but also on the level of consciousness of the fetus or neonate. Definitions of consciousness, however, are diverse and elusive. Various definitions require (1) extrauterine life (92); (2) the presence of a longer memory span associated with second-order learning (88); (3) thalamocortical connections confirmed via electrophysiologic studies (93); or (4) body awareness after 25 weeks gestation (94). Notwithstanding, consciousness may be defined more fundamentally as the state of wakefulness and awareness (95).
Animal research by Mellor and colleagues in the 2000s suggested that the fetus is not awake until after birth due to the sedative effects of endocrine neuroinhibitors in utero (18, 34, 92). Recent research, however, has discredited this hypothesis, noting that (1) human endocrine neuroinhibitors do not confer any anesthetic effect in the human fetus, but only with artificial injection at high dosages; and (2) the fetus is arousable and responsive to external stimuli (96). Fetal studies have also demonstrated arousability and responsiveness (Table 1 and Figures 7, 8), leading to questions about the onset of fetal awareness.
Conscious awareness is categorized, according to a stepwise developmental process beginning with basic awareness of the external environment, followed by awareness of one's body, and finally, higher-order internal awareness of oneself (such as mind-wandering or daydreaming, associated with the Default Mode Network) (97). The emergence of consciousness occurs along a continuum (27) and has been likened to a dimmer switch beginning with a minimum basic consciousness, mediated by subcortical structures (98, 99) and possibly the subplate (4), to higher order consciousness associated with cortical processing and decision-making (Figure 9) (98, 100). Neuroscientists hold that basic conscious awareness requires the subjective ability to evaluate the environment and form coordinated responses (101) and may be demonstrated via action planning, learning, and purposeful movement (100).
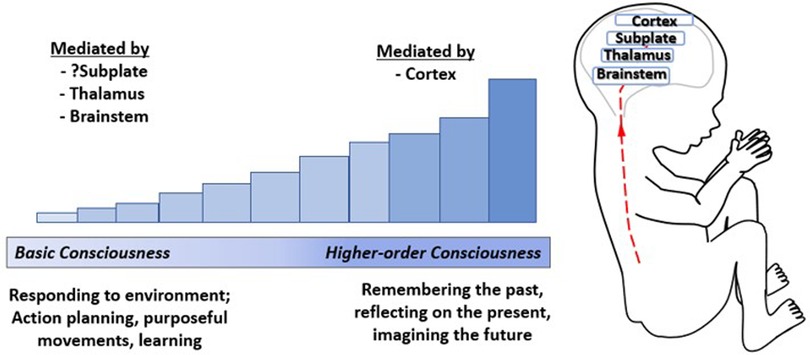
Figure 9. The emergence of consciousness. Consciousness has been likened to a dimmer switch beginning with a minimum basic level of consciousness mediated by the brainstem (100), thalamus (99), and possibly the cortical subplate (4) increasing to higher-order consciousness mediated by the cortex (100). Basic consciousness requires responsiveness to the environment (101), demonstrated by action planning, purposeful movements, and leaming (100), while higher-order consciousness involves memory, self-reflection, and imagining the future (98).
Fetal neurobehavioral studies analyze observable fetal movements via four-dimensional ultrasound or other diagnostic modalities to assess fetal neurologic development (102, 103). Such studies indicate directed actions, motor planning, and learning prior to cortical development. Evidence includes (1) goal-oriented hand movements by 13 weeks gestation (94); (2) differential velocities of fetal hand movements toward the sensitive eye and mouth regions by 22 weeks gestation (104); (3) in twin gestations, evidence of socially-aware motor planning of fetal hand movements toward the co-twin by 14 weeks gestation (105). These studies indicate early action planning, learning, and the emergence of a basic minimum level of consciousness in the fetus by 13–14 weeks gestation. The minimum conscious level marks the starting point of a consciousness that is unreflective, focused on the present, and without a requirement for memory or self-reflection. With further brain development, complex levels of consciousness emerge (106).
The following sections discuss the evidence for and against the hypotheses of cortical necessity and subplate modulation. Tables 2, 3 summarize this evidence.
The hypothesis of cortical necessity
According to the hypothesis of cortical necessity, there is no capacity to experience pain prior to 24–28 weeks gestation when connections from the thalamus reach the cortical plate (1, 2, 11). Evidence proposed for cortical necessity (Table 2) includes (1) neuroanatomic structural evidence of thalamocortical connections emerging at 24–28 weeks gestation (1); (2) functional evidence of noxious-evoked brain activity in the cortical plate, after 24–28 weeks gestation (1); (3) the presence of resting state networks (spontaneous neural activity) involving the cortex after 28 weeks gestation (2); (4) a reported lack of discriminative facial responses between noxious and non-noxious stimuli before 33 weeks gestation (2); (5) a reported lack of distinction between innocuous touch and noxious-evoked withdrawal reflexes before 35 weeks gestation (2); and (6) case studies of adult post-lobotomy patients dating from the 1950s, some of whom experienced indifference to pain (1, 107).
First, thalamic connectivity to the somatosensory cortex at 24–28 weeks gestation is widely acknowledged. Proponents of cortical necessity state that the cortex is the sole structure that can interpret stimuli as painful; therefore, prior to its development, pain experience is impossible (1, 11). This circular argumentation has been challenged for overreliance on neuroanatomical hypotheses, as was done in the era of untreated neonatal pain, rather than correlation with clinical behavior and other pain indicators which occur before 24–28 weeks gestation. Other researchers note that the same indicators of pain that are present after thalamocortical connectivity are already present, developing, and maturing prior to 24 weeks gestation. No observable behavioral or physiologic indicators at 24–28 weeks gestation have been identified which demonstrate the impact from these connections, suggesting that pre-existing neural pathways mediate pain perception prior to 24–28 weeks.
Second, noxious stimuli evoke cortical brain activation patterns, or a neurological pain signature (NPS), after 28 weeks gestation. The neurological pain signature, after thalamocortical connectivity, involves the primary and secondary somatosensory, the prefrontal cortex, the anterior cingulate cortex, the amygdala, and the insula. Such cortical activation is present in adults and term infants (29), but is generally absent in extremely preterm infants <28 weeks gestation (91, 108). This is cited as evidence of cortical necessity for pain perception. However, such studies involve testing for a cortical network that does not yet exist in the preterm neonate or fetus <24–28 weeks gestation (Figure 10). Earlier developing subplate circuitry, rather than cortical circuitry, is the predominant network during this time period (22).
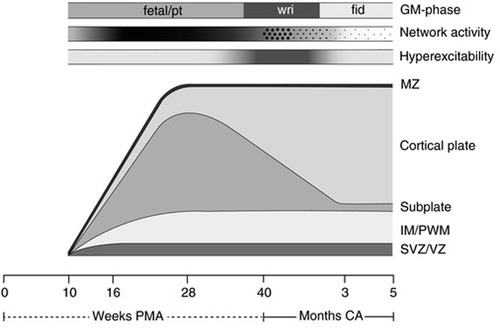
Figure 10. Schematic presentation of the processes underlying the subplate and cortical plate modulation hypothesis (5). The bottom line denotes age, first in weeks PMA, after term (40 weeks) in months (corrected age). Above the age line the developmental changes in the human cortex are depicted. SVZ/VZ represents the subventricular and ventricular zones where the neurons and glial cells are generated; IM/PWM denotes the intermediate zone that gradually develops into the periventricular white matter; MZ is the marginal zone. The following three timelines represent from bottom to top: the hyperexcitability of the nervous system, in which the intensity of the grey shading represents the degree of hyperexcitability; the cortical network activity that emerges across the brain from 9 to 10 weeks PMA, this gradually increases (indicated by increasing shading) to be full-blown present (in the subplate) at mid-fetal age, before moving from global and widespread activity to local and limited activity to local and limited activity (“sparsification”, indicated by the diminution of the dots); on top the developmental changes in general movements. GM, general movements; PMA, postmenstrual age; CA, corrected age; pt, preterm.
