- 1Institute of Medical Sciences, University of Toronto, Toronto, ON, Canada
- 2Brain Health Imaging Centre, Centre for Addiction and Mental Health, Toronto, ON, Canada
- 3Faculty of Kinesiology & Physical Education, University of Toronto, Toronto, ON, Canada
- 4Defence Research and Development Canada, Toronto Research Centre, Toronto, ON, Canada
- 5Campbell Mental Health Research Institute, Centre for Addiction and Mental Health, Toronto, ON, Canada
- 6Department of Psychiatry, University of Toronto, Toronto, ON, Canada
- 7Department of Pharmacology and Toxicology, University of Toronto, Toronto, ON, Canada
- 8The MacDonald Franklin Operational Stress Injury (OSI) Research Centre, Lawson Health Research Institute, London, ON, Canada
- 9Department of Psychiatry, Western University, London, ON, Canada
- 10Department of Psychiatry and Behavioural Neurosciences, McMaster University, Hamilton, ON, Canada
- 11St. Joseph's London Operational Stress Injury (OSI), Parkwood Institute, St. Joseph's Health Care, London, ON, Canada
Introduction: Oxidative stress has been implicated in psychiatric disorders, including posttraumatic stress disorder (PTSD). Currently, the status of glutathione (GSH), the brain's most abundant antioxidant, in PTSD remains uncertain. Therefore, the current study investigated brain concentrations of GSH and peripheral concentrations of blood markers in individuals with PTSD vs. Healthy Controls (HC).
Methods: GSH spectra was acquired in the anterior cingulate cortex (ACC) and dorsolateral prefrontal cortex (DLPFC) using MEGA-PRESS, a J-difference-editing acquisition method. Peripheral blood samples were analyzed for concentrations of metalloproteinase (MMP)-9, tissue inhibitors of MMP (TIMP)-1,2, and myeloperoxidase (MPO).
Results: There was no difference in GSH between PTSD and HC in the ACC (n = 30 PTSD, n = 20 HC) or DLPFC (n = 14 PTSD, n = 18 HC). There were no group differences between peripheral blood markers (P > 0.3) except for (non-significantly) lower TIMP-2 in PTSD. Additionally, TIMP-2 and GSH in the ACC were positively related in those with PTSD. Finally, MPO and MMP-9 were negatively associated with duration of PTSD.
Conclusions: We do not report altered GSH concentrations in the ACC or DLPFC in PTSD, however, systemic MMPs and MPO might be implicated in central processes and progression of PTSD. Future research should investigate these relationships in larger sample sizes.
1. Introduction
There is a need to develop effective medication for posttraumatic stress disorder (PTSD), as currently, < 30% of people diagnosed with PTSD will achieve remission (1). Employing brain imaging to understand the underlying neurobiology of PTSD can identify therapeutic targets. Magnetic Resonance Spectroscopy (MRS) is a valuable imaging modality that enables the in vivo measurement of brain-based metabolites. Indeed, MRS has been employed in PTSD to measure selected brain metabolites; to date the literature reports decreases in N-acetylaspartate (NAA) a marker of neuronal integrity and density, and mixed or null findings of glutamate (Glu), γ-aminobutyric acid (GABA), myo-inositol (mI), and choline (Cho) (2). Since NAA is a mitochondrial metabolite, decreased NAA could reflect damaged mitochondria in cerebral tissue (3). Oxidative stress is a primary driver of mitochondrial dysfunction/damage (4). Interestingly, the status of glutathione (GSH), the brain main's antioxidant in PTSD remains uncertain, as to our knowledge, the one study (5), investigating GSH in PTSD employed an MRS sequence that is not validated to measure GSH in the brain [see Rae and Williams (6) and discussion].
It is not surprising that oxidative stress and redox biology have been implicated in PTSD (7, 8), considering that the brain in particular is susceptible to oxidative stress related damage due to the central organ's high lipid content and metabolic rate (7). Notably, relevant features of PTSD, including dysregulated hypothalamic pituitary adrenal (HPA) axis (9), sleep disturbances (10), metabolic syndromes (11), neurodegeneration (12), inflammation (13), and brain atrophy (14) are also associated with oxidative stress (15). Preclinical research investigating oxidative stress in animal models of PTSD has reported decreased antioxidants (e.g., GSH) or increased free radical by-products [e.g., malondialdehyde (MDA)] and related enzymes in various regions of the brain such as the hippocampus (16–19), amygdala (20, 21), and prefrontal cortex (PFC) (20, 22, 23). Human post-mortem brain analysis has also reported alterations in genes related to oxidative stress in the dorsolateral PFC (DLPFC) of patients with PTSD (24). GSH plays an important role in protecting the central nervous system from oxidative stress related damage, where it serves as a co-factor for antioxidant enzymes including glutathione peroxidase, which functions to detoxify peroxides, as well as glutathione-s-transferase, which functions to reverse oxidized protein residues (25), and is, therefore, a useful molecule to investigate to understand the role oxidative stress might have in PTSD.
Also of interest, are various molecules circulating in peripheral blood that might relate to central markers of oxidative stress. Several lines of inquiry have investigated concentrations of common inflammatory mediators, including interleukin (IL)-1β, IL-6, and tumor necrosis factor (TNF)- α, with mixed results reported (26). An emerging line of interest (not yet investigated in humans living with PTSD), has begun to explore matrix metalloproteinases (MMPs) and their tissue inhibitors (tissue inhibitor of metalloproteinases (TIMPs) in psychiatric disorders (27). MMPs are extracellular matrix (ECM) proteins that help degrade ECM molecules and release growth factors. MMP-9 (28–30) and TIMP-2 (31) have been implicated in preclinical models of PTSD and its clinical features including learned fear. Also of interest is myeloperoxidase (MPO), a peroxidase enzyme that can catalyze the formation of ROS. MPO has also been implicated in PTSD (32), as well as major depressive disorder (MDD) (33). While these proteins are not by-products of oxidative stress or antioxidants, they can be activated by oxidative stress and correlate with TBARS (by-product of lipid peroxidation) (34, 35). To date, the relationship between these peripheral markers and brain GSH has not been explored. Therefore, the current study employed MEGA-PRESS (optimized for GSH) to quantify brain GSH in the ACC and DLPFC [to replicate previous research (5)] in people with PTSD and healthy controls. The investigation of brain GSH was complemented by the measurement of plasma levels of MMP-9, MPO, TIMP-1&2. Our primary aim was to investigate group differences in brain GSH and peripheral blood markers; secondarily, we aimed to explore relationships between central and peripheral markers and features/symptoms related to PTSD.
2. Materials and methods
2.1. Participants
This study was performed from September 2017 to October 2022 at the Center for Addiction and Mental Health (CAMH, Toronto, ON). After receiving approval for this study from the CAMH Research Ethics Board, research participants were recruited from the Greater Toronto Area and relevant communities/clinics (including Parkwood Operational Stress Injury Clinic, London ON) using posted and online advertisements and brochures. After providing written informed consent, research participants completed a comprehensive medical and psychiatric screening assessment (using the structured clinical interview for DSM (SCID)-IV/5) (36) and questionnaires assessing mood [Generalized Anxiety Disorder (GAD)-7 (37), Patient Health Questionnaire (PHQ)-9 (38), Beck Depression Inventory (BDI) (39)], PTSD symptoms [PTSD symptom scale (PSS) (40) and PTSD Checklist (PCL) (41)], and the traumatic life events questionnaire (42) at CAMH. Research staff also collected urine samples to screen for drug use, medication and pregnancy in female participants. Volunteers were eligible to participate if they were 17 years old or older, physically healthy, and had no current or previous DSM Axis I diagnosis except co-morbid mood disorder with PTSD (PTSD group only). PTSD participants were included if they met criteria for PTSD based on the Structured Clinical Interview for DSM-IV-5. Cannabis and medication use was not exclusionary in the PTSD group as long as participants did not meet criteria for current substance use disorder (according to DSM-IV/5 criteria). Nicotine dependence was not exclusionary in both study groups.
