- 1Department of Experimental Pathology, Immunology and Microbiology, Faculty of Medicine, American University of Beirut, Beirut, Lebanon
- 2Center for Drug Discovery, American University of Beirut, Beirut, Lebanon
- 3Department of Biological Sciences, Beirut Arab University, Beirut, Lebanon
- 4College of Medicine, Mohammed Bin Rashid University of Medicine and Health Sciences, Dubai, United Arab Emirates
Cutaneous Leishmaniasis (CL) is a neglected tropical disease, classified by the World Health Organization (WHO) as one of the most unrestrained diseases. The Syrian war and the significant displacement of refugees aggravated the spread of this ailment into several neighboring countries in the Eastern Mediterranean Region (EMR). In Syria, Leishmania tropica is identified as one of the most aggressive and endemic identified species, causing localized or generalized lesions, often chronic or relapsing. Pentavalent antimonial drugs are currently used as first line treatment against CL. Nonetheless, these drugs exhibit several limitations, including the repetitive painful injections, high cost, poor availability, and mainly systemic toxicity. Besides, the emergence of acquired parasitic resistance hinders their potency, stressing the need for new therapies to combat CL. Natural products (NPs) epitomize a valuable source in drug discovery. NPs are secondary metabolites (SMs) produced by plants, sponges, or a wide variety of organisms, including environmental microorganisms. The EMR is characterized by its immense biodiversity, yet it remains a relatively untapped area in drug discovery. NPs of the region were explored over the last 2 decades, but their discoveries lack biogeographical diversity and are limited to the Red Sea. Here, we isolated previously uncultured environmental soil-dwelling Streptomyces sp. HAS1, from Hasbaya region in southeast Lebanon. When fermented in one of our production media named INA, HAS1 produced a crude extract with significant potency against a clinical Leishmania tropica isolate. Using bio-guided fractionation, the bioactive compound was purified and the structure was elucidated by NMR and LC-HRMS. Our findings establish NPs as strong candidates for treating Leishmania tropica and further dwells on the importance of these natural sources to combat microbial infections.
Introduction
Cutaneous Leishmaniasis (CL) is a parasitic disease caused by the protozoan Leishmania species. CL is classified by the World Health Organization (WHO) as one of the most significant neglected tropical diseases (Cavalli and Bolognesi, 2009), affecting around 12 to 15 million people worldwide, and 600,000 to one million new cases occurring annually (Reithinger, 2016; Torres-Guerrero et al., 2017). Particularly, the last decade witnessed an alarming increase in CL incidence (Bailey et al., 2017). CL is endemic in various areas including the Americas, the Mediterranean basin, the Middle East and Central Asia. In the Eastern Mediterranean (EMR) region, Syria partakes the highest prevalence of CL (Mcdowell et al., 2011). Moreover, the Syrian crisis led to increased frightening numbers of CL (Al-Salem et al., 2016), across Syria itself, and its neighboring countries following the displacement of refugees who fled the war (Saroufim et al., 2014; Sharara and Kanj, 2014; Hayani et al., 2015; Burza et al., 2018; Rehman et al., 2018; Bizri et al., 2021). Clinically, CL is characterized by localized or generalized lesions, often chronic or relapsing, on exposed parts of the body (Sharma et al., 2005; Steverding, 2017; Burza et al., 2018). The severity of these clinical manifestations is variable and depends on the causative species among other factors (Torres-Guerrero et al., 2017). The most prominent etiologic agents of CL in the Middle East region are Leishmania tropica (L. tropica) and Leishmania major (Roberts, 2005; Masmoudi et al., 2013; Fakhar et al., 2016), with a predominance of L. tropica among Syrian refugees (Saroufim et al., 2014; Bizri et al., 2021). Various therapeutic approaches exist for CL, with multiple variables affecting the type of applied treatment (Heras-Mosteiro et al., 2017). In addition to physical approaches, remedies can be administered topically, orally, or systemically (Palumbo, 2009; Eiras et al., 2015; Heras-Mosteiro et al., 2017; Briones Nieva et al., 2021). Pentavalent antimonials are the first line of defense against CL, with an extensive use of Meglumine antimoniate (Glucantime) (Esfandiarpour and Alavi, 2002; Palumbo, 2009; Eiras et al., 2015; Briones Nieva et al., 2021). These drugs can be injected intra-lesionally, intramuscularly, or intravenously (Palumbo, 2009; Eiras et al., 2015; Briones Nieva et al., 2021). Nonetheless, they exhibit numerous adverse effects such as their painful repetitive injections and systemic toxicity (Torres-Guerrero et al., 2017; Garza-Tovar et al., 2020). Moreover, several reports documented the emergence of resistant parasites to these therapies (Kazemi-Rad et al., 2013a; Kazemi-Rad et al., 2013b; Mohebali et al., 2019; Ozbilgin et al., 2020). Among the emerging treatments of CL, the potency of an immunomodulatory drug, Imiquimod (Arevalo et al., 2001; Firooz et al., 2006; Arevalo et al., 2007; Miranda-Verastegui et al., 2009) and its analog 1-(3-methoxyphenyl)-Nmethylimidazo [1,2-a]quinoxalin-4-amine (EAPB0503) (El Hajj et al., 2018) was reported. Yet, the use of Imiquimod as first-line therapy and the clinical use of its derivative against CL are still out of reach. Thus, the discovery of new alternative drugs capable of efficiently eliminating CL while inducing minimal side effects is fundamental.
Natural products (NPs), also known as secondary metabolites (SMs), originate from a plethora of organisms including environmental microorganisms and remain an important source in the field of anti-infective drug discovery (Pink et al., 2005; Chin et al., 2006). Indeed, approximately 50% of all antimicrobials discovered over the last 4 decades were NPs, their derivatives, or their mimics (Newman and Cragg, 2020). Among NPs, different examples are reported with anti-leishmanial potency (Gervazoni et al., 2020; Carter et al., 2021). Yet, these NPs were produced from a small pool of bacteria, limiting the opportunity to find novel molecules with specific targets and selective toxicity against Leishmania species, particularly L. tropica (Zhu et al., 2011; Zhu et al., 2012). Thus, the quest for these molecules against this endemic parasite in the EMR, requires the search for uncultured bacteria. Such microbes can typically be found in untapped geographical locations whose full potential was not yet unraveled (Cordell, 2000; Tulp and Bohlin, 2002; Lefevre et al., 2008). The EMR is a great example of unexploited biodiverse area, where the antimicrobial drug discovery was almost exclusively made at the level of the Red Sea. In this study, we purified NPs synthesized by uncultured environmental bacteria inhabiting the soil of Lebanon, and explored their anti-leishmanial activity against a clinical L. tropica isolate from a CL patient.
Materials and methods
Soil sample collection and bacterial isolation
Soil samples were collected from Hasbaya (HAS), Koura (KR) and Bchamoun (BCH) specific regions in Lebanon. Each of the isolates was named after the region it originated from and the order of its isolation. For example, HAS1 is the first bacterium isolated from the soil of the Hasbaya region, while BCH 8 and KR 24 correspond to 8th and 24th bacterial isolates from the Bchamoun and Koura regions respectively. Soil samples were collected in a sterile container and transported to the laboratory for further processing. The soil was first dried for 7 days at 37°C. Afterward, 3 g of the dried sample were dissolved in 100 ml of autoclaved distilled water and incubated at 55°C for 30 min. Subsequently, serial dilutions (1/5, 1/10, 1/100, and 1/1,000) of the soil suspension were performed in a final volume of 1 ml. These dilutions, along with the original soil solution, were inoculated on soil agar (30 g dried soil, 18 g bacteriological agar, and 10 g corn starch in 1 L distilled water) or on International Streptomyces Project-3 (ISP3) agar (20 g oats, 18 g bacteriological agar, and 2.5 ml ISP3 trace elements in 1 L distilled water, pH = 7.8). The plates were then incubated at 28°C for 14 days. Once colonies started to appear, they were purified via continuous subculturing on ISP3 agar. When pure cultures were obtained, bacteria were stored at -80°C in a 50% aqueous glycerol solution.
Secondary metabolite production and extraction
A starter culture, known as the first seed, was initiated by inoculating 35 μl of the bacterial stock in 5 ml of liquid ISP3 (20 g oats and 2.5 ml ISP3 trace elements in 1 L distilled water). This suspension was then incubated at 28°C at 130 rpm for 48 h, before 1 ml was transferred into 10 ml of sterile ISP3 media. This second seed was subjected to identical incubation conditions, after which, 1 ml was used to inoculate 50 ml of each of our 14 different media to induce SM production (Table 1). The cultures were then incubated under the same conditions for 7 days. Then, 1 ml of Amberlite XAD 16N resins was added to each culture and left for 3–4 h to adsorb the SMs. The resin and cell pellets were centrifuged and consequently extracted with methanol. The methanol solution was then defatted with hexane and the layer was evaporated to dryness. The crude was resuspended with ethyl acetate and extracted with 1M HCl. The organic layer was then evaporated to dryness and resuspended in DMSO for further purification by HPLC (Figure 1A). A Phenomenex Luna® 5 μm C18 column 100 Å 250 × 10 mm was used and a gradient from 5 to 95% B in 40 min with (A) H2O+ 0.1% FA and (B) ACN +0.1% FA at a flow rate of 5 ml/min at room temperature. Elution was monitored at 220 and 280 nm. Accordingly, the crude extracts stocks were named HAS1 INA, C and M8 (corresponding to the crude produced by the bacterium HAS1 in medium INA, C and M8 respectively), BCH 8 NL2 (corresponding to the crude produced by the bacterium BCH8 in medium NL2), and KR24 V6 (corresponding to the crude produced by the bacterium KR24 in medium V6).