Third, resting state networks (RSNs), measurable by functional MRI, are defined as a set of brain regions that show functional connectivity during task-free spontaneous brain activity (109). In 2010, Doria et al. analyzed fMRI of preterm infants to determine when adult-like RSNs could first be detected. At 30 weeks postmenstrual age, fragments of adult-like cortical networks are detectable, increasing to an adult-like repertoire at term age (110). Adult-like RSNs thus emerge during the time period when thalamocortical connectivity is established, subplate circuitry regresses, and cortical circuitry begins to predominate (Figure 10). In 2018, van den Heuvel et al. analyzed fetal resting-state MRI in third trimester fetuses (29–37 weeks gestation), likewise identifying regions or hubs of cortical involvement after thalamocortical connectivity (111). Notably, these studies (1) analyzed fetuses and neonates after 28 weeks, during the phase of cortical plate dominance (Figure 10); and (2) utilized adult resting state networks as the basis of comparison. Brain networks vary by age and may not be comparable to adult networks (Figure 10). At earlier gestations (<28 weeks), fetal resting state fMRI studies indicate that the center of gravity of most activations is located in the subplate zone (13).
Fourth, some researchers suggest that preterm infants and fetuses are incapable of experiencing pain until after 28–33 weeks gestation as they may display nondiscriminative pain-related facial expressions to both noxious and innocuous stimuli at earlier gestations (2). This conclusion is based on a 2019 study of preterm infants, 28–42 weeks postmenstrual age, which utilized a modified PIPP-R pain scale to evaluate 3 facial responses to a control heel lance (which did not pierce the skin) compared to a noxious heel lance used to obtain blood (28). Facial responses were analyzed using a partial PIPP-R pain scale on a 9-point scoring system, compared to the 21-point scoring system in the validated PIPP-R (77). Overall, 24% of infants displayed facial expressions to the non-noxious heel lance, while 69% exhibited facial expressions to the noxious heel lance. Preterm infants <33 weeks gestation were more likely to exhibit pain-related facial expressions to both the control heel lance and the noxious heel lance than those after 33 weeks gestation. Additionally, in 42 of the preterm neonates, EEG responses to both the noxious and non-noxious heel lance were measured, demonstrating (1) nondiscriminative delta brush activity at earlier gestations (<33 weeks), often in response to both noxious and non-noxious heel lance; and (2) increasingly discriminative sensory-evoked potentials and noxious-specific brain activity at later gestations (>34 weeks). Based on this study, some investigators suggest that a sense of pain distinct from benign tactile stimulation does not develop until 32–33 weeks gestation (2).
This conclusion is controversial for the following reasons: (1) the use of 3 facial expressions alone is insufficient to assess pain in early gestations. Rather, per the American Academy of Pediatrics, validated pain scales require assessment of 7–9 facial expressions or multidimensional scoring of behavioral, physiological, and contextual factors to discriminate pain from non-pain states, particularly in extreme prematurity. Increased sensitivity to noxious and non-noxious stimulation at early gestations may be due to sensitization from prior pain experiences (i.e., repeated heel lances), lack of descending inhibitory pathways, and large receptive fields, resulting in lower pain thresholds and increased excitability (6). (2) A subsequent study in 2022 of neonates 28–40 weeks gestation concluded that the use of multimodal pain assessment tools (facial expression, brain activity, heart rate, and limb withdrawal) discriminates noxious from non-noxious procedures in early preterm infants with an accuracy of 78%–79% at 28–31 weeks (61); (3) noxious-evoked brain activity, whether delta brushes or noxious-specific cortical activity, is widely regarded as an unreliable univariate measure of pain, but may hold promise as part of a multimodal pain assessment strategy to discriminate pain from non-pain states (20, 31, 61, 91); (4) It is noteworthy that a heel lancet device was used to test both noxious and non-noxious stimulation. During the non-noxious heel lance, infants experienced not only the tactile stimulation of the lancet device against the heel but also the audible click associated with blade release away from the skin. This raises the question as to whether this method may have triggered anticipatory reactions, particularly in infants previously exposed and perhaps sensitized to noxious heel lances.
Fifth, some researchers report a lack of distinction between noxious and non-noxious evoked limb withdrawal in early preterm infants <35 weeks gestation. In a study by Cornelissen et al., a heel lancet device was also used to deliver both noxious and non-noxious stimuli (see description above), while limb withdrawal responses of the biceps femoris were measured via EMG (79). 100% of the infants (30–42 weeks gestation) demonstrated robust withdrawal to the noxious heel lance. However, 29% of the preterm neonates exhibited limb withdrawal in response to the non-noxious heel lance that was indistinguishable in magnitude from noxious-evoked withdrawal. 40% of term neonates likewise responded with limb withdrawal to non-noxious stimulation, but with a significantly smaller magnitude on EMG. The study concludes that flexion reflexes, which may be interpreted as signs of pain in adults, may not reflect pain perception before 35 weeks gestation. Some researchers cite this as evidence that pain perception is unlikely until at least the third trimester (2), however this conclusion is likewise disputed for the use of limb withdrawal as a univariate measure of pain. As previously discussed, multimodal pain assessment tools are necessary to differentiate pain from non-pain states, particularly at early gestational ages.
Finally, case studies of adult post-lobotomy patients dating from the 1950s, some of whom experienced indifference to pain (107), have been cited by the Society for Maternal Fetal Medicine as evidence of cortical necessity for pain perception (1). However, it is unclear how to correlate variably controlled case studies of some adult post-lobotomy patients, in the era before neuroimaging, to the unique structures and mechanisms used for pain processing in fetal and neonatal life (112).
Researchers observe that the primary purpose of acute pain perception is a behavioral drive to survive. This drive serves as a protective mechanism which seeks to remove an individual from a damaging stimulus and is critical for the survival of the organism. As such, scientists expect neural circuitry responsible for this drive to be in phylogenetically older regions of the brain, which appear earlier in development (113). Such regions include the subplate and subcortical structures such as the thalamus and brainstem. Cortical regions, which are phylogenetically newer, may be involved in the processing and regulation of pain, rather than pain perception itself (114, 115).
