- 1Children’s Health and Exercise Research Centre, Public Health and Sports Sciences, University of Exeter Medical School, University of Exeter, Exeter, United Kingdom
- 2Royal Devon University Healthcare NHS Foundation Trust, Exeter, United Kingdom
- 3Biomedical and Clinical Science, University of Exeter Medical School, University of Exeter, Exeter, United Kingdom
Background: Increased maximal oxygen uptake (V̇O2max) is beneficial in children with cystic fibrosis (CF) but remains lower compared to healthy peers. Intrinsic metabolic deficiencies within skeletal muscle (muscle “quality”) and skeletal muscle size (muscle “quantity”) are both proposed as potential causes for the lower V̇O2max, although exact mechanisms remain unknown. This study utilises gold-standard methodologies to control for the residual effects of muscle size from V̇O2max to address this “quality” vs. “quantity” debate.
Methods: Fourteen children (7 CF vs. 7 age- and sex-matched controls) were recruited. Parameters of muscle size – muscle cross-sectional area (mCSA) and thigh muscle volume (TMV) were derived from magnetic resonance imaging, and V̇O2max obtained via cardiopulmonary exercise testing. Allometric scaling removed residual effects of muscle size, and independent samples t-tests and effect sizes (ES) identified differences between groups in V̇O2max, once mCSA and TMV were controlled for.
Results: V̇O2max was shown to be lower in the CF group, relative to controls, with large ES being identified when allometrically scaled to mCSA (ES = 1.76) and TMV (ES = 0.92). Reduced peak work rate was also identified in the CF group when allometrically controlled for mCSA (ES = 1.18) and TMV (ES = 0.45).
Conclusions: A lower V̇O2max was still observed in children with CF after allometrically scaling for muscle size, suggesting reduced muscle “quality” in CF (as muscle “quantity” is fully controlled for). This observation likely reflects intrinsic metabolic defects within CF skeletal muscle.
1. Introduction
Higher levels of aerobic fitness [represented by maximal oxygen uptake (V̇O2max)] are associated with improved long-term outcomes [e.g., reduced risk of mortality and transplantation (1)] in children with cystic fibrosis (CF). It has further been shown that aerobic fitness is reduced in children with CF compared to healthy peers (2, 3), and whilst many physiological factors are associated with reduced V̇O2max in CF. Precise mechanisms remain unclear in relation to skeletal muscle (4), whose importance has been well established (5).
In particular, debate surrounds whether muscle size (affected by nutritional compromise, attenuated pubertal growth, and catabolism during pulmonary exacerbations) or its intrinsic function [via expression of the CF Transmembrane Conductance Regulator (CFTR) protein within the sarcoplasm (6)] contribute towards skeletal muscle metabolism and reduced V̇O2max in people with CF. This has resulted in a in a muscle “quantity” vs. “quality” debate (7, 8), although these two mechanisms are not guaranteed to be mutually exclusive.
In relation to the “quantity” aspect of this debate, previous studies have expressed V̇O2max relative to body mass and fat-free mass (FFM) using ratio-standard scaling, to account for differences in body size (2, 9, 10). However, this may be inappropriate, as: (a) these variables only provide surrogates for metabolically active muscle during exercise; and (b) the ratio between FFM and leg muscle volume (MV) is not proportional during periods of growth such as puberty (11) and therefore, FFM may be an inferior surrogate of MV during growth. Therefore, accounting for leg MV when assessing V̇O2peak in CF may be more appropriate than body mass and/or FFM as a parameter of body size.
Previous research has tried to account for muscle size in CF when assessing V̇O2max, by using ratio-standard scaling of muscle cross sectional area (mCSA), to identify reduced V̇O2max in CF relative to healthy controls (12). However, this is flawed because mCSA poorly represents total leg MV in youth (13), and allometric scaling models have been shown to be more effective in removing residual effects of body size, relative to ratio-standard models (14). Several scaling exponents have been derived previously, all specific to populations being examined (15), indicating the importance of this procedure in eliminating effects of body size from parameters of aerobic fitness, such as V̇O2max. Therefore, use of both MV as a scaling factor, and allometry as a scaling method, may better reflect body size when assessing V̇O2max and provide further insight into the quantitative arguments for the “quality vs. quantity” debate.
