DNA Methylation of TLR4, VEGFA, and DEFA5 Is Associated With Necrotizing Enterocolitis in Preterm Infants
- 1Division of Neonatology, Beatrix Children's Hospital, University Medical Center Groningen, University of Groningen, Groningen, Netherlands
- 2Department of Obstetrics and Gynecology, University Medical Center Groningen, University of Groningen, Groningen, Netherlands
- 3Division of Pediatric Surgery, Department of Surgery, University Medical Center Groningen, University of Groningen, Groningen, Netherlands
Background: Epigenetic changes, such as DNA methylation, may contribute to an increased susceptibility for developing necrotizing enterocolitis (NEC) in preterm infants. We assessed DNA methylation in five NEC-associated genes, selected from literature: EPO, VEGFA, ENOS, DEFA5, and TLR4 in infants with NEC and controls.
Methods: Observational cohort study including 24 preterm infants who developed NEC (≥Bell Stage IIA) and 45 matched controls. DNA was isolated from stool samples and methylation measured using pyrosequencing. We investigated differences in methylation prior to NEC compared with controls. Next, in NEC infants, we investigated methylation patterns long before, a short time before NEC onset, and after NEC.
Results: Prior to NEC, only TLR4 CpG 2 methylation was increased in NEC infants (median = 75.4%, IQR = 71.3–83.8%) versus controls (median = 69.0%, IQR = 64.5–77.4%, p = 0.025). In NEC infants, VEGFA CpG 3 methylation was 0.8% long before NEC, increasing to 1.8% a short time before NEC and 2.0% after NEC (p = 0.011; p = 0.021, respectively). A similar pattern was found in DEFA5 CpG 1, which increased from 75.4 to 81.4% and remained 85.3% (p = 0.027; p = 0.019, respectively). These changes were not present for EPO, ENOS, and TLR4.
Conclusion: Epigenetic changes of TLR4, VEGFA, and DEFA5 are present in NEC infants and can differ in relation to the time of NEC onset. Differences in DNA methylation of TLR4, VEGFA, and DEFA5 may influence gene expression and increase the risk for developing NEC. This study also demonstrates the use of human DNA extraction from stool samples as a novel non-invasive method for exploring the bowel of preterm infants and which can also be used for necrotizing enterocolitis patients.
Introduction
Necrotizing enterocolitis (NEC) is a severe gastrointestinal inflammatory disease that mainly affects preterm-born infants. The etiology of NEC is not completely understood but is most likely multifactorial, with prematurity having a central role (1). Four of the main components preceding NEC are the immature intestinal barrier, immature circulatory regulation of the intestines, abnormal motility patterns and an immature immune system. Other contributing factors are formula feeding, abnormal colonization by potentially pathogenic bacteria and hypoxic ischemic injury (1, 2).
However, when comparing preterm infants with a similar risk profile, some develop NEC while others do not (3). This variable susceptibility in some infants suggests that the current multifactorial model may not be complete (4). We hypothesize here that epigenetic alterations contribute to this predisposition of some infants for developing necrotizing enterocolitis. The study of epigenetics involves changes in gene expression that are not caused by modifications in the genetic code. Increasing evidence suggests epigenetic modifications to be the link between early environmental stressors and later disease (5). We know of several environmental risk factors for NEC, such as antibiotic exposure, formula feeds, gut microbiota alterations, prolonged gut hypoxia and red blood cell transfusions. Several of these have already been directly associated with epigenetic alterations. Microbiota alterations in particular can induce epigenetic changes in immature intestinal epithelial cells (6).
The best studied epigenetic mechanism is DNA methylation. Methylation usually occurs at cytosine-phosphate-guanosine (CpG) positions in the DNA. An increase of methylation in gene promoters is often associated with a decrease in gene expression. DNA methylation is dynamic and can change over time, in response to environmental stimuli (5). The fetal and neonatal period is known to be prone for changes in DNA methylation and many differentially methylated CpGs have been identified in preterm-born infants (7). Changes in DNA methylation of several percentages can already cause significant changes in gene expression. DNA methylation in preterm born infants with NEC has been evaluated only in surgically treated NEC patients. There, clear differences in the epigenome of NEC infants was found compared to controls, as well as an association between DNA methylation and gene expression (8). Preterm born pigs affected by NEC also show differences in DNA methylation of several intestinal genes, compared to unaffected preterm born pigs. The same study underlines the dynamic nature of DNA methylation of intestinal genes in the first day after birth, which is increased after exposing the immature intestine to formula feeding (9). Other differences in epigenetic mechanisms, for example dysregulated micro-RNAs, are present as well in NEC affected infants (10).
The above led us to hypothesize a potential role for DNA methylation in the development of NEC. We selected five genes after reviewing literature on methylation of intestinal wall derived genes previously reported to have a potential role in the development of NEC including erythropoietin (EPO) (11, 12), vascular endothelial growth factor A (VEGFA) (13–15), endothelial nitric oxide synthesis (ENOS) (16), defensin alpha 5 (DEFA5) (17), and toll-like receptor 4 (TLR4) (18–20).
EPO, VEGFA, and ENOS are involved in the perfusion of the intestine. EPO regulates the red blood cell production and prevents down-regulation of the intestinal tight-junction protein ZO-1 (11). Its gene product, erythropoietin, naturally occurs in breast milk, which reduces the risk of NEC development, especially in very low birth weight infants (12). Treatment with erythropoietin also lowered the incidence of NEC and tight junction damage in mouse pups (11). VEGFA promotes proliferation of endothelial cells, tube formation, and facilitates angiogenesis (13). In human and experimental NEC models, there is a decrease in the VEGFA protein in intestinal tissue (14). Genetic variations of VEGF that decrease VEGF expression increase the risk of NEC development (15). ENOS is responsible for the majority of nitric oxide (NO) production, the most potent vasodilator stimulus active in the new-born intestine. Expression of ENOS and production of NO is decreased in the arterioles of NEC infants (16).
DEFA5 and TLR4 are both important for the intestinal immunity. The gene product of DEFA5 is an anti-microbial protein secreted by Paneth cells and TLR4 belongs to the group of pattern recognition receptors. Both an increase and a decrease of immune competent Paneth cells and DEFA5 expression has been suggested as a cause of NEC (17). TLR4 is expressed on both immune and intestinal cells and can be upregulated in response to stress (18). TLR4 is also required for the development of the gut, it is therefore highly expressed during fetal development. With the abundance of TLR4 receptors in the preterm gut, activation by LPS may cause an overwhelming inflammatory response. The release of inflammatory cytokines as well as an increase in enterocyte apoptosis and a reduction in enterocyte proliferation can lead to NEC (19). Small intestinal cells have a higher expression of TLR4 when NEC is present in animal studies, compared to healthy intestinal cells (18, 20).
To test our hypothesis that methylation of these genes is indeed associated with NEC we first aimed to determine whether a difference between NEC affected infants and their non-affected peers existed in DNA methylation prior to NEC onset of the EPO, VEGFA, ENOS, DEFA5, and TLR4 genes. Second, in the infants diagnosed with NEC, we aimed to investigate whether methylation patterns were different long before, a short time before and after NEC onset.