2.2. MRI session
On magnetic resonance imaging (MRI) scan day, urine toxicology (BTNX Inc. Pickering, Canada), breath alcohol and expired carbon monoxide measurements were taken to assess recent alcohol and smoking. Additionally, a urine sample was collected to detect substance use and medications (and to confirm that female participants were not pregnant). MRI scans took place in a 3T GE Discovery scanner (GE Healthcare; SW: DV26 201) in the Brain Health Imaging Center at CAMH for ~1.5 h. To minimize head movement, each participant was positioned at the center of the eight-channel head coil with soft padding around the head. Magnet homogeneity was adjusted using the manufacture automated shimming routine. High resolution SagT1-weighted BRAVO images were obtained for each participant [echo time (TE) = 3.016 ms; recovery time (TR) = 6.768 ms; field of view (FOV) = 256 × 256; scan time = 4:42 (min:sec)].
2.3. MRS data acquisition and analysis
All participants completed an MRS scan where spectra were obtained from one region of interest: the anterior cingulate cortex (ACC). A subset of participants also completed an MR scan where spectra were obtained from the ACC as well as the left DLPFC (Figure 1). Voxel dimensions for both ROIs were 4 cm x 2 cm x 3 cm, resulting in a nominal size of 24 cc. Shimming was performed using the manufacture automated shimming routine (AUTOSHIM), to achieve a full-width at half maximum (FWHM) ≤ 10 Hz. The MEGA-PRESS sequence was used to obtain MRS spectra as previously described (43, 44). MEGA-PRESS acquires spectra under two different conditions in an interleaved manner: editing “on,” which applies a frequency selective RF inversion (editing) pulse targeting the protons of GSH's cysteine moiety at 4.56 ppm; and editing “off” with editing pulse set to 7.5 ppm, a region with no metabolite resonances. Upon subtraction of the “on” and “off” conditions, the edited-GSH resonance at 2.95 ppm is observed, uncovered from the previously overlapped Cr resonant peak (Figure 1). Data acquisition parameters were: TE = 68 ms; TR = 1.5 s; spectral width = 5,000 Hz; number of points per spectrum = 4,096; NEX = 8; total averages acquired = 512; editing RF pulse width = 14.4 ms; scan time = 13:12 (min:sec).
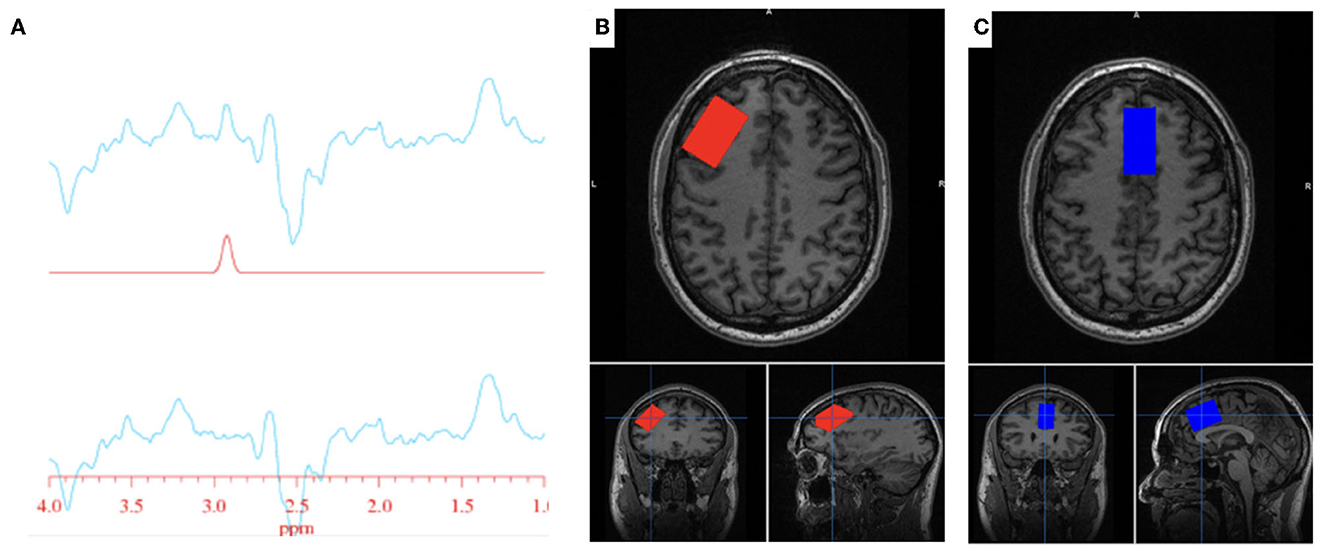
Figure 1. GSH metabolite acquisition. (A) Fitted GSH spectra obtained at 2.95 ppm (top line: difference spectrum; middle line: GSH model fit; bottom line: difference spectrum—model fit). (B) Voxel placement in the left DLPFC; (C) Voxel placement in the ACC.
IDL-based software [XsOs-NMR (45)] was used to process the edited GSH and the unsuppressed water spectra. Raw MRS data from each coil was combined in the time domain based on coil sensitivity (46) from the unsuppressed water signal, weighted by the sum of squares of the signal intensities from each coil. The data was spectrally apodised with a 3 Hz Gaussian filter and then zero filled to 8,192 points, prior to being Fourier transformed. Frequency alignment, additional manual phasing and baseline correction was performed on the data prior to fitting. Edited GSH and unsuppressed water peaks were modeled using pseudo-voight fitting functions and then fitted in the frequency domain using a highly optimized public-domain Levenberg-Marquardt non-linear least-squares minimization routine, MPFIT (47). Due to the manual phasing and baseline correction that require user input, the data set was randomized and processed two more times by the same user, resulting in three measurements per scan. The measurements were averaged together, and the standard deviation (SD) was calculated. The coefficient of variability (%CV = SD/average) was used to assess the reproducibility of the user. Histograms of the %CV could be used to identify outliers. We found that a %CV threshold of 10% yielded good results and excluded spectra that were visibly of poor quality. SPM12 (48) was used for tissue segmentation of the T1 images. MRS voxel and image registration and fractional tissue within voxel was performed using Gannet and SPM12 (49, 50); data were inspected for correct voxel placement.
2.4. Blood samples
Participants provided peripheral blood samples as part of a larger study (51). Venous blood was drawn into a 10-mL K2EDTA tube and left at room temperature for ~45 min before a 20-min centrifugation at room temperature. Plasma supernatant was then aliquoted and frozen at −80°C until analysis. Biofluid analysis comprised the quantitation of MMP-9, TIMP-1,2, and MPO using Simple Plex™ cartridges on the automated Ella® fluorescence-based detection immunoassay system (ProteinSimple, Biotechne, San Jose, CA, USA) (52). Simple Plex cartridges were run according to the manufacturer's instructions, and data were processed automatically using default software settings as outlined in the Ella User's Guide. About an hour after test initiation, triplicate results for every analyte of each sample are provided and blood analyte concentrations are reported as the calculated mean of triplicate values.
2.5. Statistical analysis
Descriptive statistics (mean/median, standard deviation/interquartile ranges) were calculated for participant demographics and medical history (e.g., age, sex, race, and questionnaire scores). Group differences were evaluated by independent samples t-tests, Mann–Whitney U tests, or Chi square tests where appropriate. Independent samples t-tests were employed to evaluate group differences in GSH (in the ACC and DLPFC), MPO, MMP-9, and TIMP-1,2, with follow up tests completed to control for age, sex, BMI, and cannabis. Additional t-tests were completed in the PTSD only group to assess differences in GSH and blood markers between subgroups of PTSD participants (e.g., medication, history of brain injury, comorbid MDD). Two-tailed Pearson correlations were employed to evaluate possible correlations between (1) peripheral and central markers of oxidative stress and (2) markers of oxidative stress and PTSD characteristics and symptoms. Next, interacting variables between centered peripheral blood marker data and group status were computed (biomarker * group) and entered into a linear regression model to predict brain GSH in the ACC and DLPFC. All statistical analysis was conducted using IBM SPSS Statistics 27 (Armonk, New York, USA).