This study sought to utilise allometric scaling procedures to investigate whether V̇O2max is reduced in CF compared to healthy controls after normalising for thigh MV (TMV) and thigh mCSA. Should V̇O2max remain lower in CF once muscle size is scaled for, this would proffer support for intrinsic muscular defects in this population, as the scaling approach will have accounted for the quantitative effects.
2. Methods
2.1. Participants & ethics approval
Between 2015 and 2016, seven children with mild-to-moderate CF related lung disease (i.e., forced expiratory volume in one second [FEV1] ≥ 70% (16)) were recruited via convenience sampling from routine outpatient clinics and physiotherapy annual review appointments at a local CF centre. Once these participants had completed the study, age- and sex-matched non-CF control children were recruited from a local sports club and state secondary school. An NHS Research Ethics Committee provided ethics approval (14/SW/0061), with written informed consent and assent being obtained from parents/guardians and children respectively prior to participation. Inclusion and exclusion criteria for CF and control groups are provided in Supplementary File 1.
2.2. Experimental timeline
Participants underwent two separate visits to the laboratory at the University of Exeter. During an initial familiarisation visit to the laboratory, anthropometric measures, maturity status, lung function, and exercise function were assessed. Participants were also habituated to the magnetic resonance (MR) scanner environment.
2.3. Outcome measures
For assessment of maturity status, two approaches were used. Firstly, self-assessment of pubertal status using a validated scale of genital development (17) was undertaken following explanation from a researcher of the same sex, and returned via a sealed envelope. Secondly, via estimated age from peak height velocity (aPHV) using established equations (18), with participants being categorised as “pre-aPHV” or “post-aPHV”.
Lung function, obtained values of FEV1 and forced vital capacity (FVC) via hand-held spirometry, with outcomes normalised to global, sex-specific, multi-ethnic, reference values (19).
2.3.1. Cardiopulmonary exercise testing
Participants undertook a cardiopulmonary exercise test (CPET) to volitional exhaustion on an electronically braked cycle ergometer (Lode, Groningen, the Netherlands), using a combined ramp-incremental and supramaximal verification protocol, validated in youth with and without CF (20, 21) to establish a “true” maximal effort (i.e., V̇O2max). Breath-by-breath gas exchange data were collected (Cortex Metalyzer, Cranlea, UK), with V̇O2max taken as the highest 10-second average from either the ramp or supramaximal phase, in line with existing validation studies (20, 21). Identification of the gas exchange threshold (GET) was undertaken using the V-slope method (22) and maximal voluntary ventilation (MVV) was estimated by multiplying FEV1 by 35 (23). Data for V̇O2max and peak work rate (WRpeak) was normalised to “percent of predicted” using sex- and modality-specific equations developed in European youth (24).
For children with CF, CPET was undertaken at their physiotherapy annual review, whereby data from this test was obtained retrospectively from medical records to avoid undue burden via multiple tests. For control children, CPET was undertaken in the laboratory at the host institution as previously noted. The same equipment was used in both locations, overseen by the same investigator (OWT), with additional site-specific supervision from staff (PW, JS).
2.3.2. Muscle volume
On the second visit (∼1 week following familiarisation), participants were positioned prone within a 1.5 T superconducting whole-body MR scanner (Gyroscan Intera, Philips, the Netherlands). A T1 weighted image sequence optimised fat/muscle signal contrast, obtaining a stack of axial images from below the knee to above the hip, with TMV and mCSA quantified using methods previously described (13).
2.3.3. Physical activity
Physical activity (PA) was monitored via (non-dominant) wrist-mounted accelerometry for one week (GENEActiv, Activinsights, UK), with time spent being sedentary and in moderate-vigorous activity calculated using age-appropriate cut points (25), and maximal reliability coefficients for minimum daily wear time (26).
2.3.4. Scaling of VO2max
Allometric scaling removed residual effects of muscle size from VȮmax and WRpeak. This process obtains scaling exponents (b) and associated 95% confidence intervals (95% CI) from log-linear regressions to use as power functions and to which muscle size is raised (i.e., Y/Xb), with group included as a covariate within analyses (27). Allometric scaling has been widely used for controlling for body size in relation to interpretation of exercise performance (15), and is a process that has also been used in analysing parameters of aerobic fitness in CF (27).
2.4. Statistical analyses
Data are reported as mean ± standard deviation. Pearson's correlation coefficients established relationships between V̇O2max and WRpeak, and muscle size. Differences between groups were established using independent sample t-tests for normally distributed data (determined via Shapiro–Wilk testing), with differences between groups for non-normal data being identified via Mann–Whitney U tests. For all tests, p < 0.05 indicated statistical significance. Effect sizes (ES) using established thresholds from Cohen, 0.2 = (small), 0.5 = (medium), 0.8 = (large) (28), described magnitudes of differences between groups.