Methods
We performed an observational cohort study, with a nested case-control design. We included infants admitted to the level III Neonatal Intensive Care Unit of the University Medical Center Groningen (UMCG) from April 2016 to December 2018. For this pilot study, we used residual stool samples of an ongoing multicenter observational cohort study on fecal volatile organic compounds (VOCs), analyzed by an electronic nose (21). We included infants born after <30 weeks of gestation. Written informed consent was obtained from the parents and the Institutional Review Board of the UMCG approved the study. Using the residual samples for this study was permitted under the Dutch Medical Research with Human Subjects Law. Of all participating infants in the original cohort study, 24 developed definite NEC with Bell's stage 2A or higher. The diagnosis NEC was established via the presence of pneumatosis intestinalis and/or portal venous gas on the abdominal X-ray, or during surgery. Cases of SIP were therefore excluded on a clinical and pathological basis. Out of the remaining 150 infants, 48 were matched to the NEC affected infants. The primary matching criterium was gestational age, for which we accepted a difference of maximum 4 days. In case there were multiple candidates, similar birth weight and sex were used to select the controls. We excluded infants with suspected NEC (Bell's stage < II). In Figure 1 we display the subject selection.
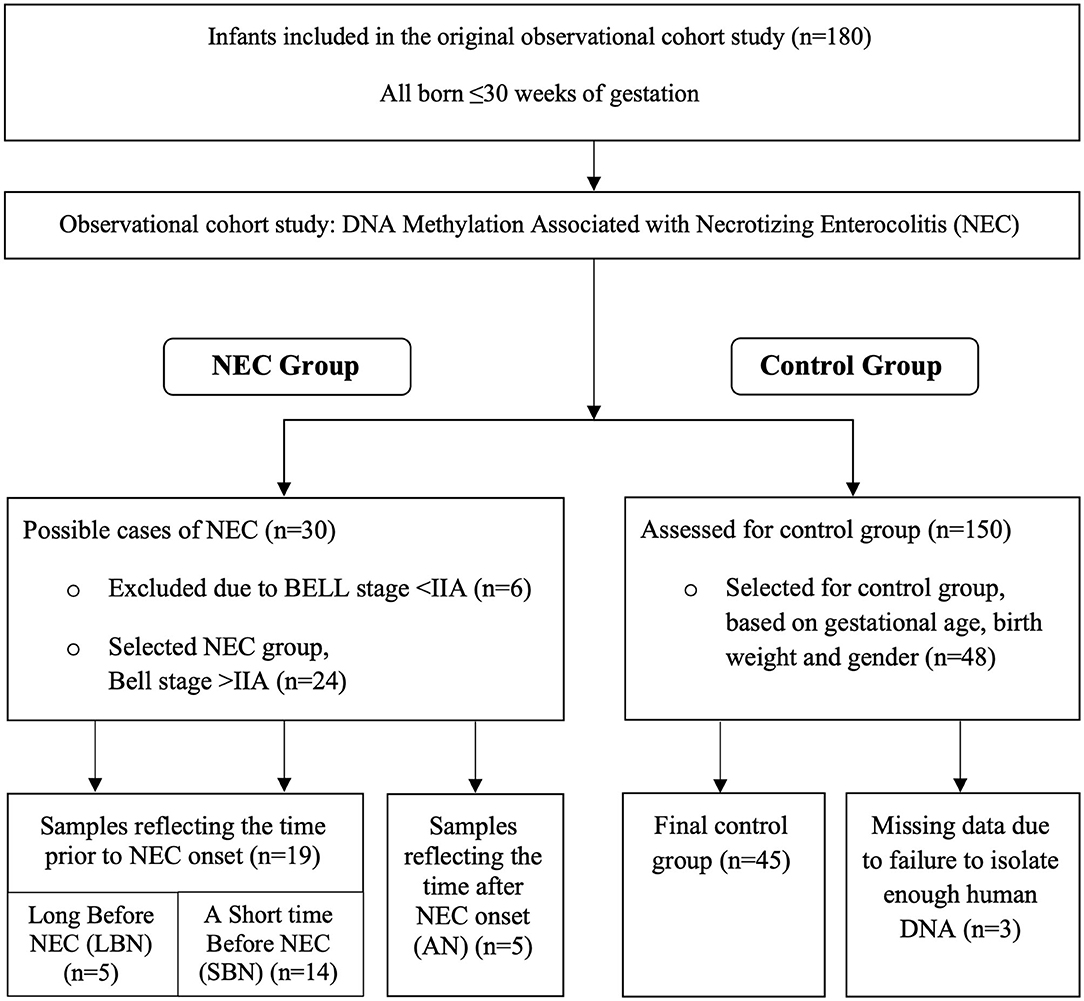
Figure 1. Flow diagram presenting the selection of Necrotizing Enterocolitis (NEC) infants and controls.
Fecal Sample Selection
We measured DNA methylation of EPO, VEGFA, ENOS, DEFA5, and TLR4 by isolating human DNA from stool samples. Human DNA in stool samples mainly originates from intestinal epithelium cells. These cells renew every 4–5 days in adults and are shed into the feces, however this proliferation process may be increased in preterm infants (22). The main types of cells shed into the stool in infants are enterocytes, goblet cells, Paneth cells, and enteroendocrine cells, whereas only a small number of cells of lymphoid origin is present (23, 24). The amount of human DNA in stool samples is low, <1% of the total DNA, as the vast majority of DNA present in feces is bacterial DNA from the gut microbiota (25).
From every stool in the first 28 days after birth or until transfer to a level II hospital, samples were collected and stored at −20 degrees. The stool samples used for the current study were preferably selected from the days around onset of NEC, and in controls around a similar postnatal age. We collected samples at three different time points and defined three groups accordingly.
The first group (a Short time Before NEC, SBN) consisted of infants in which we selected samples from the week before NEC onset or until 2 days after NEC onset, depending on the availability of the samples. Samples taken in this window contain intestinal cells that are around 4–5 days old. They therefore reflect DNA methylation patterns which are present a short time before the onset of NEC. In the second group (Long Before NEC, LBN) we collected samples from infants who developed NEC after the first 28 days in which the stool samples were collected. For these infants, we selected the last collected sample for analysis. These samples reflect a somewhat longer time before NEC onset.
For the control infants, we matched stool samples based on postnatal day of the samples from the NEC affected infants. The stool samples from the SBN and LBN group and controls were used in the primary objective: to compare DNA methylation prior to NEC in children who developed NEC with DNA methylation in postnatal-age-matched controls, who did not develop NEC.