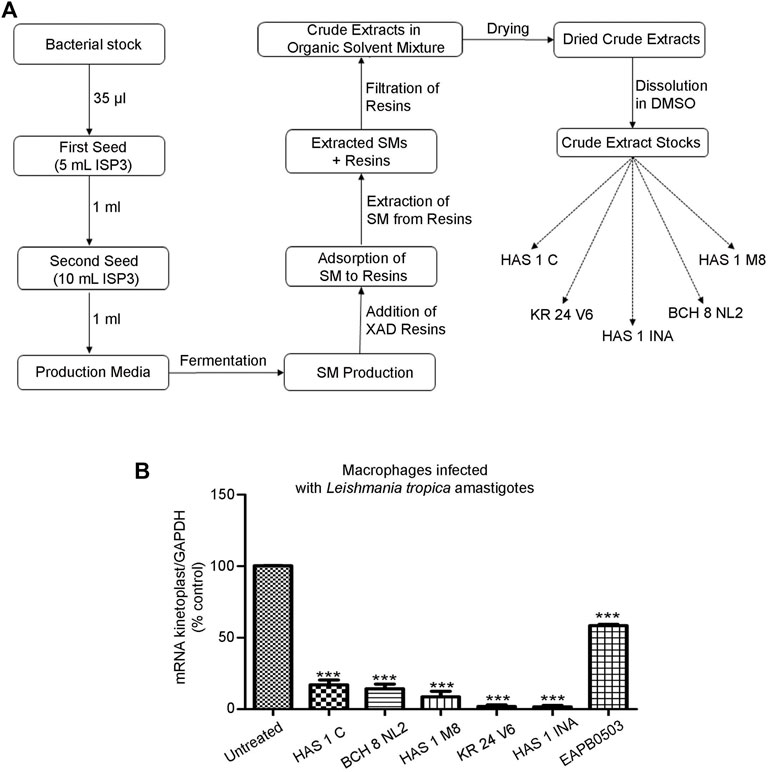
FIGURE 1. Crude extracts produced by environmental bacteria derived from Lebanese soil inhibit L. tropica amastigotes replication. (A). Pipeline of secondary metabolite production by environmental bacteria isolated from soils collected from selected region across Lebanon. Bacteria from Hasbaya (HAS), Koura (KR) and Bchamoun (BCH) were fermented in a collection of 14 different production media. XAD resins were added 14 days post bacterial inoculation to adsorb the SMs, which were then extracted via organic solvents. The overall SMs collected from an isolate in a specific medium were named after the isolate followed by the medium name, for example HAS1 INA. (B). Real-time quantitative PCR detection of THP-1 derived macrophages infected with patient-derived L. tropica amastigotes treated with 0.01 μg/ml of crude extracts HAS1 C, BCH8 NL2, HAS1 M8, KR24 V6, and HAS1 INA for 24 h. Briefly, THP-1 monocytes were differentiated into macrophages, activated with LPS, and infected with L. tropica with a ratio of one parasite/three cells. Treatment with 0.01 μg/ml of the crude extracts or 1 μM of EABP0503 was then performed for 24 h. The results are shown as percentage of untreated infected macrophages. Amastigote transcripts were evaluated by Syber green RT-PCR using kinetoplast specific primers and their percentage of expression was normalized to GAPDH. Results are expressed as percentage of the untreated control (±) SEM and are representative of at least three independent experiments. To validate statistical significance, t-tests were used with *** representing p-values < 0.001.
HAS1 isolate characterization
Since extracts of HAS1 isolate exhibited the highest potency against L. tropica, HAS1 INA was chosen for further purification. HAS1 was subjected to genomic DNA extraction using the QIAamp DNA Mini Kit (Qiagen). The resulting genomic DNA was used as a template for the preparation of whole-genome sequencing libraries through the Nextera XT library prep kit (Illumina GmbH). The libraries were then sequenced on the Illumina MiniSeq sequencer, 2 × 150 bp. Trimmomatic (Bolger et al., 2014) was used for quality control and trimming of the reads, while assembly was achieved through Unicycler (Wick et al., 2017) on Galaxy. The resulting FASTA file was fed into KmerFinder 3.2 to determine the bacterial species (Larsen et al., 2014). Based on the sequencing results, HAS1 was characterized according to the taxonomic description of the Actinobacteria as follows: first, to visualize the morphology and color of the mycelium, HAS1 was streaked on the ISP3 agar. Second, the isolate was tested for its resistance to sodium chloride (NaCl) through its culture on agar 5,339 (10 g of casein peptone, 5 g of yeast extract, and 20 g of agar in 1 L of distilled water, pH = 7) containing various percentages of NaCl. Third, the isolate’s pH tolerance was investigated by culturing it on ISP2 agar (10 g of malt extract, 4 g of yeast extract, 4 g of glucose, and 15 g of agar in 1 L of distilled water) with different pH levels. For all three tests, the plates were then incubated at 28°C for 10 days before the observation of the growth which was recorded and documented. The biochemical fingerprints of HAS1 were evaluated using the API 20E kit (BioMérieux), according to the manufacturer’s recommendations.
High performance liquid chromatography mass spectrometry (HPLC-MS)
Measurements were performed using an AB Sciex X500R QTOF ESI mass spectrometer. LC flow was split to 500 nL/min before entering the ion source. Mass spectra were acquired in centroid mode ranging from 150 to 1,000 m/z, resolution R = 30,000. A Luna Omega C18, 150 × 2.1 mm, 1.6 µm column was used, injection volume = 1 µL. A gradient of A) H2O+ 0.1% FA and B) MeCN +0.1% FA at a flow rate of 0.55 ml/min was used to achieve separation. Gradient conditions start at 5% B, increase to 10% B in 1 min, then to 35% B from minute 1→15, then to 50% B from minute 15→22, and finally to 80% B from minute 22→25. After a 1-min hold at 80% B, the system was re-equilibrated for 5 min with the initial conditions. UV data was acquired using a PDA (wavelength 200–800 nm ± 8 nm), MS detection was performed simultaneously.
Leishmania tropica promastigotes culture
L. tropica promastigotes were isolated from biopsies of CL patients as described (El Hajj et al., 2018). Sample collection was approved by the Institutional Review Board of the American University of Beirut (PALK.IK.01) (El Hajj et al., 2018). Parasites were maintained in RPMI-1640 (Lonza) supplemented with 10% Fetal Bovine Serum (FBS), 100IU/ml streptomycin/penicillin (Sigma).
Macrophage culture, infection, and treatment
Human monocytic THP-1 cells (American Type Culture Collection ATCC TIB-202) were seeded at a density of one million cells/well in six well-plates, and grown in RPMI medium supplemented with 10% Fetal Bovine Serum, 1% penicillin-streptomycin, and 1% glutamine. The differentiation of monocytes into macrophages was performed as described (El Hajj et al., 2018). Briefly, the differentiation was induced using 50 ng/ml of phorbol 12-myristate 13-acetate (PMA, Sigma) overnight. The adherent differentiated macrophages were then activated using 1 μg/ml of lipopolysaccharide (LPS) for 4 h. The activated macrophages were subsequently infected with the patient-derived L. tropica promastigotes at the ratio of one parasite/3 macrophages. After a 24 h incubation at 37°C, non-internalized parasites were washed out with phosphate buffer saline (PBS). Subsequently, the media was replenished, and a final concentration of 0.01 μg/ml of the crude extracts or the fractions (HAS1 INA Hexane, HAS1 INA chloroform, HAS1 INA Ethyl Acetate, and HAS1 INA Water) or 1 μg/ml of the purified compounds (HAS1 F1, HAS1 F2, HAS1 F3, and HAS1 F4) were added and incubated for 24 h at 37°C. EAPB0503 was used as positive control (El Hajj et al., 2018). The drug was prepared as a 0.1M stock in DMSO and stored at -80°C. Working solutions of 1 μM were freshly prepared in culture media.
Anti-amastigote activity
After 24 h of incubation with the respective treatments, infected macrophages with amastigotes were washed with PBS, scraped, and collected in microcentrifuge tubes. Tubes were centrifuged at 1,200 rpm for 5 min at 4°C. The supernatant was discarded. The anti-amastigote activity was assessed by quantitative real time PCR and by Immunofluorescence assay.