3. Results
3.1. Participants
Thirty-two participants with PTSD and 24 healthy controls (HC) were enrolled and scanned with MRS in the current study. ACC single-voxel MRS was acquired in 32 PTSD participants and 24 HC; of those 30 PTSD participants and 24 HC had usable data. DLPFC single-voxel MRS was acquired in 17 PTSD participants and 24 HC and of those 14 PTSD participants and 18 HC had usable data. Peripheral venous blood samples were obtained from 48 participants (n = 25 PTSD and n = 23 HC). One hundred percent of samples across all four blood biomarkers (MPO, MMP-9, TIMP-1, and TIMP-2) were within the level of detection and had a triplicate CV value < 20%. Of the PTSD participants with blood marker data, 23 had GSH scan data in the ACC and 14 in the DLPFC. Of the HC participants with blood marker data, 19 had GSH scan data in the ACC and 17 in the DLPFC (see Supplementary Figure 1 for sample visualization). A table of demographics for participants with available MRS data in the ACC is presented in Table 1. A separate table of demographics is available for participants with usable MRS data in the DLPFC (Supplementary Table 1). Participants with PTSD were older than HC participants and reported using more cannabis compared to HC.
PTSD specific characteristics are presented in Table 2. Participants scored ~36 on the PSS and 60 on the PCL questionnaires and had been suffering from PTSD related symptoms for 6 years. Twenty-five percent of participants were also diagnosed with current co-morbid MDD and half reported a history of mild traumatic brain injury (mTBI). The majority (88%) of participants were on medication, the most common type being selective serotonin reuptake inhibitor (SSRI)s, followed by cannabis (25%). Although the primary trauma was occupation related in 84% of participants, participants reported a range of trauma exposure including early childhood trauma (38%) and physical (56%) and sexual (22%) violence.
3.2. No group differences in brain levels of GSH
We found no differences in brain levels of GSH in the ACC (-3%, p = 0.536) or in the DLPFC in PTSD vs. HC (−3.5%, p = 0.618, see Figure 2). GSH levels were not related to age (ACC: p = 0.621; DLPFC: p = 0.805), sex (ACC: p = 0.681; DLPFC: p = 0.142) and BMI (ACC: p = 0.492; DLPFC: p = 0.527) in the overall sample and in the groups independently (PTSD and HC; P > 0.2). Testing positive for cannabis on scan day did not affect GSH levels in HC participants (4 THC+ vs. 20 THC-; ACC: p = 0.449; DLPFC: p = 0.326); however, GSH levels in the DLPFC were marginally higher in PTSD participants who tested positive for cannabis (n = 10) on scan day (p = 0.053, 25% higher), compared to those who tested negative (n = 4). Use of SSRIs did not influence brain levels of GSH (ACC n = 12/30: p = 0.275; DLPFC n = 7/14: p = 0.912). There was no difference in GSH levels in the ACC between PTSD participants with (n = 15) and without (n = 15) a history of mTBI (p = 0.449); however, GSH was 24% higher in the DLPFC (p = 0.03) in PTSD participants with a history of mTBI (n = 6) compared to those without (n = 8). Comorbid MDD (PTSD + MDD) did not affect GSH in the ACC (p = 0.623, n = 23 PTSD, n = 7 PTSD+MDD), nor in the DLPFC (p = 0.457, n = 9 PTSD, n = 5 PTSD + MDD).
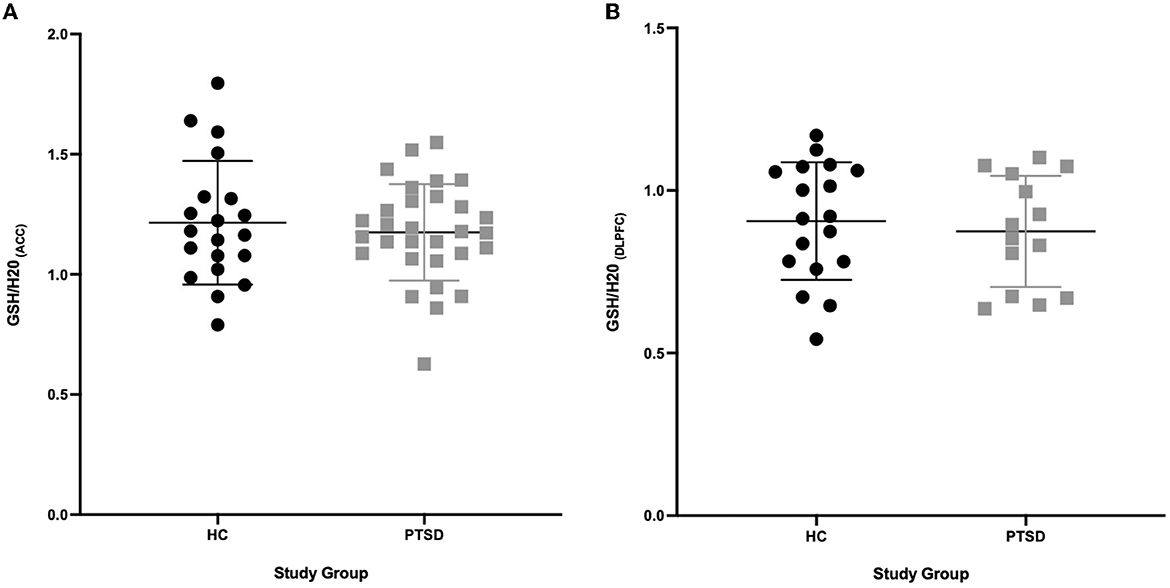
Figure 2. GSH between PTSD and HC in the ACC and DLPFC. (A) No difference (P = 0.536) in GSH concentrations in the ACC between PTSD participants (n = 30) and HC (n = 20). (B) No difference (P = 0.618) in GSH concentrations in the DLPFC between PTSD participants (n = 14) and HC (n = 18).
3.3. Are there group differences in circulating blood markers?
There were no group differences in circulating plasma concentrations of MPO (p = 0.887), MMP-9 (p = 0.345), or TIMP-1 (p = 0.881). TIMP-2 was marginally lower in PTSD participants compared to HC (11%; p = 0.052). All group means are presented in Table 3.
There was no effect of sex (p = 0.321; p = 0.362; p = 0.301; p = 0.216) and BMI (p = 0.564; p = 0.209; p = 0.405; p = 0.935) on MPO, MMP-9 and TIMP-1 and TIMP-2 concentrations in the sample overall. Age was positively related to TIMP-1 levels in the overall sample (p = 0.024). An analysis of variance taking age into consideration did not change the TIMP-1 finding (p = 0.463).
Testing positive for cannabis on scan day did not affect MMP-9, TIMP-1 and TIMP-2 concentrations (P > 0.4). However, PTSD participants testing positive for cannabis on scan day (n = 10) had nominally higher MPO concentrations (p = 0.167, 26% higher) compared to PTSD participants who tested negative (n = 15). Use of SSRIs did not appear to influence circulating concentrations of MPO (p = 0.522), MMP-9 (p = 0.645), nor TIMP-2 (p = 0.337). Concentrations of TIMP-1 were 15% higher (p = 0.074) among PTSD participants on SSRIs (n = 13) compared to participants not on SSRIs (n = 12). There were no differences in circulating concentrations of MMP-9 (p = 0.697), MPO (p = 0.544), TIMP-1 (p = 0.252), nor TIMP-2 (p = 0.971) between PTSD participants with (n = 13) and without (n = 12) a history of mTBI. PTSD + MDD (n = 18) and PTSD only (n = 7) participants did not differ in circulating levels of MMP-9 (p = 0.282), TIMP-1 (p = 0.394), nor TIMP-2 (p = 0.784). PTSD+MDD (n = 7) participants had 30% higher (p = 0.015) MPO concentrations compared to PTSD only participants (n = 18).