In the third group (After NEC, AN) we collected stool samples only after NEC development because these children had already developed NEC within a few days after birth. In this group stool samples from the preferred window around NEC onset were either not available or meconium-like. Meconium-like samples include intestinal cells that were shed during gestation and thus may not show a representative DNA methylation pattern. For these NEC infants we therefore chose to include the first available sample taken after the onset of NEC. DNA methylation changes of these samples may reflect ongoing NEC or NEC recovery instead of changes prior to NEC onset. These samples were only used in the secondary objective of the study, to assess the DNA methylation within NEC infants, at various time frames in relation to NEC. The selection of stool samples and the division in several time frames is displayed in Figure 1.
DNA Isolation
The QIAamp Fast DNA stool mini kit (Qiagen, Hilden, Germany) was used according to the protocol to isolate DNA. From each stool sample, 220 mg of stool was used. An additional 30 s TissueLyser LT (Qiagen) step at 50 Hz with a stainless-steel bead was included at step 2 to obtain a homogenous stool sample. We checked quality and concentration of the isolated DNA with both the NanoDrop®ND-1000 spectrophotometer (Thermo Fisher Scientific, Waltham, MA) and by gel electrophoresis with 10 μl of the isolated DNA on 1% agarose gel with ethidium bromide staining. In case of a low DNA yield, DNA isolation was repeated using a different stool sample from the same infant, with a maximum of three repetitions per infant. We classified low DNA yield as <5 ng/μl of DNA on spectrophotometry or no DNA visible on gel electrophoresis. After DNA isolation, all samples underwent bisulfite treatment using the EZ DNA methylation Gold-Kit (Zymo Research, Irvine, CA) according to the supplier's protocol with an additional 30 s centrifuge round at full speed after step 8.
Primer Design
The genomic target region selected for analysis was chosen based on relevant existing literature on gene expression and DNA methylation. DNA methylation occurs at cytosine-phosphate-guanosine (CpG) positions, therefore CpG rich areas in the promotor area were preferably selected. The analyzed promotor region for EPO includes the binding site for the hypoxia inducible factor (HIF) complex, which represses transcription through hypermethylation in several human cancers (26). The primers designed for VEGFA were designed to fit an area around a HIF binding site where hypomethylation is present in placental tissue following preeclampsia (27). For ENOS, the chosen site is part of a positive regulatory domain in the ENOS promotor, including binding sites for the transcriptional activators Sp-1/Sp-3 (28). Hypermethylation in this area is related to decreased activity and endothelial dysfunction (29). The promotor region selected for DEFA5 is a CpG rich area in which hypermethylation and decreased expression is present in intestinal cells of Crohn's disease patients (30). The area selected for TLR4 was chosen due to the presence of the binding sites for Sp-1 and regulatory factor X1, a TLR4 transcription suppressor. The methylation status in this area is strongly associated with TLR4 expression (31, 32). All PCR and sequencing primers for these target regions were designed using the Pyromark Assay Design software (Qiagen) and are presented in Table 1.
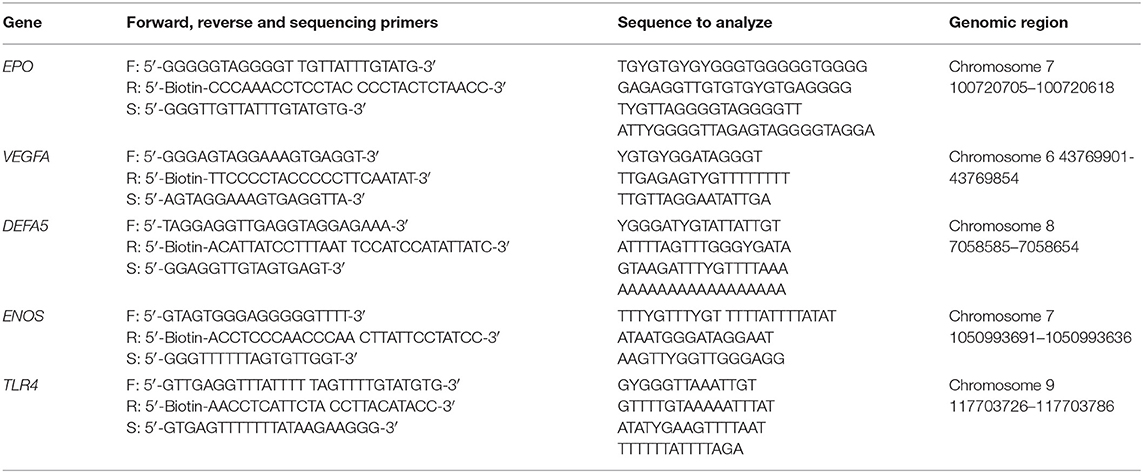
Table 1. PCR forward and reverse primer sequences accompanied by sequencing primer and the sequence to analyze, with its genomic region (Homo sapiens GRCh38.p12 primary assembly).
PCR
For the DNA amplification we used 12.5 μl of Qiagen HotStar Taq Master Mix, 1 μl of 10 μM forward and reverse primer, 1 μl of bisulfite converted DNA and 10.5 μl purified water. Cycling conditions on the T100 Thermal Cycler (Bio-rad, Hercules, CA) were the same for all genes, except for ENOS: 95°C for 15 min, 50 cycles of 94°C for 30 s, 58°C (ENOS 56°C) for 30 s, 72°C for 30 s, followed by a final step of 72°C for 7 min and stored at 4°C. Due to the generally low yield of human DNA from stool samples, we optimized the PCR protocol to 50 cycles to increase the DNA amplification.
Pyrosequencing
Pyrosequencing on a Pyromark Q48 system (Qiagen) was performed according to manufacturer's protocol followed by analysis using PyroMark Q48 Advanced Software (Qiagen). All samples were quality control checked and the percentage of DNA methylation at every CpG position calculated. Samples that failed quality control were excluded from data analysis.
Statistical Analysis
Statistical analysis was performed using SPSS for Windows, version 23.0 (IBM Corp., Armonk, New York). Visual inspection of a Q-Q plot as well as a Shapiro-Wilk test were used to assess normality, with a p-value of <0.05 to be considered as non-normal distribution. Next, a parametric or non-parametric approach was chosen as appropriate. In case of a small sample size (n <5), we also chose a non-parametric approach. Continuous variables were assessed using a Mann-Whitney U, Kruskal-Wallis or Student's T-test. Categorical variables were analyzed using a Chi-Square test or a Fisher's Exact Test in case of an expected cell count below 5 and correlations were assessed using Pearson's Test or Spearman's Rho. Results with a p < 0.05 (two-tailed) were considered to be statistically significant. GraphPad Prism 7.02 was used to construct all figures.
Results
This study cohort consisted of 24 infants with NEC and 48 controls. DNA isolation for three infants in the control group did not yield enough human DNA for further analysis. Therefore, the final control group consisted of 45 infants. The characteristics of the NEC infants and the control group are presented in Table 2. The median gestational age of the cohort was 27.4 weeks (IQR = 26.0–28.3) with a median birth weight of 920 grams (IQR = 780–1,075).
DNA Methylation Prior to NEC, Comparing NEC Infants With Controls
Samples reflecting methylation prior to NEC onset (SBN and LBN, n = 19) were taken a median of 2 days (IQR = 1–11) before NEC onset. Due to varying DNA quality, not all infants have DNA methylation results available for all five genes. The number of NEC infants and controls included for each gene as well as the mean DNA methylation percentages are presented in Table 3.