Evidence for the role of cortical regions in the processing and modulation of pain, rather than pain perception per se includes (1) the presence of validated pain indicators prior to connections to the cortex (6); (2) several clinical cases of preserved pain perception despite lesions of critical regions including the insula, anterior cingulate, and even the entire contralateral hemisphere, confirmed by neuroimaging (114–116); (3) no alteration of pain perception with stimulation or ablation of the somatosensory cortex, while altered pain perception occurs with stimulation or ablation of the thalamus (117); (4) cases of infants and children with hydranencephaly (congenitally decorticate) who demonstrate pain-related responses as well as elements of consciousness (118–120); notably, 96% of parents of children with hydranencephaly stated that their child can feel pain (119); (5) research in term infants showing the top-down inhibitory effects of the prefrontal cortex, anterior cingulate cortex, and the anterior insula, as part of the DPMS, in dampening the pain experience and modifying pain behavior (29). This indicates that noxious-evoked cortical activity may represent modulation and regulation of pain rather than perception of pain itself.
The hypothesis of cortical necessity has also been challenged for (1) disregarding clinical practice in neonatology and anesthesiology in which pain is acknowledged and treated in neonates prior to thalamocortical connectivity (6); and (2) relying on neuroanatomical studies from post-mortem fetuses without functional correlation to fetal behavior in utero (93, 121).
The presence of pain in the preterm infant prior to thalamocortical connectivity has been widely acknowledged for decades and challenges the assumption of cortical necessity (Table 2). Per the American Academy of Pediatrics, prevention and management of pain in the earliest preterm infants is the standard of care, not only to prevent short- and long-term adverse consequences but to alleviate pain itself (6). In one survey of NICU clinicians, all agreed that preterm babies born at the edge of viability (21–23 weeks) are able to perceive pain and demonstrate the same signs of pain that older patients demonstrate when they are in pain (122). Neonatal pain researchers acknowledge the urgent need for improved pain assessment and management in the youngest premature infants to prevent underdiagnosis and undertreatment of pain (19, 27, 29–31). This suggests that (1) the hypothesis of cortical necessity is not congruent with clinical practice; and (2) pain perception may be mediated by functional and well-developed pathways prior to thalamocortical connections.
The subplate modulation hypothesis
The cortical subplate forms part of the transitional nociceptive circuitry during fetal life and is present in all placental animals to varying degrees. The subplate is conspicuously present in primates, particularly in humans, during early neurological development (5). Initially, the subplate was thought to be the structural analog of a warehouse, filled with neurons awaiting migration to the cortical plate (73). More recently, evidence suggests that subplate circuitry is more akin to a power station than a warehouse, forming a functionally responsive network of early cortical activity in the first through third trimesters (5, 73, 123, 124). The subplate emerges at 8 weeks gestation, reaching maximum thickness around 28 weeks (124). When thalamic fibers reach the cortical plate at 24 weeks, the gradual transition between subplate circuitry and cortical circuitry begins, reaching completion in the post-term period (5, 125). This results in two overlapping developmental phases (Figure 10) characterized by:
(a) the transient cortical subplate phase, ending at 3 months post-term when the permanent circuitries in the primary motor, somatosensory and visual cortices have replaced the subplate; and subsequently,
(b) the phase in which the permanent circuitries dominate [(22), p. 276].
This has been called a transitional pain circuitry of the fetus and neonate, similar to the transitional fetal and neonatal circulatory system (126). Before 30–32 weeks, electrical activity in the brain is centered around the subplate and is marked by the presence of delta brushes and spontaneous activity transients on EEG (28, 127, 128).
Evidence supporting the subplate as an active and functional precursor of the cortex includes modulatory activity of both sensorimotor and somatosensory functions prior to thalamic innervation of the cortical plate: (1) neurobehavioral studies indicate subplate modulation of fetal motor activity beginning at 9–10 weeks gestation (129); (2) research demonstrates subplate activation in response to sensory stimuli (130); (3) pain indicators prior to 24 weeks gestation suggest the pre-existence of pathways of pain perception (Table 3).
First, fetal neurobehavioral research indicates subplate modulation of sensorimotor activity during early gestation. Subplate modulation in the fetal and preterm periods followed by cortical plate modulation in the post-term period has been observed in the study of general movements (GMs), the most common motor behavior of the fetus and neonate (5, 129). Research indicates that subplate modulation of GMs predominates from 9 to 34 weeks gestation until the immature but progressively developing cortical plate circuitry takes over in the post-term period. The dissolution of the subplate at 3 months post-term marks the completed transition to permanent cortical plate circuitry. In the post-term period, when cortical activity in the primary sensorimotor cortex shifts from subplate to cortical plate, observable changes in motor behavior occur with the emergence of so-called fidgety movements (Figure 10). This evidence indicates that the sensorimotor region of the subplate forms an active and functioning cortical network beginning as early as 9–10 weeks gestation.
Second, in the somatosensory region of the subplate, animal research in ferrets indicates (1) subplate neurons are the first cortical neurons to respond to auditory stimuli; (2) the subplate shows topographic organization, comparable to the cortical plate; that is to say, sensory stimulation of a particular area evokes changes in predictable anatomic location in the subplate (130). Preterm human infants, likewise, show early responsiveness to external stimulation with light flashes, demonstrating evoked delta brush responses associated with subplate circuitry (131). This indicates that both the sensorimotor and somatosensory regions of the subplate are responsive and active during this developmental phase. Functional magnetic resonance imaging of the fetal subplate in utero corroborates these findings. At 20 weeks gestation (the earliest gestation studied), the brain region with the highest activity is the cortical subplate (5, 13).
Finally, fetal and preterm neonatal responses to noxious stimuli predate thalamocortical connections at 24 weeks gestation (Figure 1). The presence of an early pain circuitry is highly suggested by the occurrence of the same pain-related responses before and after 24 weeks gestation. These indicators include facial expressions of pain, vigorous body movements, and physiologic and hormonal stress responses that are mitigated by analgesics. The subplate forms the most significant functional network during the preterm age, is functionally active in the sensorimotor and somatosensory regions of the subplate (5, 130), and has been implicated in early responses to painful stimulation (14).
The predominant criticism of the subplate modulation hypothesis is the limited direct evidence that the subplate and subcortical structures modulate pain perception in addition to modulating other sensorimotor or somatosensory functions (12). It is accurate that noxious-induced testing of the human subplate during corticogenesis is limited by ethical, technical, and legal considerations (132). If the subplate is an active and functional precursor of the cortex corresponding in topography, then subplate and subcortical modulation of other somatosensory functions, such as pain perception, may be anticipated. Additional research opportunities may be possible during therapeutically-indicated noxious procedures in extremely preterm infants and during intrauterine fetal surgery.
Discussion
While the acknowledgment of pain perception in the fetus prior to thalamocortical connectivity at 24–28 WGA is controversial, the acknowledgment of pain in the age-matched preterm infant is not. In clinical practice, the extremely preterm infant is the focus of extensive research efforts to better assess and treat pain in the neonatal intensive care unit. Numerous studies acknowledge that pain in the preterm infant is underrecognized and undertreated with focused research on ways to better ameliorate pain in this population (19, 27, 29–31).
Research and clinical practice indicate that fetal pain perception is possible prior to thalamocortical connectivity via pre-existing pathways of pain perception. Some researchers argue that neither consciousness nor pain capacity exists prior to 24–28 weeks gestation (1, 2, 11). Advances in the fields of neonatology, fetal surgery, fetal anesthesiology, and fetal neurobehavior make this viewpoint no longer appropriate. However, a fetal pain paradox continues to exist in which pain-related responses in the extremely preterm infant are regarded as evidence of pain, while the same responses to noxious stimuli in a similarly-aged fetus are dismissed as reflexive responses, not indicative of a pain experience.