Hematoxylin and eosin (H&E) stain
Activated macrophages seeded onto coverslips in six well-plates were subjected to H&E staining. Following infection with L. tropica promastigotes at the ratio of one parasite/3 macrophages, and treatment for 24 h with either HAS1-F1 or HAS1-F2, cells were fixed with methanol for 1 min. The coverslips were then covered in Hematoxylin (Fisher Scientific, Canada) and a counterstaining was performed for 30 s before rinsing for 5 min with water. Subsequently, the coverslips were dipped in an alcoholic eosin Y solution (Leica Microsystems, Canada), and rinsed with deionized water before mounting on slides.
Anti-promastigote activity
To investigate the anti-promastigote activity of the crude extract HAS1 INA and its bioactive fractions (HAS1-F1 and HAS1-F2), a blind counting assay was performed. First, 100 μL per well of L. tropica promastigote culture (106 cells/mL) were added in round-bottom 96-well plates. Then, 100 μL of various dilutions of the treatments were added to each well, to obtain final concentrations between 0.01 and 1 μg/ml for HAS1 INA and between 0.5 and 10 μg/ml for HAS1-F1 and HAS1-F2. The plates were then incubated at 25°C for 24 h, then transferred to 37°C for 20 min to reduce parasite motility. The motile promastigotes were then counted using a hemocytometer.
Quantitative real-time PCR
Total RNA was extracted using the RNeasy Plus Mini Kit (Qiagen). cDNA synthesis was performed using 1 μg of the RNA of each sample as a template, using the iScript cDNA Synthesis Kit (BIO-RAD). Syber green qRT PCR was then completed using the BIORAD-CFX96 machine as described (El Hajj et al., 2018). Primers for amastigote detection target the minicircle kinetoplast DNA (kDNA) (Table 2). Primers for the housekeeping Glyceraldehyde-3-Phosphate dehydrogenase GAPDH, are also listed in Table 2. The PCR cycles included a denaturation step for 3 min at 95°C, followed by 39 cycles of (denaturation for 15 s at 95°C, annealing for 1 min at 57°C, elongation for 30 s at 72°C), and a final elongation for 5 min at 72°C. Percentage of expression was calculated according to the Livak method (Schmittgen and Livak, 2008).
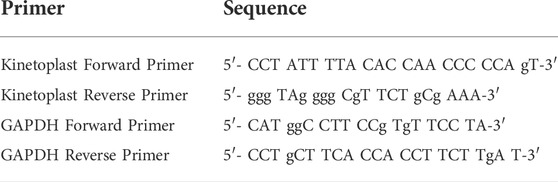
TABLE 2. Sequence of primers used in the q-RT-PCR for detection of kinetoplast and the housekeeping gene GAPDH.
Immunofluorescence microscopy
P6 well plates were seeded with activated macrophages infected with L. tropica (1 parasite per three cells) for 24 h and treated with HAS1 F1 or HAS1 F2 for 24 h. After 24 h, coverslips were fixed in 4% paraformaldehyde for 20 min. Permeabilization was performed in Triton (0.2%) for 10 min. Following one PBS wash, a blocking step for 30 min with PBS-10% FBS was performed. A conjugated FITC anti-Leishmania Major Surface Protease (Gp63) monoclonal antibody (CEDARLANE CLP005F) was used at the dilution of 1:1000. Staining of nuclei was performed using 1 μg/ml of Hoechst 33,342 in trihydrochloride trihydrate solution (Invitrogen, H33342) for 5 min. Finally, coverslips were mounted onto slides using a Prolong Anti-fade kit (Invitrogen, P36930). Images were acquired by the Zeiss Axio microscope (Zeiss, Germany) and analyzed using ZEN software.
Cytotoxicity on PBMCs
To assess the cytotoxicity of HAS1 INA, Has1-F1, and HAS1-F2, trypan blue exclusion assay was performed on human Peripheral Blood Mononuclear Cells (PBMCs). PBMCs were isolated using a Ficoll gradient from left-over peripheral blood specimens collected from the American University of Beirut Medical Center. PBMCs were seeded in 96-cell plates at the density of 200,000 cells/mL in RPMI media supplemented with 20% FBS and 1% penicillin-streptomycin. The cells were then incubated with different concentrations of HAS-F1 or HAS1-F2 (1, 5, or 10 μg/ml) or with 0.01 μg/ml of HAS1 INA for 24 h, and the number of living cells was counted using a hemocytometer.
Statistical analysis
Graphs were plotted and analyzed using GraphPad Prism. The statistical significance was validated for each treatment using an unpaired t-test with the non-treated control. p-values < 0.05 were considered as significant. *, **, *** were used for p-value < 0.05, <0.01 and <0.001 respectively.
Results
Crude extracts from soil isolated bacteria of different Lebanese areas exhibit a potency against a clinical Leishmania tropica isolate
The EMR is an unexploited biodiverse area when pertaining to antimicrobial drug discovery. We first collected soil samples from specific regions in Lebanon: Hasbaya (HAS), Koura (KR), and Bchamoun (BCH). The isolation of crude extracts from different soils is described in Figure 1A. The production of secondary metabolites does not only depend on the growth of bacteria, but also on the conditions imposed by the medium and the surroundings. For instance, the carbon source plays an important role for the precursors and energy required for the synthesis of biomass building blocks and secondary metabolites production (Torres-Bacete et al., 2005). Thus, designing an appropriate production medium is an important step in the production of secondary metabolites. The crude extract of isolates from our collection of environmental bacteria from different soil samples were cultured in 14 different production media (Table 1), and tested for their antileishmanial activity, against a Leishmania tropica clinical isolate. The antileishmanial effect was assessed by quantifying the expression of Leishmania-kinetoplast reflecting the multiplication of amastigotes within macrophages infected with L. tropica (El Hajj et al., 2018). Among all tested crude extracts in different production media, five extracts from three soil sources and different production media were able to significantly reduce the kinetoplast transcript levels at a concentration as low as 0.01 μg/ml (Figure 1B), and exhibited a significantly higher potency than EAPB0503, used as positive control (El Hajj et al., 2018) (Figure 1B). While HAS1 C and BCH8 NL2 and HAS1 M8 significantly reduced the multiplication of L. tropica amastigotes to less than 20%, KR24 V6 and HAS1 INA almost cleared the parasites (Figure 1B). Notably, HAS1 INA showed the most prominent inhibition of amastigote multiplication with a decline in kinetoplast expression surpassing 98%, when compared to the untreated control (p-value < 0.0001) (Figure 1B). Hence the remainder of the present study was based on the bio-guided fractionation of this crude extract to identify novel antileishmanial compounds.
HAS1 producing strain characterization
Using KmerFinder 3.2, HAS1 was most significantly identified as Streptomyces sp. CB00271 (q-value = 67,548.74; p-value = 1.0e-26). Thus, HAS1 was characterized by standard tests for Streptomyces. On ISP3 agar (Figure 2A), HAS1 presented a substrate mycelium with a sand yellow color (RAL 1002) and an aerial mycelium with an oyster white color (RAL 1013). This bacterium formed spores as well. HAS1 was also able to grow normally on the agar 5,339 when the NaCl content was lower or equal to 5%. Whereas minimal growth was observed on the same agar when it contained 7.5% NaCl and no growth was observed at a NaCl percentage of 10 (Figure 2B).
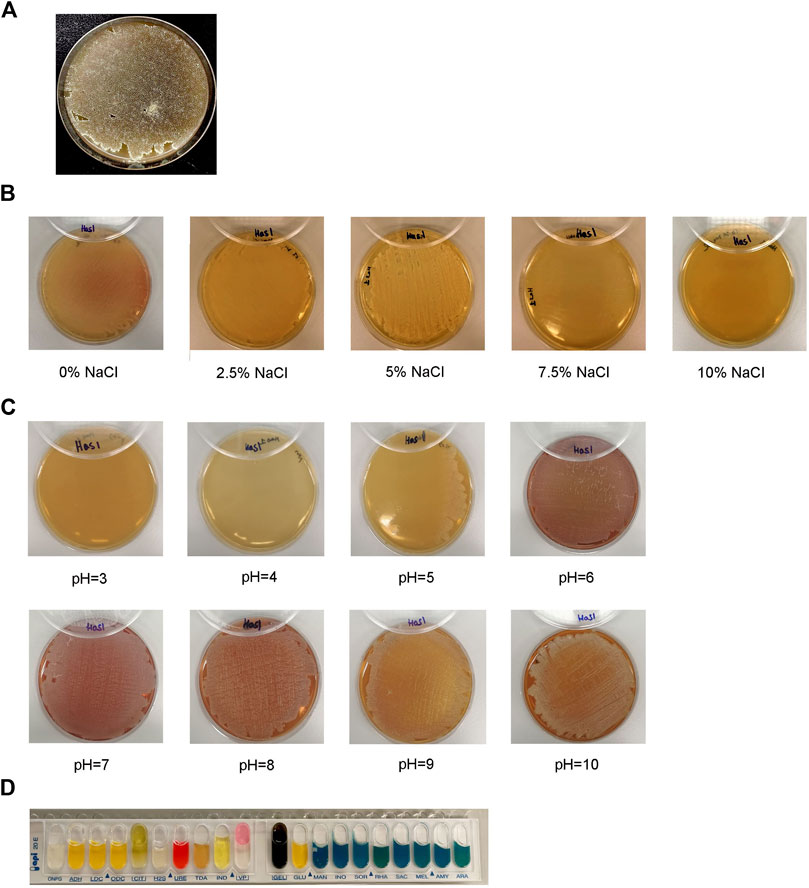
FIGURE 2. Morphological, physiological, and biochemical characterization of the bacterial isolate HAS1. (A). Growth, shape, color of the mycelium of the isolate HAS1 and spore formation on ISP3 agar 10 days after bacterial inoculation with an incubation at 28°C. (B). Physiological characterization of HAS1 isolate using bacterial growth on the 5,339 agar with different NaCl concentrations (0, 2.5, 5, 7.5 and 10%) after incubation for 10 days at 28°C. (C). Growth of HAS1 on ISP2 agar with different pH (3, 4, 5, 6, 7, 8, 9, and 10) after a 10 days at 28°C. (D). Biochemical testing of HAS1 using the API 20E kit.