For the genes studied, we found a difference comparing the individual CpG positions of TLR4, which is involved in intestinal immunity through pattern recognition receptors, for NEC infants with controls. The DNA methylation of the individual CpG positions for all genes is displayed in Figure 2. TLR4 CpG position 2 showed a significantly higher methylation (p = 0.025) in the NEC group (median = 75.4%, IQR = 71.3–83.8) than in the control group (median = 69.0%, IQR = 64.5–77.4). These differences did not lead to consistent changes in the mean methylation of the 2 CpG positions studied for TLR4 between NEC infants and controls (Table 3). We noticed two outliers (>3 sd) in the control group. We repeated our analysis without these outliers and found similar results. No differences were found comparing the DNA methylation of EPO, VEGFA, DEFA5, and ENOS prior to NEC with controls.
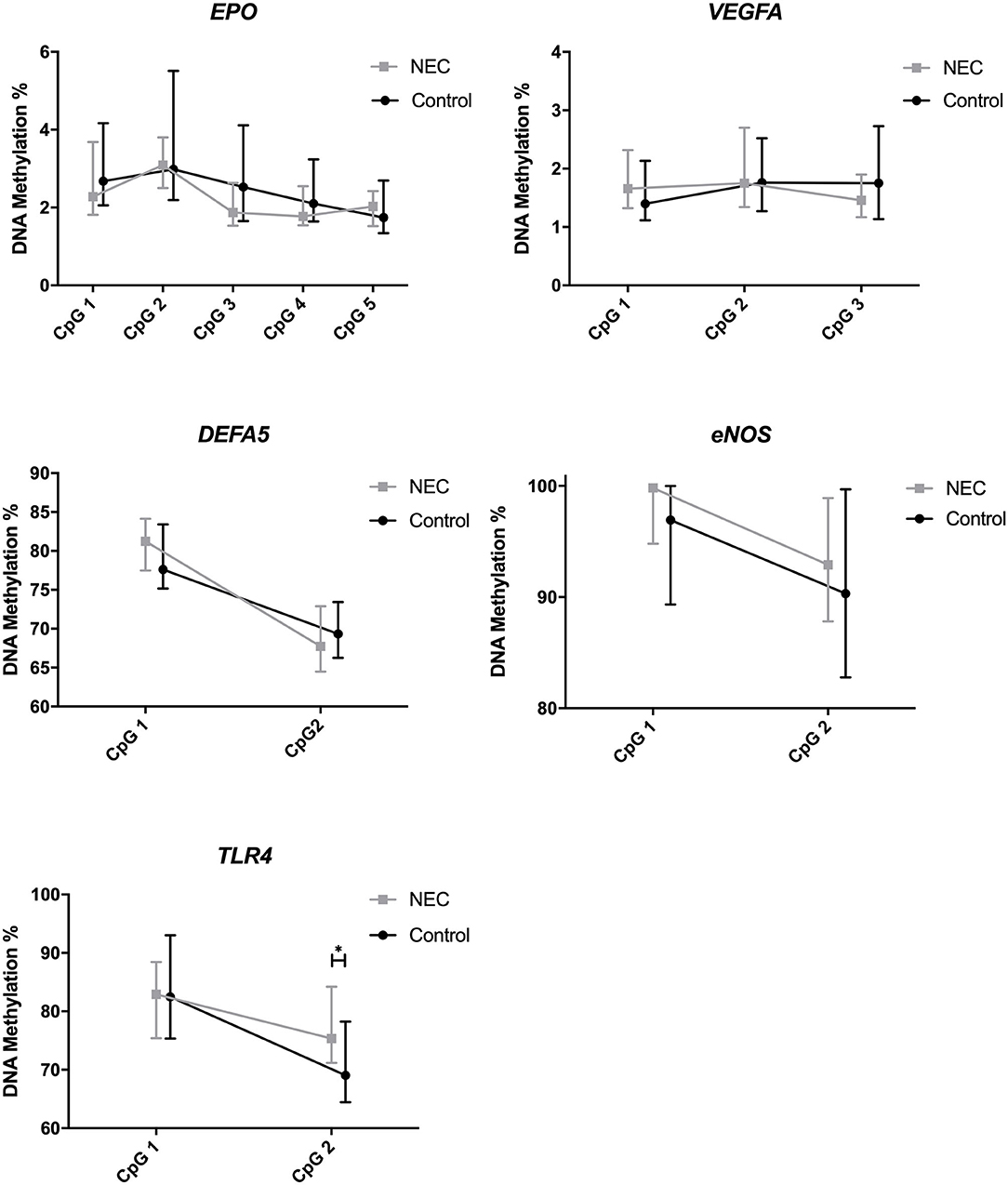
Figure 2. DNA methylation of EPO, VEGFA, DEFA5, ENOS, and TLR4, including individual CpG positions. Presented as median and IQR. * = P <0.05. NEC, Necrotizing Enterocolitis.
DNA Methylation in NEC Infants at Three Separate Time Frames
The characteristics of the NEC infants in the LBN, SBN, and AN group are presented in Table 4. NEC infants in the SBN group (n = 14) were significantly younger (median postnatal age = 12 days, IQR = 9–19) at the time of sample, compared with NEC infants in the LBN group (n = 5, median = 26 days, IQR = 24–27, p = 0.003) and the AN group (n = 5, median = 24 days, IQR = 21–27, p = 0.007).
We found a significant hypermethylation in the SBN and AN group for individual CpG positions of VEGFA and DEFA5. VEGFA is involved in intestinal perfusion, while DEFA5 is involved in intestinal immunity. Figure 3 shows the DNA methylation of VEGFA CpG position 3 and DEFA5 CpG position 1 in the three different groups. The number of infants included in each group as well as the DNA methylation percentages for all genes can be found in Supplementary Table 1.
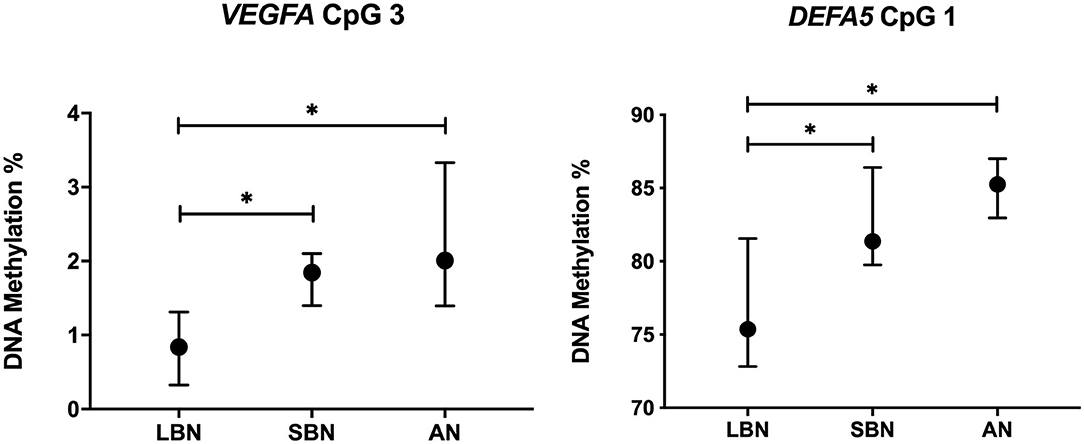
Figure 3. DNA methylation long before, a short time before and after NEC onset of VEGFA CpG 3 and DEFA5 CpG 1. Presented as median and IQR. * = P <0.05. LBN, Long Before NEC, SBN, a Short time Before NEC; AN, After NEC; NEC, Necrotizing Enterocolitis.