3. Results
Seven children with CF, and seven age- and sex-matched control children provided data, with a flow chart detailing participation included in Supplementary File 1. Within each group, aPHV data were available for n = 6 participants due to non-applicability of equations in females above 16 years of age. For the CF group, n = 2 and n = 4 were reported as pre- and post-aPHV respectively, and within the control group, n = 4 and n = 2 were reported as pre- and post-aPHV respectively.
Participants with CF had few comorbidities. All presented as pancreatic insufficient, and one presented with CF related diabetes. None were chronically infected with Pseudomonas aeruginosa. Participant characteristics and mean differences between groups for anthropometric, pulmonary, and MR-derived variables are listed in Table 1. Small ES were found between groups, with mCSA, TMV, FEV1 (%Pred) being higher in the CF group.
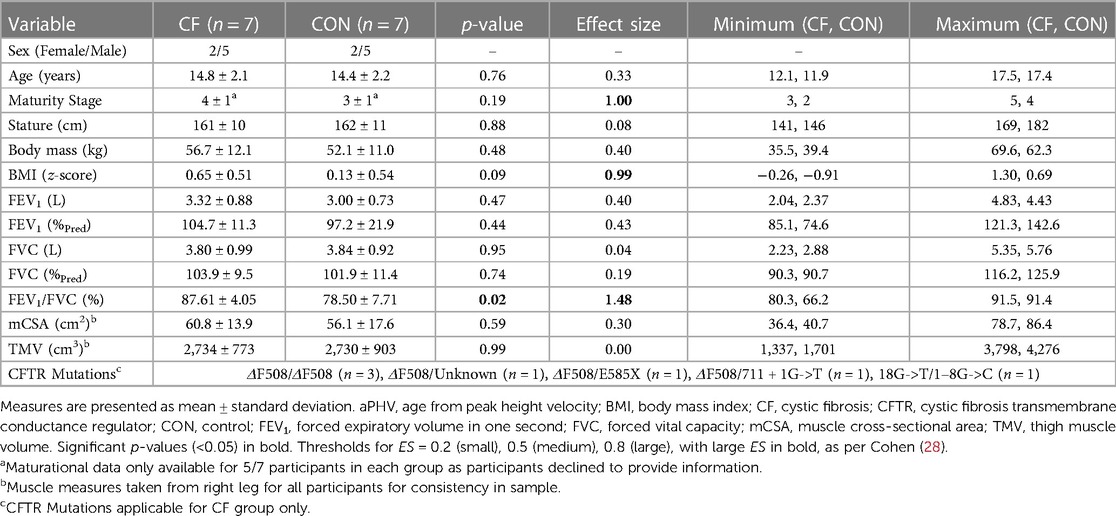
Table 1. Anthropometric, pulmonary, and magnetic resonance-derived muscle-related differences between CF and control groups.
Mean differences between groups for exercise data are found in Table 2, and PA data in Table 3. During CPET, 7/14 children obtained their highest V̇O2 in the ramp-incremental phase, 6/14 in the supramaximal phase (within accepted variation (20)), and 1/14 obtained the same value in both exercise bouts.
V̇O2max was highly correlated with mCSA (CF: r = 0.93, p < 0.01; control: r = 0.92, p < 0.01) and TMV in both groups (CF: r = 0.84, p < 0.05; control: r = 0.90, p < 0.01). WRpeak was also highly correlated with mCSA (CF: r = 0.93, p < 0.01; control: r = 0.96, p < 0.01) and TMV (CF: r = 0.87, p < 0.05; control: r = 0.94, p < 0.01) in both groups.
In relation to V̇O2max, log-linear regression produced scaling exponents for mCSA (b = 0.98, 95% CI = 0.66–1.30, p < 0.001) and TMV (b = 0.78, 95% CI = 0.47–1.10, p < 0.001). For WRpeak, log-linear regression produced scaling exponents for mCSA (b = 0.99, 95% CI = 0.67–1.30, p < 0.001) and TMV (b = 0.81, 95% CI = 0.52–1.10, p < 0.001). These exponents successfully removed residual effects of muscle size from V̇O2peak and WRpeak, evidenced by non-significant Pearson's correlations between scaled V̇O2peak and mCSA (r = −0.12, p = 0.68) and TMV (r = 0.03, p = 0.92), and scaled WRpeak and mCSA (r = −0.13, p = 0.65) and TMV (r = −0.004, p = 0.99).