3.4. Relationship between GSH and peripheral blood markers
Correlational analysis was employed to evaluate relationships between central and peripheral markers of oxidative stress and PTSD clinical characteristics within the PTSD group. GSH in the ACC was positively correlated with circulating concentrations of TIMP-2 (R = 0.539, p = 0.008). MPO (R = −0.566, p = 0.044) and MMP-9 (R = −0.441, p = 0.05) were negatively correlated and TIMP-1 (R = 0.367, p = 0.089) was marginally positively correlated with duration of PTSD illness (see Figure 3). There were no other relationships between brain, peripheral markers of oxidative stress and symptom scores on questionnaires assessing PTSD, anxiety, nor depression (P > 0.3).
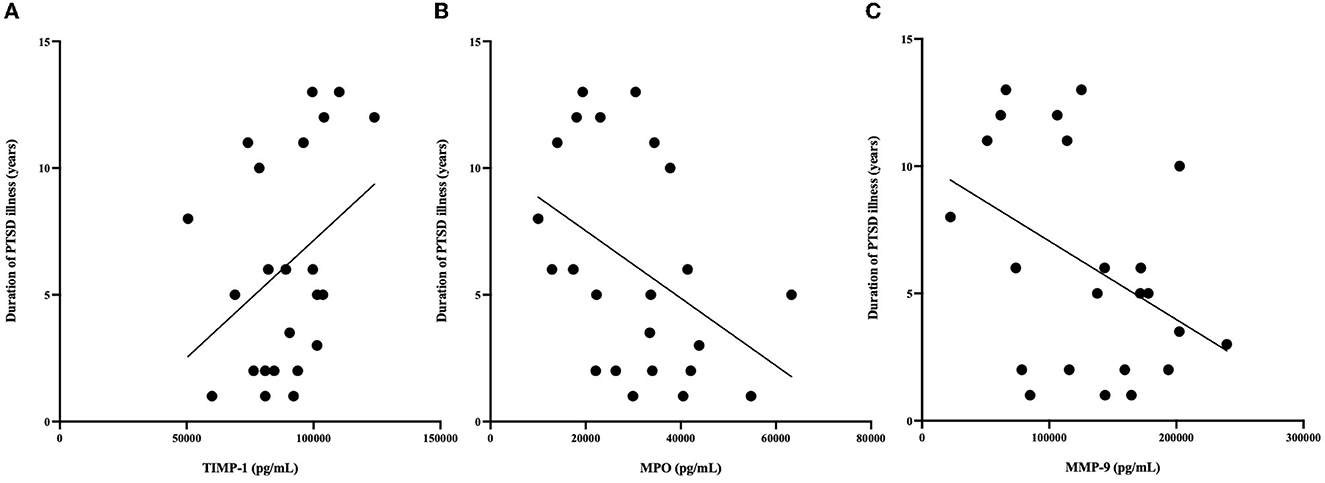
Figure 3. (A) Relationship between MPO (pg/mL) and duration of illness. MPO concentrations were negatively correlated with duration of PTSD illness (R = −0.566, P = 0.044). (B) Relationship between MMP-9 (pg/mL) and duration of illness. MMP-9 concentrations were negatively correlated with duration of PTSD illness (R = −0.41 P = 0.05). (C) Relationship between TIMP-1 (pg/mL) and duration of illness. TIMP-1 concentrations were correlated with duration of PTSD illness (R = 0.367, P = 0.089). *n = 23 since age at time of PTSD diagnosis was not available for 2 PTSD participants.
To determine if the relationship between peripheral markers and central GSH concentrations was group dependent, interaction terms were calculated for group * peripheral marker and entered into linear regression models to predict GSH in the ACC and DLPFC. There were no significant group*blood marker interactions in predicting GSH in the DLPFC (P > 0.7). Additionally, there were no group*blood marker interactions for MMP-9 nor TIMP-1 (P > 0.4). The relationship between TIMP-2 and GSH in the ACC seemed marginally group dependent (β = −0.784, p =0.051, see Figure 4), where the relationship was positive in PTSD participants but negative among HC participants. The relationship between MPO and GSH in the ACC also appeared to be marginally influenced by group (β = −0.389, p =0.099), where the relationship was positive among PTSD participants and negative among HC participants.
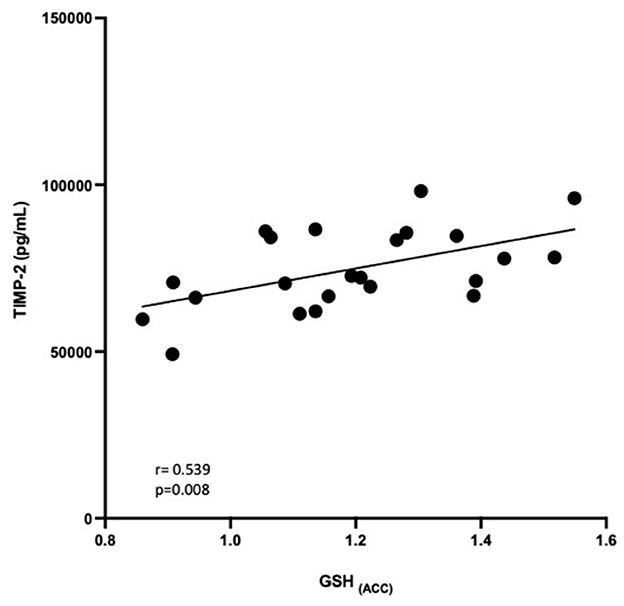
Figure 4. Relationship between GSH in the ACC and TIMP-2 concentrations (pg/mL). GSH in the ACC was positively correlated with TIMP-2 concentrations (R = 0.539, P = 0.008).
4. Discussion
To the extent of our knowledge, this is the first study to quantify brain GSH, using a validated MRS method (53), and explore relationships between peripheral MMPs, TIMPs, and MPO in participants with PTSD and HC. We did not observe any significant group differences in brain GSH or circulating concentrations of peripheral blood markers. Interestingly, peripheral TIMP-2 concentrations (which were marginally lower in PTSD) were positively correlated with GSH in the ACC within the PTSD group only. Additionally, we observed that, in PTSD participants, GSH in the DLPFC (but not in the ACC) is elevated in cannabis users and in individuals with a history of mTBI (albeit a small n = 10 sample size); that MPO was associated with cannabis use and comorbid MDD and that TIMP-1 was elevated among PTSD participants who endorsed SSRI use. Finally, duration of PTSD illness was negatively related with MMP-9 and MPO.
4.1. Group differences
Our null GSH finding in the ACC and DLPFC is at odds with Michels et al. (5) who reported a 22% increase in GSH (in ACC and DLPFC) among PTSD participants. Notably, the GSH acquisition methods were different between studies; while both employed MEGA-PRESS to acquire, Michels et al. (5)'s scanning protocol was optimized for detecting GABA and not GSH, while our scanning protocol was optimized for GSH. Next, PTSD participants enrolled in both studies were suffering from PTSD for similar durations (~5 years) and were similar ages (38 vs. 42 years). However, the current study's PTSD sample consisted of 16 males (53%) while Michels et al. (5)'s sample was 91% (11/12) female. Additionally, we enrolled 8 cannabis users while Michels et al. (5) did not report substance use. We observed marginally higher GSH (DLPFC) in PTSD participants who reported cannabis use (n = 10). Previous research has reported no difference in brain GSH (medial PFC) between healthy controls and regular cannabis users (54), while preclinical research has observed increased brain GSH following administration of cannabis (55). A recent review assessing the therapeutic potential for cannabis in counteracting inflammation and oxidative stress (56) concluded that while preclinical evidence supports this, the clinical evidence in humans is not convincing. We also observed nominally higher (16%) GSH (DLPFC) in PTSD males compared to females and higher GSH (DLPFC) among PTSD participants with a history of mTBI (n = 6). Previous research has associated increased brain GSH in a persistent concussion symptom cohort (57) and athletes exposed to repetitive head impacts during sport (58). The authors speculated that increased GSH reflected a compensatory response to ongoing inflammatory processes related to brain injury. That this finding was only observed in the DLPFC (and not the ACC) in our cohort is in line with research observing frontal cortical thinning in blast exposed veterans (59), decreased activity (60), and white matter damage (61) in the PFC following mTBI. It is possible the ongoing inflammatory injury processes related to mTBI exacerbated oxidative stress in this region.