Analyses of the individual CpG positions revealed a significant hypermethylation in VEGFA CpG 3 (p = 0.011) of infants in the SBN group (n = 14, median = 1.8%, IQR = 1.4–2.1) compared with infants in the LBN group (n = 5, median = 0.8%, IQR = 0.7–1.2). A significantly higher methylation (p = 0.021) was also found for infants in the AN group (n = 5, median = 2.0%, IQR = 1.5–2.1) compared with infants in the LBN group. Similarly, for DEFA5 CpG 1, infants in the SBN group (n = 9, median = 81.4%, IQR = 79.9–84.8) showed a significantly higher methylation (p = 0.027) than infants in the LBN group (n = 4, median = 75.4%, IQR = 73.5–79.6). Comparing DEFA5 CpG 1 for NEC infants in the AN group (n = 4, median = 85.3%, IQR = 83.4–86.8) with those in the LBN group also showed a significantly higher DNA methylation (p = 0.019).
Due to the selection procedure, postnatal age of the NEC infants differed between the three groups. We calculated the correlation coefficients between DNA methylation and postnatal age. DNA methylation for both VEGFA CpG 3 and DEFA5 CpG 1 did not correlate with postnatal age (rho = −0.07, p = 0.74 and rho = −0.13, p = 0.63, respectively). We also calculated the correlation coefficients for DNA methylation of VEGFA CpG 3 and DEFA5 CpG 1 and postnatal age in the control group. In this group, again DNA methylation for both VEGFA CpG 3 (n = 42) and DEFA5 CpG 1 (n = 26) did not correlate with postnatal age (rho = 0.08, p = 0.61 and rho = 0.16, p = 0.44, respectively).
Discussion
We compared DNA methylation of NEC infants with controls and found a hypermethylation in CpG position 2 of the TLR4 gene in intestinal epithelial cells of preterm infants, prior to NEC onset. Furthermore, we found DNA methylation of VEGFA CpG 3 and DEFA5 CpG 1 to be substantially higher in NEC infants a short time before and after NEC onset (SBN and AN) than long before NEC onset (LBN). We did neither find any differences in the DNA methylation of EPO and ENOS in NEC infants prior to NEC onset compared to controls, nor differences between the various time frames within the NEC group. The differences in DNA methylation of TLR4, VEGFA, and DEFA5 may partially explain why some preterm infants with a similar risk profile develop NEC, while others do not. This exploratory study is the first to demonstrate that epigenetic changes such as DNA methylation early in life may contribute to this increased susceptibility. In the next sections we will discuss and interpret our findings in more detail, for each gene individually.
Regarding TLR4, we found a hypermethylation of CpG position 2 prior to NEC onset (LBN and SBN) when comparing NEC infants with controls. CpG position 2 is partially regulated by regulating factor X1, a potent transcription suppressor (32). A negative correlation between DNA methylation at CpG position 2 and mRNA expression of TLR4 in CD14+ monocytes is reported in earlier studies (32). There, a decrease in DNA methylation of the non-affected group is associated with almost a doubling of TLR4 expression. The difference in methylation between both groups is around twice the size of the difference we found in the current study. Translating their findings to our own results, hypermethylation at CpG position 2 would still suggest a substantial decrease in the expression of TLR4 in intestinal cells prior to NEC onset compared with controls. The downregulation of TLR4 may cause an increased susceptibility for developing NEC, as it causes a weakened first line of defense against infiltration of pathogens, a well-known cornerstone of NEC development. Similar to our findings, a recent study shows a 13% increase of DNA methylation in the promotor region of TLR4 in surgically resected NEC colon (8). Here, TLR4 was one of several genes within the pathway of pattern recognition receptors that was hypermethylated compared to controls. While this hypermethylation suggests a downregulation of TLR4, no significant increase or decrease in TLR4 expression was reported.
Further evidence on TLR4 methylation and expression in human tissue is limited. A higher expression of TLR4 is present in human cell lines after NEC induction using experimental hypoxia and LPS (34). Furthermore, TLR4 is expressed at higher levels in healthy preterm-born infants than in term-born infants and TLR4 is assumed to be critical for fetal gut development in utero (35). Most of the evidence of TLR4 involvement in NEC has been found in animal studies. The main findings include an increased expression of TLR4 messenger RNA and protein in mice intestine after NEC induction and the inability to induce NEC in TLR4 deficient mice (18, 20, 34, 36). The collective hypothesis of these studies suggests that the increased expression of TLR4 causes an exaggerated TLR4 activation and inflammatory response after bacterial colonization of the gut.
Concluding, TLR4 is needed for mounting an adequate inflammatory response, therefore a decrease in TLR4 might be detrimental because the inflammatory response might become inadequate, but on the other hand overactivation of TLR4 might lead to an exaggerated inflammatory response inducing intestinal injury as seen in NEC. This “balancing-act” by TLR4 needs further elucidation. Our findings suggest a downregulation in TLR4 before NEC onset. Because of the lack of expression data in our study we can just urge that further studies into the regulation of TLR4 regulation are necessary. Methylation changes in other areas or other concurrent epigenetic regulations, which were not evaluated here, may interfere with TLR4 expression.
A second finding in this study was the hypermethylation, within our NEC infants, of VEGFA CpG position 3 and DEFA5 CpG position 1 a short time before NEC onset and after NEC. Increasing methylation in VEGFA CpG position 3 is negatively associated with VEGFA expression (27). This is possibly due to the nearby binding site of hypoxia inducible factor 1A (HIF1A), a transcriptional factor known to suppress expression through hypermethylation (37). Similar to previous studies, the differences in DNA methylation percentages we found between groups were small. However, in previous studies a difference of several percentages is associated with an increase in VEGFA expression of 50–100% (27). Therefore, the hypermethylation of VEGFA CpG 3 we found in the period a short time before and after NEC suggests a reduced VEGFA expression due to NEC. Reduction in this angiogenic factor could result in an underdeveloped microvasculature of the intestine and poor perfusion a short time before onset and during or after NEC. Our findings are in line with results from experimental NEC models, in which a lower VEGFA expression is reported as well as a decrease in the VEGFA protein in intestinal tissue around NEC (14). We support the hypothesis that a decrease in VEGFA expression, possibly through DNA hypermethylation, increases the risk of developing NEC. Furthermore, continued downregulation of VEGFA after NEC onset may impair the recovery process.