Therefore, once these exponents were applied, a large ES was found between groups for both mCSA and TMV scaled V̇O2max, being higher in the control group (Figure 1). When used to scale WRpeak, a large ES was found between groups for mCSA but not TMV, but both values being higher in the control group (Figure 1).
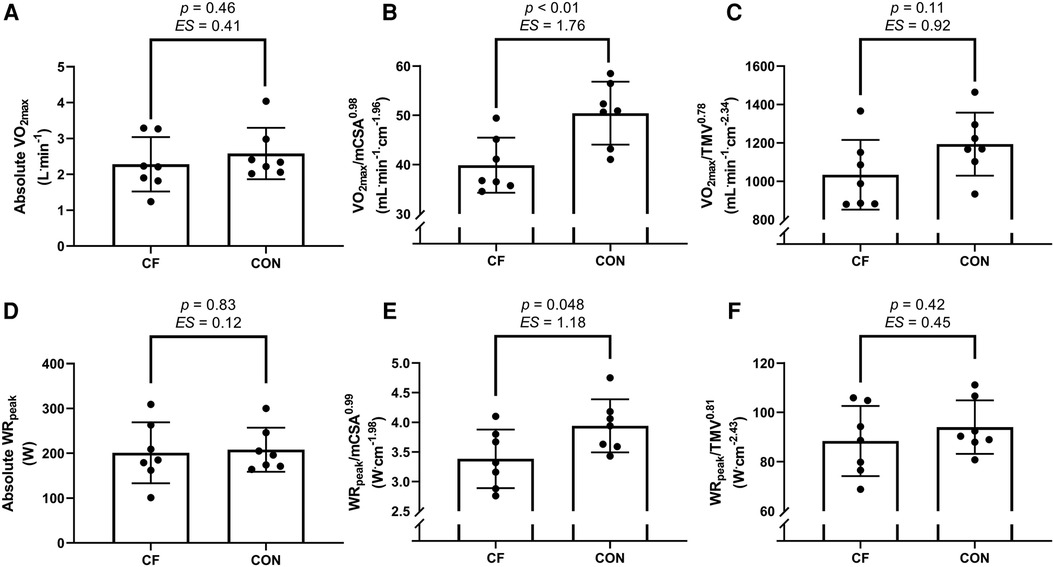
Figure 1. Mean differences between groups for parameters of V̇O2max (A–C) and WRpeak (D–F), presented as an absolute value (A,D) and when allometrically scaled to muscle cross sectional area (mCSA) of the right mid-thigh (B,E) and to thigh muscle volume (TMV) of the right leg (C,F). CF, cystic fibrosis; CON, control; ES, effect size; V̇O2max, maximal oxygen uptake, WRpeak, peak work rate. Data presented as mean ± standard deviation. Thresholds for ES = 0.2 (small), 0.5 (medium), 0.8 (large) as per Cohen (13).
4. Discussion
The main result of this study has shown that when parameters of muscle size are allometrically scaled for, a large difference (as shown by ES) in V̇O2max is found, whereby this is higher in the control group relative to CF. Thus, after robustly accounting for muscle “quantity”, a difference remains between groups and thus supports the case for muscle “quality” accounting for the reduced V̇O2max (and WR) in CF.
Previous work indicates a strong correlation (r = 0.89) between mCSA and V̇O2max in CF (12), agreeing with the coefficient found within the present work (r = 0.93). Moreover, the present investigation characterised the association between V̇O2max and TMV for the first time in CF, finding an equally large coefficient between variables (r = 0.84); a result that reflects prior findings in healthy children (29). These coefficients show a clear relationship between parameters, and therefore use of muscle size to scale V̇O2max is warranted.
In identifying reduced V̇O2max relative to age- and sex-matched peers, these findings corroborate previous research that identifies similar reductions in V̇O2max in children with CF when body mass and FFM (30) are accounted for. However, as previously noted, body mass and FFM are poor surrogates for metabolically active muscle (11) and therefore quantification of muscle size should be considered instead. Using muscle size, the present work supports previous findings that utilised mCSA (with a very similar scaling exponent of b = 1.03 to the present b = 0.98) to scale V̇O2max and find increased fitness in control children relative to those with CF (12). Moreover, when also scaling for TMV, the present work found a broadly similar scaling exponent (b = 0.78) to that previously found in healthy children (29) (b = 0.55; the present 95% CI encompasses this value). Within the present study, both groups presented with remarkably similar mean values, and thus when the scaling exponent is applied, collective findings of previous (12) and present work indicate “qualitative” defects in CF skeletal muscle once “quantitative” differences are controlled.