There are several possible reasons why the current study did not observe group differences in brain GSH. First, GSH is present in low concentrations in the brain (62), therefore any changes related to oxidative damage that might occur in PTSD might not be reflected in GSH quantifications. Several studies report alterations in peripheral plasma concentrations of GSH and GSH related enzymes, however research suggests central GSH dysfunction differs from peripheral dysfunction (62). It is also possible that any oxidative damage that might occur in PTSD, is not sufficient to alter GSH concentrations. GSH is synthesized de novo in the brain by astrocytes and research has demonstrated increased GSH synthesis during oxidative stress-related toxicity in vitro, proposing in vivo synthesis of this antioxidant might be sufficient to not detect significant changes (14). Perhaps concentrations of the molecules from which GSH is derived might reveal group differences in this oxidative stress defense system. Follow-up investigations should continue to explore the role brain GSH and other central markers of oxidative stress might have in PTSD.
4.2. TIMP-2 is positively correlated with GSH in the ACC
Although we did not detect group differences in GSH between groups, we observed a positive relationship between GSH in the ACC and TIMP-2 concentrations in the PTSD group only (TIMP-2 was non-significantly, negatively correlated with GSH in the ACC in the HC group). Notably, TIMP-2 was marginally lower in PTSD compared to HC. This group dependent relationship of TIMP-2 and GSH suggests GSH could still be implicated in PTSD. Lower TIMP-2 has been reported in other psychiatric disorders, including MDD (63), and schizophrenia (64). Interestingly, TIMP-2 knock out mice show deficits in fear potentiated startle (31), a relevant feature of PTSD (65). TIMP-2 is a tissue inhibitor for MMP-2, another ECM protein. MMP-2 has been shown to have a role in synaptic plasticity (66) and be upregulated by noradrenaline (67)—two biological mechanisms implicated in PTSD. ROS can activate MMPs (including MMP-2) and simultaneously decrease concentrations of TIMPs (including TIMP-2) and this can contribute to BBB permeability (68). It is possible that ongoing oxidative stress in the brain related to PTSD is depleting GSH concentrations and the lower TIMP-2 is detected in peripheral circulation due to enhanced BBB permeability. Additionally, MMP-2 is frequently implicated in cardiac pathologies (69), a common outcome in those diagnosed with PTSD (70). Therefore, more research should explore the role TIMP-2 and MMP-2 may have in PTSD, and how they not only relate to central oxidative stress processes, but common somatic comorbidities in PTSD as well.
4.3. Relationships with duration of PTSD
We observed duration of PTSD illness (years) was negatively correlated with MPO and MMP-9 (after controlling for age). Michels et al. (5) reported a positive correlation between GSH in the DLPFC and duration of PTSD in their cohort. While we believe that this is the first study to observe this relationship in PTSD, MPO has been positively correlated with duration of bipolar disorder (71), and higher MMP-9 has been reported in younger youth diagnosed with bipolar disorder compared to older adults (72). MPO is an enzyme that carries out peroxidative activities and is released by neutrophils, also reflecting the state of neutrophils in the innate immune response (73). Research has also reported increased MPO in MDD (33), neurodegenerative disorders including Alzheimer's Disease (74), and preclinical PTSD (32). It is noteworthy that we observed lower MPO concentrations in participants who had been suffering from PTSD for longer. There was no difference in severity of PTSD or symptom scores between participants diagnosed with PTSD recently or several years prior. During the innate immune response, neutrophils respond by releasing a burst of ROS (and MPO); however, research has suggested chronic stress can compromise this function (75). Therefore, it is possible the chronic duration of PTSD has resulted in a similar immune exhaustion in our cohort. MMP-9 is an ECM protein that has been increasingly implicated in psychiatric disorders, including PTSD (28). There is a strong line of research implicating MMP-9 in sleep and memory consolidation processes and MMP-9 is upregulated during contextual fear learning (76). Furthermore, treatment with an MMP inhibitor can disrupt fear reconsolidation (77) and fear memory (78) in preclinical research. Again, the negative relationship between duration of PTSD and MMP-9 is important, considering the research discussed above. Glucocorticoids, including cortisol, can regulate MMP-9 (79), therefore, it is possible that this negative relationship is related to chronic cortisol dysregulation in PTSD. MMP-9 is inhibited by TIMP-1, an MMP tissue inhibitor. TIMP-1 was positively related with duration of PTSD (before controlling for age). Preclinical research suggests TIMP-1 is implicated in fear and memory processes (80), and has shown to have a protective role in neurodegenerative disorder (81). Further research is required to understand the role MMPs, TIMPs, and MPO have in the chronic, progressive nature of PTSD (82).
4.4. Limitations
While this research is an important contribution to understanding oxidative stress in PTSD, it is not without limitations. First, we used a manual software (XsOs) to process (baseline correct and phase) our GSH spectra (manual processing yielded higher quality spectra than automatic processing software); we attempted to negate any operator bias by randomizing the data and having each data point processed three times by the same research staff member (SEW). Next, while the MEGA-PRESS sequence was optimized for GSH, the obtained signal is relatively small and can only be quantified with relatively large error. However, test re-test %CV of our GSH acquisition was 15%, which is within range of previous research (83, 84). Research (53) also demonstrated MEGA-PRESS is able to accurately quantify GSH at low physiological concentrations. Next, we had a small sample size (n = 14) in the DLPFC which made it difficult to make comparisons between regions (ACC vs. DLPFC) and dissect the influence of medication, mTBI, and cannabis on GSH in the region, therefore findings in this region should be interpreted with caution and merit further investigation. Additionally, it would have been useful to assess GSH in other regions implicated in PTSD, including the hippocampus and amygdala. Finally, while we believe the collection of peripheral blood markers complimented our central measures, it would have been informative to measure additional redox proteins directly implicated in oxidative stress processes, including peripheral TBARS and MDA concentrations.
4.5. Conclusions and next steps
In summary, the current study did not observe altered brain GSH concentrations in PTSD. We did however report a non-significant decrease in TIMP-2 in PTSD and TIMP-2 was positively correlated with GSH in the ACC in the PTSD group only. Finally, we observed negative relationships between MPO, MMP-9 and duration of PTSD illness. Our interesting findings among the peripheral blood markers warrant further investigation to understand how, if at all, systemic dysregulation of systems is implicated in the progression of PTSD over time. Future research is needed to better understand ongoing central oxidative stress processes in relation to peripheral mechanisms, and how these mediators change across the course of disease in people with PTSD.
Data availability statement
The raw data supporting the conclusions of this article will be made available by the authors, without undue reservation.
Ethics statement
The studies involving human participants were reviewed and approved by CAMH Research Ethics Board. The patients/participants provided their written informed consent to participate in this study.
Author contributions
SR, JR, SK, and IB contributed to study conceptualization and study design. SW, JW, DG, TM, PT, and SC contributed to data collection and data processing. SW, SR, and IB contributed to manuscript drafting. All authors contributed to manuscript editing and reviewing.
Funding
Funded by the Department of National Defence and Canadian Institute for Military and Veteran Health Research (CIMVHR) through a sub-award to IB.
Acknowledgments
We would like to thank the staff of the Centre for Addiction and Mental Health Brain Health Imaging Centre.
Conflict of interest
The authors declare that the research was conducted in the absence of any commercial or financial relationships that could be construed as a potential conflict of interest.
Publisher's note
All claims expressed in this article are solely those of the authors and do not necessarily represent those of their affiliated organizations, or those of the publisher, the editors and the reviewers. Any product that may be evaluated in this article, or claim that may be made by its manufacturer, is not guaranteed or endorsed by the publisher.