Regarding DEFA5, we found similar results for its CpG position 1, with hypermethylation in the samples of our NEC group a short time before and after NEC onset compared with long before NEC onset. A negative relation between DNA methylation of the DEFA5 promoter and its expression is reported in intestinal cells of Crohn patients (30). In this study, even small differences of several percentages in DNA methylation are associated with a 25–45% decrease in DEFA5 expression. Extending these results to ours would imply that DEFA5 expression is lowered from a short time before NEC onset onwards. A decreased expression would result in a lowered secretion of defensins, the antimicrobial agents produced by Paneth cells. This relative depletion in defensins could result in a weakened defense system, increasing the infant's risk for developing NEC. Currently, there are two contradicting hypotheses for the role of Paneth cells and DEFA5 expression in NEC. The first hypothesis consists of an increased DEFA5 expression, resulting in an increased inflammatory response as seen in NEC (38). Supporting this hypothesis is an increase of immune-competent Paneth cells and DEFA5 expression starting at 29 weeks of gestation, corresponding with the peak incidence of NEC (38). The second hypothesis suggests a relative depletion or dysfunction of Paneth cells and a lowered DEFA5 expression in NEC (39). This hypothesis is supported by animal studies using Paneth cell ablation to induce necrotizing enterocolitis in mice and the markedly diminished expression of defensins in preterm-born infants (39). Our results are more in line with this second hypothesis. In the inflamed intestinal cells of Crohn patients, a reduction of defensin expression is seen together with a hypermethylation of the promotor region of DEFA5 (30). Here, the reduction of defensins is hypothesized to be a consequence of inflammatory changes, rather than the cause. It may be that a similar mechanism causes the hypermethylation we found in our study.
We did not find any differences in the DNA methylation of EPO and ENOS. For EPO we saw an overall low DNA methylation level, with little variation between subjects. If any differences between NEC infants and controls, or within the NEC group are present, it would probably require a larger sample size to detect these differences. We saw the opposite for ENOS, with generally high methylation levels, suggesting an overall low expression in intestinal cells. To detect any differences of ENOS methylation in NEC infants, possibly a cell type in which ENOS is expressed at a higher level should be used. Further studies should explore this.
The epigenetic changes we found may be related to environmental stressors known to be involved in NEC. For example, hypoxia can induce changes in DNA methylation of VEGFA, partially through hypoxia inducible factors, such as HIF1A (40). Furthermore, microbiota alterations are associated with differences in DNA methylation of TLR4 and have also been linked with Paneth cell dysfunction (6, 41). As we did not evaluate these factors in the current study, we can only suggest their role in causing changes in DNA methylation.
An important additional finding and strength of this study is the novel non-invasive method we used to investigate the bowel of NEC infants. Until now, DNA analyses of stool samples of preterm infants were solely used for microbiome analysis. In this pilot study we extracted human DNA from intestinal cells in stool samples. The majority of fecal samples were taken right before NEC diagnosis, a median of 1 day before NEC onset in the largest group (SBN). This ensures a DNA methylation profile as close as possible to NEC onset. DNA methylation analysis by targeted pyrosequencing includes a sequence-specific PCR step, which precludes bacterial DNA from the analysis. Studies using human material for NEC research are limited and groups are often small. Collecting intestinal cells directly from the intestinal wall is only possible if an infant requires surgery. This occurs in around one third of NEC infants and virtually no healthy preterm infants, making it difficult to collect control group samples (42). A recent study used surgical samples in combination with laser capture microdissection, to measure epithelial cell-specific methylation (8). Their findings include a hypermethylation of TLR4 similar to our findings in this manuscript. This increases our confidence that the DNA methylation we measure is mainly from epithelial cells in stool. Using stool samples for DNA collection resulted in a relatively large and well-matched NEC-infant and control group in our study. While this study proves the use of stool samples as a non-invasive method for collecting human DNA in preterm NEC infants and controls, more research has to be done to further validate this method and determine the relation with gene expression.
We acknowledge several limitations of our study. First, it was not possible to include measurements of gene expression, as RNA is far less stable than DNA and degenerates quickly in stool samples. Therefore, we need to rely on literature for the extrapolation of our data to physiological function. Previous studies, however, have investigated the relation between methylation on the promotor regions of TLR4, VEGFA, and DEFA5 and their gene expression. These studies all reveal a negative association between methylation and gene expression, which is in line with the general dogma that hypermethylation of promoter areas results in gene suppression. We therefore assumed this relation also existed in our cohort and that our findings are reflecting gene expression. In addition, we included several important CpG positions in the promotor region of each gene, but other CpG rich areas exist, in which similar or contrasting changes may occur. It is important to note that alongside DNA methylation, other epigenetic mechanisms such as histone modification and non-coding RNA regulate gene expression (43). We did not investigate these epigenetic mechanisms in this pilot study.
A second limitation concerns the low human DNA concentrations. Due to this we did not manage to successfully extract DNA from each stool sample, which resulted in some missing data. Furthermore, several outliers were present in all five genes, with methylation percentages very different in comparison to the rest of the group. We do not have indications that these were caused by analytical errors. As DNA methylation is known to be highly variable, we did not exclude these results from analysis. Even so, we also presented the results of the analyses excluding these outliers. A third limitation concerns multiple testing. As this was an exploratory study, we deliberately chose not to correct for multiple comparisons, acknowledging that some of our results may have been found by chance. In line with this, we did not perform multivariate testing due to the small sample size. Further larger prospective studies are warranted to confirm our results. Finally, we acknowledge that missing data may have biased our findings on the longitudinal part of our study, i.e., the changes of DNA methylation within the NEC infants, long before, a short time before and after NEC onset. We compared these three separate timeframes with varying sizes of samples and individuals. In addition, sample bias may unintentionally be present as a result of the variable onset of NEC in time. In future studies, prospectively and longitudinally collected stool samples of the same infants are required to confirm the change in DNA methylation over time that we found.
In conclusion, this is the first study to show differences in DNA methylation of TLR4, VEGFA, and DEFA5 in infants affected by necrotizing enterocolitis. This partially supports our hypothesis that epigenetic modifications contribute to the increased susceptibility of some infants for developing NEC. Whether the observed differences are sufficient to alter gene expression and indeed are causally related to NEC should be explored in future studies.
Data Availability Statement
The raw data supporting the conclusions of this article will be made available by the authors, without undue reservation.
Ethics Statement
The studies involving human participants were reviewed and approved by Medical Ethical Review Board of the University Medical Center Groningen. Written informed consent to participate in this study was provided by the participants' legal guardian/next of kin.
Author Contributions
DK, TP, EK, and AB conceptualized and designed the study. DK and RV-S collected the data. DK analyzed the data and drafted the initial manuscript. RV-S, TP, EK, and AB supervised the study. RV-S, TP, JH, EK, and AB reviewed and revised the manuscript critically. All authors approved the final manuscript as submitted.