These novel, methodologically robust data, supports studies conducted in vitro that show that CFTR affects mitochondrial function, resting adenosine triphosphate levels and Ca2+ regulation (6, 31). Moreover, in vivo research corroborates these findings as CFTR expression within skeletal muscle is associated with upregulation of genes responsible for muscle atrophy (32) and prolonged phosphocreatine recovery following exercise (33). In addition, prior work undertaken in adolescents with CF indicates that exercise intolerance may be intensity-dependent, whereby moderate-intensity exercise does not differ between groups, but it is in the high-intensity domain where differences in oxidative metabolism emerge (30). This would be supported by the current work, as exercise was performed at maximal levels during CPET.
The data presented within the current study indicates a “qualitative” defect within skeletal muscle, adding evidence to the “quality” side of the “quality vs. quantity” debate (7, 8). However, contrasting studies show that muscle power is not different between people with CF and healthy controls when muscle size is accounted for (34, 35). However, muscular power, in these studies, has been obtained via Wingate testing (34) and via plantar flexion testing (35), and not via CPET. However, V̇O2peak remains impaired in these studies, thus evidencing that skeletal muscle “quality” likely impairs oxidative metabolism, if not force generation. Moreover, these prior studies (34, 35) include heterogenous patient groups with greater levels of pulmonary impairment and comorbidities, contrasting the present work whereby the relatively good health status of the CF group ensures that no additional disease associated complications (poor lung function, bacterial infection, sarcopenia, hypoxemia) confound results.
Whilst this study has a limited sample size (typical of research in clinical populations and those using costly MR techniques), several strengths remain. Notably, age- and sex-matching of participants ensured disease status remained the discerning characteristic between groups. Whilst matching via calendar age alone is flawed, particularly in youth, assessments of maturity status ensured that groups were broadly matched in terms of biological and somatic maturation as well. Moreover, participants with CF had preserved pulmonary function, as well as similar exercise function (i.e., VE/MVV, VO2/WR), physical activity status and muscle size parameters, and yet V̇O2max was still reduced relative to controls. This observation further indicates that predominantly skeletal muscle defects, and not necessarily pulmonary or cardiovascular factors, likely affect V̇O2max in CF.
High quality physiological techniques were used in this study, including gold-standard CPET and associated supramaximal verification bouts (21). The choice of the latter being vindicated by the observation that 43% of children achieved their highest V̇O2 during the supramaximal verification bout, thus enhancing the evidence base for why this additional verification is needed in paediatric studies. In addition to gold-standard CPET, MR imaging derived measures of mCSA and MV, and robust allometric scaling aided in the removal of residual effects of muscle size. All these testing and analytical procedures combined to create a robust design and analyses.
With regards to limitations, it is acknowledged that an element of selection bias may be present, as children were selected from a singular paediatric clinic in the UK. Therefore, it is possible that children who enjoy exercise were more likely to volunteer for an exercise-based study and thus the lack of individuals with more severe disease in this study does not necessarily reflect the wider, heterogenous, CF community. This is reflected by participants lacking chronic infection, as well as data in Tables 1, 3, whereby mean FEV1 > 100%Pred, and mean MVPA equated to >90 min per day, respectively. However, even with selection bias, and inclusion of nominally healthy and active children with CF, we still observed a large effect size between groups, indicating an inherent CF-related cause to exercise intolerance, which may become even more pronounced if children with less stable CF were included. We therefore call for replication of the current work to replicate these initial findings.
Finally, we acknowledge this work was conducted prior to the widespread introduction of CFTR modulators that may affect exercise function (4). However, as not all patients are eligible for modulator therapy either through ineligible genotype, clinical access arrangements, global cost and differences in healthcare systems or adverse reactions (36). Additionally, as there is no association between genotype and fitness (37) (which may be impacted by modulators if an association existed), the current findings retain applicability to a wide number of people with CF and to the continued exercise management of the disease. The subsequent impact of CFTR modulator therapy upon skeletal muscle function should be examined in this group to provide additional mechanistic insight into this “quality vs. quantity” debate.