Determining the exact onset of pain perception in the fetus is challenging. Fetal responses to therapeutically indicated noxious procedures are evident by 15–16 weeks gestation and are alleviated by analgesics. Prior to this time frame, published research is lacking. Researchers acknowledge that “where it is uncertain whether harm may result, it is advisable to apply a precautionary principle that errs on the side of caution to prevent potential harms, even if scientific uncertainty exists about their extent [(28), p. 498].” Certainly, such a viewpoint is prudent to avoid the errors of the past.
With the exponential increase in invasive fetal procedures, particularly in North America, it is surprising that more studies of fetal responses to noxious stimuli are not available. This is a field worthy of further investigation, as we will not see what we do not look for. A systematic review of 165 fetal surgical studies with over 5,000 fetal surgical procedures observed that none of these studies fully analyzed fetal reactions to tissue-damaging procedures via intraoperative fetal monitoring, such as fetal movements, fetal hormonal responses, and heart rate variability (81). A lack of standardized dosage regimens of direct fetal anesthesia was also noted, with dosages of opioids varying by hundreds of micrograms per kilogram, raising the question of inadequate analgesia. This is noteworthy, as there is increasing evidence that painful procedures early in life are instrumentally harmful in altering pain sensitivity and cognition later in life (28).
Implications of fetal pain perception at earlier gestational ages include the need for research in fetal pain assessment and management strategies to ensure adequate procedural and post-procedural pain control (3, 57, 58) and to prevent adverse short- and long-term sequelae, including the potential for preterm labor (1, 18). An ethical obligation also exists to prevent, mitigate, and treat pain whenever it can be anticipated. Finally, informed consent regarding fetal pain capacity is an important ethical consideration. The informed consent process should distinguish between the surgical procedures, anesthesia, and analgesia utilized for the pregnant woman and those utilized for the fetus. Reports over the past 20 years indicate that the potential for pain perception in the fetus is a concern for women and families (34, 122, 133), which has implications for fetal surgery as well as abortion.
Author contributions
The author is the sole writer of and contributor to this manuscript.
Conflict of interest
The author declare that the research was conducted in the absence of any commercial or financial relationships that could be construed as a potential conflict of interest.
Publisher's note
All claims expressed in this article are solely those of the authors and do not necessarily represent those of their affiliated organizations, or those of the publisher, the editors and the reviewers. Any product that may be evaluated in this article, or claim that may be made by its manufacturer, is not guaranteed or endorsed by the publisher.
References
1. Society for Maternal-Fetal Medicine (SMFM), Society of Family Planning (SFP), Norton ME, Cassidy A, Ralston SJ, Chatterjee D, et al. Society for maternal-fetal medicine consult series #59: the use of analgesia and anesthesia for maternal-fetal procedures. Am J Obstet Gynecol. (2021) 225(6):B2–B8. doi: 10.1016/j.ajog.2021.08.031
2. Royal College of Obstetricians and Gynaecologists. RCOG Fetal Awareness Evidence Review (2022). Available from: https://www.rcog.org.uk/guidance/browse-all-guidance/other-guidelines-and-reports/fetal-awareness-updated-review-of-research-and-recommendations-for-practice/ (Accessed January 20, 2023).
3. Bellieni CV. Foetal pain and anaesthesia during prenatal surgery. Clin Exp Obstet Gynecol. (2022) 49(4):79. doi: 10.31083/j.ceog4904079
4. Derbyshire SW, Bockmann JC. Reconsidering fetal pain. J Med Ethics. (2020) 46(1):3–6. doi: 10.1136/medethics-2019-105701
5. Hadders-Algra M. Neural substrate and clinical significance of general movements: an update. Dev Med Child Neurol. (2018) 60(1):39–46. doi: 10.1111/dmcn.13540. Figure 3 relabeled as Figure 10. Reproduced by permission from John Wiley and Sons.28832987
6. American Academy of Pediatrics. Committee on Fetus and Newborn and Section on Anesthesiology and Pain Medicine. Prevention and management of procedural pain in the neonate: an update. Pediatrics. (2016) 137(2):e20154271. doi: 10.1542/peds.2015-4271
7. Ruano R, Yoshisaki CT, Salustiano EMA, Giron AM, Srougi M, Zugaib M. Early fetal cystoscopy for first-trimester severe megacystis. Ultrasound Obstet Gynecol. (2011) 37(6):696–701. doi: 10.1002/uog.8963
8. Sananes N, Favre R, Koh CJ, Zaloszyc A, Braun MC, Roth DR, et al. Urological fistulas after fetal cystoscopic laser ablation of posterior urethral valves: surgical technical aspects. Ultrasound Obstet Gynecol. (2015) 45(2):183–9. doi: 10.1002/uog.13405
9. Gupta R, Wimalasundera R, Moore P. Anaesthetic considerations in fetal therapy. In: Goudra B, Singh P, Green M, editors. Anaesthesia for uncommon and emerging procedures. 1st ed. Cham: Springer (2021). p. 277–88.
10. Chatterjee D, Arendt KW, Moldenhauer JS, Olutoye OA, Parikh JM, Tran KM, et al. Anesthesia for maternal-fetal interventions: a consensus statement from the American Society of Anesthesiologists Committees on Obstetric and Pediatric Anesthesiology and the North American Fetal Therapy Network. Anesth Analg. (2021) 132(4):1164–73. doi: 10.1213/ANE.0000000000005177
11. American College of Obstetricians and Gynecologists. Facts are Important: Gestational Development and Capacity for Pain. (2022). Available at: https://www.acog.org/en/advocacy/facts-are-important/gestational-development-capacity-for-pain (Accessed January 20, 2023).
12. Lee SJ, Ralston HJP, Drey EA, Partridge JC, Rosen MA. Fetal pain: a systematic multidisciplinary review of the evidence. JAMA. (2005) 294(8):947–54. doi: 10.1001/jama.294.8.947
13. Schöpf V, Kasprian G, Brugger PC, Prayer D. Watching the fetal brain at « rest ». Int J Dev Neurosci. (2012) 30(1):11–7. doi: 10.1016/j.ijdevneu.2011.10.006
14. Kostović I, Jovanov-Milosević N. Subplate zone of the human brain: historical perspective and new concepts. Coll Antropol. (2008) 32(Suppl 1):3–8. 18405051.