On ISP2 agar, HAS1 showed no growth at pH three or 4, while it grew on the same type of agar when the pH was superior or equal to 5 (Figure 2C). Finally, using the API 20E kit, HAS1 was able to ferment glucose (GLU-positive) and produce acetoin (VP-positive), and proved to possess both the urease (URE-positive) and gelatinase (GEL-positive) enzymes (Figure 2D).
HAS1-F1 and HAS1-F2 fractions of HAS1 INA are the bioactive compounds exhibiting the antileishmanial activity against L. tropica isolate
The prominent activity of HAS1 INA crude extract prompted us to isolate the bioactive compounds from this mixture. An upscaled fermentation was produced and the SMs were first segregated via liquid-liquid partition (Figure 3A). Among the four obtained fractions in Hexane, chloroform, Ethyl Acetate and water, the HAS1 chloroform fraction revealed the most prominent decrease in amastigote multiplication (>98%), at a concentration of 0.01 μg/mL as compared to the untreated control (Figure 3B). Therefore, this fraction was considered for further purification. After HPLC, HAS1 INA chloroform generated four distinct fractions named HAS1-F1, HAS1-F2, HAS1-F3, and HAS1-F4 (Figure 3C). Upon testing their biological activity against L. tropica amastigotes, all HAS1 fractions did not result in significant anti-leishmanial activity at the concentration of 0.01 μg/ml. Importantly, at the concentration of 1 μg/ml, HAS1-F1 and HAS1-F2 showed a significant decrease in kinetoplast transcript expression to 47% (p-value = 0.0170) and 38% (p-value = 0.0029) respectively, as compared to the untreated control (Figure 3C). Importantly, the potency of both fractions was higher than that of EAPB0503, used as positive control (El Hajj et al., 2018) (Figure 3C). The infection of macrophages and the effect HAS1-F1 and HAS1-F2 fractions were then verified by H&E stain (Figure 3D), and quantified using the Leishmania Glycoprotein Gp63. Indeed, treatment with 1 μg/ml of HAS1-F1 or HAS1-F2 significantly decreased the percentage of amastigotes, as compared to untreated or DMSO treated controls (Figure 3E). In more details, HAS1-F1 lessened the percentage of intracellular parasites to 62% (p-value = 0.0122), while HAS1-F2 reduced the percentage of internalized amastigotes to 50% (p-value = 0.0012). As for vehicle control, DMSO did not affect the replication of the intramacrophage amastigotes (Figures 3C,E). Importantly, no cytotoxic effect of either fractions on human peripheral blood mononuclear cells was observed, even at concentrations reaching 5 and 10 μg/ml (Supplementary Figure S1).
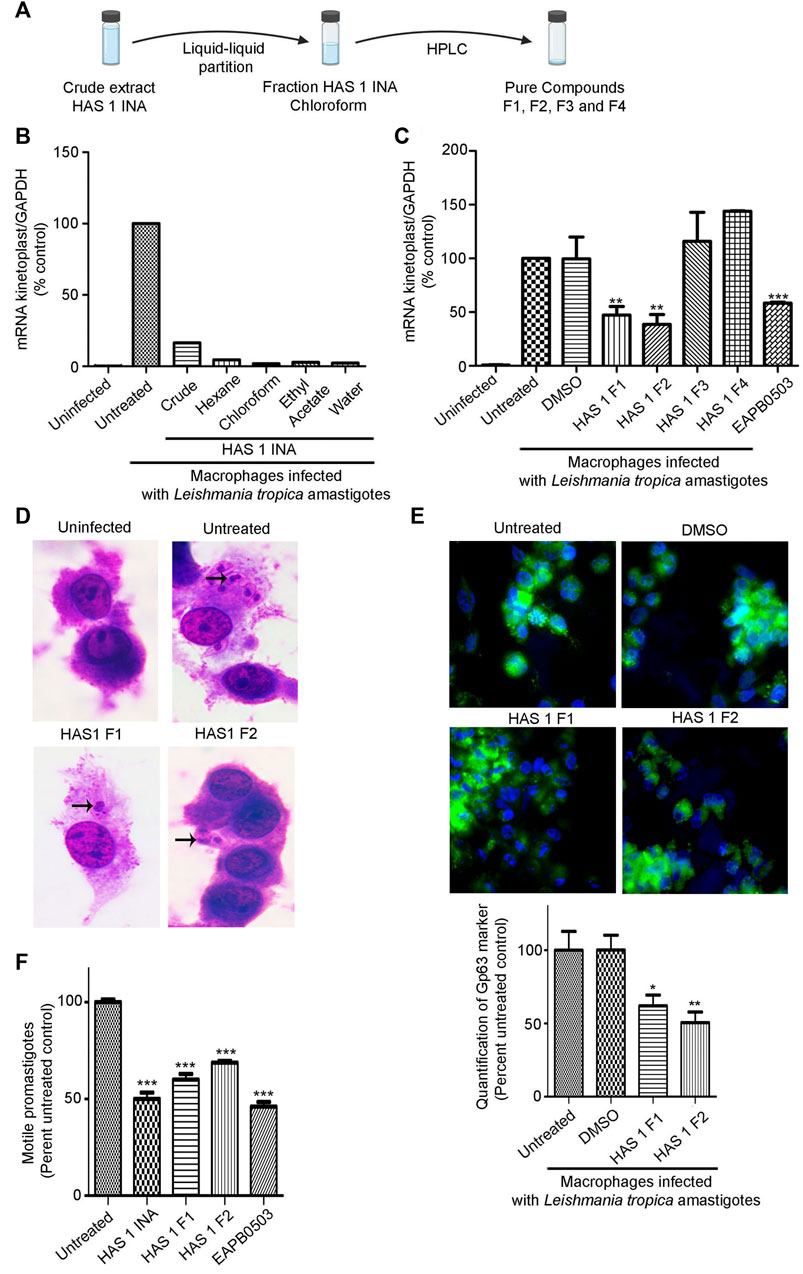
FIGURE 3. Bio-guided fractionation of the antileishmanial compounds of HAS1 INA. (A). An upscaled fermentation of the HAS1 INA crude was first subjected to liquid-liquid partition generating four fractions: HAS1 INA Hexane, HAS1 INA chloroform, HAS1 INA Ethyl Acetate, and HAS1 INA Water. HAS1 INA chloroform was further fractionated into four pure compounds using HPLC: HAS1-F1, HAS1-F2, HAS-F3, and HAS1-F4. (B,C). Real-time quantitative PCR detection of THP-1 derived macrophages infected with patient-derived L. tropica amastigotes treated with 0.01 μg/ml of HAS1 INA fractions and the crude extract (B) or with 1 μg/ml of the pure compounds for 24 h or with 1 μM of EABP0503 for 24 h (C). Amastigote transcripts were evaluated by Syber green RT-PCR using kinetoplast specific primers and their percentage of expression was normalized to GAPDH. Results are expressed as percentage of the untreated control (±) SEM and are representative of at least three independent experiments (C) and one representative experiment for (B). To validate statistical significance, t-tests were used with ** representing p-values < 0.01, and *** representing p-values < 0.001. (D). H&E staining of THP-1 derived macrophages infected with patient-derived L. tropica amastigotes treated with 1 μg/ml of HAS1-F1 or HAS1-F2 for 24 h. (E). Immunofluorescence microscopy of THP-1 derived macrophages infected with patient-derived L. tropica amastigotes treated with 1 μg/ml of HAS1-F1 or HAS-F2. The Gp63 surface parasite was stained with an anti-Gp63 antibody (green), and nuclei were stained with Hoechst 33,342 (blue). The graph shows the quantification of GP63 as averages from 50 different cells which were then expressed as percentage of the untreated control (±) SEM. To validate statistical significance, t-tests were used with * representing p-values < 0.05, and ** representing p-values < 0.01. (F). Blind count of motile promastigotes of L. tropica treated with 0.01 μg/ml of HAS1 INA, 1 μg/ml of HAS1-F1 or HAS1-F2, or 1 μM of EABP0503 for 24 h. Results are expressed as percentage of the untreated control (±) SEM and are representative of at least three independent experiments. To validate statistical significance, t-tests were used with *** representing p-values < 0.001.