5. Conclusion
In summary, through the use of gold-standard CPET and MR procedures, this study has found a reduced V̇O2max in children with CF once muscle size is fully accounted for, thus furthering evidence for the proposed intrinsic muscular defect in this population (7). Whilst this data does not wholly confirm a “qualitative” defect and that muscle “quality” and “quantity” may not be mutually exclusive, we recommend rehabilitation programmes and exercise training regimens should consider improvements in both muscle “quality” and “quantity” for people with CF.
Data availability statement
The raw data supporting the conclusions of this article will be made available by the authors, without undue reservation.
Ethics statement
The studies involving human participants were reviewed and approved by NRES Committee South West - Exeter (14/SW/0061). Written informed consent to participate in this study was provided by the participants’ legal guardian/next of kin.
Author contributions
OT, AB, JF, PO and CW: conceived and developed study. OT, JF, PW and JS: collected all data. OT: analysed data. OT, AB, PO and CW: interpreted data. OT: drafted manuscript. All authors contributed to the article and approved the submitted version.
Funding
The facility requirements for this study were financially supported by the Discipline of Sport & Health Science at the University of Exeter and the Royal Devon & Exeter CF Research Charitable Fund. In addition, Jonathan Fulford's salary was supported via an NIHR grant to the University of Exeter (CRF/2016/10027). No further funding is reported.
Acknowledgments
The authors would like to thank the staff of the Royal Devon and Exeter NHS Foundation Trust Hospital, Exmouth Community College, the Children's Health and Exercise Research Centre, and coaches at CrossFit Exeter for assistance in recruitment of participants and data collection. Furthermore, we would like to thank all the children and their families for their time devoted to this study.
Conflict of interest
The authors declare that the research was conducted in the absence of any commercial or financial relationships that could be construed as a potential conflict of interest.
Publisher's note
All claims expressed in this article are solely those of the authors and do not necessarily represent those of their affiliated organizations, or those of the publisher, the editors and the reviewers. Any product that may be evaluated in this article, or claim that may be made by its manufacturer, is not guaranteed or endorsed by the publisher.
Supplementary material
The Supplementary Material for this article can be found online at: https://www.frontiersin.org/articles/10.3389/fped.2023.1211547/full#supplementary-material
References
1. Pianosi P, LeBlanc J, Almudevar A. Peak oxygen uptake and mortality in children with cystic fibrosis. Thorax. (2005) 60(1):50–4. doi: 10.1136/thx.2003.008102
2. Saynor ZL, Barker AR, Oades PJ, Williams CA. Impaired aerobic function in patients with cystic fibrosis during ramp exercise. Med Sci Sports Exercise. (2014) 46(12):2271–778. doi: 10.1249/MSS.0000000000000369
3. Bongers BC, Werkman MS, Takken T, Hulzebos EH. Ventilatory response to exercise in adolescents with cystic fibrosis and mild-to-moderate airway obstruction. SpringerPlus. (2014) 3:696. doi: 10.1186/2193-1801-3-696
4. Caterini JE, Ratjen F, Barker AR, Williams CA, Rendall K, Schneiderman JE, et al. Exercise intolerance in cystic fibrosis-the role of CFTR modulator therapies. J Cyst Fibros. (2022) 21(2):282–92. doi: 10.1016/j.jcf.2021.11.011
5. Lands LC, Heigenhauser GJF, Jones NL. Analysis of factors limiting maximal exercise performance in cystic fibrosis. Clin Sci. (1992) 83(4):391–7. doi: 10.1042/cs0830391
6. Lamhonwah AM, Bear CE, Huan LJ, Kim Chiaw P, Ackerley CA, Tein I. Cystic fibrosis transmembrane conductance regulator in human muscle: dysfunction causes abnormal metabolic recovery in exercise. Ann Neurol. (2010) 67(6):802–8. doi: 10.1002/ana.21982