15. Giannakoulopoulos X, Sepulveda W, Kourtis P, Glover V, Fisk NM. Fetal plasma cortisol and beta-endorphin response to intrauterine needling. Lancet. (1994) 344(8915):77–81. doi: 10.1016/s0140-6736(94)91279-3
16. Bernardes LS, Rosa AS, Carvalho MA, Ottolia J, Rubloski JM, Castro D, et al. Facial expressions of acute pain in 23-week fetus. Ultrasound Obstet Gynecol. (2022) 59(3):394–5. doi: 10.1002/uog.23709. Figure 1 relabeled as Figure 8. Reproduced by permission from John Wiley and Sons34129710
17. Jones L, Fabrizi L, Laudiano-Dray M, Whitehead K, Meek J, Verriotis M, et al. Nociceptive cortical activity is dissociated from nociceptive behavior in newborn human infants under stress. Curr Biol. (2017) 27(24):3846–51.e3. doi: 10.1016/j.cub.2017.10.063
18. Lagercrantz H. Chapter 4: fetal moving and sensing. In: Lagercrantz H, editor. Infant brain development: Formation of the mind and the emergence of consciousness. Cham: Springer International Publishing (2016). p. 43–52. doi: 10.1007/978-3-319-44845-9_8
19. Tracey I, Woolf CJ, Andrews NA. Composite pain biomarker signatures for objective assessment and effective treatment. Neuron. (2019) 101(5):783–800. doi: 10.1016/j.neuron.2019.02.019
20. Gursul D, Hartley C, Slater R. Nociception and the neonatal brain. Semin Fetal Neonatal Med. (2019) 24(4):101016. doi: 10.1016/j.siny.2019.05.008
21. Slater R, Fabrizi L, Worley A, Meek J, Boyd S, Fitzgerald M. Premature infants display increased noxious-evoked neuronal activity in the brain compared to healthy age-matched term-born infants. Neuroimage. (2010) 52(2):583–9. doi: 10.1016/j.neuroimage.2010.04.253
22. Hadders-Algra M. Early human brain development: starring the subplate. Neurosci Biobehav Rev. (2018) 92:276–90. doi: 10.1016/j.neubiorev.2018.06.017
23. Sekulic S, Gebauer-Bukurov K, Cvijanovic M, Kopitovic A, Ilic D, Petrovic D, et al. Appearance of fetal pain could be associated with maturation of the mesodiencephalic structures. J Pain Res. (2016) 9:1031–8. doi: 10.2147/JPR.S117959
24. Goksan S, Baxter L, Moultrie F, Duff E, Hathway G, Hartley C, et al. The influence of the descending pain modulatory system on infant pain-related brain activity. Elife. (2018) 7:e37125. doi: 10.7554/eLife.37125
25. Hatfield LA. Neonatal pain: what’s age got to do with it? Surg Neurol Int. (2014) 5(Suppl 13):S479–89. doi: 10.4103/2152-7806.144630
26. Jones L, Verriotis M, Cooper RJ, Laudiano-Dray MP, Rupawala M, Meek J, et al. Widespread nociceptive maps in the human neonatal somatosensory cortex. Elife. (2022) 11:e71655. doi: 10.7554/eLife.71655
27. Duff EP, Moultrie F, van der Vaart M, Goksan S, Abos A, Fitzgibbon SP, et al. Inferring pain experience in infants using quantitative whole-brain functional MRI signatures: a cross-sectional, observational study. Lancet Digit Health. (2020) 2(9):e458–67. doi: 10.1016/S2589-7500(20)30168-0
28. Green G, Hartley C, Hoskin A, Duff E, Shriver A, Wilkinson D, et al. Behavioural discrimination of noxious stimuli in infants is dependent on brain maturation. Pain. (2019) 160(2):493–500. doi: 10.1097/j.pain.0000000000001425
29. Goksan S, Hartley C, Emery F, Cockrill N, Poorun R, Moultrie F, et al. fMRI reveals neural activity overlap between adult and infant pain. Elife. (2015) 4:e06356. doi: 10.7554/eLife.06356
30. Moultrie F, Slater R, Hartley C. Improving the treatment of infant pain. Curr Opin Support Palliat Care. (2017) 11(2):112–7. doi: 10.1097/SPC.0000000000000270
31. van der Vaart M, Duff E, Raafat N, Rogers R, Hartley C, Slater R. Multimodal pain assessment improves discrimination between noxious and non-noxious stimuli in infants. Paediatr Neonatal Pain. (2019) 1(1):21–30. doi: 10.1002/pne2.12007
32. Anand KJ, Hickey PR. Pain and its effects in the human neonate and fetus. N Engl J Med. (1987) 317(21):1321–9. doi: 10.1056/NEJM198711193172105
33. Rogers MC. Do the right thing. Pain relief in infants and children. N Engl J Med. (1992) 326(1):55–6. doi: 10.1056/NEJM199201023260109
34. Royal College of Obstetricians and Gynaecologists. Fetal Awareness: Review of Research and Recommendations for Practice. (2010). Available from: https://www.rcog.org.uk/guidance/browse-all-guidance/other-guidelines-and-reports/fetal-awareness-updated-review-of-research-and-recommendations-for-practice/ (Accessed January 20, 2023).
35. American College of Pediatricians—January 2021. Fetal pain: what is the scientific evidence? Issues Law Med. (2021) 36(1):113–22. 33939344.33939344
36. Pierucci R. Fetal pain: the science behind why it is the medical standard of care. Linacre Q. (2020) 87(3):311–6. doi: 10.1177/0024363920924877
37. Lagercrantz H. Chapter 8: pain. In: Lagercrantz H, editor. Infant brain development: formation of the mind and the emergence of consciousness. Cham: Springer International Publishing (2016). p. 99–106. doi: 10.1007/978-3-319-44845-9_8
38. Yaster M. Analgesia and anesthesia in neonates. J Pediatr. (1987) 111(3):394–6. doi: 10.1016/s0022-3476(87)80461-4
39. Teixeira J, Fogliani R, Giannakoulopoulos X, Glover V, Fisk NM. Fetal haemodynamic stress response to invasive procedures. Lancet. (1996) 347(9001):624. doi: 10.1016/s0140-6736(96)91327-6
40. Teixeira JM, Glover V, Fisk NM. Acute cerebral redistribution in response to invasive procedures in the human fetus. Am J Obstet Gynecol. (1999) 181(4):1018–25. doi: 10.1016/s0002-9378(99)70340-6
41. Giannakoulopoulos X, Teixeira J, Fisk N, Glover V. Human fetal and maternal noradrenaline responses to invasive procedures. Pediatr Res. (1999) 45(4 Pt 1):494–9. doi: 10.1203/00006450-199904010-00007
42. Fisk NM, Gitau R, Teixeira JM, Giannakoulopoulos X, Cameron AD, Glover VA. Effect of direct fetal opioid analgesia on fetal hormonal and hemodynamic stress response to intrauterine needling. Anesthesiology. (2001) 95(4):828–35. doi: 10.1097/00000542-200110000-00008
43. Anand KJ, Maze M. Fetuses, fentanyl, and the stress response: signals from the beginnings of pain? Anesthesiology. (2001) 95(4):823–5. doi: 10.1097/00000542-200110000-00006
44. Anand KJ, Hickey PR. Halothane-morphine compared with high-dose sufentanil for anesthesia and postoperative analgesia in neonatal cardiac surgery. N Engl J Med. (1992) 326(1):1–9. doi: 10.1056/NEJM199201023260101
45. Bellieni CV. New insights into fetal pain. Semin Fetal Neonatal Med. (2019) 24(4):101001. doi: 10.1016/j.siny.2019.04.001
46. Bellieni CV. Pain in the fetus and the preterm baby. In: van Rysewyk S, editor. Meanings of pain: Volume 3: Vulnerable or special groups of people. Cham: Springer International Publishing (2022). p. 17–32. doi: 10.1007/978-3-030-95825-1_2
47. Thill B. Fetal pain in the first trimester. Linacre Q. (2022) 89(1):73–100. doi: 10.1177/00243639211059245
48. Humphrey T. Some correlations between the appearance of human fetal reflexes and the development of the nervous system. In: Purpura DP, Schadé JP, editors. Progress in brain research. Amsterdam: Elsevier (1964). p. 93–135. (Growth and Maturation of the Brain; vol. 4). doi: 10.1016/S0079-6123(08)61273-X
49. Okado N, Kojima T. Ontogeny of the central nervous system: neurogenesis, fibre connection, synaptogenesis and myelination in the spinal cord. In: Prechtl H, editor. Continuity of neural functions from prenatal to postnatal life. Philadelphia, PA: JB Lippincott (1984). p. 31–45.