We then assessed the potency of HAS1 INA and its bioactive fractions on the promastigote stages of the used L. tropica isolate. Interestingly, treatment of promastigotes with HAS1 INA, HAS1-F1, or HAS1-F2 decreased the motile promastigotes count (Figure 3F). Indeed, HAS one INA at the concentration of 0.01 μg/ml exhibited the same potency as EAPB0503 (positive control) and decreased the motile promastigote count to around 50% (p-value <0.0001). HAS1-F1 or HAS1-F2, used at the concentration of 1 μg/ml, also exhibited a significant effect on L. tropica motile promastigote count, but were less potent than the crude extract or the positive control, and led to decrease of 40% (p-value <0.0001) and 32% (p-value <0.0001) respectively (Figure 3F). Altogether, our results demonstrate the anti-leishmanial activity of HAS1-F1 and HAS1-F2 bioactive fractions on both the promastigote and amastigotes stages of the tested clinical Leishmania tropica isolate.
Structure elucidation of HAS-1 bioactive fraction F1
To elucidate the structure of the two active fractions, HAS1-F1 and HAS1-F2 were run on HPLC-MS (high resolution) in ESI positive mode. HAS1-F1 gave a peak with m/z of 449.3585 for [M + Na] and 427.3779 for [M + H]. The molecular formula generated from these peaks was C26H50O4 with two double bonds. As for HAS1-F2, the peak had an m/z of 393.3443 for [M + H] and a molecular formula of C25H45O3 with four double bonds. While investigating the HPLC-MS of the crude extracts and the various fractions, HAS1-F2 was revealed as a shunt product from biosynthesis, that disappeared overtime when the fermentation was left longer. Hence the possible structure of HAS1-F1 was solely investigated using a Bruker 500 MHz Ascend HD NMR. The elucidation revealed an α-angelica lactone ring and an alcohol on the beta position of the ring (Figure 4). The remaining portion is a fatty acid (Figure 4). This is characteristic to acetogenines that have previously been reported from Streptomyces sp. (Taechowisan et al., 2016).
Discussion
Cutaneous Leishmaniasis remains one of the most important neglected tropical diseases worldwide (Cavalli and Bolognesi, 2009) with an alarming increase in CL incidence reported over the past decade (Bailey et al., 2017). In the EMR, Syria is characterized by the highest prevalence of CL (Mcdowell et al., 2011). The economic deterioration and the war in this country led to a huge displacement of refugees from endemic areas, leading to alarming increases of CL in the neighboring countries including Lebanon (Saroufim et al., 2014; Sharara and Kanj, 2014; Hayani et al., 2015; Al-Salem et al., 2016; Burza et al., 2018; Rehman et al., 2018; Bizri et al., 2021). L. tropica is among the most prevalent etiologic agents of CL among Syrian refugees (Saroufim et al., 2014; Bizri et al., 2021).
The treatment of CL still heavily relies on drugs exhibiting a plethora of adverse effects. In that sense, a staple among first-line CL therapies is the pentavalent antimonial Glucantime, which requires excessive repeated parenteral injections inducing localized painful sensation and systemic toxicity (Frezard et al., 2009). Moreover, resistance to Glucantime is emerging, reducing its efficiency in combating CL (Guerin et al., 2002). Second-line drugs against CL such as the antifungal Amphotericin B and the antiprotozoal Pentamidine still pose issues when utilized clinically as they also generate systemic toxicity (Oliveira et al., 2011). Although Miltefosine was recognized as a good candidate for the bedside treatment of CL, evidence from preclinical and clinical studies pointed to the limitations of its use (Monge-Maillo and Lopez-Velez, 2015).
Natural Products were historically, and remain an invaluable source for drug discovery (Newman and Cragg, 2020). This interest in exploiting NPs to create new drug scaffolds has extended to include neglected tropical diseases. Accordingly, leishmaniasis was the target of many studies investigating the inhibitory effects of NPs on its causative species. The majority of NPs with antileishmanial activity originate from plants, namely those which are a part of traditional or folk medicine. Numerous plant-derived crude extracts and fractions revealed their ability to inhibit the replication of Leishmania sp. Promastigotes and amastigotes. Using bio-guided fractionation, a variety of structurally diverse antileishmanial pure compounds from NPs were isolated (Rocha et al., 2005; Gervazoni et al., 2020; Carter et al., 2021). In particular, the flavonol Quercetin and the flavonoid Rutin (Mehwish et al., 2019), the sesquiterpene (-)-α-Bisabolol (Corpas-Lopez et al., 2016), Epigallo-catechin 3-O-Gallate (Saduqi et al., 2019) inhibited the proliferation of both promastigotes and amastigotes of L. tropica. Furthermore, all these NPs demonstrated lower cytotoxicity levels when compared to the pentavalent antimonial control drug both in vitro and in vivo. Therefore, NPs offer a vital pool of structurally variable molecules that can compete in both effectiveness and safety with the clinically utilized anti-leishmanials.
The anti-infective efficacy of NPs depended historically on a genus of environmental bacteria known as Streptomyces (Antoraz et al., 2015; Quinn et al., 2020). The exploitation of Streptomyces sp. in the discovery of novel anti-parasitic agents is typically less common. Nevertheless, these bacteria yielded compounds with important medical efficacy. Ivermectin, a macrocyclic lactone from Streptomyces avermitilis is widely used to treat helminth infections (Chabala et al., 1980). The aminoglycoside Paromomycin from Streptomyces rimosus var. Paromomycinus, is prescribed for several parasitic infections including leishmaniasis (Wiwanitkit, 2012). We evaluated the anti-leishmanial potential of crude extracts produced by environmental Streptomyces, isolated from the understudied niche of the Lebanese soil. The crude extracts produced by Strepomyces species from Hasbaya region were the most potent in inhibiting intra-macrophagic L. tropica amastigotes in vitro. At a concentration as low as 0.01 μg/ml, the crude HAS1 INA caused an almost complete inhibition of the replication of the intracellular amastigotes of an L. tropia clinical isolate. This immense activity has not been previously recorded for plants derived extracts tested as antileishmanial compounds against patient-derived internalized L. tropica amastigotes. In that sense, the methanolic extract of the leaves of Myrtus communis inhibited amastigote replication with an IC50 of 40.8 μg/ml (Mahmoudvand et al., 2015), while the alcoholic extract of Pistacia khinjuk fruits decreased the growth rate of intramacrophage amastigotes with an IC50 of 37.3 μg/ml (Ezatpour et al., 2015).
Following bio-assayed fractionation, two novel compounds HAS1-F1 and HAS1-F2 inhibited the replication of these parasites. Our results follow a recent trend that revolves around screening NPs from Streptomyces for activity against Leishmania species. Indeed, an extract produced by Streptomyces sp. VITBVK2, isolated from a soil sample of a salt pan effectively killed intramacrophage L. donovani amastigotes at a dose of 100 μg/ml, and yielded same activity than Miltefosine (Sreedharan and Bhaskara Rao, 2017). Our crude extracts and their bioactive fractions exhibited anti-leishmanial potency 100 to 1,000 folds lower than the extract of VITBVK2. Under the same line, Streptomyces sp. isolated from Mediterranean sponges produced the cyclodepsipeptide Valinomycin and the indolocarbazole alkaloid Staurosporine, whose antileishmanial activities were unraveled for the first time. Both compounds inhibited the proliferation of L. major promastigotes with IC50 values less than 0.11 and 5.3 μM respectively (Pimentel-Elardo et al., 2010). HAS1-F1 and HAS1-F2 inhibited the replication of L. tropica amastigotes at doses of 2.3 and 2.5 μM, which are of similar order to that of Staurosporine. Beyond CL, compounds secreted by ants-associated Streptomyces sp. inhibited L. donovani, the causative agent of another spectrum of leishmaniasis, the fatal visceral leishmaniasis. Indeed, Ortega et al. isolated four known molecules (Mer-A2026B, piericidin-A1, Dinactin, and Nigericin) from three different Streptomyces sp. originating from attine ants and reported their antileishmanial activity against L. donovani promastigotes (Ortega et al., 2021). Importantly, Dinactin and Nigericin showed superior action than Miltefosine, and were extremely effective in the eradication of the intramacrophage L. donovani amastigotes with IC50 values of 0.018 and 0.129 μM respectively. Although these compounds are of higher order of activity than HAS1-F1 and HAS1-F2, their cytotoxicity against THP-1 macrophages was extremely elevated (Ortega et al., 2019). The same research group further isolated from the ant-associated Streptomyces sp. ISID311, three structurally related macrolides, Cyphomycin, Caniferolide C, and GT-35 which all displayed activity against L. donovani promastigotes and intracellular amastigotes (Ortega et al., 2021). Cyphomycin inhibited the replication of intracellular amastigotes with a concentration similar to the HAS1 derived compounds (2.32 μM) while demonstrating low cyctotoxicty. Caniferolide C and GT-35 were more potent (IC50 of 0.091 and 0.073 μM respectively) yet showcased intense cytotoxic effects on macrophages.