7. Rodriguez-Miguelez P, Erickson ML, McCully KK, Harris RA. Crosstalk proposal: skeletal muscle oxidative capacity is altered in patients with cystic fibrosis. Physiol J. (2017) 595(5):1423–5. doi: 10.1113/JP272486
8. Hulzebos HJ, Jeneson JA, van der Ent CK, Takken T. Crosstalk opposing view: skeletal muscle oxidative capacity is not altered in cystic fibrosis patients. Physiol J. (2017) 595(5):1427–8. doi: 10.1113/JP272505
9. Stevens D, Oades PJ, Armstrong N, Williams CA. Exercise metabolism during moderate-intensity exercise in children with cystic fibrosis following heavy-intensity exercise. Appl Physiol Nutr Metab. (2011) 36(6):920–7. doi: 10.1139/h11-117
10. Tucker MA, Berry B, Seigler N, Davison GW, Harris RA, Quindry JC, et al. Blood flow regulation and oxidative stress during submaximal cycling exercise in patients with cystic fibrosis. J Cyst Fibros. (2018) 17(2):256–63. doi: 10.1016/j.jcf.2017.08.015
11. Tolfrey K, Barker A, Thom JM, Morse CI, Narici MV, Batterham AM. Scaling of maximal oxygen uptake by lower leg muscle volume in boys and men. J Appl Physiol. (2006) 100(6):1851–6. doi: 10.1152/japplphysiol.01213.2005
12. Moser C, Tirakitsoontorn P, Nussbaum E, Newcomb R, Cooper DM. Muscle size and cardiorespiratory response to exercise in cystic fibrosis. Am J Respir Crit Care Med. (2000) 162(5):1823–7. doi: 10.1164/ajrccm.162.5.2003057
13. Tomlinson OW, Barker AR, Fulford J, Wilson P, Oades PJ, Williams CA. Quantification of thigh muscle volume in children and adolescents using magnetic resonance imaging. Eur J Sport Sci. (2020) 20(9):1215–24. doi: 10.1080/17461391.2019.1707292
14. Welsman J, Armstrong N. Interpreting aerobic fitness in youth: the fallacy of ratio scaling. Pediatr Exerc Sci. (2019) 31(2):184–90. doi: 10.1123/pes.2018-0141
15. Lolli L, Batterham AM, Weston KL, Atkinson G. Size exponents for scaling maximal oxygen uptake in over 6500 humans: a systematic review and meta-analysis. Sports Med. (2017) 47(7):1405–19. doi: 10.1007/s40279-016-0655-1
16. Cystic Fibrosis Foundation. Patient registry annual data report 2015. Bethesda, MD, United States: Cystic Fibrosis Foundation (2016).
17. Morris NM, Udry JR. Validation of a self-administered instrument to assess stage of adolescent development. J Youth Adolesc. (1980) 9(3):271–80. doi: 10.1007/BF02088471
18. Mirwald RL, Baxter-Jones ADG, Bailey DA, Beunen GP. An assessment of maturity from anthropometric measurements. Med Sci Sports Exercise. (2002) 34(4):689–94. doi: 10.1097/00005768-200204000-00020
19. Quanjer PH, Stanojevic S, Cole TJ, Baur X, Hall GL, Culver BH, et al. Multi-ethnic reference values for spirometry for the 3–95-year age range: the global lung function 2012 equations. Eur Respir J. (2012) 40(6):1324–43. doi: 10.1183/09031936.00080312
20. Saynor ZL, Barker AR, Oades PJ, Williams CA. A protocol to determine valid VO2max in young cystic fibrosis patients. J Sci Med Sport. (2013) 16(6):539–44. doi: 10.1016/j.jsams.2013.01.010
21. Barker AR, Williams CA, Jones AM, Armstrong N. Establishing maximal oxygen uptake in young people during a ramp cycle test to exhaustion. Br J Sports Med. (2011) 45(6):498–503. doi: 10.1136/bjsm.2009.063180
22. Beaver WL, Wasserman K, Whipp BJ. A new method for detecting anaerobic threshold by gas exchange. J Appl Physiol. (1986) 60(6):2020–7. doi: 10.1152/jappl.1986.60.6.2020
23. American Thoracic Society, American College of Chest Physicians. ATS/ACCP statement on cardiopulmonary exercise testing. Am J Respir Crit Care Med. (2003) 167(2):211–77. doi: 10.1164/rccm.167.2.211
24. Bongers BC, van Brussel M, Hulzebos EHJ, Takken T. Pediatric norms for cardiopulmonary exercise testing: In relation to sex and age. 2nd ed. ‘s-Herogenbosch, Netherlands: Uitgeverij BOXPress (2014).