Conflict of Interest
The authors declare that the research was conducted in the absence of any commercial or financial relationships that could be construed as a potential conflict of interest.
Acknowledgments
We would like to thank the nurses and medical staff of the NICU of the Beatrix Children's Hospital in Groningen, as well as the laboratory staff of the Obstetrics laboratory for helping with the data collection and for creating the opportunity to carry out this study.
Supplementary Material
The Supplementary Material for this article can be found online at: https://www.frontiersin.org/articles/10.3389/fped.2021.630817/full#supplementary-material
References
1. Lin PW, Stoll BJ. Necrotising enterocolitis. Lancet. (2006) 368:1271–83. doi: 10.1016/S0140-6736(06)69525-1
2. Heida FH, van Zoonen AGJF, Hulscher JBF, Te Kiefte BJC, Wessels R, Kooi EMW, et al. A necrotizing enterocolitis-associated gut microbiota is present in the meconium: results of a prospective study. Clin Infect Dis. (2016) 62:863–70. doi: 10.1093/cid/ciw016
3. Samuels N, van de Graaf RA, de Jonge RCJ, Reiss IKM, Vermeulen MJ. Risk factors for necrotizing enterocolitis in neonates: a systematic review of prognostic studies. BMC Pediatr. (2017) 17:105. doi: 10.1186/s12887-017-0847-3
4. Neu J. Necrotizing enterocolitis: the mystery goes on. Neonatology. (2014) 106:289–95. doi: 10.1159/000365130
5. Cutfield WS, Hofman PL, Mitchell M, Morison IM. Could epigenetics play a role in the developmental origins of health and disease? Pediatr Res. (2007) 61:68R−75R. doi: 10.1203/pdr.0b013e318045764c
6. Takahashi K, Sugi Y, Nakano K, Tsuda M, Kurihara K, Hosono A, et al. Epigenetic control of the host gene by commensal bacteria in large intestinal epithelial cells. J Biol Chem. (2011) 286:35755–62. doi: 10.1074/jbc.M111.271007
7. Merid SK, Novoloaca A, Sharp GC, Küpers LK, Kho AT, Roy R, et al. Epigenome-wide meta-analysis of blood DNA methylation in newborns and children identifies numerous loci related to gestational age. Genome Med. (2020) 12:25. doi: 10.1186/s13073-020-0716-9
8. Good M, Chu T, Shaw P, McClain L, Chamberlain A, Castro C, et al. Global hypermethylation of intestinal epithelial cells is a hallmark feature of neonatal surgical necrotizing enterocolitis. Clin Epigenetics. (2020) 12:190. doi: 10.1186/s13148-020-00983-6
9. Gao F, Zhang J, Jiang P, Gong D, Wang JW, Xia Y, et al. Marked methylation changes in intestinal genes during the perinatal period of preterm neonates. BMC Genomics. (2014) 15:716. doi: 10.1186/1471-2164-15-716
10. Ng PC, Chan KYY, Leung KT, Tam YH, Ma TPY, Lam HS, et al. Comparative MiRNA expressional profiles and molecular networks in human small bowel tissues of necrotizing enterocolitis and spontaneous intestinal perforation. PLoS ONE. (2015) 10:e0135737. doi: 10.1371/journal.pone.0135737
11. Shiou SR, Yu Y, Chen S, Ciancio MJ, Petrof EO, Sun J, et al. Erythropoietin protects intestinal epithelial barrier function and lowers the incidence of experimental neonatal necrotizing enterocolitis. J Biol Chem. (2011) 286:12123–32. doi: 10.1074/jbc.M110.154625
12. Sullivan S, Schanler RJ, Kim JH, Patel AL, Trawöger R, Kiechl-Kohlendorfer U, et al. An exclusively human milk-based diet is associated with a lower rate of necrotizing enterocolitis than a diet of human milk and bovine milk-based products. J Pediatr. (2010) 156:562–7.e1. doi: 10.1016/j.jpeds.2009.10.040
13. Bowker RM, Yan X, De Plaen IG. Intestinal microcirculation and necrotizing enterocolitis: the vascular endothelial growth factor system. Semin Fetal Neonatal Med. (2018) 23:411–5. doi: 10.1016/j.siny.2018.08.008
14. Sabnis A, Carrasco R, Liu SX, Yan X, Managlia E, Chou PM, et al. Intestinal vascular endothelial growth factor is decreased in necrotizing enterocolitis. Neonatology. (2015) 107:191–8. doi: 10.1159/000368879
15. Cuna A, Sampath V. Genetic alterations in necrotizing enterocolitis. Semin Perinatol. (2017) 41:61–9. doi: 10.1053/j.semperi.2016.09.019
16. Nowicki PT, Caniano DA, Hammond S, Giannone PJ, Besner GE, Reber KM, et al. Endothelial nitric oxide synthase in human intestine resected for necrotizing enterocolitis. J Pediatr. (2007) 150:40–5. doi: 10.1016/j.jpeds.2006.09.029
17. Underwood MA. Paneth cells and necrotizing enterocolitis. Gut Microbes. (2012) 3:562–5. doi: 10.4161/gmic.21738
18. Hackam DJ, Sodhi CP. Toll-Like Receptor-Mediated Intestinal Inflammatory Imbalance in the Pathogenesis of Necrotizing Enterocolitis. Cell Mol Gastroenterol Hepatol. (2018) 6:229–38.e1. doi: 10.1016/j.jcmgh.2018.04.001
19. Sodhi CP, Neal MD, Siggers R, Sho S, Ma C, Branca MF, et al. Intestinal epithelial Toll-like receptor 4 regulates goblet cell development and is required for necrotizing enterocolitis in mice. Gastroenterology. (2012) 143:708–18.e5. doi: 10.1053/j.gastro.2012.05.053
20. Lu Y-C, Yeh W-C, Ohashi PS. LPS/TLR4 signal transduction pathway. Cytokine. (2008) 42:145–51. doi: 10.1016/j.cyto.2008.01.006
21. De Meij TGJ, Van Der Schee MPC, Berkhout DJC, Van De Velde ME, Jansen AE, Kramer BW, et al. Early detection of necrotizing enterocolitis by fecal volatile organic compounds analysis. J Pediatr. (2015) 167:562–7.e1. doi: 10.1016/j.jpeds.2015.05.044
22. van der Flier LG, Clevers H. Stem cells, self-renewal, and differentiation in the intestinal epithelium. Annu Rev Physiol. (2009) 71:241–60. doi: 10.1146/annurev.physiol.010908.163145
23. Chapkin RS, Zhao C, Ivanov I, Davidson LA, Goldsby JS, Lupton JR, et al. Noninvasive stool-based detection of infant gastrointestinal development using gene expression profiles from exfoliated epithelial cells. Am J Physiol Gastrointest Liver Physiol. (2010) 298:G582–9. doi: 10.1152/ajpgi.00004.2010