50. Derbyshire SWG. Can fetuses feel pain? Br Med J. (2006) 332(7546):909–12. doi: 10.1136/bmj.332.7546.909
51. Derbyshire SWG. Fetal pain: do we know enough to do the right thing? Reprod Health Matters. (2008) 16(31 Suppl):117–26. doi: 10.1016/S0968-8080(08)31370-6
52. Bystron I, Blakemore C, Rakic P. Development of the human cerebral cortex: boulder committee revisited. Nat Rev Neurosci. (2008) 9(2):110–22. doi: 10.1038/nrn2252
53. Kostović I. The enigmatic fetal subplate compartment forms an early tangential cortical nexus and provides the framework for construction of cortical connectivity. Prog Neurobiol. (2020) 194:101883. doi: 10.1016/j.pneurobio.2020.101883
54. Petrikovsky BM, Kaplan GP. Fetal responses to inadvertent contact with the needle during amniocentesis. Fetal Diagn Ther. (1995) 10(2):83–5. doi: 10.1159/000264210
55. Gitau R, Fisk NM, Teixeira JM, Cameron A, Glover V. Fetal hypothalamic-pituitary-adrenal stress responses to invasive procedures are independent of maternal responses. J Clin Endocrinol Metab. (2001) 86(1):104–9. doi: 10.1210/jcem.86.1.7090
56. Mayorga-Buiza MJ, Marquez-Rivas J, Gomez-Gonzalez E. Can fetus feel pain in the second trimester? Lessons learned from a sentinel event. Childs Nerv Syst. (2018) 34(2):195–6. doi: 10.1007/s00381-017-3677-6
57. Bernardes LS, Ottolia JF, Cecchini M, de Amorim Filho AGA, Teixeira MJ, Francisco RPV, et al. On the feasibility of accessing acute pain-related facial expressions in the human fetus and its potential implications: a case report. Pain Rep. (2018) 3(5):e673. doi: 10.1097/PR9.0000000000000673
58. Bernardes LS, Carvalho MA, Harnik SB, Teixeira MJ, Ottolia J, Castro D, et al. Sorting pain out of salience: assessment of pain facial expressions in the human fetus. Pain Rep. (2021) 6(1):e882. doi: 10.1097/PR9.0000000000000882. Figure 4 relabeled as Figure 7. Reproduced by permission from Wolters Kluwer Health, Inc.33537520
59. Merskey H. Pain terms: a list with definitions and notes on usage. Recommended by the IASP subcommittee on taxonomy. Pain. (1979) 6(3):249. 460932.460932
60. Raja SN, Carr DB, Cohen M, Finnerup NB, Flor H, Gibson S, et al. The revised international association for the study of pain definition of pain: concepts, challenges, and compromises. Pain. (2020) 161(9):1976–82. doi: 10.1097/j.pain.0000000000001939
61. van der Vaart M, Hartley C, Baxter L, Mellado GS, Andritsou F, Cobo MM, et al. Premature infants display discriminable behavioral, physiological, and brain responses to noxious and nonnoxious stimuli. Cereb Cortex. (2022) 32(17):3799–815. doi: 10.1093/cercor/bhab449
62. International Association for the Study of Pain (IASP). Terminology. Available from: https://www.iasp-pain.org/resources/terminology/ (Accessed January 20, 2023).
63. Benatar D, Benatar M. A pain in the fetus: toward ending confusion about fetal pain. Bioethics. (2001) 15(1):57–76. doi: 10.1111/1467-8519.00212
64. Van de Velde M, De Buck F. Fetal and maternal analgesia/anesthesia for fetal procedures. Fetal Diagn Ther. (2012) 31(4):201–9. doi: 10.1159/000338146
65. Glover V, Fisk NM. Fetal pain: implications for research and practice. Br J Obstet Gynaecol. (1999) 106(9):881–6. doi: 10.1111/j.1471-0528.1999.tb08424.x
66. Glover V. The fetus may feel pain from 20 weeks. Conscience. (2004) 25(3):35–7. 16538756.16538756
67. Belle M, Godefroy D, Couly G, Malone SA, Collier F, Giacobini P, et al. Tridimensional visualization and analysis of early human development. Cell. (2017) 169(1):161–73.e12. doi: 10.1016/j.cell.2017.03.008. Figure 2(B) relabeled as Figure 5. Reproduced by permission from Elsevier.28340341
68. Kostović I, Judaš M. The role of the subplate zone in the structural plasticity of the developing human cerebral cortex. NBA. (2002) 1(4):145–53. doi: 10.1159/000066270
69. Kostovic I, Rakic P. Developmental history of the transient subplate zone in the visual and somatosensory cortex of the macaque monkey and human brain. J Comp Neurol. (1990) 297(3):441–70. doi: 10.1002/cne.902970309
70. Hevner RF. Development of connections in the human visual system during fetal mid-gestation: a DiI-tracing study. J Neuropathol Exp Neurol. (2000) 59(5):385–92. doi: 10.1093/jnen/59.5.385
71. Judaš M, Sedmak G, Kostović I. The significance of the subplate for evolution and developmental plasticity of the human brain. Front Hum Neurosci. (2013) 7:423. doi: 10.3389/fnhum.2013.00423
72. Saleem SN. Fetal magnetic resonance imaging (MRI): a tool for a better understanding of normal and abnormal brain development. J Child Neurol. (2013) 28(7):890–908. doi: 10.1177/0883073813486296. Figure 2 relabeled as Figure 6. Reproduced by permission from SAGE Publications23644716
73. Luhmann HJ, Kirischuk S, Kilb W. The superior function of the subplate in early neocortical development. Front Neuroanat. (2018) 12:97. doi: 10.3389/fnana.2018.00097
74. Chambers CT, Mogil JS. Ontogeny and phylogeny of facial expression of pain. Pain. (2015) 156(5):798–9. doi: 10.1097/j.pain.0000000000000133
75. Madathil S, Thomas D, Chandra P, Agarwal R, Sankar MJ, Thukral A, et al. « NOPAIN-ROP » trial: intravenous fentanyl and intravenous ketamine for pain relief during laser photocoagulation for retinopathy of prematurity (ROP) in preterm infants: a randomised trial. BMJ Open. (2021) 11(9):e046235. doi: 10.1136/bmjopen-2020-046235
76. Gibbins S, Stevens BJ, Yamada J, Dionne K, Campbell-Yeo M, Lee G, et al. Validation of the premature infant pain profile-revised (PIPP-R). Early Hum Dev. (2014) 90(4):189–93. doi: 10.1016/j.earlhumdev.2014.01.005
77. Stevens BJ, Gibbins S, Yamada J, Dionne K, Lee G, Johnston C, et al. The premature infant pain profile-revised (PIPP-R): initial validation and feasibility. Clin J Pain. (2014) 30(3):238–43. doi: 10.1097/AJP.0b013e3182906aed
78. Hartley C, Goksan S, Poorun R, Brotherhood K, Mellado GS, Moultrie F, et al. The relationship between nociceptive brain activity, spinal reflex withdrawal and behaviour in newborn infants. Sci Rep. (2015) 5:12519. doi: 10.1038/srep12519
79. Cornelissen L, Fabrizi L, Patten D, Worley A, Meek J, Boyd S, et al. Postnatal temporal, spatial and modality tuning of nociceptive cutaneous flexion reflexes in human infants. PLoS One. (2013) 8(10):e76470. doi: 10.1371/journal.pone.0076470
80. Laudiano-Dray MP, Pillai Riddell R, Jones L, Iyer R, Whitehead K, Fitzgerald M, et al. Quantification of neonatal procedural pain severity: a platform for estimating total pain burden in individual infants. Pain. (2020) 161(6):1270–7. doi: 10.1097/j.pain.0000000000001814
81. Duci M, Pulvirenti R, Fascetti Leon F, Capolupo I, Veronese P, Gamba P, et al. Anesthesia for fetal operative procedures: a systematic review. Front Pain Res (Lausanne). (2022) 3:935427. doi: 10.3389/fpain.2022.935427
82. Ferschl M, Rollins MD. Ch. 63: anesthesia for fetal surgery and other fetal therapies. In: Gropper MA, Miller RD, Eriksson LI, Fleisher LA, Wiener-Kronish JP, Cohen NH, et al. editors Miller’s anesthesia. 9th éd. Philadelphia, PA: Elsevier (2019). p. 1042–70.