25. Phillips LR, Parfitt G, Rowlands AV. Calibration of the GENEA accelerometer for assessment of physical activity intensity in children. J Sci Med Sport. (2013) 16(2):124–8. doi: 10.1016/j.jsams.2012.05.013
26. Rich C, Geraci M, Griffiths L, Sera F, Dezateux C, Cortina-Borja M. Quality control methods in accelerometer data processing: defining minimum wear time. PLoS One. (2013) 8(6):e67206. doi: 10.1371/journal.pone.0067206
27. Tomlinson OW, Barker AR, Oades PJ, Williams CA. Scaling the oxygen uptake efficiency slope for body size in cystic fibrosis. Med Sci Sports Exercise. (2017) 49(10):1980–6. doi: 10.1249/MSS.0000000000001314
29. Welsman JR, Armstrong N, Kirby BJ, Winsley RJ, Parsons G, Sharpe P. Exercise performance and magnetic resonance imaging-determined thigh muscle volume in children. Eur J Appl Physiol Occup Physiol. (1997) 76(1):92–7. doi: 10.1007/s004210050218
30. Saynor ZL, Barker AR, Oades PJ, Williams CA. Impaired pulmonary VO2 kinetics in cystic fibrosis depend on exercise intensity. Med Sci Sports Exercise. (2016) 48(11):2090–9. doi: 10.1249/MSS.0000000000001004
31. Valdivieso AG, Clauzure M, Marin MC, Taminelli GL, Massip Copiz MM, Sanchez F, et al. The mitochondrial complex I activity is reduced in cells with impaired cystic fibrosis transmembrane conductance regulator (CFTR) function. PLoS One. (2012) 7(11):e48059. doi: 10.1371/journal.pone.0048059
32. Divangahi M, Balghi H, Danialou G, Comtois AS, Demoule A, Ernest S, et al. Lack of CFTR in skeletal muscle predisposes to muscle wasting and diaphragm muscle pump failure in cystic fibrosis mice. PLoS Genet. (2009) 5(7):e1000586. doi: 10.1371/journal.pgen.1000586
33. Wells GD, Wilkes DL, Schneiderman JE, Rayner T, Elmi M, Selvadurai H, et al. Skeletal muscle metabolism in cystic fibrosis and primary ciliary dyskinesia. Pediatr Res. (2011) 69(1):40–5. doi: 10.1203/PDR.0b013e3181fff35f
34. Ruf K, Beer M, Kostler H, Weng AM, Neubauer H, Klein A, et al. Size-adjusted muscle power and muscle metabolism in patients with cystic fibrosis are equal to healthy controls - a case control study. BMC Pulm Med. (2019) 19(1):269. doi: 10.1186/s12890-019-1039-8
35. Decorte N, Gruet M, Camara B, Quetant S, Mely L, Vallier JM, et al. Absence of calf muscle metabolism alterations in active cystic fibrosis adults with mild to moderate lung disease. J Cyst Fibros. (2017) 16(1):98–106. doi: 10.1016/j.jcf.2016.05.010
36. Fajac I, Sermet I. Therapeutic approaches for patients with cystic fibrosis not eligible for current CFTR modulators. Cells. (2021) 10(10):2793. doi: 10.3390/cells10102793
Keywords: exercise capacity, modelling, adolescence, respiratory disease, musculoskeletal
Citation: Tomlinson OW, Barker AR, Fulford J, Wilson P, Shelley J, Oades PJ and Williams CA (2023) Skeletal muscle contributions to reduced fitness in cystic fibrosis youth. Front. Pediatr. 11:1211547. doi: 10.3389/fped.2023.1211547
Received: 24 April 2023; Accepted: 2 June 2023;
Published: 14 June 2023.
Edited by:
Gary James Connett, University Hospital Southampton NHS Foundation Trust, United KingdomReviewed by:
Erik Hulzebos, University Medical Center Utrecht, NetherlandsLarry C. Lands, McGill University, Canada
© 2023 Tomlinson, Barker, Fulford, Wilson, Shelley, Oades and Williams. This is an open-access article distributed under the terms of the Creative Commons Attribution License (CC BY). The use, distribution or reproduction in other forums is permitted, provided the original author(s) and the copyright owner(s) are credited and that the original publication in this journal is cited, in accordance with accepted academic practice. No use, distribution or reproduction is permitted which does not comply with these terms.
*Correspondence: Craig Anthony Williams Qy5BLldpbGxpYW1zQGV4ZXRlci5hYy51aw==
†PW was affiliated with institution #1 at time of work and is now affiliated with Barts Health NHS Trust. JS was affiliated with institution #2 at time of work and is now affiliated with Lancaster Medical School.