24. Chandel DS, Braileanu GT, Chen JHJ, Chen HH, Panigrahi P. Live colonocytes in newborn stool: surrogates for evaluation of gut physiology and disease pathogenesis. Pediatr Res. (2011) 70:153–8. doi: 10.1203/PDR.0b013e3182225ac9
25. Stinson LF, Keelan JA, Payne MS. Comparison of meconium DNA extraction methods for use in microbiome studies. Front Microbiol. (2018) 9:270. doi: 10.3389/fmicb.2018.00270
26. Steinmann K, Richter AM, Dammann RH. Epigenetic silencing of erythropoietin in human cancers. Genes Cancer. (2011) 2:65–73. doi: 10.1177/1947601911405043
27. Sundrani DP, Reddy US, Joshi AA, Mehendale SS, Chavan-Gautam PM, Hardikar AA, et al. Differential placental methylation and expression of VEGF, FLT-1 and KDR genes in human term and preterm preeclampsia. Clin Epigenetics. (2013) 5:6. doi: 10.1186/1868-7083-5-6
28. Karantzoulis-Fegaras F, Antoniou H, Lai SL, Kulkarni G, D'Abreo C, Wong GK, et al. Characterization of the human endothelial nitric-oxide synthase promoter. J Biol Chem. (1999) 274:3076–93. doi: 10.1074/jbc.274.5.3076
29. Kheirandish-Gozal L, Khalyfa A, Gozal D, Bhattacharjee R, Wang Y. Endothelial dysfunction in children with obstructive sleep apnea is associated with epigenetic changes in the eNOS gene. Chest. (2013) 143:971–7. doi: 10.1378/chest.12-2026
30. Cerrillo E, Moret I, Iborra M, Ramos D, Busó E, Tortosa L, et al. Alpha-defensins (α-Defs) in Crohn's disease: decrease of ileal α-Def 5 via permanent methylation and increase in plasma α-Def 1-3 concentrations offering biomarker utility. Clin Exp Immunol. (2018) 192:120–8. doi: 10.1111/cei.13085
31. Kim TW, Lee S-J, Oh BM, Lee H, Uhm TG, Min J-K, et al. Epigenetic modification of TLR4 promotes activation of NF-κB by regulating methyl-CpG-binding domain protein 2 and Sp1 in gastric cancer. Oncotarget. (2016) 7:4195. doi: 10.18632/oncotarget.6549
32. Du P, Gao K, Cao Y, Yang S, Wang Y, Guo R, et al. RFX1 downregulation contributes to TLR4 overexpression in CD14+ monocytes via epigenetic mechanisms in coronary artery disease. Clin Epigenetics. (2019) 11:44. doi: 10.1186/s13148-019-0646-9
33. Hoftiezer L, Hof MHP, Dijs-Elsinga J, Hogeveen M, Hukkelhoven CWPM, van Lingen RA. From population reference to national standard: new and improved birthweight charts. Am J Obstet Gynecol. (2019) 220:383.e1–17. doi: 10.1016/j.ajog.2018.12.023
34. Leaphart CL, Cavallo J, Gribar SC, Cetin S, Li J, Branca MF, et al. A critical role for TLR4 in the pathogenesis of necrotizing enterocolitis by modulating intestinal injury and repair. J Immunol. (2007) 179:4808–20. doi: 10.4049/jimmunol.179.7.4808
35. Meng D, Zhu W, Shi HN, Lu L, Wijendran V, Xu W, et al. Toll-like receptor-4 in human and mouse colonic epithelium is developmentally regulated: a possible role in necrotizing enterocolitis. Pediatr Res. (2015) 77:416–24. doi: 10.1038/pr.2014.207
36. Hackam DJ, Good M, Sodhi CP. Mechanisms of gut barrier failure in the pathogenesis of necrotizing enterocolitis: toll-like receptors throw the switch. Semin Pediatr Surg. (2013) 22:76–82. doi: 10.1053/j.sempedsurg.2013.01.003
37. Siddique AN, Nunna S, Rajavelu A, Zhang Y, Jurkowska RZ, Reinhardt R, et al. Targeted methylation and gene silencing of VEGF-A in human cells by using a designed Dnmt3a–Dnmt3L single-chain fusion protein with increased DNA methylation activity. J Mol Biol. (2013) 425:479–91. doi: 10.1016/j.jmb.2012.11.038
38. Heida FH, Beyduz G, Bulthuis ML, Kooi EMW, Bos AF, Timmer A, et al. Paneth cells in the developing gut: when do they arise and when are they immune competent? Pediatr Res. (2016) 80:306–10. doi: 10.1038/pr.2016.67
39. McElroy SJ, Underwood MA, Sherman MP. Paneth cells and necrotizing enterocolitis: a novel hypothesis for disease pathogenesis. Neonatology. (2013) 103:10–20. doi: 10.1159/000342340
40. Choudhry H, Harris AL. Advances in hypoxia-inducible factor biology. Cell Metab. (2018) 27:281–8. doi: 10.1016/j.cmet.2017.10.005
41. Lueschow SR, Stumphy J, Gong H, Kern SL, Elgin TG, Underwood MA, et al. Loss of murine Paneth cell function alters the immature intestinal microbiome and mimics changes seen in neonatal necrotizing enterocolitis. PLoS ONE. (2018) 13:e0204967. doi: 10.1371/journal.pone.0204967
42. Heida FH, Stolwijk L, Loos MH, van den Ende SJ, Onland W, van den Dungen FA, et al. Increased incidence of necrotizing enterocolitis in the Netherlands after implementation of the new Dutch guideline for active treatment in extremely preterm infants: results from three academic referral centers. J Pediatr Surg. (2017) 52:273–6. doi: 10.1016/j.jpedsurg.2016.11.024
Keywords: neonatology, DNA methylation, epigenetics, preterm infant, necrotizing enterocolitis, Toll-like receptor 4, vascular endothelial growth factor A, defensin alpha 5
Citation: Klerk DH, Plösch T, Verkaik-Schakel RN, Hulscher JBF, Kooi EMW and Bos AF (2021) DNA Methylation of TLR4, VEGFA, and DEFA5 Is Associated With Necrotizing Enterocolitis in Preterm Infants. Front. Pediatr. 9:630817. doi: 10.3389/fped.2021.630817
Received: 18 November 2020; Accepted: 11 February 2021;
Published: 04 March 2021.
Edited by:
Maximo Vento, La Fe Hospital, SpainReviewed by:
Janet Elizabeth Berrington, Newcastle upon Tyne Hospitals NHS Foundation Trust, United KingdomMaryAnn Volpe, Tufts University School of Medicine, United States
Copyright © 2021 Klerk, Plösch, Verkaik-Schakel, Hulscher, Kooi and Bos. This is an open-access article distributed under the terms of the Creative Commons Attribution License (CC BY). The use, distribution or reproduction in other forums is permitted, provided the original author(s) and the copyright owner(s) are credited and that the original publication in this journal is cited, in accordance with accepted academic practice. No use, distribution or reproduction is permitted which does not comply with these terms.
*Correspondence: Daphne H. Klerk, d.h.klerk@umcg.nl