Supplementary material
The Supplementary Material for this article can be found online at: https://www.frontiersin.org/articles/10.3389/fpsyt.2023.1195012/full#supplementary-material
References
1. Stein MB, Kline NA, Matloff JL. Adjunctive olanzapine for SSRI-resistant combat-related PTSD: a double-blind, placebo-controlled study. Am J Psychiatry. (2002) 159:1777–9. doi: 10.1176/appi.ajp.159.10.1777
2. Quadrelli S, Mountford C, Ramadan S. Systematic review of in-vivo neuro magnetic resonance spectroscopy for the assessment of posttraumatic stress disorder. Psychiatry Res Neuroimaging. (2018) 282:110–25. doi: 10.1016/j.pscychresns.2018.07.001
3. Barcelos IPd, Troxell RM, Graves JS. Mitochondrial dysfunction and multiple sclerosis. Biology. (2019) 8:37. doi: 10.3390/biology8020037
4. Elfawy HA, Das B. Crosstalk between mitochondrial dysfunction, oxidative stress, and age related neurodegenerative disease: etiologies and therapeutic strategies. Life Sci. (2019) 218:165–84. doi: 10.1016/j.lfs.2018.12.029
5. Michels L, Schulte-Vels T, Schick M, O'Gorman RL, Zeffiro T, Hasler G, et al. Prefrontal GABA and glutathione imbalance in posttraumatic stress disorder: preliminary findings. Psychiatry Res Neuroimaging. (2014) 224:288–95. doi: 10.1016/j.pscychresns.2014.09.007
6. Rae CD, Williams SR. Glutathione in the human brain: review of its roles and measurement by magnetic resonance spectroscopy. Anal Biochem. (2017) 529:127–43. doi: 10.1016/j.ab.2016.12.022
7. Fung L, Hardan A. Oxidative stress in psychiatric disorders. In:Frye RE, Berk M, , editors. The Therapeutic Use of N-Acetylcysteine (NAC) in Medicine. Singapore: Springer Singapore (2019). p. 53–72. doi: 10.1007/978-981-10-5311-5_4
8. Karanikas E, Daskalakis NP, Agorastos A. Oxidative dysregulation in early life stress and posttraumatic stress disorder: a comprehensive review. Brain Sci. (2021) 11:723. doi: 10.3390/brainsci11060723
9. Dunlop BW, Wong A. The hypothalamic-pituitary-adrenal axis in PTSD: pathophysiology and treatment interventions. Progress Neuro Psychopharmacol Biol Psychiatry. (2019) 89:361–79. doi: 10.1016/j.pnpbp.2018.10.010
10. Richards A, Kanady JC, Neylan TC. Sleep disturbance in PTSD and other anxiety-related disorders: an updated review of clinical features, physiological characteristics, and psychological and neurobiological mechanisms. Neuropsychopharmacology. (2020) 45:55–73. doi: 10.1038/s41386-019-0486-5
11. Bartoli F, Carrà G, Crocamo C, Carretta D, Clerici M. Metabolic syndrome in people suffering from posttraumatic stress disorder: a systematic review and meta-analysis. Metab Syndr Relat Disord. (2013) 11:301–8. doi: 10.1089/met.2013.0010
12. Aoyama K, Nakaki T. Impaired glutathione synthesis in neurodegeneration. Int J Mol Sci. (2013) 14:21021–44. doi: 10.3390/ijms141021021
13. Hori H, Kim Y. Inflammation and posttraumatic stress disorder. Psychiatry Clin Neurosci. (2019) 73:143–53. doi: 10.1111/pcn.12820
14. Shih AY, Erb H, Sun X, Toda S, Kalivas PW, Murphy TH. Cystine/glutamate exchange modulates glutathione supply for neuroprotection from oxidative stress and cell proliferation. J Neurosci. (2006) 26:10514–23. doi: 10.1523/JNEUROSCI.3178-06.2006
15. Miller MW, Sadeh N. Traumatic stress, oxidative stress and post-traumatic stress disorder: neurodegeneration and the accelerated-aging hypothesis. Mol Psychiatry. (2014) 19:1156–62. doi: 10.1038/mp.2014.111
16. Alquraan L, Alzoubi KH, Hammad H, Rababa'h SY, Mayyas F. Omega-3 fatty acids prevent post-traumatic stress disorder-induced memory impairment. Biomolecules. (2019) 9:100. doi: 10.3390/biom9030100
17. Alzoubi KH, Rababa'h AM, Al Yacoub ON. Tempol prevents post-traumatic stress disorder induced memory impairment. Physiol Behav. (2018) 184:189–95. doi: 10.1016/j.physbeh.2017.12.002
18. Fekri K, Mahmoudi J, Sadigh-Eteghad S, Farajdokht F, Nayebi AM. Coumestrol alleviates oxidative stress, apoptosis and cognitive impairments through hippocampal estrogen receptor-beta in male mouse model of chronic restraint stress. Pharm Sci. (2021) 28:260–74. doi: 10.34172/PS.2021.44
19. Fontella FU, Siqueira IR, Vasconcellos AP, Tabajara AS, Netto CA, Dalmaz C. Repeated restraint stress induces oxidative damage in rat hippocampus. Neurochem Res. (2005) 30:105–11. doi: 10.1007/s11064-004-9691-6
20. Mejia-Carmona GE, Gosselink KL, Pérez-Ishiwara G, Martínez-Martínez A. Oxidant/antioxidant effects of chronic exposure to predator odor in prefrontal cortex, amygdala, and hypothalamus. Mol Cell Biochem. (2015) 406:121–9. doi: 10.1007/s11010-015-2430-2
21. Petrovic R, Puskas L, Jevtic Dozudic G, Stojkovic T, Velimirovic M, Nikolic T, et al. NADPH oxidase and redox status in amygdala, hippocampus and cortex of male Wistar rats in an animal model of post-traumatic stress disorder. Stress. (2018) 21:494–502. doi: 10.1080/10253890.2018.1474874
22. Kalpana T, Ramya Sree A, Kumar BN. Resveratrol reverses brain glutathione system involved neuronal loss after immobilization stress. Int J Anat Res. (2019) 7:6691–700. doi: 10.16965/ijar.2019.160
23. Madrigal JL, Olivenza R, Moro MA, Lizasoain I, Lorenzo P, Rodrigo J, et al. Glutathione depletion, lipid peroxidation and mitochondrial dysfunction are induced by chronic stress in rat brain. Neuropsychopharmacology. (2001) 24:420–9. doi: 10.1016/S0893-133X(00)00208-6
24. Su YA, Wu J, Zhang L, Zhang Q, Su DM, He P, et al. Dysregulated mitochondrial genes and networks with drug targets in postmortem brain of patients with posttraumatic stress disorder (PTSD) revealed by human mitochondria-focused cDNA microarrays. Int J Biol Sci. (2008) 4:223–35. doi: 10.7150/ijbs.4.223
25. Reed EC, Case AJ. Defining the nuanced nature of redox biomarkers in post-traumatic stress disorder. Front Physiol. (2023) 14:455. doi: 10.3389/fphys.2023.1130861
26. Peruzzolo TL, Pinto JV, Roza TH, Shintani AO, Anzolin AP, Gnielka V, et al. Inflammatory and oxidative stress markers in post-traumatic stress disorder: a systematic review and meta-analysis. Mol Psychiatry. (2022) 27:3150–63. doi: 10.1038/s41380-022-01564-0