83. Friedman KG, Tworetzky W. Fetal cardiac interventions: where do we stand? Arch Cardiovasc Dis. (2020) 113(2):121–8. doi: 10.1016/j.acvd.2019.06.007
84. Bellieni CV. Analgesia for fetal pain during prenatal surgery: 10 years of progress. Pediatr Res. (2021) 89(7):1612–8. doi: 10.1038/s41390-020-01170-2
85. Hoagland M, Chatterjee D. Anesthesia for fetal interventions—an update. Adv Anesth. (2021) 39:269–90. doi: 10.1016/j.aan.2021.08.004
86. Anderson AL, Thomason ME. Functional plasticity before the cradle: a review of neural functional imaging in the human fetus. Neurosci Biobehav Rev. (2013) 37(9 Pt B):2220–32. doi: 10.1016/j.neubiorev.2013.03.013
87. Sicard-Cras I, Rioualen S, Pellae E, Misery L, Sizun J, Roué J-M. A review of the characteristics, mechanisms and clinical significance of habituation in foetuses and newborn infants. Acta Paediatr. (2022) 111(2):245–58. doi: 10.1111/apa.16115
88. Moser J, Schleger F, Weiss M, Sippel K, Semeia L, Preissl H. Magnetoencephalographic signatures of conscious processing before birth. Dev Cogn Neurosci. (2021) 49:100964. doi: 10.1016/j.dcn.2021.100964
89. Kucyi A, Davis KD. The neural code for pain: from single-cell electrophysiology to the dynamic pain connectome. Neuroscientist. (2017) 23(4):397–414. doi: 10.1177/1073858416667716
90. Davis KD, Kucyi A, Moayedi M. The pain switch: an « ouch » detector. Pain. (2015) 156(11):2164–6. doi: 10.1097/j.pain.0000000000000303
91. Fabrizi L, Slater R, Worley A, Meek J, Boyd S, Olhede S, et al. A shift in sensory processing that enables the developing human brain to discriminate touch from pain. Curr Biol. (2011) 21(18):1552–8. doi: 10.1016/j.cub.2011.08.010
92. Mellor DJ, Diesch TJ, Gunn AJ, Bennet L. The importance of « awareness » for understanding fetal pain. Brain Res Brain Res Rev. (2005) 49(3):455–71. doi: 10.1016/j.brainresrev.2005.01.006
93. Falsaperla R, Collotta AD, Spatuzza M, Familiari M, Vitaliti G, Ruggieri M. Evidences of emerging pain consciousness during prenatal development: a narrative review. Neurol Sci. (2022) 43(6):3523–32. doi: 10.1007/s10072-022-05968-2
94. Kadic AS, Kurjak A. Cognitive functions of the Fetus. Ultraschall Med. (2018) 39(2):181–9. doi: 10.1055/s-0043-123469
95. Zhao T, Zhu Y, Tang H, Xie R, Zhu J, Zhang JH. Consciousness: new concepts and neural networks. Front Cell Neurosci. (2019) 13:302. doi: 10.3389/fncel.2019.00302
96. Bellieni CV, Vannuccini S, Petraglia F. Is fetal analgesia necessary during prenatal surgery? J Matern Fetal Neonatal Med. (2018) 31(9):1241–5. doi: 10.1080/14767058.2017.1311860
97. Li J, Curley WH, Guerin B, Dougherty DD, Dalca AV, Fischl B, et al. Mapping the subcortical connectivity of the human default mode network. Neuroimage. (2021) 245:118758. doi: 10.1016/j.neuroimage.2021.118758
98. Fabbro F, Aglioti SM, Bergamasco M, Clarici A, Panksepp J. Evolutionary aspects of self- and world consciousness in vertebrates. Front Hum Neurosci. (2015) 9:157. doi: 10.3389/fnhum.2015.00157
99. Lutkenhoff ES, Chiang J, Tshibanda L, Kamau E, Kirsch M, Pickard JD, et al. Thalamic and extrathalamic mechanisms of consciousness after severe brain injury. Ann Neurol. (2015) 78(1):68–76. doi: 10.1002/ana.24423
100. Stanojevic M, Kadic AS, Jakovljević M. Fetal awareness. Donald School J Ultrasound Obstet Gynecol. (2021) 15(2):188–94. doi: 10.5005/jp-journals-10009-1700
101. Apkarian AV. Nociception, pain, consciousness, and society: a plea for constrained use of pain-related terminologies. J Pain. (2018) 19(11). doi: 10.1016/j.jpain.2018.05.010
102. Hata T, AboEllail MA. Chapter 32—antenatal fetal neurodevelopment test: KANET assessment. In: Martin CR, Preedy VR, Rajendram R, editors. Diagnosis, management and modeling of neurodevelopmental disorders. London: Academic Press (2021). p. 367–75. doi: 10.1016/B978-0-12-817988-8.00032-4
103. Kurjak A, Stanojević M, Salihagić-Kadić A, Spalldi Barišić L, Jakovljević M. Is four-dimensional (4D) ultrasound entering a new field of fetal psychiatry? Psychiatr Danub. (2019) 31(2):133–40. doi: 10.24869/psyd.2019.133
104. Zoia S, Blason L, D’Ottavio G, Bulgheroni M, Pezzetta E, Scabar A, et al. Evidence of early development of action planning in the human foetus: a kinematic study. Exp Brain Res. (2007) 176(2):217–26. doi: 10.1007/s00221-006-0607-3
105. Castiello U, Becchio C, Zoia S, Nelini C, Sartori L, Blason L, et al. Wired to be social: the ontogeny of human interaction. PLoS One. (2010) 5(10):e13199. doi: 10.1371/journal.pone.0013199
106. Padilla N, Lagercrantz H. Making of the mind. Acta Paediatr. (2020) 109(5):883–92. doi: 10.1111/apa.15167
107. Terrier L-M, Lévêque M, Amelot A. Brain lobotomy: a historical and moral dilemma with no alternative? World Neurosurg. (2019) 132:211–8. doi: 10.1016/j.wneu.2019.08.254
108. Hartley C, Duff EP, Green G, Mellado GS, Worley A, Rogers R, et al. Nociceptive brain activity as a measure of analgesic efficacy in infants. Sci Transl Med. (2017) 9(388):eaah6122. doi: 10.1126/scitranslmed.aah6122
109. van den Heuvel MP, Sporns O. Network hubs in the human brain. Trends Cogn Sci. (2013) 17(12):683–96. doi: 10.1016/j.tics.2013.09.012
110. Doria V, Beckmann CF, Arichi T, Merchant N, Groppo M, Turkheimer FE, et al. Emergence of resting state networks in the preterm human brain. Proc Natl Acad Sci USA. (2010) 107(46):20015–20. doi: 10.1073/pnas.1007921107