HAS1-F1 and HAS1-F2 belong to a family of compounds named acetogenins. These polyketide natural products were extensively investigated for their antileishmanial activities. Squamocin and Senegalene were efficient in reducing the proliferation of L. major and L. donovani promastigotes in vitro. The compounds had minimal effective concentrations (MECs), where negative growth was observed, ranging between 25 and 50 μg/ml after 24 h (Sahpaz et al., 1994). Moreover, Squamocin alongside with Rolliniastatine one lysed the promastigotes of several Leishmania strains at a dose of 5 μg/ml, similar to the control drug pentamidine (Fevrier et al., 1999). Our results showed around five folds higher efficacy of HAS1-F1 and HAS1-F2, against L. tropica amastigotes. Nonetheless, the expression levels of the Leishmania-specific kinetoplast markers and its Gp63 decreased significantly upon treatment with either drug as compared to the Untreated infected controls, likely suggestive of an anti-parasitic mode of action. This finding is in line with previously isolated acetogenins such as Rolliniastatin one which limited the replication of both intracellular amastigotes as well as the extracellular promastigotes of L. donovani (Raynaud-Le Grandic et al., 2004). Furthermore, four acetogenins isolated from the seeds of Porcelia macrocarpa showed anti-leishmanial activity on the amastigotes of L. infantum. Among these, the compound (2S,3R,4R)-3-hydroxy-4-methyl-2-(eicos-11′-yn-19′-enyl) butanolide had an IC50 of 29.9 μM with no cytotoxic effects on the NCTC cell line (Brito et al., 2022). Although most of the compounds in this family originate from plants, recent evidence shows that Streptomyces species can generate acetogenins. In that regard, Streptomyces sp. VE2, was able to secrete acetogenins with antibacterial and antioxidant activities (Taechowisan et al., 2016). Therefore, our study is the first to report the isolation of novel acetogenins from a previously uncultured Streptomyces sp. HAS1, that were able to inhibit the replication of L. tropica amastigotes in vitro. Our work marks the first recorded case about establishing a pipeline that screens SMs produced by bacteria against L. tropica, the major causative agent of CL in the EMR region, and foreshadows the invaluable opportunities to screen SMs produced by Streptomyces for CL treatment.
Data availability statement
The datasets presented in this study can be found in online repositories. The names of the repository/repositories and accession number(s) can be found below: https://www.ncbi.nlm.nih.gov/bioproject/PRJNA873891.
Ethics statement
The studies involving human participants were reviewed and approved by Sample collection was approved by the Institutional Review Board of the American University of Beirut (PALK.IK.01). The patients/participants provided their written informed consent to participate in this study.
Author contributions
BA, MH, RH, GD, RN, PM and DA, performed experiments; BA, MH, and RH analyzed results; BA, AF and HH made the Figures; AF and HH designed the research; BA, AF and HH wrote the paper.
Funding
This work was made possible through the support from the Medical Practice Plan (Faculty of Medicine, American University of Beirut).
Acknowledgments
We thank the American University of Beirut Core Facilities for providing access to their imaging, Animal Care, and core culture facilities. We also thank the Office of Grants and Contracts at the American University of Beirut. The authors would also like to acknowledge the National Council for Scientific Research of Lebanon (CNRS-L) for granting a doctoral fellowship to BA. This fellowship was offered in collaboration with the American University of Beirut (AUB).
Conflict of interest
The authors declare that the research was conducted in the absence of any commercial or financial relationships that could be construed as a potential conflict of interest.
Publisher’s note
All claims expressed in this article are solely those of the authors and do not necessarily represent those of their affiliated organizations, or those of the publisher, the editors and the reviewers. Any product that may be evaluated in this article, or claim that may be made by its manufacturer, is not guaranteed or endorsed by the publisher.
Supplementary material
The Supplementary Material for this article can be found online at: https://www.frontiersin.org/articles/10.3389/fphar.2022.1023114/full#supplementary-material
Supplementary Figure S1 | Cytotoxicity testing of HAS1-F1 and HAS1-F2. Peripheral blood mononuclear cells were incubated with different concentrations of HAS1-F1 or HAS1-F2 (1, 5, or 10 μg/ml) for 24 h. Living cells were counted using the Trypan Blue exclusion dye assay. Results are expressed as percentage of the untreated control (±) SEM are representative of three independent experiments. To validate statistical significance, t-tests were used, and no significance was recorded when comparing any of the treatment groups to the control group.
References
Al-Salem, W. S., Pigott, D. M., Subramaniam, K., Haines, L. R., Kelly-Hope, L., Molyneux, D. H., et al. (2016). Cutaneous leishmaniasis and conflict in Syria. Emerg. Infect. Dis. 22, 931–933. doi:10.3201/eid2205.160042
Antoraz, S., Santamaria, R. I., Diaz, M., Sanz, D., and Rodriguez, H. (2015). Toward a new focus in antibiotic and drug discovery from the Streptomyces arsenal. Front. Microbiol. 6, 461. doi:10.3389/fmicb.2015.00461
Arevalo, I., Tulliano, G., Quispe, A., Spaeth, G., Matlashewski, G., Llanos-Cuentas, A., et al. (2007). Role of imiquimod and parenteral meglumine antimoniate in the initial treatment of cutaneous leishmaniasis. Clin. Infect. Dis. 44, 1549–1554. doi:10.1086/518172
Arevalo, I., Ward, B., Miller, R., Meng, T. C., Najar, E., Alvarez, E., et al. (2001). Successful treatment of drug-resistant cutaneous leishmaniasis in humans by use of imiquimod, an immunomodulator. Clin. Infect. Dis. 33, 1847–1851. doi:10.1086/324161
Bailey, F., Mondragon-Shem, K., Hotez, P., Ruiz-Postigo, J. A., Al-Salem, W., Acosta-Serrano, A., et al. (2017). A new perspective on cutaneous leishmaniasis-Implications for global prevalence and burden of disease estimates. PLoS Negl. Trop. Dis. 11, e0005739. doi:10.1371/journal.pntd.0005739
Bizri, N. A., Alam, W., Khoury, M., Musharrafieh, U., Ghosn, N., Berri, A., et al. (2021). The association between the Syrian crisis and cutaneous leishmaniasis in Lebanon. Acta Parasitol. 66, 1240–1245. doi:10.1007/s11686-021-00395-3
Bolger, A. M., Lohse, M., and Usadel, B. (2014). Trimmomatic: A flexible trimmer for Illumina sequence data. Bioinformatics 30, 2114–2120. doi:10.1093/bioinformatics/btu170
Briones Nieva, C. A., Cid, A. G., Romero, A. I., Garcia-Bustos, M. F., Villegas, M., and Bermudez, J. M. (2021). An appraisal of the scientific current situation and new perspectives in the treatment of cutaneous leishmaniasis. Acta Trop. 221, 105988. doi:10.1016/j.actatropica.2021.105988
Brito, I. A., Thevenard, F., Costa-Silva, T. A., Oliveira, S. S., Cunha, R., De Oliveira, E. A., et al. (2022). Antileishmanial effects of acetylene acetogenins from seeds of Porcelia macrocarpa (warm.) R.E. Fries (annonaceae) and semisynthetic derivatives. Molecules 27, 893. doi:10.3390/molecules27030893
Burza, S., Croft, S. L., and Boelaert, M. (2018). Leishmaniasis. Lancet 392, 951–970. doi:10.1016/S0140-6736(18)31204-2