27. Cabral-Pacheco GA, Garza-Veloz I, Castruita-De la Rosa C, Ramirez-Acuna JM, Perez-Romero BA, Guerrero-Rodriguez JF, et al. The roles of matrix metalloproteinases and their inhibitors in human diseases. Int J Mol Sci. (2020) 21:9739. doi: 10.3390/ijms21249739
28. Chevalier CM, Krampert L, Schreckenbach M, Schubert CF, Reich J, Novak B, et al. MMP9 mRNA is a potential diagnostic and treatment monitoring marker for PTSD: evidence from mice and humans. Eur Neuropsychopharmacol. (2021) 51:20–32. doi: 10.1016/j.euroneuro.2021.04.014
29. Ganguly K, Rejmak E, Mikosz M, Nikolaev E, Knapska E, Kaczmarek L. Matrix metalloproteinase (MMP) 9 transcription in mouse brain induced by fear learning. J Biol Chem. (2013) 288:20978–91. doi: 10.1074/jbc.M113.457903
30. Nagy V, Bozdagi O, Matynia A, Balcerzyk M, Okulski P, Dzwonek J, et al. Matrix metalloproteinase-9 is required for hippocampal late-phase long-term potentiation and memory. J Neurosci. (2006) 26:1923–34. doi: 10.1523/JNEUROSCI.4359-05.2006
31. Jaworski DM, Boone J, Caterina J, Soloway P, Falls WA. Prepulse inhibition and fear-potentiated startle are altered in tissue inhibitor of metalloproteinase-2 (TIMP-2) knockout mice. Brain Res. (2005) 1051:81–9. doi: 10.1016/j.brainres.2005.05.057
32. Koyuncuoglu T, Sevim H, Çetrez N, Meral Z, Gönenç B, Kuntsal Dertsiz E, et al. High intensity interval training protects from Post Traumatic Stress Disorder induced cognitive impairment. Behav Brain Res. (2021) 397:112923. doi: 10.1016/j.bbr.2020.112923
33. Vaccarino V, Brennan ML, Miller AH, Bremner JD, Ritchie JC, Lindau F, et al. Association of major depressive disorder with serum myeloperoxidase and other markers of inflammation: a twin study. Biol Psychiatry. (2008) 64:476–83. doi: 10.1016/j.biopsych.2008.04.023
34. Khan AA, Alsahli MA, Rahmani AH. Myeloperoxidase as an active disease biomarker: recent biochemical and pathological perspectives. Med Sci. (2018) 6:33. doi: 10.3390/medsci6020033
35. Kocael A, Inal BB, Guntas G, Kelten C, Inal H, Topac HI, et al. Evaluation of matrix metalloproteinase, myeloperoxidase, and oxidative damage in mesenteric ischemia-reperfusion injury. Hum Exp Toxicol. (2016) 35:851–60. doi: 10.1177/0960327115607946
36. First MB, Spitzer RL, Gibbon M, Williams JB. Structured Clinical Interview for DSM-IV Axis I Disorders-Patient Edition (SCID-I/P, Version 2.0). New York, NY: Biometrics Research Department, New York State Psychiatric Institute (1995). doi: 10.1037/t07827-000
37. Spitzer RL, Kroenke K, Williams JB, Löwe B. A brief measure for assessing generalized anxiety disorder: the GAD-7. Arch Intern Med. (2006) 166:1092–7. doi: 10.1001/archinte.166.10.1092
38. Kroenke K, Spitzer RL. The PHQ-9: a new depression diagnostic and severity measure. Psychiatr Ann. (2002) 32:509–15. doi: 10.3928/0048-5713-20020901-06
39. Beck AT, Ward CH, Mendelson M, Mock J, Erbaugh J. An inventory for measuring depression. Arch Gen Psychiatry. (1961) 4:561–71. doi: 10.1001/archpsyc.1961.01710120031004
40. Blake DD, Weathers FW, Nagy LM, Kaloupek DG, Gusman FD, Charney DS, et al. The development of a clinician-administered PTSD scale. J Traumat Stress. (1995) 8:75–90. doi: 10.1002/jts.2490080106
41. Weathers FW, Litz BT, Herman DS, Huska JA, Keane TM, editors. The PTSD Checklist (PCL): Reliability, Validity, and Diagnostic Utility. Annual Convention of the International Society for Traumatic Stress Studies, Vol. 462. San Antonio, TX (1993).
42. Kubany ES, Haynes SN, Leisen MB, Owens JA, Kaplan AS, Watson SB, et al. Development and preliminary validation of a brief broad-spectrum measure of trauma exposure: the Traumatic Life Events Questionnaire. Psychol Assess. (2000) 12:210–24. doi: 10.1037/1040-3590.12.2.210
43. Mescher M, Merkle H, Kirsch J, Garwood M, Gruetter R. Simultaneous in vivo spectral editing and water suppression. NMR Biomed. (1998) 11:266–72. doi: 10.1002/(SICI)1099-1492(199810)11:6<266::AID-NBM530>3.0.CO;2-J
44. Watling SE, Jagasar S, McCluskey T, Warsh J, Rhind SG, Truong P, et al. Imaging oxidative stress in brains of chronic methamphetamine users: a combined 1H-magnetic resonance spectroscopy and peripheral blood biomarker study. Front Psychiatry. (2022) 13:1070456. doi: 10.3389/fpsyt.2022.1070456
45. Shungu DC, Weiduschat N, Murrough JW, Mao X, Pillemer S, Dyke JP, et al. Increased ventricular lactate in chronic fatigue syndrome. III. Relationships to cortical glutathione and clinical symptoms implicate oxidative stress in disorder pathophysiology. NMR Biomed. (2012) 25:1073–87. doi: 10.1002/nbm.2772
46. Wright SM, Wald LL. Theory and application of array coils in MR spectroscopy. NMR Biomed. (1997) 10:394–410. doi: 10.1002/(SICI)1099-1492(199712)10:8<394::AID-NBM494>3.0.CO;2-0
48. Ashburner J, Friston KJ. Unified segmentation. Neuroimage. (2005) 26:839–51. doi: 10.1016/j.neuroimage.2005.02.018
49. Edden RA, Puts NA, Harris AD, Barker PB, Evans CJ. Gannet: a batch-processing tool for the quantitative analysis of gamma-aminobutyric acid–edited MR spectroscopy spectra. J Magn Reson Imaging. (2014) 40:1445–52. doi: 10.1002/jmri.24478
51. Gill T, Watling SE, Richardson JD, McCluskey T, Tong J, Meyer JH, et al. Imaging of astrocytes in posttraumatic stress disorder: a PET study with the monoamine oxidase B radioligand. Eur Neuropsychopharmacol. (2022) 54:54–61. doi: 10.1016/j.euroneuro.2021.10.006
52. Di Battista AP, Rhind SG, Richards D, Hutchison MG. An investigation of plasma interleukin-6 in sport-related concussion. PLoS ONE. (2020) 15:e0232053. doi: 10.1371/journal.pone.0232053
53. Sanaei Nezhad F, Anton A, Parkes LM, Deakin B, Williams SR. Quantification of glutathione in the human brain by MR spectroscopy at 3 Tesla: comparison of PRESS and MEGA-PRESS. Magn Reson Med. (2017) 78:1257–66. doi: 10.1002/mrm.26532
54. Da Silva T, Hafizi S, Andreazza AC, Kiang M, Bagby RM, Navas E, et al. Glutathione, the major redox regulator, in the prefrontal cortex of individuals at clinical high risk for psychosis. Int J Neuropsychopharmacol. (2018) 21:311–8. doi: 10.1093/ijnp/pyx094
55. Abdel-Salam OM, Abdel-Rahman RF, Gaafar AE-DM. Behavioral and biochemical effects of Cannabis Sativa and their modulation by antidepressant drugs. Rev Latinoamericana Química. (2013) 41:21–37.