111. van den Heuvel MI, Turk E, Manning JH, Hect J, Hernandez-Andrade E, Hassan SS, et al. Hubs in the human fetal brain network. Dev Cogn Neurosci. (2018) 30:108–15. doi: 10.1016/j.dcn.2018.02.001
112. Lowery CL, Hardman MP, Manning N, Hall RW, Anand KJS, Clancy B. Neurodevelopmental changes of fetal pain. Semin Perinatol. (2007) 31(5):275–82. doi: 10.1053/j.semperi.2007.07.004
113. Henderson LA, Keay KA. Imaging acute and chronic pain in the human brainstem and spinal cord. Neuroscientist. (2018) 24(1):84–96. doi: 10.1177/1073858417703911
114. Feinstein JS, Khalsa SS, Salomons TV, Prkachin KM, Frey-Law LA, Lee JE, et al. Preserved emotional awareness of pain in a patient with extensive bilateral damage to the insula, anterior cingulate, and amygdala. Brain Struct Funct. (2016) 221(3):1499–511. doi: 10.1007/s00429-014-0986-3
115. Apkarian A. Evidence against pain specificity in the dorsal posterior insula. F1000Res. (2015) 4:7–8. doi: 10.12688/f1000research.7347.r9627
116. Coghill RC. The distributed nociceptive system: a framework for understanding pain. Trends Neurosci. (2020) 43(10):780–94. doi: 10.1016/j.tins.2020.07.004
117. Mazzola L, Isnard J, Peyron R, Mauguière F. Stimulation of the human cortex and the experience of pain: Wilder Penfield’s observations revisited. Brain. (2012) 135(Pt 2):631–40. doi: 10.1093/brain/awr265
118. Brusseau R. Developmental perspectives: is the fetus conscious? Int Anesthesiol Clin. (2008) 46(3):11–23. doi: 10.1097/AIA.0b013e318181a88e
119. Aleman B, Merker B. Consciousness without cortex: a hydranencephaly family survey. Acta Paediatr. (2014) 103(10):1057–65. doi: 10.1111/apa.12718
120. Andersson A-KM. Congenitally decorticate children’s potential and rights. J Med Ethics. (2021) 47(12):e85. doi: 10.1136/medethics-2020-106163
121. Tadros MA, Lim R, Hughes DI, Brichta AM, Callister RJ. Electrical maturation of spinal neurons in the human fetus: comparison of ventral and dorsal horn. J Neurophysiol. (2015) 114(5):2661–71. doi: 10.1152/jn.00682.2015
122. Andaya E, Campo-Engelstein L. Conceptualizing pain and personhood in the periviable period: perspectives from reproductive health and neonatal intensive care unit clinicians. Soc Sci Med. (2021) 269:113558. doi: 10.1016/j.socscimed.2020.113558
123. Molnár Z, Luhmann HJ, Kanold PO. Transient cortical circuits match spontaneous and sensory-driven activity during development. Science. (2020) 370(6514):eabb2153. doi: 10.1126/science.abb2153
124. Wallois F, Routier L, Heberlé C, Mahmoudzadeh M, Bourel-Ponchel E, Moghimi S. Back to basics: the neuronal substrates and mechanisms that underlie the electroencephalogram in premature neonates. Neurophysiol Clin. (2021) 51(1):5–33. doi: 10.1016/j.neucli.2020.10.006
125. Kostović I, Sedmak G, Vukšić M, Judaš M. The relevance of human fetal subplate zone for developmental neuropathology of neuronal migration disorders and cortical dysplasia. CNS Neurosci Ther. (2015) 21(2):74–82. doi: 10.1111/cns.12333
126. McCarthy KF, Colvin LA. Back to the future: lifelong changes in pain processing in « ageing of prematurity ». Br J Anaesth. (2018) 121(3):529–31. doi: 10.1016/j.bja.2018.06.017
127. De Asis-Cruz J, Barnett SD, Kim J-H, Limperopoulos C. Functional connectivity-derived optimal gestational-age cut points for fetal brain network maturity. Brain Sci. (2021) 11(7):921. doi: 10.3390/brainsci11070921
128. Kidokoro H. Delta brushes are not just a hallmark of EEG in human preterm infants. Pediatr Int. (2021) 63(2):130–6. doi: 10.1111/ped.14420
129. Hadders-Algra M. Early human motor development: from variation to the ability to vary and adapt. Neurosci Biobehav Rev. (2018) 90:411–27. doi: 10.1016/j.neubiorev.2018.05.009
130. Wess JM, Isaiah A, Watkins PV, Kanold PO. Subplate neurons are the first cortical neurons to respond to sensory stimuli. Proc Natl Acad Sci USA. (2017) 114(47):12602–7. doi: 10.1073/pnas.1710793114
131. Colonnese MT, Kaminska A, Minlebaev M, Milh M, Bloem B, Lescure S, et al. A conserved switch in sensory processing prepares developing neocortex for vision. Neuron. (2010) 67(3):480–98. doi: 10.1016/j.neuron.2010.07.015
132. Wilson EK. Ex utero: live human fetal research and the films of Davenport Hooker. Bull Hist Med. (2014) 88(1):132–60. doi: 10.1353/bhm.2014.0002
Keywords: fetal pain, fetal analgesia, fetal anesthesia, fetal nociception, fetal awareness, subplate
Citation: Thill B (2023) The fetal pain paradox. Front. Pain Res. 4:1128530. doi: 10.3389/fpain.2023.1128530
Received: 20 December 2022; Accepted: 21 February 2023;
Published: 21 March 2023.
Edited by:
Kim Kopenhaver Doheny, The Pennsylvania State University, United StatesReviewed by:
Dafna Sussman, Ryerson University, CanadaPishan Chang, University College London, United Kingdom
© 2023 Thill. This is an open-access article distributed under the terms of the Creative Commons Attribution License (CC BY). The use, distribution or reproduction in other forums is permitted, provided the original author(s) and the copyright owner(s) are credited and that the original publication in this journal is cited, in accordance with accepted academic practice. No use, distribution or reproduction is permitted which does not comply with these terms.
*Correspondence: Bridget Thill bathill1@umary.edu
Specialty Section: This article was submitted to Pediatric Pain, a section of the journal Frontiers in Pain Research