Carter, N. S., Stamper, B. D., Elbarbry, F., Nguyen, V., Lopez, S., Kawasaki, Y., et al. (2021). Natural products that target the arginase in Leishmania parasites hold therapeutic promise. Microorganisms 9, 267. doi:10.3390/microorganisms9020267
Cavalli, A., and Bolognesi, M. L. (2009). Neglected tropical diseases: Multi-target-directed ligands in the search for novel lead candidates against trypanosoma and Leishmania. J. Med. Chem. 52, 7339–7359. doi:10.1021/jm9004835
Chabala, J. C., Mrozik, H., Tolman, R. L., Eskola, P., Lusi, A., Peterson, L. H., et al. (1980). Ivermectin, a new broad-spectrum antiparasitic agent. J. Med. Chem. 23, 1134–1136. doi:10.1021/jm00184a014
Chin, Y. W., Balunas, M. J., Chai, H. B., and Kinghorn, A. D. (2006). Drug discovery from natural sources. AAPS J. 8, E239–E253. doi:10.1007/BF02854894
Cordell, G. A. (2000). Biodiversity and drug discovery-a symbiotic relationship. Phytochemistry 55, 463–480. doi:10.1016/s0031-9422(00)00230-2
Corpas-Lopez, V., Merino-Espinosa, G., Diaz-Saez, V., Morillas-Marquez, F., Navarro-Moll, M. C., and Martin-Sanchez, J. (2016). The sesquiterpene (-)-alpha-bisabolol is active against the causative agents of Old World cutaneous leishmaniasis through the induction of mitochondrial-dependent apoptosis. Apoptosis 21, 1071–1081. doi:10.1007/s10495-016-1282-x
Eiras, D. P., Kirkman, L. A., and Murray, H. W. (2015). Cutaneous leishmaniasis: Current treatment practices in the USA for returning travelers. Curr. Treat. Options Infect. Dis. 7, 52–62. doi:10.1007/s40506-015-0038-4
El Hajj, R., Bou Youness, H., Lachaud, L., Bastien, P., Masquefa, C., Bonnet, P. A., et al. (2018). EAPB0503: An Imiquimod analog with potent in vitro activity against cutaneous leishmaniasis caused by Leishmania major and Leishmania tropica. PLoS Negl. Trop. Dis. 12, e0006854. doi:10.1371/journal.pntd.0006854
Esfandiarpour, I., and Alavi, A. (2002). Evaluating the efficacy of allopurinol and meglumine antimoniate (Glucantime) in the treatment of cutaneous leishmaniasis. Int. J. Dermatol. 41, 521–524. doi:10.1046/j.1365-4362.2002.01526.x
Ezatpour, B., Saedi Dezaki, E., Mahmoudvand, H., Azadpour, M., and Ezzatkhah, F. (2015). In vitro and in vivo antileishmanial effects of Pistacia khinjuk against Leishmania tropica and Leishmania major. Evid. Based. Complement. Altern. Med. 2015, 149707. doi:10.1155/2015/149707
Fakhar, M., Pazoki Ghohe, H., Rasooli, S. A., Karamian, M., Mohib, A. S., Ziaei Hezarjaribi, H., et al. (2016). Genetic diversity of Leishmania tropica strains isolated from clinical forms of cutaneous leishmaniasis in rural districts of Herat province, Western Afghanistan, based on ITS1-rDNA. Infect. Genet. Evol. 41, 120–127. doi:10.1016/j.meegid.2016.03.031
Fevrier, A., Ferreira, M. E., Fournet, A., Yaluff, G., Inchausti, A., Rojas De Arias, A., et al. (1999). Acetogenins and other compounds from Rollinia emarginata and their antiprotozoal activities. Planta Med. 65, 47–49. doi:10.1055/s-1999-13961
Firooz, A., Khamesipour, A., Ghoorchi, M. H., Nassiri-Kashani, M., Eskandari, S. E., Khatami, A., et al. (2006). Imiquimod in combination with meglumine antimoniate for cutaneous leishmaniasis: A randomized assessor-blind controlled trial. Arch. Dermatol. 142, 1575–1579. doi:10.1001/archderm.142.12.1575
Frezard, F., Demicheli, C., and Ribeiro, R. R. (2009). Pentavalent antimonials: New perspectives for old drugs. Molecules 14, 2317–2336. doi:10.3390/molecules14072317
Garza-Tovar, T. F., Sacriste-Hernandez, M. I., Juarez-Duran, E. R., and Arenas, R. (2020). An overview of the treatment of cutaneous leishmaniasis. Fac. Rev. 9, 28. doi:10.12703/r/9-28
Gervazoni, L. F. O., Barcellos, G. B., Ferreira-Paes, T., and Almeida-Amaral, E. E. (2020). Use of natural products in leishmaniasis chemotherapy: An overview. Front. Chem.579891 8. doi:10.3389/fchem.2020.579891
Guerin, P. J., Olliaro, P., Sundar, S., Boelaert, M., Croft, S. L., Desjeux, P., et al. (2002). Visceral leishmaniasis: Current status of control, diagnosis, and treatment, and a proposed research and development agenda. Lancet. Infect. Dis. 2, 494–501. doi:10.1016/s1473-3099(02)00347-x
Hayani, K., Dandashli, A., and Weisshaar, E. (2015). Cutaneous leishmaniasis in Syria: Clinical features, current status and the effects of war. Acta Derm. Venereol. 95, 62–66. doi:10.2340/00015555-1988
Heras-Mosteiro, J., Monge-Maillo, B., Pinart, M., Lopez Pereira, P., Reveiz, L., Garcia-Carrasco, E., et al. (2017). Interventions for old World cutaneous leishmaniasis. Cochrane Database Syst. Rev. 12, CD005067. doi:10.1002/14651858.CD005067.pub5
Kazemi-Rad, E., Mohebali, M., Khadem-Erfan, M. B., Hajjaran, H., Hadighi, R., Khamesipour, A., et al. (2013a). Overexpression of ubiquitin and amino acid permease genes in association with antimony resistance in Leishmania tropica field isolates. Korean J. Parasitol. 51, 413–419. doi:10.3347/kjp.2013.51.4.413
Kazemi-Rad, E., Mohebali, M., Khadem-Erfan, M. B., Saffari, M., Raoofian, R., Hajjaran, H., et al. (2013b). Identification of antimony resistance markers in Leishmania tropica field isolates through a cDNA-AFLP approach. Exp. Parasitol. 135, 344–349. doi:10.1016/j.exppara.2013.07.018
Larsen, M. V., Cosentino, S., Lukjancenko, O., Saputra, D., Rasmussen, S., Hasman, H., et al. (2014). Benchmarking of methods for genomic taxonomy. J. Clin. Microbiol. 52, 1529–1539. doi:10.1128/JCM.02981-13
Lefevre, F., Robe, P., Jarrin, C., Ginolhac, A., Zago, C., Auriol, D., et al. (2008). Drugs from hidden bugs: Their discovery via untapped resources. Res. Microbiol. 159, 153–161. doi:10.1016/j.resmic.2007.12.011
Mahmoudvand, H., Ezzatkhah, F., Sharififar, F., Sharifi, I., and Dezaki, E. S. (2015). Antileishmanial and cytotoxic effects of essential oil and methanolic extract of Myrtus communis L. Korean J. Parasitol. 53, 21–27. doi:10.3347/kjp.2015.53.1.21
Masmoudi, A., Hariz, W., Marrekchi, S., Amouri, M., and Turki, H. (2013). Old World cutaneous leishmaniasis: Diagnosis and treatment. J. Dermatol. Case Rep. 7, 31–41. doi:10.3315/jdcr.2013.1135
Mcdowell, M. A., Rafati, S., Ramalho-Ortigao, M., and Ben Salah, A. (2011). Leishmaniasis: Middle East and north africa research and development priorities. PLoS Negl. Trop. Dis. 5, e1219. doi:10.1371/journal.pntd.0001219
Mehwish, S., Khan, H., Rehman, A. U., Khan, A. U., Khan, M. A., Hayat, O., et al. (2019). Natural compounds from plants controlling leishmanial growth via DNA damage and inhibiting trypanothione reductase and trypanothione synthetase: An in vitro and in silico approach. 3 Biotech. 9, 303. doi:10.1007/s13205-019-1826-1
Miranda-Verastegui, C., Tulliano, G., Gyorkos, T. W., Calderon, W., Rahme, E., Ward, B., et al. (2009). First-line therapy for human cutaneous leishmaniasis in Peru using the TLR7 agonist imiquimod in combination with pentavalent antimony. PLoS Negl. Trop. Dis. 3, e491. doi:10.1371/journal.pntd.0000491
Mohebali, M., Kazemirad, E., Hajjaran, H., Kazemirad, E., Oshaghi, M. A., Raoofian, R., et al. (2019). Gene expression analysis of antimony resistance in Leishmania tropica using quantitative real-time PCR focused on genes involved in trypanothione metabolism and drug transport. Arch. Dermatol. Res. 311, 9–17. doi:10.1007/s00403-018-1872-2
Monge-Maillo, B., and Lopez-Velez, R. (2015). Miltefosine for visceral and cutaneous leishmaniasis: Drug characteristics and evidence-based treatment recommendations. Clin. Infect. Dis. 60, 1398–1404. doi:10.1093/cid/civ004
Newman, D. J., and Cragg, G. M. (2020). Natural products as sources of new drugs over the nearly four decades from 01/1981 to 09/2019. J. Nat. Prod. 83, 770–803. doi:10.1021/acs.jnatprod.9b01285