56. Graczyk M, Lewandowska AA, Dzierzanowski T. The therapeutic potential of cannabis in counteracting oxidative stress and inflammation. Molecules. (2021) 26:4551. doi: 10.3390/molecules26154551
57. Joyce JM, Mercier LJ, Stokoe M, La PL, Bell T, Batycky JM, et al. Glutamate, GABA and glutathione in adults with persistent post-concussive symptoms. Neuroimage Clin. (2022) 36:103152. doi: 10.1016/j.nicl.2022.103152
58. Koerte IK, Lin AP, Muehlmann M, Merugumala S, Liao H, Starr T, et al. Altered neurochemistry in former professional soccer players without a history of concussion. J Neurotrauma. (2015) 32:1287–93. doi: 10.1089/neu.2014.3715
59. Clark AL, Merritt VC, Bigler ED, Bangen KJ, Werhane M, Sorg SF, et al. Blast-exposed veterans with mild traumatic brain injury show greater frontal cortical thinning and poorer executive functioning. Front Neurol. (2018) 9:873. doi: 10.3389/fneur.2018.00873
60. Witt ST, Lovejoy DW, Pearlson GD, Stevens MC. Decreased prefrontal cortex activity in mild traumatic brain injury during performance of an auditory oddball task. Brain Imaging Behav. (2010) 4:232–47. doi: 10.1007/s11682-010-9102-3
61. Bigler ED, Maxwell WL. Neuropathology of mild traumatic brain injury: relationship to neuroimaging findings. Brain Imaging Behav. (2012) 6:108–36. doi: 10.1007/s11682-011-9145-0
63. Bobińska K, Szemraj J, Gałecki P, Talarowska M. The role of MMP genes in recurrent depressive disorders and cognitive functions. Acta Neuropsychiatr. (2016) 28:221–31. doi: 10.1017/neu.2015.72
64. Chen Q, Wei Z, Wang L, Xu X, Wei Z, Zheng P, et al. Dry eye disease in patients with schizophrenia: a case-control study. Front Med. (2022) 9:831337. doi: 10.3389/fmed.2022.831337
65. Li H, Sheng Z, Khan S, Zhang R, Liu Y, Zhang Y, et al. Matrix Metalloproteinase-9 as an important contributor to the pathophysiology of Depression. Front Neurol. (2022) 13:861843. doi: 10.3389/fneur.2022.861843
66. Kucharczyk M, Kurek A, Detka J, Slusarczyk J, Papp M, Tota K, et al. Chronic mild stress influences nerve growth factor through a matrix metalloproteinase-dependent mechanism. Psychoneuroendocrinology. (2016) 66:11–21. doi: 10.1016/j.psyneuen.2015.12.019
67. Maolood N, Hardin-Pouzet H, Grange-Messent V. Matrix metalloproteinases MMP2 and MMP9 are upregulated by noradrenaline in the mouse neuroendocrine hypothalamus. Europ J Neurosci. (2008) 27:1143–52. doi: 10.1111/j.1460-9568.2008.06099.x
68. Haorah J, Ramirez SH, Schall K, Smith D, Pandya R, Persidsky Y. Oxidative stress activates protein tyrosine kinase and matrix metalloproteinases leading to blood-brain barrier dysfunction. J Neurochem. (2007) 101:566–76. doi: 10.1111/j.1471-4159.2006.04393.x
69. Hardy E, Hardy-Sosa A, Fernandez-Patron C. MMP-2: is too low as bad as too high in the cardiovascular system? Am J Physiol Heart Circ Physiol. (2018) 315:H1332–40. doi: 10.1152/ajpheart.00198.2018
70. Ryder AL, Azcarate PM, Cohen BE. PTSD and physical health. Curr Psychiatry Rep. (2018) 20:116. doi: 10.1007/s11920-018-0977-9
71. Selek S, Altindag A, Saracoglu G, Aksoy N. Oxidative markers of Myeloperoxidase and Catalase and their diagnostic performance in bipolar disorder. J Affect Disord. (2015) 181:92–5. doi: 10.1016/j.jad.2015.03.058
72. Rybakowski JK, Remlinger-Molenda A, Czech-Kucharska A, Wojcicka M, Michalak M, Losy J. Increased serum matrix metalloproteinase-9 (MMP-9) levels in young patients during bipolar depression. J Affect Disord. (2013) 146:286–9. doi: 10.1016/j.jad.2012.07.019
73. Arnhold J. The dual role of myeloperoxidase in immune response. Int J Mol Sci. (2020) 21:8057. doi: 10.3390/ijms21218057
74. Ray RS, Katyal A. Myeloperoxidase: bridging the gap in neurodegeneration. Neurosci Biobehav Rev. (2016) 68:611–20. doi: 10.1016/j.neubiorev.2016.06.031
75. Amer J, Fibach E. Chronic oxidative stress reduces the respiratory burst response of neutrophils from beta-thalassaemia patients. Br J Haematol. (2005) 129:435–41. doi: 10.1111/j.1365-2141.2005.05463.x
76. Gisabella B, Babu J, Valeri J, Rexrode L, Pantazopoulos H. Sleep and memory consolidation dysfunction in psychiatric disorders: evidence for the involvement of extracellular matrix molecules. Front Neurosci. (2021) 15:646678. doi: 10.3389/fnins.2021.646678
77. Brown TE, Wilson AR, Cocking DL, Sorg BA. Inhibition of matrix metalloproteinase activity disrupts reconsolidation but not consolidation of a fear memory. Neurobiol Learn Mem. (2009) 91:66–72. doi: 10.1016/j.nlm.2008.09.003
78. Bach DR, Tzovara A, Vunder J. Blocking human fear memory with the matrix metalloproteinase inhibitor doxycycline. Mol Psychiatry. (2018) 23:1584–9. doi: 10.1038/mp.2017.65
79. Lutgendorf SK, Lamkin DM, Jennings NB, Arevalo JM, Penedo F, DeGeest K, et al. Biobehavioral influences on matrix metalloproteinase expression in ovarian carcinoma. Clin Cancer Res. (2008) 14:6839–46. doi: 10.1158/1078-0432.CCR-08-0230
80. Jourquin J, Tremblay E, Bernard A, Charton G, Chaillan FA, Marchetti E, et al. Tissue inhibitor of metalloproteinases-1 (TIMP-1) modulates neuronal death, axonal plasticity, and learning and memory. Eur J Neurosci. (2005) 22:2569–78. doi: 10.1111/j.1460-9568.2005.04426.x
81. Saha P, Sarkar S, Paidi RK, Biswas SC. TIMP-1: a key cytokine released from activated astrocytes protects neurons and ameliorates cognitive behaviours in a rodent model of Alzheimer's disease. Brain Behav Immunity. (2020) 87:804–19. doi: 10.1016/j.bbi.2020.03.014
82. Beroun A, Mitra S, Michaluk P, Pijet B, Stefaniuk M, Kaczmarek L. MMPs in learning and memory and neuropsychiatric disorders. Cell Mol Life Sci. (2019) 76:3207–28. doi: 10.1007/s00018-019-03180-8
83. Wijtenburg SA, Near J, Korenic SA, Gaston FE, Chen H, Mikkelsen M, et al. Comparing the reproducibility of commonly used magnetic resonance spectroscopy techniques to quantify cerebral glutathione. J Magn Reson Imaging. (2019) 49:176–83. doi: 10.1002/jmri.26046
Keywords: magnetic resonance spectroscopy, glutathione, metalloproteinase (MMP), myeloperoxidase (MPO), psychiatric disorder, posttraumatic stress disorder (PTSD)
Citation: Watling SE, Rhind SG, Warsh J, Green D, McCluskey T, Tong J, Truong P, Chavez S, Richardson JD, Kish SJ and Boileau I (2023) Exploring brain glutathione and peripheral blood markers in posttraumatic stress disorder: a combined [1H]MRS and peripheral blood study. Front. Psychiatry 14:1195012. doi: 10.3389/fpsyt.2023.1195012
Received: 27 March 2023; Accepted: 09 May 2023;
Published: 02 June 2023.
Edited by:
Jeffrey A. Stanley, Wayne State University, United StatesReviewed by:
Gabriele Ende, University of Heidelberg, GermanyKim M. Cecil, Cincinnati Children's Hospital Medical Center, United States
Copyright © 2023 Watling, Rhind, Warsh, Green, McCluskey, Tong, Truong, Chavez, Richardson, Kish and Boileau. This is an open-access article distributed under the terms of the Creative Commons Attribution License (CC BY). The use, distribution or reproduction in other forums is permitted, provided the original author(s) and the copyright owner(s) are credited and that the original publication in this journal is cited, in accordance with accepted academic practice. No use, distribution or reproduction is permitted which does not comply with these terms.
*Correspondence: Isabelle Boileau, aXNhYmVsbGUuYm9pbGVhdUBjYW1oLmNh