Oliveira, L. F., Schubach, A. O., Martins, M. M., Passos, S. L., Oliveira, R. V., Marzochi, M. C., et al. (2011). Systematic review of the adverse effects of cutaneous leishmaniasis treatment in the New World. Acta Trop. 118, 87–96. doi:10.1016/j.actatropica.2011.02.007
Ortega, H. E., Ferreira, L. L. G., Melo, W. G. P., Oliveira, A. L. L., Ramos Alvarenga, R. F., Lopes, N. P., et al. (2019). Antifungal compounds from Streptomyces associated with attine ants also inhibit Leishmania donovani. PLoS Negl. Trop. Dis. 13, e0007643. doi:10.1371/journal.pntd.0007643
Ortega, H. E., Lourenzon, V. B., Chevrette, M. G., Ferreira, L. L. G., Alvarenga, R. F. R., Melo, W. G. P., et al. (2021). Antileishmanial macrolides from ant-associated Streptomyces sp. ISID311. Bioorg. Med. Chem. 32, 116016. doi:10.1016/j.bmc.2021.116016
Ozbilgin, A., Zeyrek, F. Y., Guray, M. Z., Culha, G., Akyar, I., Harman, M., et al. (2020). Determination of antimony resistance mechanism of Leishmania tropica causing cutaneous leishmaniasis in Turkey. Mikrobiyol. Bul. 54, 444–462. doi:10.5578/mb.69702
Palumbo, E. (2009). Current treatment for cutaneous leishmaniasis: A review. Am. J. Ther. 16, 178–182. doi:10.1097/MJT.0b013e3181822e90
Pimentel-Elardo, S. M., Kozytska, S., Bugni, T. S., Ireland, C. M., Moll, H., and Hentschel, U. (2010). Anti-parasitic compounds from Streptomyces sp. strains isolated from Mediterranean sponges. Mar. Drugs 8, 373–380. doi:10.3390/md8020373
Pink, R., Hudson, A., Mouries, M. A., and Bendig, M. (2005). Opportunities and challenges in antiparasitic drug discovery. Nat. Rev. Drug Discov. 4, 727–740. doi:10.1038/nrd1824
Quinn, G. A., Banat, A. M., Abdelhameed, A. M., and Banat, I. M. (2020). Streptomyces from traditional medicine: Sources of new innovations in antibiotic discovery. J. Med. Microbiol. 69, 1040–1048. doi:10.1099/jmm.0.001232
Raynaud-Le Grandic, S., Fourneau, C., Laurens, A., Bories, C., Hocquemiller, R., and Loiseau, P. M. (2004). In vitro antileishmanial activity of acetogenins from Annonaceae. Biomed. Pharmacother. 58, 388–392. doi:10.1016/j.biopha.2004.02.007
Rehman, K., Walochnik, J., Mischlinger, J., Alassil, B., Allan, R., and Ramharter, M. (2018). Leishmaniasis in northern Syria during civil war. Emerg. Infect. Dis. 24, 1973–1981. doi:10.3201/eid2411.172146
Reithinger, R. (2016). Global burden of cutaneous leishmaniasis. Lancet. Infect. Dis. 16, 1004–1005. doi:10.1016/S1473-3099(16)30195-5
Roberts, M. T. (2005). Current understandings on the immunology of leishmaniasis and recent developments in prevention and treatment. Br. Med. Bull. 75-76, 115–130. doi:10.1093/bmb/ldl003
Rocha, L. G., Almeida, J. R., Macedo, R. O., and Barbosa-Filho, J. M. (2005). A review of natural products with antileishmanial activity. Phytomedicine 12, 514–535. doi:10.1016/j.phymed.2003.10.006
Saduqi, M., Sharifi, I., Babaei, Z., Keyhani, A., Mostafavi, M., Hakimi Parizi, M., et al. (2019). Anti-leishmanial and immunomodulatory effects of epigallocatechin 3-O-gallate on Leishmania tropica: Apoptosis and gene expression profiling. Iran. J. Parasitol. 14, 521–533.
Sahpaz, S., Bories, C., Loiseau, P. M., Cortes, D., Hocquemiller, R., Laurens, A., et al. (1994). Cytotoxic and antiparasitic activity from Annona senegalensis seeds. Planta Med. 60, 538–540. doi:10.1055/s-2006-959566
Saroufim, M., Charafeddine, K., Issa, G., Khalifeh, H., Habib, R. H., Berry, A., et al. (2014). Ongoing epidemic of cutaneous leishmaniasis among Syrian refugees, Lebanon. Emerg. Infect. Dis. 20, 1712–1715. doi:10.3201/eid2010.140288
Schmittgen, T. D., and Livak, K. J. (2008). Analyzing real-time PCR data by the comparative C(T) method. Nat. Protoc. 3, 1101–1108. doi:10.1038/nprot.2008.73
Sharara, S. L., and Kanj, S. S. (2014). War and infectious diseases: Challenges of the Syrian civil war. PLoS Pathog. 10, e1004438. doi:10.1371/journal.ppat.1004438
Sharma, N. L., Mahajan, V. K., Kanga, A., Sood, A., Katoch, V. M., Mauricio, I., et al. (2005). Localized cutaneous leishmaniasis due to Leishmania donovani and Leishmania tropica: Preliminary findings of the study of 161 new cases from a new endemic focus in Himachal Pradesh, India. Am. J. Trop. Med. Hyg. 72, 819–824. doi:10.4269/ajtmh.2005.72.819
Sreedharan, V., and Bhaskara Rao, K. V. (2017). Efficacy of protease inhibitor from marine Streptomyces sp. VITBVK2 against Leishmania donovani - an in vitro study. Exp. Parasitol. 174, 45–51. doi:10.1016/j.exppara.2017.02.007
Steverding, D. (2017). The history of leishmaniasis. Parasit. Vectors 10, 82. doi:10.1186/s13071-017-2028-5
Taechowisan, T., Singtotong, C., and Phutdhawong, W. S. (2016). Antibacterial and antioxidant activities of acetogenins from Streptomyces sp. VE2; an endophyte in vernonia cinerea (L.) less. J. Appl. Pharm. Sci. 6, 067–072. doi:10.7324/japs.2016.60810
Torres-Bacete, J., Arroyo, M., Torres-Guzman, R., De La Mata, I., Acebal, C., and Castillon, M. P. (2005). Optimization of culture medium and conditions for penicillin acylase production by Streptomyces lavendulae ATCC 13664. Appl. Biochem. Biotechnol. 126, 119–132. doi:10.1385/abab:126:2:119
Torres-Guerrero, E., Quintanilla-Cedillo, M. R., Ruiz-Esmenjaud, J., and Arenas, R. (2017F1000). Leishmaniasis a Rev. 6, 750.
Tulp, M., and Bohlin, L. (2002). Functional versus chemical diversity: Is biodiversity important for drug discovery? Trends Pharmacol. Sci. 23, 225–231. doi:10.1016/s0165-6147(02)02007-2
Wick, R. R., Judd, L. M., Gorrie, C. L., and Holt, K. E. (2017). Completing bacterial genome assemblies with multiplex MinION sequencing. Microb. Genom. 3, e000132. doi:10.1099/mgen.0.000132
Wiwanitkit, V. (2012). Interest in paromomycin for the treatment of visceral leishmaniasis (kala-azar). Ther. Clin. Risk Manag. 8, 323–328. doi:10.2147/TCRM.S30139
Zhu, F., Ma, X. H., Qin, C., Tao, L., Liu, X., Shi, Z., et al. (2012). Drug discovery prospect from untapped species: Indications from approved natural product drugs. PLoS One 7, e39782. doi:10.1371/journal.pone.0039782
Keywords: soil extract, streptomyces, Leishmania tropica, cutaneous leishmaniasis, natural products, bio-guided fractionation
Citation: Awada B, Hamie M, El Hajj R, Derbaj G, Najm R, Makhoul P, Ali DH, Abou Fayad AG and El Hajj H (2022) HAS 1: A natural product from soil-isolated Streptomyces species with potent activity against cutaneous leishmaniasis caused by Leishmania tropica. Front. Pharmacol. 13:1023114. doi: 10.3389/fphar.2022.1023114
Received: 19 August 2022; Accepted: 26 September 2022;
Published: 10 October 2022.
Edited by:
Hidayat Hussain, Leibniz Institute of Plant Biochemistry, GermanyReviewed by:
Muzamil Yaqub Want, Roswell Park Comprehensive Cancer Center, United StatesChaitenya Verma, The Ohio State University, United States
Copyright © 2022 Awada, Hamie, El Hajj, Derbaj, Najm, Makhoul, Ali, Abou Fayad and El Hajj. This is an open-access article distributed under the terms of the Creative Commons Attribution License (CC BY). The use, distribution or reproduction in other forums is permitted, provided the original author(s) and the copyright owner(s) are credited and that the original publication in this journal is cited, in accordance with accepted academic practice. No use, distribution or reproduction is permitted which does not comply with these terms.
*Correspondence: Antoine G. Abou Fayad, YWEzMjhAYXViLmVkdS5sYg==; Hiba El Hajj, aGUyMUBhdWIuZWR1Lmxi
†These authors share first authorship
‡These authors have contributed equally to this work and share senior authorship